the Creative Commons Attribution 4.0 License.
the Creative Commons Attribution 4.0 License.
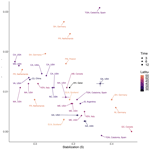
Decomposing the Tea Bag Index and finding slower organic matter loss rates at higher elevations and deeper soil horizons in a minerogenic salt marsh
Satyatejas G. Reddy
W. Reilly Farrell
Fengrun Wu
Steven C. Pennings
Jonathan Sanderman
Meagan Eagle
Christopher Craft
Environmental gradients can affect organic matter decay within and across wetlands and contribute to spatial heterogeneity in soil carbon stocks. We tested the sensitivity of decay rates to tidal flooding and soil depth in a minerogenic salt marsh using the Tea Bag Index (TBI). Tea bags were buried at 10 and 50 cm depths across an elevation gradient in a subtropical Spartina alterniflora marsh in Georgia (USA). Plant and animal communities and soil properties were characterized once, while replicate tea bags and porewaters were collected several times over 1 year. TBI decay rates were faster than prior litterbag studies in the same marsh, largely due to rapid green tea loss. Rooibos tea decay rates were more comparable to natural marsh litter, potentially suggesting that is more useful as a standardized organic matter proxy than green tea. Decay was slowest at higher marsh elevations and not consistently related to other biotic (e.g., plants, crab burrows) or abiotic factors (e.g., porewater chemistry), indicating that local hydrology strongly affected organic matter loss rates. TBI rates were 32 %–118 % faster in the 10 cm horizon than at 50 cm. Rates were fastest in the first 3 months and slowed 54 %–60 % at both depths between 3 and 6 months. Rates slowed further between 6 and 12 months, but this was more muted at 10 cm (17 %) compared to 50 cm (50 %). Slower rates at depth and with time were unlikely due to the TBI stabilization factor, which was similar across depths and decreased from 6 to 12 months. Slower decay at 50 cm demonstrates that rates were constrained by environmental conditions in the deeper horizon rather than the composition of this highly standardized litter. Overall, these patterns suggest that hydrological setting, which affects oxidant introduction and reactant removal and is often overlooked in marsh decomposition studies, may be a particularly important control on organic matter loss in the short term (3–12 months).
- Article
(3137 KB) - Full-text XML
-
Supplement
(528 KB) - BibTeX
- EndNote
Long-term sustainability of salt marshes and their role as a carbon sink depend on efficient preservation of organic matter. Preservation is generally ascribed to a combination of rapid deposition of refractory organic matter and slow decay in anoxic soils (Benner et al., 1991; Morris and Bowden, 1986; Valiela et al., 1985). Yet variability in soil carbon stocks and accumulation rates within and across marshes indicates that controls on preservation are more complex (Arriola and Cable, 2017; Holmquist et al., 2018). Differential tidal flooding across marsh elevations affects aboveground plant production and the belowground soil environment that, in turn, constrains microbial access to oxidants and organic matter (Guimond and Tamborski, 2021; Morris and Bowden, 1986; Spivak et al., 2019). In surface horizons, marsh plant roots and animal burrows further alter soil structure and chemical gradients that affect organic matter decay, while deeper horizons are generally more stable environments (Gribsholt and Kristensen, 2002; Guimond et al., 2020). Characterizing patterns in organic matter decay across tidal inundation gradients and soil depths may therefore provide a useful framework to assess processes contributing to marsh-scale spatial variability in carbon stocks.
Tidal flooding effects on plant and soil processes are generally strongest along creek banks and at lower elevations, which are inundated more frequently and for longer periods, relative to interior and higher-elevation areas (Guimond and Tamborski, 2021; Howes and Goehringer, 1994; Reed and Cahoon, 1992). Rising and falling tides result in oscillating soil redox conditions, with greater oxygen penetration during emergent periods and more strongly reducing conditions under submergence (Fettrow et al., 2023; Seyfferth et al., 2020; Spivak et al., 2023). Flooding changes the soil environment for decomposition by altering availability of terminal electron acceptors, increasing pore space connectivity, leaching organic matter, and changing microbial access to bioavailable compounds (e.g., sorption, enzyme functionality, molecular configuration) (Bradley and Morris, 1990; Giblin and Howarth, 1984; Liu and Lee, 2006; Morrissey et al., 2014).
Plant and animal effects on soil decomposition are layered on top of this hydrogeomorphic template. The classic parabolic relationship between flooding and plant productivity suggests there is an optimal flooding regime, where productivity and, presumably, root release of oxygen and bioavailable carbon compounds (hereafter, exudates) peak, while elevations with greater or lesser flooding receive fewer plant inputs into the rhizosphere to fuel soil microbes (Megonigal et al., 1999; Morris et al., 2002; Mueller et al., 2016; Spivak and Reeve, 2015). The physical properties of surficial soils are also altered by roots and animal burrows, which reduce bulk density and allow for greater infiltration of oxygen to anoxic horizons. Bioturbation by animals can strongly affect decay rates in surface soil horizons, by altering redox conditions, producing excreta, and moving organic matter between oxic and anoxic layers (Kostka et al., 2002b; Kristensen et al., 2012). Soils buried below animal burrows and the active rooting zone are more insulated from inputs of oxygen and root exudates and daily tidal oscillations, typically have higher bulk densities, and are more compacted (Turner et al., 2006). This more stable soil environment is likely a key reason that decomposition slows with depth, but attributing causality is complicated by differences in organic matter composition between surface and deeper horizons (Bulseco et al., 2020; Luk et al., 2021; Yousefi Lalimi et al., 2018). Standardized litter approaches offer ways to test controls on organic matter loss across ecological, geomorphic, and spatial gradients while avoiding potential confounding factors of litter composition and preparation.
Decades of field, lab, and theoretical experiments have reported a wide range of decay rates, but robust relationships with climatic, landscape, and ecological drivers remain difficult to quantify (Charles and Dukes, 2009; Kirwan et al., 2013, 2014; Kirwan and Blum, 2011; Mueller et al., 2016; Noyce et al., 2023; Tang et al., 2023). The diversity of decay rates likely reflects spatial heterogeneity within wetlands as well as the variety of measurement techniques applied over different timescales (Blum, 1993; Kirby and Gosselink, 1976; Luk et al., 2023; Luk et al., 2021; Newell et al., 1989). Litterbags have the advantage of assessing mass loss of local marsh detritus, but this bulk approach lacks sensitivity, and results can be difficult to compare across studies due to differences in the use of aboveground and/or belowground material and deployment duration and depth, among other factors (Blum and Christian, 2004; Charles and Dukes, 2009; Christian, 1984; Kirwan and Blum, 2011; Windham, 2001). Geochemical approaches describe organic matter loss and transformations (e.g., C content, stable isotopes, thermal reactivity, biomarkers), can be applied over timescales of seasons to centuries (e.g., radiocarbon), and benefit from multiple proxies, but they are resource-intensive and require specialized instrumentation (e.g., mass spectrometry) (Benner et al., 1984a, b, 1987, 1991; Duddigan et al., 2020; Luk et al., 2021, 2023; Moran et al., 1989).
The Tea Bag Index (TBI) is an alternative approach that has been widely adopted because the standardized method and materials allow for greater comparability between studies; it is cost-effective, does not require specialized instrumentation, and can be accomplished within months by nonexperts (Keuskamp et al., 2013; Mueller et al., 2018). However, like all methods, the TBI rests on assumptions. One key assumption is that the decay dynamics and chemical composition of two different litter types (green and rooibos teas) can be integrated to estimate loss of natural detritus that has characteristics of the proxy constituents. The TBI, in effect, applies a simplified two-pool decay model and assumes that the biochemical compositions of both pools are broadly applicable. An assumption of this model is that the decay dynamics of very specific types of terrestrial organic matter (i.e., rooibos and green teas) reasonably approximate those of local plant detritus. Chemical characterization of green and rooibos teas during a 91 d incubation described changes in carbon functional groups that are consistent with decomposition and not dissimilar to natural litter, supporting the TBI approach (Duddigan et al., 2020). However, green tea is rich in tannins, which are lost rapidly (Duddigan et al., 2020), raising the question of whether the mechanism is leaching, microbial decomposition, or some combination (Lind et al., 2022). Consequently, comparing decay rates estimated from the TBI with natural local litter is important for determining the applicability of this approach to the target study system.
Here, we aimed to gain insight into spatial and temporal patterns in decomposition by testing how tidal inundation affects organic matter decay rates across soil depths and over time in a salt marsh. We employed the TBI to examine the effects of these environmental gradients on decay without the potential confounding factor of varying organic matter composition. Recognizing potential limitations of the TBI, we extended the prescribed incubation time from 3 to 12 months and compared TBI decay estimates with mass loss rates of rooibos and green teas and of local plant detritus. We predicted that TBI decay rates would be fastest in shallower soil horizons and lower marsh elevations, where porewater flushing is greater and tidal flooding is more frequent, respectively, and positively correlated with plant biomass, bioturbation (as crab burrow density), and porewater redox levels. We further predicted that TBI rates would be fastest in the first 3 months and then decrease over the following 9 months and that this pattern would be more pronounced in shallower compared to deeper horizons. Lastly, we expected that decay rates of rooibos tea and local detritus to be comparable and slower than TBI rates.
2.1 Study site and design
We tested whether TBI decay rates differ within vs. below the rhizosphere of Spartina alterniflora marshes within the Georgia Coastal Ecosystems Long Term Ecological Research (GCE-LTER) domain (31.421°, −81.290°). Total suspended sediment levels are high in the Altamaha River, which feeds into the GCE-LTER domain and contributes to salt marsh vertical accretion (Langston et al., 2021; Mariotti et al., 2024). As a result, these minerogenic marshes have lower soil carbon content, porosity, and permeabilities but higher bulk densities compared to organic-rich marshes (Giblin and Howarth, 1984). Tides are semidiurnal with a ∼ 2 m range.
Study plots were established in summer 2019 along a tidal creek, with a total of 23 plots placed at 3 distances from the creek-bank edge (creek: 0 m, 7 plots; middle: 4 m, 8 plots; platform: 14 m, 8 plots) that captured a range of marsh surface elevations, from 0.55 to 1.13 m (North American Vertical Datum of 1988, NAVD 88; Fig. 1). Tea bag decay rates and porewater chemistry were measured at two depths (10 and 50 cm) at discrete intervals over 1 year (July 2019–2020). Soil temperatures were continuously monitored for ∼ 6 months at both depths. This study was conducted alongside Wu et al. (2022), who measured plot elevations, plant and animal community characteristics, and soil shear strength once during summer 2019, which we use to contextualize and interpret our results.
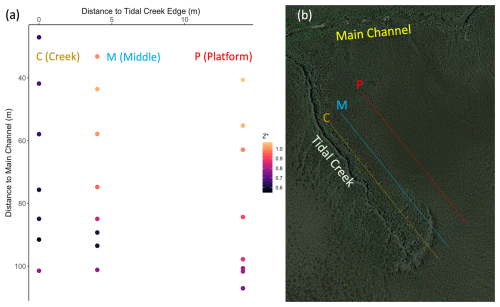
Figure 1(a) Study plot orientation relative to the main channel, tidal creek, and relative marsh surface elevation (Z*; see Eq. 1). Plots were distributed along the tidal creek (C, 0 m), in the marsh platform (P, 14 m), or in between (M, 4 m) across an elevation range of 0.55–1.13 m (NAVD 88). Plot color corresponds to Z* or relative position in the tidal frame (m). Spacing between plots reflects Wu et al.'s (2022) goal of capturing marsh processes around the fan of a headward eroding creek. (b) Aerial photograph of study site (© Google Earth 2024) with demarcated lines showing approximate plot distribution with respect to the headward eroding creek. Exact plot locations are described by Wu et al. (2022). Plots affected by the headward eroding creek were excluded from this study.
2.2 Marsh surface elevation
Marsh surface elevations were measured within 2 m of each plot, to minimize trampling, using a Trimble R6 real-time kinematic global positioning system receiver (RTK-GPS; Table S1). The RTK-GPS has a vertical error of ∼ 20 mm. Relative marsh surface elevation of each plot within the tide frame (Z*) was calculated as
where MSL is mean sea level and MHHW is mean highest high water, referenced to the nearest NOAA tide station (Fort Pulaski, GA, 8670870). Elevation data were compared with tide heights, recorded in a nearby creek (31.4437673, −81.2838603), to distinguish between periods of tidal inundation and exposure. Tide heights were recorded in 5 min intervals by a titanium pressure transducer (Campbell Scientific™ CS456) deployed at a verified elevation and operated by the GCE-LTER project.
2.3 Temperature
Soil temperatures at 10 and 50 cm depths were recorded by HOBO loggers (UA-002-08, Onset Computer Corp, accuracy: ± 0.53 °C from 0 to 50 °C) in 15 min intervals. Loggers were intercalibrated prior to deployment (SE ± 0.07 °C). The loggers were deployed in July 2019 and collected 188 d (∼ 6 months) later in January 2020. We calculated the average, minimum, and maximum daily temperatures for each of the 15 loggers deployed at 10 cm and the 16 loggers deployed at 50 cm that were recovered and functioning.
2.4 Porewater chemistry
We collected samples for porewater salinity, pH, and redox in each plot using passive sippers with collection windows at 10 and 50 cm that were deployed in July 2019 (Hughes et al., 2012; Paludan and Morris, 1999). Glass scintillation vials, filled with Milli-Q water (18.2 MΩ) and fitted with open top caps and 50 m Nitex mesh, were placed upside down in each collection window. Porewater vials were retrieved 2 months later and again at 98, 188, and 363 d, which correspond with the 3- , 6-, and 12-month tea bag collections. Collected vials were replaced in the sippers with fresh vials and Milli-Q water. Samples were sealed with solid caps and transported on ice to the University of Georgia Marine Institute for measurement. This sampling approach relies on equilibration of water inside the vial with the surrounding porewater, which happens within 1 month and was assessed using salinity readings. Salinity was measured with a handheld refractometer, while pH levels and redox potential were measured with a benchtop dual-channel pH/ISE meter (Fisherbrand™ Accumet™ XL250, accuracy ± 0.002 pH units) and a calibrated pH combination electrode (Fisherbrand™ accuTupH™) or redox oxidation–reduction potential electrode (Mettler Toledo™InLab™Redox ORP Electrode), respectively. Redox potential readings (mV) were recorded relative to a reference electrode in a 3.5 M potassium chloride solution, and values were subsequently corrected to the standard hydrogen electrode.
2.5 Plants, animals, and soil stiffness
Plant characteristics, animal abundances, and soil stiffness were reported previously by Wu et al. (2022). Soil shear strength was measured in the top 4 cm using a field shear vane (GEONOR H-6O). Spartina alterniflora aboveground biomass was estimated based on stem density counts and known masses of representative stems. Belowground biomass was measured by collecting soil cores (10 cm diameter, 30 cm depth) centered on a culm of S. alterniflora in each plot and then washing roots and rhizomes free of soil before drying and weighing. Two major groups of invertebrates were present: crabs (Uca pugilator, Sesarma reticulatum, Panopeus) and snails (Littoraria irrorata). The densities of burrows (> 0.5 cm diameter, all species pooled) and snails (> 0.3 cm spire height) were recorded in 0.5 × 0.5 m quadrats at each plot as individuals per square meter.
2.6 Decay rates
Decay coefficients (hereafter, rates) were approximated by measuring mass loss over time of a standardized litter. We chose the TBI because this approach has been used broadly across ecosystem types, allowing for intercomparisons (Keuskamp et al., 2013). This method, introduced by Keuskamp et al. (2013), assumes that natural litter is comprised of labile and refractory pools that can be represented by Lipton™ green (European Article Number: 87 22700 05552 5) and rooibos (European Article Number: 87 22700 18843 8) teas, respectively, which we used here. Tea bags were dried at 60 °C to constant mass prior to deployment, during which triplicate bags of each tea type were buried in every plot at 10 and 50 cm depth in July 2019 (initial tea weights: rooibos: 2.01± 0.004 g; green: 2.17± 0.004 g). Burial depths were chosen to assess decay rates within the more dynamic rhizosphere (10 cm) compared to more stable deeper horizons (50 cm). Single replicates were collected after 98 d (∼ 3 months), 188 d (∼ 6 months), and 363 d (∼ 12 months, July 2020). Collected tea bags were dried at 60 °C, and mass loss was calculated as the difference between the dried initial and final tea masses, after correcting for contributions from the tea bag, string, and label. However, we could not recover all tea bags or use all bags that were recovered due to root ingrowth or other disturbances to the integrity of the bags. Such losses were small: fewer than 10 tea bags across all 3 times (3, 6, and 12 months) and depths (10 and 50 cm) were lost and therefore excluded.
Decay rates were calculated per Keuskamp et al. (2013) using four equations:
Equation (2) combines decay of labile (k1) and refractory (k2) organic matter and requires time series data. The TBI eliminates the need for a time series by simplifying Eqs. (2) to (3) using the assumptions that decay rates of refractory organic matter are negligible (i.e., k2=0) and that the decomposable fraction of organic matter (i.e., a) can be represented by combining different characteristics of rooibos and green teas. In Eq. (3), W(t) is the mass fraction of rooibos tea remaining at time t, k is the decay coefficient, and S is a stabilization factor. The inhibitory effect of environmental conditions on decay (i.e., S) is calculated based on green tea but assumed to be the same for both tea types. The decomposable fraction (a) of green tea (ag) is estimated by the mass fraction lost, while that of rooibos tea (ar) is based on its hydrolysable fraction (Hr) and S. We used the tea-specific H values reported by Keuskamp et al. (2013) that were calculated as the sum of nonpolar extractable, water-soluble, and acid-soluble fractions (Hr: rooibos, 0.552 g g−1; Hg: green, 0.842 g g−1).
We then compared TBI decay rates with tea-specific rates estimated from a first-order decay model, which requires time series data:
where a is the tea mass fraction remaining after a given amount of time (t), ao is the initial mass fraction (i.e., 1), and k is the calculated decay coefficient. Single exponential models were fitted to tea-specific mass loss fractions at 0, 98, 188, and 363 d and included 2, 3, or 4 time points, respectively, producing kg and kr (Fig. S1). The fraction of variance explained by the decay models was generally greater for green tea than rooibos. We suspect this is because mass loss rates are a fairly insensitive metric and were much slower for rooibos than green tea. Only models with r2 values > 0.60 were included for the 6- and 12-month time points to be as representative of the full dataset as possible (i.e., 61 % and 100 % for the rooibos and green tea bags, respectively). Outliers were removed prior to fitting Eq. (5) for the 3-month tea bags (see Sect. 2.7). To further assess assumptions of the TBI approach, we calculated tea-specific decomposable fractions (ag and ar) and stabilization factors (Sg and Sr). Decomposable fractions were defined as mass lost during incubation and calculated as 1−a, resulting in ag (same as Keuskamp et al., 2013) and ar, for green and rooibos teas, respectively. We used Keuskamp et al.'s (2013) formulation of S in Eq. (3) to represent Sg but modified it for rooibos tea (Sr) by substituting ar (decomposable fraction) and Hr:
From here forward, rates and variables calculated using the approach of Keuskamp et al. (2013) or the first-order decay approach are referred to as TBI and empirical, respectively. For the TBI, this includes TBI k (Eq. 3), TBI ag (fraction mass loss of green tea), TBI ar (Eq. 5), and TBI S (Eq. 4) to denote decay rate, the decomposable fractions of green and rooibos teas, and the stabilization factor, respectively. The empirical calculations include kg and kr (Eq. 6), ag and ar (mass fractions lost of each tea type), and Sg (Eq. 4) and Sr (Eq. 7), where “g” and “r” refer to green or rooibos teas, respectively. Importantly, there are two commonalities between these approaches: TBI ag is the same as empirical ag, and TBI S is the same as empirical Sg.
2.7 Decay rates from marsh litterbags
Geochemical changes of natural marsh organic matter undergoing decomposition have been well studied in Georgia marshes (Benner et al., 1984b, 1987, 1991; Rice and Tenore, 1981). However, we were unable to find published organic matter mass loss rates from litterbags, which would be a more comparable complement to the TBI. Instead, we draw on a prior experiment conducted in June 2003–2004 that used S. alterniflora roots collected from the levee and plain of a marsh within GCE-LTER. Decay rates were measured following the methods of Blum (1993) in which 10 g of root material was placed in nylon mesh (2 mm × 2 mm) bags (30 cm × 7 cm) and buried (10 to 20 cm) for 3–12 months. Root detritus is a good proxy for evaluating soil organic matter dynamics since this material is deposited directly into anoxic horizons and contributes to soil accumulation. Sixteen replicates each were initially buried in the marsh levee and plain, and four replicates were retrieved from both sites every ∼ 3 months. Bags were transported on ice to the lab and dried at 70 °C to constant mass. Decay rates were calculated as in Eq. (6). Environmental conditions, including precipitation and temperature, were similar (p> 0.05) between the litterbag study in the summer of 2003–2004 (7.7± 1.4 cm yr−1 and 20.4± 2.1 °C) and TBI experiment in the summer of 2019–2020 (12.1± 1.9 cm yr−1 and 21.4± 2.3 °C).
2.8 Data analyses
Changes in belowground environmental conditions across marsh surface elevations, between soil depths, and over time were assessed with regression analyses and t tests. Distance category identity (C, M, P; Fig. 1) was excluded from all statistical analyses because it was confounded with marsh elevation, which we predicted would be a key factor affecting environmental conditions and decay rates. Tidal flooding effects on soil porewater chemistry and temperature were tested by constructing regression models against relative elevation (Z*). Porewater data were then aggregated by sampling event, and two sample t tests were used to detect differences between 10 and 50 cm depths. Correlations between Z* and soil temperature were further tested by partitioning according to season (summer: 18 July to 22 September 2019; fall: 23 September to 22 December 2019; winter: 23 December 2019 to 19 January 2020) and periods of tidal inundation or exposure; differences between slope coefficients were evaluated based on Clogg et al. (1995). We tested whether soil temperatures differed between depths within each season using paired t tests.
We tested whether decay rates (TBI k, empirical kg, empirical kr; d−1) and stabilization factors (TBI S, empirical Sg, empirical Sr) differed over time (3, 6, or 12 months) and between soil depths (10 vs. 50 cm) by constructing linear mixed-effect models using the nlme package for R (Pinheiro et al., 2016). The mixed models evaluated the fixed effects of time and depth and included plot number as a random factor. We then conducted paired t tests to further explore how TBI and empirical decay rates, decomposable fractions (TBI and empirical ag and ar), and stabilization factors changed over time within a depth horizon.
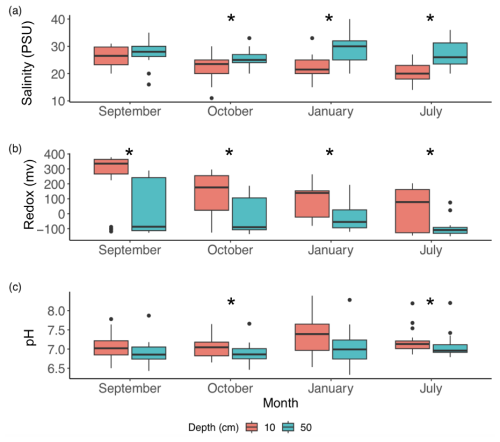
Figure 2Soil porewater salinity (a), redox (b), and pH (c) pooled across all 23 study plots at 2, 3, 6, and 12 months (September, October, January, and July, respectively) at 10 cm (red) and 50 cm (blue) depths (n=20–23 for each box at each depth). Significant differences (p<0.05, as determined by t tests; see Sect. 2.8) between depths are denoted by an asterisk (*).
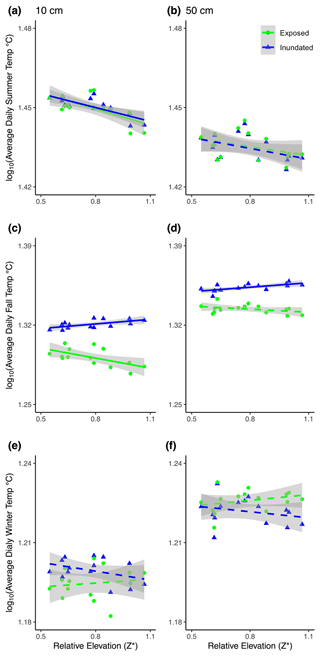
Figure 3Soil temperatures at 10 cm (a, c, e) and 50 cm (b, d, f) changed with tidal stage (inundated, blue; exposed, green) and relative mash surface elevation in the tidal frame (Z*) during summer (a, b), fall (c, d), and winter (e, f). Correlations with p<0.05 or > 0.05 are denoted by solid or dashed lines, respectively. See Table 1 for statistical results.
Potential drivers of TBI k were evaluated by calculating Spearman rank correlation coefficients between rates and environmental conditions for the three time points (3, 6, or 12 months) and two soil depths (10 and 50 cm). Porewater data for the 3, 6, and 12 month periods were combined with data from previous time points (e.g., 2, 3, or 6 months) to better represent cumulative conditions. Temperature was excluded because the shared time series with TBI k violated assumptions of independence. The TBI k values correlated strongly with relative elevation (Z*), S. alterniflora rhizome and aboveground biomass, and soil stiffness. We then evaluated interdependencies between these potential drivers by using subsequent single-factor regressions of relative elevation (Z*) vs. aboveground biomass and soil stiffness. Correlations between these variables limited further hypothesis testing of decay drivers to plot position within the tidal frame (Z*). We tested whether TBI k rates and S values changed with relative elevation (Z*) using linear regression models and then evaluated differences between the resulting slope coefficients over time and with depth, as described by Clogg et al. (1995).
Data were tested for outliers using a 1.5 interquartile range cutoff and log10 transformed as needed to meet assumptions of normality. Analyses were conducted using R software (R Development Core Team, 2023). Data are presented as means ± standard error (SE) unless noted otherwise.
3.1 Soil porewater chemistry
Porewater chemistry differed between depths and changed over the year but was only weakly sensitive to relative marsh elevation (Z*). Salinities were lower at 10 cm than 50 cm after the first 2 months of the experiment (Fig. 2a) and correlated positively with relative elevation (Z*) at 50 cm (r2=0.28, p < 0.05) but not at 10 cm (r2=0.17, p > 0.05). Redox potentials were more oxidizing in the shallower horizon and generally decreased over time at both depths but did not vary with relative elevation (Z*) at 10 cm (r2=0.06, p > 0.05) or 50 cm (r2=0.00, p > 0.05) (Fig. 2b). Porewater pH levels were similar between depths or slightly higher at 10 cm, with little change over the year (Fig. 2c) and no change with relative marsh elevation (Z*) at either 10 cm (r2=0.17, p > 0.05) or 50 cm (r2= 0.15, p > 0.05).
Temperature differences between 10 and 50 cm were slight (∼ 1 °C) and changed seasonally, with tidal inundation and relative marsh elevation (Z*) (Fig. 3a–f; Table 1). The warmest temperatures were at 10 cm during summer, but temperatures were higher at 50 cm in the fall and winter (Table 1a). Soil temperatures were similar across tidal stages in the summer and winter but were warmer during periods of inundation in the fall. Temperatures at 10 cm decreased with increasing relative marsh elevation (Z*) in the summer, regardless of tidal stage, and the fall but only during periods of exposure (Fig. 3a, c, f; Table 1b). In contrast, temperatures at 50 cm were less sensitive to marsh elevation within the tidal frame.
Table 1Soil temperatures by depth and season and with relative position in the tidal frame (Z*). (a) Average (± SE) temperatures (°C) by depth and season and during periods of tidal inundation or exposure. Significant differences between tidal stages within a season are denoted by * (p<0.05) or (p<0.01) as determined by t tests; see Sect. 2.8. (b) Linear regression models tested temperature changes with relative marsh surface elevation (Z*) over tidal stages and seasons. Correlations between temperature and relative elevation (Z*) are described by the slope coefficients, associated p values, and the multiple (Mult.) and adjusted (Adj.) r2, which reflect the variance explained by fixed effects alone or by fixed and random effects combined, respectively. Significant differences (< 0.05) between tidal stages within a depth and season are denoted by superscripts. Significant differences in slope coefficients (p<0.05) between tidal stages within a depth and season are denoted by superscripts (a, b, c).
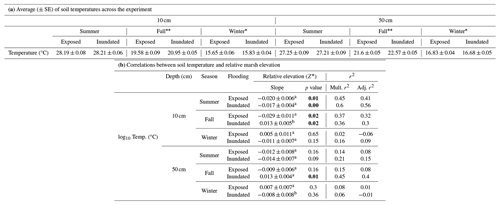
3.2 Decay rates and stabilization factors
TBI decay rates (k, d−1) decreased with depth and time (Fig. 4a, b; Table 3). Rates were 50 %, 32 %, and 118 % faster in the 10 cm horizon at the 3-, 6-, and 12-month time points, respectively, compared to 50 cm depth. Decay rates slowed to a similar degree between 3 and 6 months at 10 cm (60 %) and 50 cm (54 %), but there was less of a slowdown between 6 and 12 months at 10 cm (17 %) compared to 50 cm (50 %; Table 3a). This translated into a 3-fold slowing of turnover times from 140 to 416 d at 10 cm but a 4-fold slowing from 209 to 903 d at 50 cm over the year-long experiment (Table 3a).
Tea-specific empirical decay rates (kg, kr) bookended TBI k, differed between burial depths, and changed over time. Green tea rates were 67 %–162 % and 150 %–327 % higher, and rooibos tea rates were 48 %–64 % and 34 %–63 % lower at 10 and 50 cm, respectively, than TBI rates (Fig. 4c–f; Table 3). The percent difference between shallow and deeper rates was much greater for rooibos (29 %–36 %) than green tea (0 %–2.7 %), but both tea types slowed to similar extents at 10 and 50 cm between 3–6 months (37 %–38 % for green, 39 %–42 % for rooibos) and 6–12 months (37 %–38 % for green, 34 %–35 % for rooibos).
We next compared decomposable fractions (a) and stabilization factors (S) for green and rooibos teas. The TBI and empirical approaches produce the same S and a values for green tea (i.e., TBI S = empirical Sg, TBI ag= empirical ag) but not for rooibos (Eqs. 2–5). The TBI ar values, calculated from Hg and Sg (Eq. 4), were 71 %–200 % higher than empirical ar values, based on the mass fraction of rooibos tea lost at each time point and depth (Table 3). Stabilization factors for rooibos tea (empirical Sr) were 247 %–285 % and 279 %–423 % greater than for green tea (TBI S, empirical Sg) at 10 cm and 50 cm, respectively (Table 3). For both tea types S values were lowest at 12 months, but only empirical Sr had lower values at the deeper depth.
Root decay rates estimated from litterbags buried in a nearby S. alterniflora marsh ranged from 0.0015–0.0021 d−1 and were slightly faster in the interior marsh plain compared to the creek-bank levee (Table S2). These rates were slower than decay estimates calculated from the TBI approach and green tea (empirical kg) but comparable to rooibos tea (empirical kr) loss rates (Table 3).
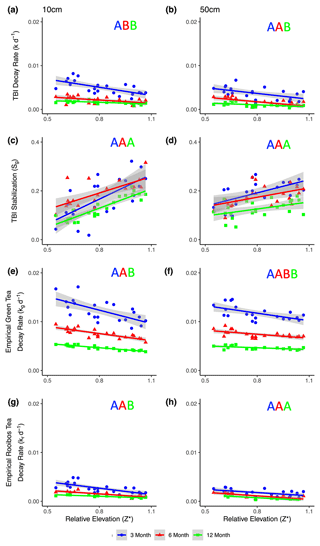
Figure 4TBI decay rates (a, b) and TBI stabilization factors (c, d) and empirical decay rates (e, f, g, h) at 10 cm (a, c, e, g) and 50 cm (b, d, f, h) and at 3, 6, or 12 months (blue, red, and green, respectively). Decay rates decreased, while TBI stabilization factors increased with relative marsh surface elevation within the tidal frame (Z*) at both 10 and 50 cm depths. Significant correlations (p < 0.05, as determined by linear regressions; see Sect. 2.8) are denoted by solid lines. Significant differences between linear regressions of TBI k, empirical kg and kr, and TBI S with Z* at the different time points are denoted by letters of the same color. See Table 5 for statistical results.
Table 2Tea Bag Index (TBI) and empirical decay rates across soil depths and deployment times. Linear mixed-effect models were used to test decay and stabilization responses to the depth (10 or 50 cm) and length (3, 6, or 12 months) of deployments. The marginal (Mar.) and conditional (Cond.) r2 reflect the variance explained by fixed effects alone or by fixed and random effects combined, respectively. Significant p values (<0.05) are in bold.

Table 3Average ± SE of decomposable fractions (a) and stabilization factors used to estimate decay rates (k) and turnover times (d) with the TBI (a) and empirical (b) approaches. Significant differences (p > 0.05; as determined by t tests; see Sect. 2.8) between deployment times within a depth horizon are denoted by superscripts. Significant differences in k values (p>0.05; as determined by t tests; see Sect. 2.8) between deployment times within a depth horizon are denoted by superscripts (a, b, c).
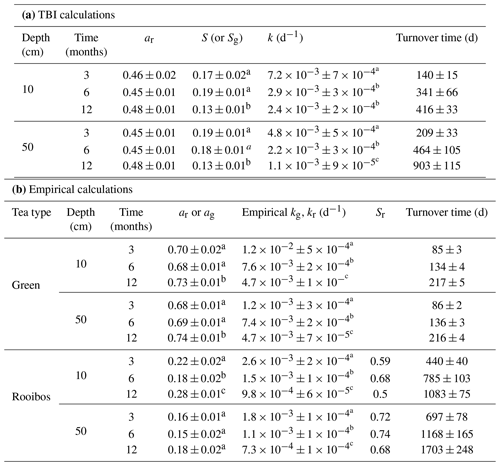
3.3 Plants, soil stiffness, and animal communities
Linear regression analyses demonstrated that soil shear strength decreased with increasing relative elevation (Z*; r2=0.21, p<0.05) (Wu et al., 2022). Plant density did not change across the relative elevation gradient (p> 0.05), while stem height (r2=0.36, p<0.05) and aboveground biomass (r2=0.60, p<0.05) both decreased with increasing Z* (Wu et al., 2022). Two major groups of invertebrates were present: crabs (Uca pugilator, Sesarma reticulatum, Panopeus) and snails (Littoraria irrorata) (Wu et al., 2022). Densities of crab burrows and snails were not correlated with relative elevation (Z*; p> 0.05).
3.4 Biotic and abiotic variables correlated with TBI decay rates
TBI decay rates (i.e., TBI k) at 10 and 50 cm depth negatively correlated with relative elevation (Z*) across all time points (Table 5). Rates also correlated with other abiotic and biotic factors, but, unlike Z*, none of these relationships were consistently significant at either depth or throughout the experiment (Table 4). For instance, porewater salinity correlated with TBI decay at 50 cm but not 10 cm. Variables reflecting certain plant (stem height, aboveground and belowground biomass) and soil (stiffness) characteristics consistently correlated with decay rates at 3 and 6 months but not at 12 months at either depth. Other variables representing bioturbation (crab burrows), grazing (snails), and porewater chemistry (pH, redox) correlated sporadically, if at all, with TBI decay (Table 4). The directionality of many of these correlations (but not statistical significance) remained unchanged over the 12-month period. To explore this further, we evaluated correlations between relative elevation (Z*) and aboveground plant biomass and soil stiffness. Negative relationships with aboveground biomass (r2=0.60, p<0.05) point to lower grass production at higher elevations. Positive correlations with soil stiffness (r2=0.21, p<0.05) are consistent with less consolidation (i.e., greater porewater flushing, more root production) or other gradients in soil properties (e.g., grain size, which affects burrowing) at lower elevations. Because plant and soil properties affect decay (Liu et al., 2008; Noyce et al., 2023) and we cannot separate those drivers from relative marsh surface elevation (Z*), we focus on how decay changes with position in the tidal frame from here forward.
3.5 Decay, stabilization, and relative marsh surface elevation (i.e., Z*)
TBI and empirical decay rates decreased, while TBI S increased at higher relative elevations (Z*; Table 5, Fig. 4). Changes in TBI and empirical green tea decay rates with relative elevation (Z*) at 10 and 50 cm were sharpest at 3 months and became less pronounced with time. Further, these gradients were generally steeper at 10 cm and more gradual at 50 cm. In contrast, correlations between relative elevation (Z*) and empirical rooibos decay rates were more similar between soil depths and stable over time. Correlations with relative elevation (Z*) generally accounted for greater fractions of the variability in empirical green (r2=0.44–0.86) and rooibos (r2=0.50–0.75) rates compared to TBI decay (r2=0.33–0.41) at 10 cm. The explanatory power of relative elevation (Z*) was lower at 50 cm for the empirical rates, but differences between depths were less clear for TBI rates.
The TBI S factors had the opposite relationship with relative elevation (Z*) and increased at higher elevations, but these correlations did not change over time at either 10 or 50 cm depth (Fig. 4c–d; Table 5). The change in TBI S with relative elevation (Z*) was greater at 10 cm compared to 50 cm, and these correlations were largely constant throughout the experiment (Table 5).
Table 5Decay rates (k, d−1) decreased, and TBI stabilization factors (Sg) increased relative to marsh surface position in the tidal frame (Z*) at both depths and throughout the experiment. Differences between slopes across the three time points within a depth horizon are denoted by superscripts (a, b, c) as determined by the methods of Clogg et al. (1995); see Sect. 2.8.
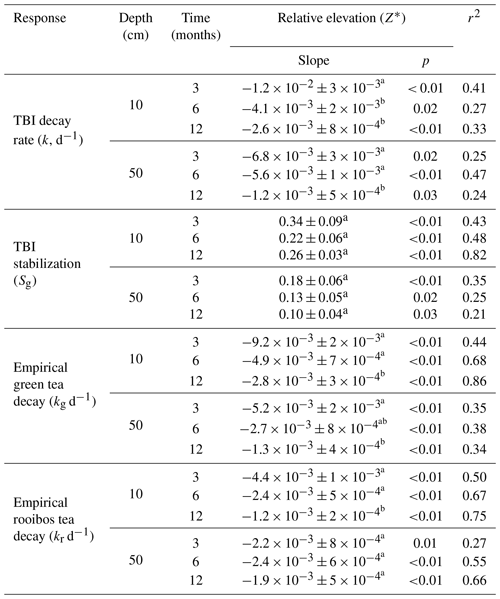
4.1 Methodological considerations
Decay rates based on the TBI and empirical green and rooibos teas (i.e., kg, kr) slowed over time and with depth and were fastest in plots sitting lower in the tidal frame (Fig. 4, Tables 3, 5). Average green tea decay rates were 67 %–327 % faster than TBI k, which was 34 %–64 % faster than rooibos rates (Table 3). Between soil depths, TBI and rooibos decay rates were 30 %–118 % and 34 %–44 % faster, respectively, at 10 cm compared to 50 cm, whereas empirical green tea rates were nearly equivalent (0 %–3 % change; Table 3). Differences between TBI and empirical decay rates at both depths and, for rooibos tea, with time were relatively constant, while the gap between TBI and green tea widened over the year. These patterns suggest that, at the plot scale and over 1 year, TBI rates reflect rooibos tea dynamics (oxidized needles and branches from the rooibos bush) slightly more than green tea (Camellia sinensis leaves and buds).
At a larger scale across marsh surface elevations, changes in TBI k with relative elevation (Z*) closely mirrored empirical green tea dynamics, especially in the shallower soil horizon (Table 5; Fig. 4). Steeper drops in TBI decay with relative elevation (Z*) during the first 3 months were driven by changes in green tea mass loss and likely influenced by leaching due to greater tidal flushing of porewater at lower elevations (Fig. 4, Table 5). In contrast, mass loss rates of rooibos tea changed less across relative marsh surface elevations (Z*) and were more constant over time. These patterns are consistent with a short-term leaching experiment demonstrating faster losses of green tea (10 %–50 %) than rooibos (< 5 %–20 %) and greater sensitivity to temperature, water turnover, and soil moisture content (Lind et al., 2022). We cannot isolate the magnitude of leaching effects from microbial decomposition since both would have occurred during the first several months. That leaching accelerates decay is not a problem exclusive to the TBI – it also affects interpretation of mass loss rates from litterbags with local detritus (Cotrufo et al., 2010; Gessner et al., 1999; MacDonald et al., 2018; Seelen et al., 2019) – but the potential magnitude of abiotic loss highlights that decay coefficients from 3-month deployments, as prescribed by Keuskamp et al. (2013), should not be interpreted solely as a function of the microbial community. Instead, extending the duration and increasing the number of sampling points of the TBI could result in decay rates that are more representative of microbial processing (Lind et al., 2022; Marley et al., 2019).
Another argument for extending the duration of TBI studies in marshes is that mass loss rates of green tea did not plateau after 3 months. Green tea mass loss increased by 4.3 % at 10 cm and 8.8 % at 50 cm over the final 9 months of the experiment (Table 3b, ag values). The fraction of green tea mass loss was never greater than Hg (hydrolysable fraction of green tea) reported by Keuskamp et al. (2013), as has happened in short-term leaching studies (Lind et al., 2022) and forest soils (Mori et al., 2022). As a result, TBI S did not fall below zero, which would have skewed the TBI ar. Choice of Hr and Hg values is important because the hydrolysable fraction, operationally defined as the sum of nonpolar, water soluble, and acid soluble compounds, is sensitive to methodology (e.g., Mueller et al., 2018) and affects S.
A central tenant of the TBI is that S asymptotes at 3 months and values are the same for green and rooibos teas (Keuskamp et al., 2013). However, we found that TBI S (equivalent to Sg) and Sr decreased from 3–12 months, and values were 247 %–423 % higher for rooibos than green tea (Table 3). Moreover, differences between Sg and Sr increased over time. The caveat that Sg and Sr were not equal is not a function of the marsh environment as Mori et al. (2022) also report differences across four temperate forest stands. The assertion that S is the same for green and rooibos teas rests on the assumption that stabilization is controlled by environmental factors (Keuskamp et al., 2013) and independent of compositional differences that affect organic matter–soil interactions (e.g., mineral association, incorporation into aggregates) and, as a result, decomposition rates (Marschner et al., 2008; Mikutta et al., 2006). Yet easily degradable nonstructural compounds can be preserved over long timescales due to physio-chemical interactions, while complex macromolecules are not intrinsically recalcitrant (Dungait et al., 2012; Kallenbach et al., 2016; Mikutta et al., 2006). As such, there appears to be limited theoretical support for S as formulated by the TBI.
Comparisons between TBI and empirical decay rates averaged across plots demonstrate that this index is weighted slightly more by rooibos mass loss rather than being an equal blend of both teas. However, when distributed across the environmental gradient of tidal flooding (i.e., Z*), the different sensitivities of each tea type became more apparent. Faster mass loss rates of green and rooibos teas, which are rich in soluble tannins and aromatic compounds associated with lignin monomers, respectively (Duddigan et al., 2020), in lower relative elevation (Z*) plots are consistent with leaching in the short term (3 months) and the effects of porewater turnover on decomposition in the longer term (Fig. 4). Running experiments beyond 3 months and increasing sampling frequencies will likely allow for better distinctions between leaching and decomposition effects (Duddigan et al., 2020; Lind et al., 2022; Marley et al., 2019). The TBI was developed for terrestrial soils, and our results demonstrate that some assumptions need to be carefully assessed when applying this method to saturated, wetland soils. Knowing the different sensitivities of green and rooibos teas to physical, chemical, and biological processes is valuable for interpreting controls on organic matter mass loss rates across environmental gradients and different ecosystems.
4.2 Decay rate context
Organic matter decay rates estimated by the TBI were higher than previous measurements of 0.0010–0.0026 d−1 from Georgia's minerogenic salt marshes based on field litterbags and laboratory leaching and incubation experiments conducted over 150–540 d (Benner et al., 1984b, 1987, 1991; Rice and Tenore, 1981) (Table S2). The slowest rates were based on lignin, while faster rates were estimated from losses of structural polysaccharides (cellulose, hemicellulose) or plant tissue mass. The highest decay rate was calculated from polysaccharides in root and rhizome litter (Benner et al., 1991) and was 30 %–73 % faster than root mass loss along creek-bank levees (0.0015 d−1) and marsh interiors (0.0020 d−1; Table S2). The TBI rates at 3 months were 2.8–7.2 times faster than prior studies, but they drop to roughly double over the longer 6–12-month periods (10 cm horizon only; Table 3; Fig. 4), with the exception of the rapid polysaccharide-specific rate (Benner et al., 1991). This is perhaps not surprising since nuclear magnetic resonance (NMR) spectroscopy demonstrates sharp reductions in O-alkyl compounds consistent with carbohydrates and polysaccharides and aromatic compounds consistent with tannins during green tea incubations (Duddigan et al., 2020). Higher TBI rates could also reflect differences in the preparation and processing (e.g., milling, oxidation) of the organic matter filling tea bags and litter bags. While the TBI greatly overestimates decay of natural litter (120 %), empirical rooibos mass loss rates are only slightly faster (36 %; Tables 3, S2). This suggests that rooibos tea may adequately mimic decay dynamics of local litter, depending on study goals, but combining it with green tea in the TBI results in accelerated rate estimates.
We expected TBI rates to be faster in our study in subtropical Georgia, USA, compared to higher latitudes based on the metabolic theory of ecology, which predicts that decay rates increase with rising temperatures (Yvon-Durocher et al., 2010), and based on observations that warming accelerates loss of labile compounds in soils (Conant et al., 2011; Melillo et al., 2002). To test this, we compared our rates with those from 7 other salt marsh TBI studies that encompass 11 countries and span a latitudinal gradient of 93.7° (−37.7 to 56°; Fig. 5, Table S3) (Mueller et al., 2018; Puppin et al., 2023; Marley et al., 2019; Alsafran et al., 2017; Yousefi Lalimi et al., 2018; Sanderman and Eagle, unpublished; Tang et al., 2023). North America accounted for 50 % of the observations, and only one observation came from the Southern Hemisphere. Tea bags were buried at 8 cm in most of those studies, per Keuskamp et al. (2013), whereas we used 10 cm depth. It is unlikely that this slight difference in burial depth skews comparisons since both are within the rooting zone. Marley et al. (2019) used locally sourced tea, rather than the prescribed Lipton brand, but reported that the two were compositionally similar. Potentially more important is that most studies used TBI Hg and TBI Hr values reported by Keuskamp et al. (2013), while Tang et al. (2023) performed different extractions to derive their own estimates of hydrolysable fractions. Differences in H values across studies are relatively minor and would more strongly affect TBI S (stabilization) than TBI k (decay). Our Sapelo Island, Georgia, 3-month rates were similar to salt marshes in North Carolina, Virginia, Maryland, California, and Massachusetts in the United States and Zhejiang Province (ZJ) in China (Fig. 5). The lack of a directional trend within these latitudes contrasts with small-to-moderate warming effects on marsh litter decomposition in field experiments (Charles and Dukes, 2009; Tang et al., 2023) and across spatial gradients (Kirwan et al., 2014). The absence of a general latitudinal trend (p> 0.05, r2=0.01) for 3-month decay rates could reflect interactions within the soil environment that affect decomposition, such as bioturbation, leaching, tidal flushing, redox conditions, salinity, mineral associations (Conant et al., 2011; Craine et al., 2010), and plant species effects such as rooting depth, growth rate, and exudate composition, among other variables (Fettrow et al., 2023; Keiluweit et al., 2015; Seyfferth et al., 2020; Spivak et al., 2023). It is also possible that 3-month rates are more sensitive to leaching than temperature in saturated marsh soils as differences between sampling time points (3, 6, or 12 months) are often as great as between latitudes (e.g., Sapelo Island, GA, USA; Schleswig-Holstein (SH), Germany; East Lothian (ELN), Scotland). It is possible that latitudinal trends may become more apparent following longer tea bag deployments when microbial processing would be the dominant control on organic matter loss.
The TBI's stabilization factor (S) is meant to represent the process by which labile compounds in green tea become refractory under certain environmental conditions (Keuskamp et al., 2013) and should increase over time as decay progresses (Marschner et al., 2008; Mikutta et al., 2006). A slight negative correlation between 3-month TBI S and k values (r2=0.11, p>0.05) across the compiled data from all seven studies supports this prediction (Fig. 5). However, the negative relationship between TBI S and k is not universal across individual studies (Keuskamp et al., 2013; Seelen et al., 2019). In our experiment on Sapelo Island (GA, USA), the highest S values coincided with the fastest decay rates in the first 3 months (Fig. 5). Stabilization values then decreased between 3 and 6 months, but there was no overall temporal trend because values increased at 12 months. This variability is not unique to our site; TBI S values decreased and increased at East Lothian (ELN) in Scotland and Schleswig-Holstein (SH) in Germany, respectively, between 3 and 12 months (Fig. 5). The absence of a clear latitudinal gradient in TBI S values suggests that this proxy is largely insensitive to global-scale temperature gradients (Fig. 5). Yet this contrasts with Mueller et al. (2018), who reported higher TBI S values at higher latitudes along the North American Atlantic coast. The mixed relationships between TBI S and k and across latitudes could reflect variability in the many physicochemical processes that affect microbial access to and efficient use of organic matter (e.g., mineral association; Georgiou et al., 2024; Tao et al., 2023). This interpretation adds a caveat to the finding that green and rooibos teas do not share S values, which violates assumptions of the TBI method (Table 3; Mori et al. 2002).
Faster decay rates estimated in this study using the TBI method relative to more conventional litterbag and laboratory experiments suggest that these approaches are not interchangeable. It is possible that tea processing, including oxidation and milling into small pieces, increases vulnerability to decomposition and that microbes respond strongly to compositional differences between allochthonous organic matter (i.e., tea) and local marsh detritus. Similar decay rates between rooibos tea and more conventional approaches suggest that this aspect of the TBI could be a reasonable proxy when the experimental goal is to assess drivers independently of site-specific differences in organic matter composition and material preparation. Few studies like ours have directly compared decay rates from the TBI, its components, and more conventional approaches, but this would be useful in assessing whether Keuskamp et al.'s (2013) method can be applied broadly, in dry and saturated soils. If rooibos tea decay rates from 6-month and longer deployments are comparable to more conventional approaches, then clearer patterns between marshes and across latitudes may become more apparent.
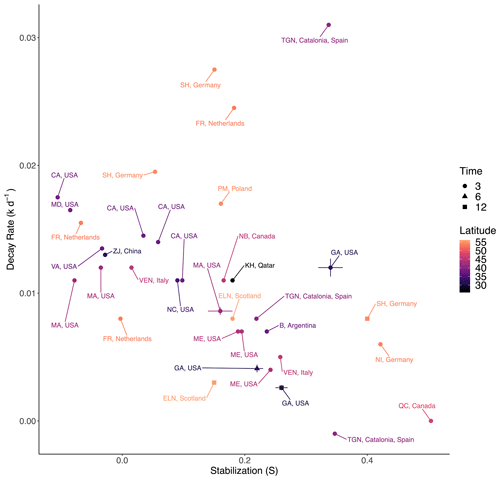
Figure 5Average TBI decay rates and stabilization factors in salt marshes as reported by seven datasets spanning a latitudinal gradient of 56.0° N to 37.7° S (see Table S3). To facilitate comparison across northern and southern latitudes, all coordinates are represented as positive values. Data are from 3, 6, or 12 months of burial at 8–10 cm depth, and error bars represent standard error. The Georgia, USA, data are from this study.
4.3 Decay within and below the rhizosphere
The trajectory of rapid initial TBI decay rates followed by progressive slowing is consistent with decomposition models (Morris and Bowden, 1986; Valiela et al., 1985), litterbags (Benner et al., 1991), and depth profiles of marsh soil organic matter (Luk et al., 2021) and likely reflects initial losses of soluble and bioavailable compounds and relative accumulation of larger macromolecules (Benner et al., 1991; Marley et al., 2019; Moran et al., 1989; Wilson et al., 1986). Slower rates at deeper depths are consistent with a more stable environment relative to the rhizosphere where root oxygen loss and exudates, bioturbation, and porewater flushing are associated with faster decay (Furukawa et al., 2004; Li et al., 2021; Wilson et al., 2012). However, negative correlations between decay at 50 cm and relative elevation (Z*) demonstrate that deeper soil horizons are not isolated from surface processes.
Faster decay rates during the first 3 months at 10 and 50 cm were likely driven by leaching from green and rooibos teas, while slower rates in the following 9 months may be more representative of microbial decomposition (Duddigan et al., 2020; Lind et al., 2022) (Table 3). The 3-month rates may also reflect the summertime deployment since warmer temperatures can accelerate leaching and decay (Kirwan et al., 2014; Lind et al., 2022; Tang et al., 2023). However, seasonality effects are likely small in Georgia, where average daily soil temperatures ranged from ∼ 15 to ∼ 28 °C between winter and summer (Table 1a). This is narrower than the temperature gradient in an hours-long leaching study that found ∼ 5 % and ∼ 10 % increases in green tea mass loss between 8 and 19 °C and between 19 and 60 °C, respectively (Lind et al., 2022). Microbial decomposition is temperature-sensitive (Yvon-Durocher et al., 2010), but responsiveness in wetlands across latitudes and experiments is mixed (Kirwan et al., 2014; Tang et al., 2023). Seasonal changes in plant production and root–microbe interactions also affect decomposition by altering the belowground chemical and physical environment (Van Der Nat et al., 1998; Pett-Ridge et al., 2021). Summertime aboveground and rhizome biomasses and stem height correlated positively with decay, particularly in the first 6 months, indicating that higher plant abundances correspond to increased TBI mass loss (Table 4). It is unclear whether decay responded to plant–microbe interactions or plant effects on soil structure since correlations were inconsistent at 12 months (i.e., the following summer). We cannot tease apart temperature effects on leaching and decay further because tea bags were deployed, and plants were surveyed only once, during summer. Better assessment of temperature effects on the TBI requires multiple deployments and collections and repeated characterizations of aboveground and belowground plant processes across different seasons.
Decay was faster in the top 10 cm, as predicted, but not for the expected reasons (Table 3). We hypothesized that TBI decay and that of green and rooibos teas would be faster in the surface horizon due to greater rhizodeposition, bioturbation, and more oxidizing conditions. Instead, green tea loss rates were similar at both depths, and slower TBI rates at 50 cm were driven by the rooibos tea (Table 3). TBI decay correlated positively with plant characteristics at both 10 and 50 cm (Table 4). The rooting zone of S. alterniflora extends 20–30 cm and is generally above the 50 cm deployment horizon. Plant effects on soil structure and porewater movement are strongest in the rhizosphere but may extend to deeper depths more weakly, reflecting the year-over-year soil-building process and preservation of dead roots and rhizomes. Burrow density did not consistently correlate with TBI decay, but when it did, relationships were negative, meaning that rates slowed with more burrowing, which is contrary to most observations (Table 4) (Kostka et al., 2002a, b; Xiao et al., 2021). Redox conditions were less negative at 10 cm but were not correlated with TBI decay (Fig. 2; Table 4). This was unexpected given thermodynamic constraints of anoxia on decomposition but may be due to loss of compounds that are less redox-dependent during the short 1-year incubation, whereas decay over longer deployments would become more dependent on processes that are oxygen-sensitive, such as depolymerization (Huang et al., 2020; LaCroix et al., 2019). Plant production, bioturbation, redox conditions, and porewater exchange change on timescales of hours to seasons and our summertime ecological observations and periodic porewater collections may have been at too coarse a resolution to adequately capture belowground environmental conditions at 10 and 50 cm. Alternatively, the unexpected correlations (or lack thereof) may highlight that many factors influence decay and that short-term rates are more sensitive to other drivers. Regardless, slower rates at 50 cm demonstrate that decay is more constrained by environmental conditions than litter composition, which is consistent with emerging frameworks of organic matter decomposition (Lehmann and Kleber, 2015; Marín-Spiotta et al., 2014; Spivak et al., 2019).
Differences in TBI decay between 10 and 50 cm persisted across the relative elevation (Z*) gradient, with faster rates in plots that were lower in the tidal frame (Fig. 4; Table 5). Marsh surface elevation gradients and tidal flooding dictate many aspects of marsh functioning, including plant production and surface soil stiffness, which increased and decreased, respectively, in plots at lower relative elevation (Z*) levels. Soil temperatures at 10 cm were warmer at lower marsh surface elevations and differed between inundated and flooded tidal stages, but patterns were less clear and seasonally consistent than in a nearby marsh (Fig. 3; Table 1) (Alber and O'Connell, 2019). Porewater exchange is greater at lower elevations and closer to tidal creeks (Guimond and Tamborski, 2021), which can facilitate decomposition by increasing oxygen delivery to the subsurface, removing toxic metabolites, increasing pore space connectivity, and altering organic matter–mineral associations (Canfield, 1989; Liu et al., 2008; Xiao et al., 2021). Sharp changes in TBI rates across the relative elevation (Z*) gradient at 3 months likely reflect more extensive leaching at lower elevations where soil stiffness is also lower and porewater exchange would be greater (Fig. 4; Tables 4–5) (Guimond and Tamborski, 2021; Lind et al., 2022). This is also consistent with a sharper drop in TBI rates between 3 and 6 months in plots with the lowest relative elevation (Z*) values (Fig. 4a, Table 5). More moderate changes in TBI rates with relative elevation (Z*) at 6 and 12 months indicate that inundation effects on decay extend beyond leaching, which plateaus between 20 d (green) and 80 d (rooibos) (Duddigan et al., 2020). Our results contrast with a recent study in the Venetian Lagoon (Italy), but comparisons are tricky because Puppin et al.'s (2023) analysis combines tea bag burial depth (0–24 cm) with marsh surface elevation into the variable zβ, making it difficult to assess those factors independently. Further analyses focused on the shallowest horizon (8 cm) in Puppin et al. (2023) where TBI k slowed with increasing distance from creek-bank edges but showed no correlation with estimated time flooded over the 3-month deployment. In our study, it is not possible to differentiate effects of creek-bank distance from flooding duration because they were confounded, but testing this could provide insight into the sensitivity of decay to soil structure and hydrology. Because inundation influences many ecological, physical, and biogeochemical factors, we cannot definitively attribute correlations between relative elevation (Z*) and TBI decay to any single one or a combination. However, because relative elevation (Z*) was the only variable that consistently correlated with decay at both depths and all three time points, we suspect that gradients in porewater hydrology are particularly important (Table 4). By 12 months the regression slope between TBI decay and relative elevation (Z*) was ∼ 2 times greater at 10 cm compared to 50 cm, demonstrating that rates become less sensitive to inundation at deeper depths and over time. Moreover, the persistence of correlations between TBI decay and relative elevation (Z*) at 50 cm shows connectivity between surface and deeper horizons and that environmental conditions below the rhizosphere that affect organic matter loss are not constant or uniform.
Our results suggest that short-term organic matter decay is less sensitive to its composition than the soil environment and that porewater hydrology may be a particularly important driver. Tea composition is highly standardized, but decay rates were faster at lower relative marsh elevations (Z*) and shallower soil depths (10 cm), which points to the importance of environmental controls such as tidal flushing on organic matter loss. Rooibos tea decay rates were slightly higher, but comparable, to natural marsh litter and less affected by leaching than green tea and may therefore be a reasonable proxy for organic matter breakdown over certain timescales (Tables 2–5, S2; Fig. 4). It is possible that kinetic (e.g., temperature) and thermodynamic (e.g., redox) controls become more important over longer timescales, after low-molecular-weight, soluble compounds are lost and decay is more dependent on depolymerization of larger molecules (Conant et al., 2011; Hu et al., 2020). Deployments beginning in different seasons and lasting longer than 1 year, perhaps without green tea, could be useful in assessing within-site sensitivity of decay to temperature and how controls on organic matter loss change over time. Similarly, parallel deployments in mineral and organic marshes with similar flooding regimes but different soil properties (e.g., bulk density, porosity, permeabilities, carbon content) could provide further insight into how the belowground environment affects decay. Pairing organic matter loss rates with geochemical analyses and rates of porewater exchange would be valuable to understand molecular-level changes and explore the roles of physicochemical protection and hydrology.
In this minerogenic, subtropical salt marsh, the TBI produced faster organic matter decay rates relative to studies using local plant litter. The faster rates were largely due to initial rapid green tea loss and were greatest in the first 3 months. Placing TBI rates within the context of more traditional approaches is important for assessing the broad applicability of this method and whether changes, such as extending deployment durations and dropping green tea, are warranted. Publishing decay rates of green and rooibos teas alongside the TBI and site-specific literature values is key to evaluating potential method improvements and better identifying generalizable patterns across environmental gradients, such as elevation, flooding, and latitude. We found that rooibos tea produces decay rates comparable to local litter and that rates slow with depth, time, and increasing marsh surface elevation (Tables 2–5, S2; Fig. 4). Because the composition of rooibos tea is similar to natural litter (Duddigan et al., 2020) and preparation is highly standardized, our findings demonstrate that environmental conditions exert stronger controls on short-term decay than molecular recalcitrance, which is in line with the current theory (Tables 2–4; Fig. 4) (Lehmann and Kleber, 2015; Marín-Spiotta et al., 2014). Slower, steadier rooibos rates at 50 cm suggest that organic matter surviving transit through the rhizosphere may still be vulnerable to decomposition in deeper, more stable soil horizons. Consistent differences in rooibos decay rates across marsh surface elevation gradients (i.e., Z*), as well as over time and with depth, indicate that local hydrology strongly affects organic matter loss. This variable is often overlooked in marsh decomposition studies but may be more important than kinetic (e.g., temperature) and thermodynamic (e.g., redox) constraints in the short term.
All raw data are in the GCE-LTER and electronic data interchange (EDI) archives (Spivak, 2024) and have been assigned a publicly accessible digital object identifier.
The supplement related to this article is available online at: https://doi.org/10.5194/bg-22-435-2025-supplement.
The study was designed by SGR, FW, SCP, and ACS. Data collection and sample analyses were performed by SGR, FW, and WRF. SGR and ACS wrote the initial manuscript draft. SGR, FW, WRF, SCP, ME, JS, CC, and ACS contributed to manuscript editing and review.
The contact author has declared that none of the authors has any competing interests.
Publisher’s note: Copernicus Publications remains neutral with regard to jurisdictional claims made in the text, published maps, institutional affiliations, or any other geographical representation in this paper. While Copernicus Publications makes every effort to include appropriate place names, the final responsibility lies with the authors.
We thank Dontrece Smith, Gabriella Giordano, Sam Dong, Reese Lofgren, and Andrew Pinsonneault for assistance with field deployments and lab analyses and the Georgia Coastal Ecosystem LTER for project support (OCE-9982133). Satyatejas G. Reddy was supported by the University of Georgia's Center for Undergraduate Research. Amanda C. Spivak was supported by NSF DEB-2121019, an institutional grant (NA18OAR4170084) to the Georgia Sea Grant College Program from the National Oceanic and Atmospheric Administration, and by the US Coastal Research Program (USCRP) as administered by the US Army Corps of Engineers® (USACE), Department of Defense. The content of the information provided in this publication does not necessarily reflect the position or the policy of the government, and no official endorsement should be inferred. The authors acknowledge the USACE and USCRP's support of their effort to strengthen coastal academic programs and address coastal community needs in the United States. Fengrun Wu was supported by the Natural Science Foundation of Fujian Province (grant no. 2022J05278) and the Marine and Fishery Development Special Fund of Xiamen (grant no. 23YYST064QCB36). Any use of trade, company, or product names is for descriptive purposes only and does not imply endorsement by the US Government. This article is contribution number 1124 from the University of Georgia Marine Institute.
This research has been supported by the Directorate for Biological Sciences (grant no. NSF DEB-2121019), an institutional grant (NA18OAR4170084) to the Georgia Sea Grant College Program from the National Oceanic and Atmospheric Administration, the US Coastal Research Program (USCRP) as administered by the US Army Corps of Engineers® (USACE), Department of Defense, the Natural Science Foundation of Xiamen Municipality (grant no. 2022J05278), and the Xiamen Ocean and Fishery Bureau (grant no. 23YYST064QCB36).
This paper was edited by Nicolas Brüggemann and reviewed by two anonymous referees.
Alber, M. and O'Connell, J.: Elevation Drives Gradients in Surface Soil Temperature Within Salt Marshes, Geophys. Res. Lett., 46, 5313–5322, https://doi.org/10.1029/2019GL082374, 2019.
Alsafran, M. H. S. A., Sarneel, J., and Alatalo, J. M.: Variation in plant litter decomposition rates across extreme dry environments in Qatar, Arab. World Geographer, 20, 252–261, 2017.
R Core Team: R: A language and environment for statistical computing. R Foundation for Statistical Computing, Vienna, Austria, https://www.R-project.org/ (last access: 21 January 2025), 2023.
Arriola, J. M. and Cable, J. E.: Variations in carbon burial and sediment accretion along a tidal creek in a Florida salt marsh, Limnol. Oceanogr., 62, S15–S28, https://doi.org/10.1002/lno.10652, 2017.
Benner, R., Maccubbin, A. E., and Hodson, R. E.: Anaerobic biodegradation of the lignin and polysaccharide components of lignocellulose and synthetic lignin by sediment microflora, Appl. Environ. Microbiol., 47, 998–1004, 1984a.
Benner, R., Newell, S. Y., Maccubbin, A. E., and Hodson, R. E.: Relative contributions of bacteria and fungi to rates of degradation of lignocellulosic detritus in salt-marsh sediments, Appl. Environ. Microbiol., 48, 36–40, 1984b.
Benner, R., Fogel, M. L., Sprague, E. K., and Hodson, R. E.: Depletion of 13C in lignin and its implications for stable carbon isotope studies, Nature, 329, 708–710, 1987.
Benner, R., Fogel, M. L., and Sprague, E. K.: Diagenesis of belowground biomass of Spartina alterniflora in salt-marsh sediments, Limnol. Oceanogr., 36, 1358–1374, 1991.
Blum, L. K.: Spartina alterniflora root dynamics in a Virginia marsh, Mar. Ecol.-Prog. Ser., 102, 169–178, 1993.
Blum, L. K. and Christian, R. R.: Belowground production and decomposition along a tidal gradient in a Virginia salt marsh, Ecogeomorphol. Tidal Marsh., 59, 47–73, 2004.
Bradley, P. M. and Morris, J. T.: Influence of oxygen and sulfide concentration on nitrogen uptake kinetics in Spartina alterniflora, Ecology, 71, 282–287, 1990.
Bulseco, A. N., Vineis, J. H., Murphy, A. E., Spivak, A. C., Giblin, A. E., Tucker, J., and Bowen, J. L.: Metagenomics coupled with biogeochemical rates measurements provide evidence that nitrate addition stimulates respiration in salt marsh sediments, Limnol. Oceanogr., 65, S321–S339, https://doi.org/10.1002/lno.11326, 2020.
Canfield, D. E.: Sulfate reduction and oxic respiration in marine sediments: implications for organic carbon preservation in euxinic environments, Deep-Sea Res. Pt. A, 36, 121–138, 1989.
Charles, H. and Dukes, J. S.: Effects of warming and altered precipitation on plant and nutrient dynamics of a New England salt marsh, Ecol. Appl., 19, 1758–1773, 2009.
Christian, R. R.: A life-table approach to decomposition studies, Ecology, 65, 1693–1697, 1984.
Clogg, C. C., Petkova, E., and Haritou, A.: Statistical Methods for Comparing Regression Coefficients Between Models, Am. J. Sociol., 100, 1261–1293, 1995.
Conant, R. T., Ryan, M. G., Ågren, G. I., Birge, H. E., Davidson, E. A., Eliasson, P. E., Evans, S. E., Frey, S. D., Giardina, C. P., and Hopkins, F. M.: Temperature and soil organic matter decomposition rates–synthesis of current knowledge and a way forward, Glob. Chang Biol., 17, 3392–3404, 2011.
Cotrufo, M. F., Ngao, J., Marzaioli, F., and Piermatteo, D.: Inter-comparison of methods for quantifying above-ground leaf litter decomposition rates, Plant Soil, 334, 365–376, 2010.
Craine, J. M., Fierer, N., and McLauchlan, K. K.: Widespread coupling between the rate and temperature sensitivity of organic matter decay, Nat. Geosci., 3, 854–857, 2010.
Duddigan, S., Shaw, L. J., Alexander, P. D., and Collins, C. D.: Chemical underpinning of the tea bag index: an examination of the decomposition of tea leaves, Appl. Environ. Soil Sci., 2020, 085180, https://doi.org/10.1155/2020/6085180, 2020.
Dungait, J. A. J., Hopkins, D. W., Gregory, A. S., and Whitmore, A. P.: Soil organic matter turnover is governed by accessibility not recalcitrance, Glob. Change Biol., 18, 1781–1796, 2012.
Fettrow, S., Vargas, R., and Seyfferth, A. L.: Experimentally simulated sea level rise destabilizes carbon-mineral associations in temperate tidal marsh soil, Biogeochemistry, 163, 103–120, 2023.
Furukawa, Y., Smith, A. C., Kostka, J. E., Watkins, J., and Alexander, C. R.: Quantification of macrobenthic effects on diagenesis using a multicomponent inverse model in salt marsh sediments, Limnol. Oceanogr., 49, 2058–2072, 2004.
Georgiou, K., Koven, C. D., Wieder, W. R., Hartman, M. D., Riley, W. J., Pett-Ridge, J., Bouskill, N. J., Abramoff, R. Z., Slessarev, E. W., and Ahlström, A.: Emergent temperature sensitivity of soil organic carbon driven by mineral associations, Nat. Geosci., 17, 205–212, 2024.
Gessner, M. O., Chauvet, E., and Dobson, M.: A perspective on leaf litter breakdown in streams, Oikos, 85, 377–384, https://doi.org/10.2307/3546505, 1999.
Giblin, A. E. and Howarth, R. W.: Porewater evidence for a dynamic sedimentary iron cycle in salt marshes 1, Limnol. Oceanogr., 29, 47–63, 1984.
Gribsholt, B. and Kristensen, E.: Effects of bioturbation and plant roots on salt marsh biogeochemistry: a mesocosm study, Mar. Ecol. Prog. Ser., 241, 71–87, 2002.
Guimond, J. and Tamborski, J.: Salt marsh hydrogeology: A review, Water (Basel), 13, 1–23, 2021.
Guimond, J. A., Seyfferth, A. L., Moffett, K. B., and Michael, H. A.: A physical-biogeochemical mechanism for negative feedback between marsh crabs and carbon storage, Environ. Res. Lett., 15, 1–25, 2020.
Holmquist, J. R., Windham-Myers, L., Bliss, N., Crooks, S., Morris, J. T., Megonigal, J. P., Troxler, T., Weller, D., Callaway, J., and Drexler, J.: Accuracy and precision of tidal wetland soil carbon mapping in the conterminous United States, Sci. Rep., 8, 9478, https://doi.org/10.1038/s41598-018-26948-7, 2018.
Howes, B. L. and Goehringer, D. D.: Porewater drainage and dissolved organic carbon and nutrient losses through the intertidal creekbanks of a New England salt marsh, Marine ecology progress series, Oldendorf, 114, 289–301, 1994.
Hu, Y., Zheng, Q., Noll, L., Zhang, S., and Wanek, W.: Direct measurement of the in situ decomposition of microbial-derived soil organic matter, Soil Biol. Biochem., 141, 107660, https://doi.org/10.1016/j.soilbio.2019.107660, 2020.
Huang, W., Ye, C., Hockaday, W. C., and Hall, S. J.: Trade-offs in soil carbon protection mechanisms under aerobic and anaerobic conditions, Glob. Change Biol., 26, 3726–3737, 2020.
Hughes, A. L. H., Wilson, A. M., and Morris, J. T.: Hydrologic variability in a salt marsh: Assessing the links between drought and acute marsh dieback, Estuar. Coast Shelf S., 111, 95–106, 2012.
Kallenbach, C. M., Frey, S. D., and Grandy, A. S.: Direct evidence for microbial-derived soil organic matter formation and its ecophysiological controls, Nat. Commun., 7, 13630, https://doi.org/10.1038/ncomms13630, 2016.
Keiluweit, M., Bougoure, J. J., Nico, P. S., Pett-Ridge, J., Weber, P. K., and Kleber, M.: Mineral protection of soil carbon counteracted by root exudates, Nat. Clim. Change, 5, 588–595, 2015.
Keuskamp, J. A., Dingemans, B. J. J., Lehtinen, T., Sarneel, J. M., and Hefting, M. M.: Tea Bag Index: a novel approach to collect uniform decomposition data across ecosystems, Method. Ecol. Evol., 4, 1070–1075, 2013.
Kirby, C. J. and Gosselink, J. G.: Primary production in a Louisiana Gulf Coast Spartina alterniflora marsh, Ecology, 57, 1052–1059, 1976.
Kirwan, M. L. and Blum, L. K.: Enhanced decomposition offsets enhanced productivity and soil carbon accumulation in coastal wetlands responding to climate change, Biogeosciences, 8, 987–993, https://doi.org/10.5194/bg-8-987-2011, 2011.
Kirwan, M. L., Langley, J. A., Guntenspergen, G. R., and Megonigal, J. P.: The impact of sea-level rise on organic matter decay rates in Chesapeake Bay brackish tidal marshes, Biogeosciences, 10, 1869–1876, https://doi.org/10.5194/bg-10-1869-2013, 2013.
Kirwan, M. L., Guntenspergen, G. R., and Langley, J. A.: Temperature sensitivity of organic-matter decay in tidal marshes, Biogeosciences, 11, 4801–4808, https://doi.org/10.5194/bg-11-4801-2014, 2014.
Kostka, J. E., Roychoudhury, A., and Van Cappellen, P.: Rates and controls of anaerobic microbial respiration across spatial and temporal gradients in saltmarsh sediments, Biogeochemistry, 60, 49–76, 2002a.
Kostka, J. E., Gribsholt, B., Petrie, E., Dalton, D., Skelton, H., and Kristensen, E.: The rates and pathways of carbon oxidation in bioturbated saltmarsh sediments, Limnol. Oceanogr., 47, 230–240, 2002b.
Kristensen, E., Penha-Lopes, G., Delefosse, M., Valdemarsen, T., Quintana, C. O., and Banta, G. T.: What is bioturbation? The need for a precise definition for fauna in aquatic sciences, Mar. Ecol. Prog. Ser., 446, 285–302, 2012.
LaCroix, R. E., Tfaily, M. M., McCreight, M., Jones, M. E., Spokas, L., and Keiluweit, M.: Shifting mineral and redox controls on carbon cycling in seasonally flooded mineral soils, Biogeosciences, 16, 2573–2589, https://doi.org/10.5194/bg-16-2573-2019, 2019.
Langston, A. K., Alexander, C. R., Alber, M., and Kirwan, M. L.: Beyond 2100: Elevation capital disguises salt marsh vulnerability to sea-level rise in Georgia, USA, Estuar. Coast Shelf S., 249, 107093, https://doi.org/10.1016/j.ecss.2020.107093, 2021.
Lehmann, J. and Kleber, M.: The contentious nature of soil organic matter, Nature, 528, 60–68, 2015.
Li, H., Bölscher, T., Winnick, M., Tfaily, M. M., Cardon, Z. G., and Keiluweit, M.: Simple plant and microbial exudates destabilize mineral-associated organic matter via multiple pathways, Environ. Sci. Technol., 55, 3389–3398, 2021.
Lind, L., Harbicht, A., Bergman, E., Edwartz, J., and Eckstein, R. L.: Effects of initial leaching for estimates of mass loss and microbial decomposition – Call for an increased nuance, Ecol. Evol., 12, e9118, https://doi.org/10.1002/ece3.9118, 2022.
Liu, Z. and Lee, C.: Drying effects on sorption capacity of coastal sediment: The importance of architecture and polarity of organic matter, Geochim. Cosmochim. Ac., 70, 3313–3324, 2006.
Liu, Z., Lee, C., and Aller, R. C.: Drying effects on decomposition of salt marsh sediment and on lysine sorption, J. Mar. Res., 66, 665–689, 2008.
Luk, S., Eagle, M. J., Mariotti, G., Gosselin, K., Sanderman, J., and Spivak, A. C.: Peat decomposition and erosion contribute to pond deepening in a temperate salt marsh, J. Geophys. Res.-Biogeo., 128, e2022JG007063, https://doi.org/10.1029/2022JG007063, 2023.
Luk, S. Y., Todd-Brown, K., Eagle, M., McNichol, A. P., Sanderman, J., Gosselin, K., and Spivak, A. C.: Soil organic carbon development and turnover in natural and disturbed salt marsh environments, Geophys. Res. Lett., 48, e2020GL090287, https://doi.org/10.1029/2020GL090287, 2021.
MacDonald, E., Brummell, M. E., Bieniada, A., Elliot, J., Engering, A., Gauthier, T.-L., Saraswati, S., Touchette, S., Tourmel-Courchesne, L., and Strack, M.: Using the Tea Bag Index to characterize decomposition rates in restored peatlands, Boreal Environ. Res., 23, 221–235, 2018.
Marín-Spiotta, E., Gruley, K. E., Crawford, J., Atkinson, E. E., Miesel, J. R., Greene, S., Cardona-Correa, C., and Spencer, R. G. M.: Paradigm shifts in soil organic matter research affect interpretations of aquatic carbon cycling: transcending disciplinary and ecosystem boundaries, Biogeochemistry, 117, 279–297, 2014.
Mariotti, G., Ceccherini, G., Alexander, C. R., and Spivak, A. C.: Centennial changes of salt marsh area in Coastal Georgia (USA) related to large-scale sediment dynamics by river, waves, and tides, Estuar. Coast., 47, 1498–1516, 2024.
Marley, A. R. G., Smeaton, C., and Austin, W. E. N.: An assessment of the tea bag index method as a proxy for organic matter decomposition in intertidal environments, J. Geophys. Res.-Biogeo., 124, 2991–3004, 2019.
Marschner, B., Brodowski, S., Dreves, A., Gleixner, G., Gude, A., Grootes, P. M., Hamer, U., Heim, A., Jandl, G., and Ji, R.: How relevant is recalcitrance for the stabilization of organic matter in soils?, J. Plant Nutrition Soil Sci., 171, 91–110, 2008.
Megonigal, J. P., Whalen, S. C., Tissue, D. T., Bovard, B. D., Allen, A. S., and Albert, D. B.: A plant-soil-atmosphere microcosm for tracing radiocarbon from photosynthesis through methanogenesis, Soil Sci. Soc. Am. J., 63, 665–671, 1999.
Melillo, J. M., Steudler, P. A., Aber, J. D., Newkirk, K., Lux, H., Bowles, F. P., Catricala, C., Magill, A., Ahrens, T., and Morrisseau, S.: Soil warming and carbon-cycle feedbacks to the climate system, Science, 298, 2173–2176, 2002.
Mikutta, R., Kleber, M., Torn, M. S., and Jahn, R.: Stabilization of soil organic matter: association with minerals or chemical recalcitrance?, Biogeochemistry, 77, 25–56, 2006.
Moran, M. A., Benner, R., and Hodson, R. E.: Kinetics of microbial degradation of vascular plant material in two wetland ecosystems, Oecologia, 158–167, 1989.
Mori, T., Nakamura, R., and Aoyagi, R.: Risk of misinterpreting the Tea Bag Index: Field observations and a random simulation, Ecol. Res., 37, 381–389, 2022.
Morris, J. T. and Bowden, W. B.: A mechanistic, numerical model of sedimentation, mineralization, and decomposition for marsh sediments, Soil Sci. Soc. Am. J., 50, 96–105, 1986.
Morris, J. T., Sundareshwar, P. V, Nietch, C. T., Kjerfve, B., and Cahoon, D. R.: Responses of coastal wetlands to rising sea level, Ecology, 83, 2869–2877, 2002.
Morrissey, E. M., Gillespie, J. L., Morina, J. C., and Franklin, R. B.: Salinity affects microbial activity and soil organic matter content in tidal wetlands, Glob. Change Biol., 20, 1351–1362, 2014.
Mueller, P., Jensen, K., and Megonigal, J. P.: Plants mediate soil organic matter decomposition in response to sea level rise, Glob. Change Biol., 22, 404–414, 2016.
Mueller, P., Schile-Beers, L. M., Mozdzer, T. J., Chmura, G. L., Dinter, T., Kuzyakov, Y., de Groot, A. V., Esselink, P., Smit, C., D'Alpaos, A., Ibáñez, C., Lazarus, M., Neumeier, U., Johnson, B. J., Baldwin, A. H., Yarwood, S. A., Montemayor, D. I., Yang, Z., Wu, J., Jensen, K., and Nolte, S.: Global-change effects on early-stage decomposition processes in tidal wetlands – implications from a global survey using standardized litter, Biogeosciences, 15, 3189–3202, https://doi.org/10.5194/bg-15-3189-2018, 2018.
Newell, S. Y., Fallon, R. D., and Miller, J. D.: Decomposition and microbial dynamics for standing, naturally positioned leaves of the salt-marsh grass Spartina alterniflora, Mar. Biol., 101, 471–481, 1989.
Noyce, G. L., Smith, A. J., Kirwan, M. L., Rich, R. L., and Megonigal, J. P.: Oxygen priming induced by elevated CO2 reduces carbon accumulation and methane emissions in coastal wetlands, Nat. Geosci., 16, 63–68, 2023.
Paludan, C. and Morris, J. T.: Distribution and speciation of phosphorus along a salinity gradient in intertidal marsh sediments, Biogeochemistry, 45, 197–221, 1999.
Pett-Ridge, J., Shi, S., Estera-Molina, K., Nuccio, E., Yuan, M., Rijkers, R., Swenson, T., Zhalnina, K., Northen, T., and Zhou, J.: Rhizosphere carbon turnover from cradle to grave: The role of microbe–plant interactions, Rhizosphere Biology, Springer, Singapore, 51–73, https://doi.org/10.1007/978-981-15-6125-2_2, 2021.
Pinheiro, J., Bates, D., and R Core Team: _nlme: Linear and Nonlinear Mixed Effects Models_, R package version 3.1-162, https://CRAN.R-project.org/package=nlme (last access: 21 January 25), 2016.
Puppin, A., Roner, M., Finotello, A., Ghinassi, M., Tommasini, L., Marani, M., and D'Alpaos, A.: Analysis of Organic Matter Decomposition in the Salt Marshes of the Venice Lagoon (Italy) Using Standard Litter Bags, J. Geophys. Res.-Biogeo., 128, e2022JG007289, https://doi.org/10.1029/2022JG007289, 2023.
Reed, D. J. and Cahoon, D. R.: The relationship between marsh surface topography, hydroperiod, and growth of Spartina alterniflora in a deteriorating Louisiana salt marsh, J. Coast Res., 8, 77–87, 1992.
Rice, D. L. and Tenore, K. R.: Dynamics of carbon and nitrogen during the decomposition of detritus derived from estuarine macrophytes, Estuar. Coast Shelf S., 13, 681–690, 1981.
Seelen, L. M. S., Flaim, G., Keuskamp, J., Teurlincx, S., Font, R. A., Tolunay, D., Fránková, M., Šumberová, K., Temponeras, M., and Lenhardt, M.: An affordable and reliable assessment of aquatic decomposition: Tailoring the Tea Bag Index to surface waters, Water Res., 151, 31–43, 2019.
Seyfferth, A. L., Bothfeld, F., Vargas, R., Stuckey, J. W., Wang, J., Kearns, K., Michael, H. A., Guimond, J., Yu, X., and Sparks, D. L.: Spatial and temporal heterogeneity of geochemical controls on carbon cycling in a tidal salt marsh, Geochim. Cosmochim. Ac., 282, 1–18, 2020.
Spivak, A. C.: Decomposition, porewater, plant and animal collection, and soil temperature data in Airport Marsh, Sapelo Island, 7/2019–7/2020, Georgia Coastal Ecosystems LTER Project, University of Georgia, Long Term Ecological Research Network [data set], https://doi.org/10.6073/pasta/a47f22f05b4a3a0f93f8ae4c0af5b291, 2024.
Spivak, A. C. and Reeve, J.: Rapid cycling of recently fixed carbon in a Spartina alterniflora system: a stable isotope tracer experiment, Biogeochemistry, 125, 97–114, 2015.
Spivak, A. C., Sanderman, J., Bowen, J. L., Canuel, E. A., and Hopkinson, C. S.: Global-change controls on soil-carbon accumulation and loss in coastal vegetated ecosystems, Nat. Geosci., 12, 685–692, 2019.
Spivak, A. C., Pinsonneault, A. J., Hintz, C., Brandes, J., and Megonigal, J. P.: Ephemeral microbial responses to pulses of bioavailable carbon in oxic and anoxic salt marsh soils, Soil Biol. Biochem., 185, 109157, https://doi.org/10.1016/j.soilbio.2023.109157, 2023.
Tang, H., Nolte, S., Jensen, K., Rich, R., Mittmann-Goetsch, J., and Mueller, P.: Warming accelerates belowground litter turnover in salt marshes – insights from a Tea Bag Index study, Biogeosciences, 20, 1925–1935, https://doi.org/10.5194/bg-20-1925-2023, 2023.
Tao, F., Huang, Y., Hungate, B. A., Manzoni, S., Frey, S. D., Schmidt, M. W. I., Reichstein, M., Carvalhais, N., Ciais, P., and Jiang, L.: Microbial carbon use efficiency promotes global soil carbon storage, Nature, 618, 981–985, 2023.
Turner, R. E., Milan, C. S., and Swenson, E. M.: Recent volumetric changes in salt marsh soils, Estuar. Coast Shelf S., 69, 352–359, 2006.
Valiela, I., Teal, J. M., Allen, S. D., Van Etten, R., Goehringer, D., and Volkmann, S.: Decomposition in salt marsh ecosystems: the phases and major factors affecting disappearance of above-ground organic matter, J. Exp. Mar. Biol. Ecol., 89, 29–54, 1985.
Van Der Nat, F.-F. W. A., Middelburg, J. J., Van Meteren, D., and Wielemakers, A.: Diel methane emission patterns from Scirpus lacustris and Phragmites australis, Biogeochemistry, 41, 1–22, 1998.
Wilson, C. A., Hughes, Z. J., and FitzGerald, D. M.: The effects of crab bioturbation on Mid-Atlantic saltmarsh tidal creek extension: Geotechnical and geochemical changes, Estuar. Coast Shelf S., 106, 33–44, 2012. Wilson, J. O., Valiela, I., and Swain, T.: Carbohydrate dynamics during decay of litter of Spartina alterniflora, Mar. Biol., 92, 277–284, 1986.
Windham, L.: Comparison of biomass production and decomposition between Phragmites australis (common reed) and Spartina patens (salt hay grass) in brackish tidal marshes of New Jersey, USA, Wetlands, 21, 179–188, 2001.
Wu, F., Pennings, S. C., Ortals, C., Ruiz, J., Farrell, W. R., McNichol, S. M., Angelini, C., Spivak, A. C., Alber, M., and Tong, C.: Disturbance is complicated: Headward-eroding saltmarsh creeks produce multiple responses and recovery trajectories, Limnol. Oceanogr., 67, S86–S100, 2022.
Xiao, K., Wilson, A. M., Li, H., Santos, I. R., Tamborski, J., Smith, E., Lang, S. Q., Zheng, C., Luo, X., and Lu, M.: Large CO2 release and tidal flushing in salt marsh crab burrows reduce the potential for blue carbon sequestration, Limnol. Oceanogr., 66, 14–29, 2021.
Yousefi Lalimi, F., Silvestri, S., D'Alpaos, A., Roner, M., and Marani, M.: The spatial variability of organic matter and decomposition processes at the marsh scale, J. Geophys. Res.-Biogeo., 123, 3713–3727, 2018.
Yvon-Durocher, G., Jones, J. I., Trimmer, M., Woodward, G., and Montoya, J. M.: Warming alters the metabolic balance of ecosystems, Philos. T. R. Soc. B, 365, 2117–2126, 2010.