the Creative Commons Attribution 4.0 License.
the Creative Commons Attribution 4.0 License.
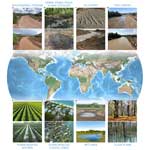
Reviews and syntheses: Variable inundation across Earth's terrestrial ecosystems
Amy J. Burgin
Michelle H. Busch
Joshua B. Fisher
Joshua Ladau
Jenna Abrahamson
Lauren Kinsman-Costello
Xingyuan Chen
Thibault Datry
Nate McDowell
Corianne Tatariw
Anna Braswell
Jillian M. Deines
Julia A. Guimond
Peter Regier
Kenton Rod
Edward K. P. Bam
Etienne Fluet-Chouinard
Inke Forbrich
Kristin L. Jaeger
Teri O'Meara
Tim Scheibe
Erin Seybold
Jon N. Sweetman
Jianqiu Zheng
Daniel C. Allen
Elizabeth Herndon
Beth A. Middleton
Scott Painter
Kevin Roche
Julianne Scamardo
Ross Vander Vorste
Kristin Boye
Ellen Wohl
Margaret Zimmer
Kelly Hondula
Maggi Laan
Anna Marshall
Kaizad F. Patel
The structure, function, and dynamics of Earth's terrestrial ecosystems are profoundly influenced by how often (frequency) and how long (duration) they are inundated with water. A diverse array of natural and human-engineered systems experience temporally variable inundation whereby they fluctuate between inundated and non-inundated states. Variable inundation spans extreme events to predictable sub-daily cycles. Variably inundated ecosystems (VIEs) include hillslopes, non-perennial streams, wetlands, floodplains, temporary ponds, tidal systems, storm-impacted coastal zones, and human-engineered systems. VIEs are diverse in terms of inundation regimes, water chemistry and flow velocity, soil and sediment properties, vegetation, and many other properties. The spatial and temporal scales of variable inundation are vast, ranging from sub-meter to whole landscapes and from sub-hourly to multi-decadal. The broad range of system types and scales makes it challenging to predict the hydrology, biogeochemistry, ecology, and physical evolution of VIEs. Despite all experiencing the loss and gain of an overlying water column, VIEs are rarely considered together in conceptual, theoretical, modeling, or measurement frameworks and approaches. Studying VIEs together has the potential to generate mechanistic understanding that is transferable across a much broader range of environmental conditions, relative to knowledge generated by studying any one VIE type. We postulate that enhanced transferability will be important for predicting changes in VIE function in response to global change. Here we aim to catalyze cross-VIE science that studies drivers and impacts of variable inundation across Earth's VIEs. To this end, we complement expert mini-reviews of eight major VIE systems with overviews of VIE-relevant methods and challenges associated with scale. We conclude with perspectives on how cross-VIE science can derive transferable understanding via unifying conceptual models in which the impacts of variable inundation are studied across multi-dimensional environmental space.
- Article
(9876 KB) - Full-text XML
- BibTeX
- EndNote
The chemical and biological processes within terrestrial ecosystems hinge on the presence, residence time, volume, and chemistry of water (Arias-Real et al., 2024; Lohse et al., 2009; Schimel et al., 1991). A variety of factors influence water retention, infiltration, flow, and surface expression within an ecosystem, such as land surface relief, topographic slope, subsurface permeability, evapotranspiration, and human-based modifications of the landscape (Appels et al., 2016; Horton, 1940; McDowell et al., 2023; McGrane, 2016; Orozco-López et al., 2018; Ribolzi et al., 2011). Water supply is most commonly “top-down” in the form of precipitation and overland flow or “bottom-up” due to rising water tables and transient saturation in the subsurface (Freeze, 1974; Smith et al., 2017; Stewart et al., 2019). Inundation, however, may also occur because of lateral inputs, as is common in tidal systems, or because of upslope inputs, as in floodplains. Regardless of where water comes from, its expression at the land–atmosphere interface occurs when the rate of water supply is greater than the rate of export via infiltration, evapotranspiration, and runoff (Tromp-van Meerveld and McDonnell, 2006).
Here, we define inundation as occurring when there is an uninterrupted aqueous barrier that limits diffusive gas exchange at the land–atmosphere interface (Elberling et al., 2011; Smith et al., 2018). This conceptualization includes diverse hydrological conditions ranging from freestanding water to soil surface saturation. Hence, our broad definition spans extreme events such as hurricane-driven inundation to shallow intermittent overland runoff across hillslopes. This definition does not attempt to separate “inundation” from “flooding” based on temporal frequency and duration, as this has been proposed elsewhere (Flick et al., 2012). To avoid confusion from interchangeable use of these two words (as in US Army Corps of Engineers, 2024), we exclusively use “inundation” and avoid references to “flooding” in this paper. We define variably inundated ecosystems (VIEs) as areas of any spatial and temporal scale that experience transitions between the presence and absence of inundated conditions. Variable inundation is natural in many systems and can be critical to system function (Shaeri Karimi et al., 2022; Tsoi et al., 2022), while in other systems it represents a disturbance (Hopple et al., 2023; Sun et al., 2022a). Variably inundated ecosystems cover at least 5×106–9×106 km2, or 4 %–7 %, of Earth's land surface excluding Greenland and Antarctica. These estimates are according to monthly data over multiple decades (Davidson et al., 2018; Zhang et al., 2017, 2021). Current areal estimates of VIEs may, however, be underestimates as many VIEs are not detectable with current remote sensing techniques.
Variable inundation occurs across a wide range of terrestrial ecosystems, but the factors governing its influences are typically studied independently without cross-ecosystem comparisons. Some examples of VIEs are hillslopes with overland flow, non-perennial streams, floodplains and parafluvial zones, variably inundated wetlands, vernal ponds/pools/playas, tidal systems, coastal systems impacted by storm-driven inundation, and human-engineered systems intended to shift inundation dynamics (e.g., flood-irrigated agriculture, stormwater infrastructure, and constructed wetlands) (Fig. 1). A given system may not fit clearly into a single VIE category and/or may transition across categories through time. For example, when flow ceases and isolated pools form in a non-perennial stream network, the stream begins to behave more like a wetland or vernal pond as opposed to a flowing stream (Day et al., 2019). Further, while VIEs may be classified as wetlands under the broadest definition of the Ramsar Convention on Wetlands (Ramsar Secretariat, 2016), there is significant variation in how wetlands are defined (Finlayson and Van Der Valk, 1995), and we do not attempt to rectify or clarify variation in those definitions. Here, when using the term “wetland”, we simply align with the perspective that wetlands are similar to marshes, swamps, and bogs.
Inundation dynamics are changing due to increased variability and magnitudes of precipitation and evapotranspiration, accelerated sea level rise, and human modifications to Earth's land surface, giving rise to an increase in extreme events (Konapala et al., 2020; Li et al., 2022a). For example, extreme events such as coastal inundation are increasingly frequent (Vitousek et al., 2017). However, inundation patterns are changing in different ways across different VIEs (Londe et al., 2022; Zipper et al., 2021). For example, in river systems, seasonal drying is becoming more common in multiple biomes (Sweet et al., 2014; Zipper et al., 2021), while some rivers are shifting from non-perennial to perennial (Datry et al., 2018c; Döll and Schmied, 2012) and others have fewer no-flow days than they did historically (Zipper et al., 2021). Divergence in the direction of change, with some systems being inundated less and others being inundated more, is likely linked to diverse drivers of change associated with changing climates and/or direct human impacts (Datry et al., 2023). Therefore, researchers and decision-makers cannot rely exclusively on historical trends to predict future impacts (e.g., on species diversity) of changing inundation dynamics (Culley et al., 2016; Li et al., 2022b; Quinn et al., 2018; Rameshwaran et al., 2021).
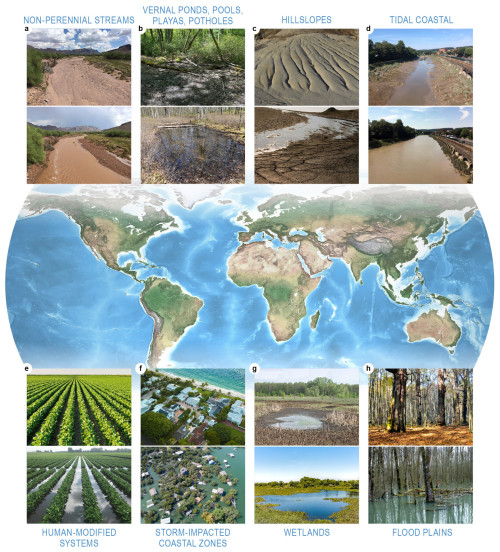
Figure 1Variably inundated ecosystems (VIEs) span numerous ecosystem types and are globally distributed across Earth's land surface. There are few places across Earth's land surfaces that do not experience variable inundation, which is defined here as the loss/gain of an aqueous barrier between the atmosphere and porous media (e.g., soil) that inhibits gas-phase transport. Due to global changes in the dynamics of variable inundation, there is a need to integrate knowledge into models that are predictive across VIEs. This will require intentionally studying VIEs together to understand how the details of any given VIE modulate the impacts of variable inundation. Credit: Nathan Johnson. There are several photos, used with permission, from different sources as follows: (a) Sullivan et al. (2019); (b) Jon Sweetman, co-author; (c) Shutterstock; (d) WeirdBristol (2018); (central global image, e, f, g) Shutterstock; and (h) Mikac et al. (2018).
Mechanistic knowledge that is transferable (per Schuwirth et al., 2019) across inundation regimes (i.e., from extreme events to predictable cycling) and across VIEs is required to develop hydrologic, biogeochemical, and ecological models that are predictive across contemporary and future conditions. We envision the impacts of variable inundation as dependent on the location of any given VIE within multi-dimensional environmental space. This space can be defined with a variety of environmental variables such as inundation return interval and duration, topographic slope, geology, vegetation composition, precipitation, salinity, and temperature. Similarly to multi-dimensional niche space (Hutchinson, 1978), many other variables could be used, but regardless, environmental change will alter the position of VIEs within continuous, multi-dimensional environmental space. Predicting future impacts of variable inundation requires mechanistic understanding of how the location of a VIE in this multi-dimensional space influences those potential impacts. We propose that our best chance to achieve such understanding is to generate knowledge of variable inundation impacts that is transferable across VIEs.
Here we aim to catalyze cross-VIE science for the pursuit of transferable knowledge and ultimately models that are predictive across and aid in conserving contemporary and future VIEs. We briefly summarize high-level divergences in drivers of variable inundation and commonalities in the impacts of variable inundation, and then we present expert mini-reviews of eight major VIE systems. Variable inundation occurs across vast ranges in spatial and temporal scales, which presents challenges to cross-VIE science. Therefore, we present an overview of these challenges and offer suggested solutions along with a summary of methods that are most relevant to VIE science. We conclude with perspectives on how cross-VIE science can use conceptual models based on environmental continuums to derive transferable understanding that is useful for protecting these systems and their biodiversity.
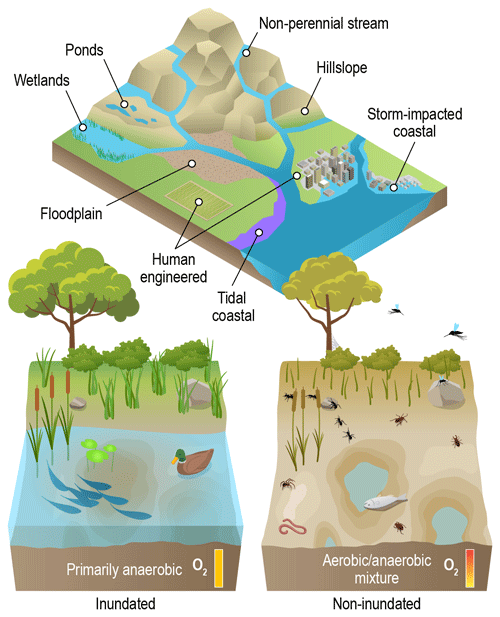
Figure 2Conceptual overview of where different types of VIEs are often found within watersheds and some common shifts in system states across inundated and non-inundated conditions. VIEs are found in locations from headwaters to coastal environments (top), and the impacts of variable inundation have some consistencies across these diverse landscapes (bottom). Organismal ecology, physiology, and demographics are altered by variable inundation, leading to shifts in community composition. Biogeochemical processes also shift, such as greater gas-phase transport of oxygen into soil/sediment when surface water is lost. A key goal for cross-VIE science is to mechanistically understand variation in the impacts of variable inundation across multi-dimensional environmental space. Credit: Nathan Johnson.
The drivers of variable inundation differ markedly across VIEs and are linked to factors such as long-term drought, heavy precipitation, evapotranspiration, changing groundwater storage, soil/sediment properties, extreme climatic events, and dams (Arnold et al., 2023; Bourke et al., 2023; Glaser et al., 2021; Shanafield et al., 2021; Swenson et al., 2024). This leads to significant variation across VIEs in inundation regimes, which includes variations in inundation timing, return intervals, duration, spatial extent, depth, and flow rates (Celi and Hamilton, 2020; Dee and Tank, 2020; Van Appledorn et al., 2021). For example, sediments within the active channel of tidal rivers can experience sub-daily losses and gains of surface water (Tagestad et al., 2021), while other coastal zones may experience extreme inundation events at a 100-year return interval (Clementson et al., 2021; Slater et al., 2021). Other systems, such as non-perennial streams and vernal ponds, also experience a broad range of inundation regimes, ranging from sporadic and extreme inundation following rain events to more regular seasonal cycles (Allen et al., 2020; Barczok et al., 2023). Variation in the spatial scale of inundation is also large, with floodplains and storm-impacted coastal zones experiencing inundation over tens of kilometers, whereas non-perennial streams and ponds can experience changes across a few meters (Allen et al., 2020; Hamilton et al., 2002; Vousdoukas et al., 2016). As discussed below, within the series of VIE mini-reviews, the temporal and spatial scales of inundation also vary substantially within each type of VIE. Variation within a given type of VIE is large enough for us to suggest it cannot be used to clearly differentiate VIEs into named categories. As discussed in the “Towards cross-VIE transferable understanding” section, this is one motivation for pursuing VIE conceptual models and investigations that span broad continuums of environmental conditions.
Variable inundation impacts physical (e.g., sediment transport; Peruccacci et al., 2017; Siev et al., 2019), chemical (e.g., water quality; Whitworth et al., 2013), and biological/ecological (e.g., invertebrate communities; Plum, 2005) attributes of both natural and anthropogenically modified ecosystems, in addition to impacting human society (Dube et al., 2021) (Fig. 2). Due to intense periods of inundation and drought, these systems are often referred to as hotspots or ecosystem control points (Arias-Real et al., 2024; Bernhardt et al., 2017), with disproportionately high reaction rates or areas of high diversity (Davidson et al., 2012; Palta et al., 2014). In a qualitative sense, some of these impacts are common across VIEs even if the quantitative details vary.
During inundated periods, biogeochemical processes in VIEs often shift from a dominance of aerobic respiration during drier periods to a diverse suite of anaerobic processes, such as methanogenesis (Datry et al., 2018a; Hondula et al., 2021a). Changes in the frequency of inundation events change the dynamics of dry–wet, hot–cold, and aerobic–anaerobic transitions (Valett et al., 2005). Such dynamics can challenge existing theories. For example, while rates of soil respiration are expected to peak under aerobic conditions, periodic anaerobic conditions can lead to unexpectedly high rates of soil carbon loss (Huang et al., 2021), and the anaerobic process of methanogenesis can be fastest in well-oxygenated dry soils (Angle et al., 2017). More broadly, variable inundation can alter fluxes of greenhouse gases to the atmosphere, such as the common observation of soil rewetting leading to significant carbon loss arising from sudden intensification of soil respiration (Schimel, 2018; Shumilova et al., 2019). Variation in inundation also has large impacts on the global CH4 budget (Peng et al., 2022; Zhang et al., 2017), and rewetting of dry sediment in intermittent streams can contribute considerably to the total CO2 emissions from streams (von Schiller et al., 2019). More generally, top-down and bottom-up hydrologic inundation events broadly influence biogeochemical cycles (Smith et al., 2017) and can result in hysteretic responses to wetting and drying (Patel et al., 2022).
Across VIEs, inundation impacts the structure, composition, and function of vegetation communities. Growth and survival can either increase or decrease with inundation depending on local aridity and the impacts on soil hypoxia. Hypoxia kills roots, leading to reduced water uptake, reduced photosynthesis, mortality, and shifts in vegetation composition (Cubley et al., 2023; McDowell et al., 2022; Pedersen et al., 2021). More broadly, inundation dynamics impact organismal ecology (Datry et al., 2023) across all VIEs, such as herbivores responding to inundation-induced shifts in vegetation (De Sassi et al., 2012). Inundation can also alter arthropod communities, leading to reductions in diversity, abundance, and biomass (Plum, 2005). Changes at the base of food webs can have further cascading effects (Chen and Wise, 1999).
To pursue cross-VIE science requires knowledge of the diverse array of ecosystems that can be considered VIEs. Researchers that design and carry out cross-VIE studies may be considered generalists in terms of the breadth of systems they study, even if their science questions are highly specialized. To facilitate such researchers in the pursuit of cross-VIE science, we go beyond the high-level summaries of divergences and commonalities (above) and provide expert mini-reviews of eight primary VIE types. The following subsections present these mini-reviews, which summarize system characteristics, drivers, and impacts of variable inundation with an emphasis on biogeochemistry and organismal ecology and opportunities to better understand spatiotemporal patterns and impacts of variable inundation. Each mini-review is accompanied by a graphic that provides either a conceptual overview or imagery-based examples, with the goal of collectively touching on key drivers, dynamics, impacts, and tangible system examples. The collection is not meant to be a comprehensive classification of all possible VIE types. It does cover a broad range of VIEs and is meant to serve as an overview of individual VIEs to provide context for later sections of this paper. The sequence of mini-reviews roughly follows the flow of water moving from hillslopes to coastal environments (Fig. 2) and includes variably inundated components of (i) hillslopes, (ii) non-perennial streams, (iii) riverine floodplains and parafluvial zones, (iv) wetlands, (v) temporary ponds, (vi) storm-impacted coastal zones, and (vii) tidal systems. The final mini-review (viii) is focused on ecosystems that have been engineered to modify inundation regimes, which occur throughout the continuum from hillslopes to coasts.
We separate VIEs into categories as a heuristic simplification that allows for an appreciation of variation and commonalities in drivers, impacts, and opportunities. We anticipate that the disciplinary foci of individual researchers will align most closely with a subset of the summarized VIE types. One goal of this paper is to facilitate researchers to think about how their science applies across multiple VIEs. We emphasize that in many (and maybe all) cases there is not a clear distinction among the types of VIEs we discuss below (e.g., non-perennial streams can be inundated due to storm surge, resulting in floodplains or parafluvial zones). Ultimately, we encourage a continuum perspective that does not rely on discrete system names or hard boundaries and instead views VIEs across multi-dimensional environmental space based on inundation regimes and physical settings such as the topographic slope.
This continuum perspective is more fully developed as a conceptual model in the final section of the paper, titled “Towards cross-VIE transferable understanding”. However, we briefly summarize here that it is based on two continuous environmental axes: the inundation return interval and topographic slope. These variables can be used to define a two-dimensional environmental space that contains all VIE systems. With this model, impacts of variable inundation can be studied across environment space instead of within discrete named types of VIEs. When going through the following mini-reviews, we encourage the reader to conceptualize each VIE type in the context of the return interval and slope (e.g., hillslopes may have a long return interval and steep slopes relative to tidal systems, while coastal systems inundated by storms may have slopes similar to those of tidal systems but much longer return intervals). When VIEs are viewed through a unified lens of environmental continuums, larger interdisciplinary questions may be answered.
2.1 Hillslopes with surface runoff
Hillslopes provide water to lower-lying areas, often concentrating the water in gullies and depressions (Fig. 3). Hillslopes produce relatively transient VIE features and may often be seen as extensions of other VIEs, such as hillslope seeps co-located with a wetland or the unchannelized swales that contribute to a non-perennial stream network. In cold regions, snow, ice, and permafrost can create an impermeable layer, resulting in near-surface soil being inundated for days to weeks during spring thaw (Coles et al., 2017; Patel et al., 2020). In dry regions, intense precipitation that exceeds the local infiltration capacity can result in water ponding on the surface of hillslopes or overland flow generation down hillslopes, which can be exacerbated by the initial hydrophobicity of dry soil (Kirkby et al., 2002). Exceeding the infiltration capacity is more likely on hillslopes with low permeability, such as on clay-rich soil or when near-surface soils are frozen. This can be exacerbated by restrictive soil horizons located at shallow depths across hillslopes that generate seasonal perched water tables and lead to inundation (McDaniel et al., 2008). Overland flow can be spatially heterogeneous due to variations in soil characteristics as well as flow accumulation, leading to infiltration or exfiltration along the hillslope (Betson and Marius, 1969).
In forested hillslopes, soil infiltration often exceeds rainfall intensity (Burt and Swank, 2010; McDonnell et al., 2009) and lateral flow towards topographic depressions can lead to saturation and ponding (Anderson and Burt, 1978) (Fig. 3a). Microtopography within hillslopes (Fig. 3b) can also lead to temporary ponding, e.g., from rain in tropical environments and from spring snowmelt in colder environments (Clark et al., 2014). Toe slopes can generate wedges of saturation that grow upslope (Choularton and Perry, 1986; Weyman, 1973), although subsurface saturation and ponding can also occur on upper slopes where the soil is thinner (e.g., Tromp-van Meerveld and McDonnell, 2006). Finally, spatial variation in topographic characteristics (e.g., aspect, slope, curvature) can result in differences in soil moisture, incoming energy, and vegetation, affecting evapotranspiration and inundation patterns (McVicar et al., 2007).
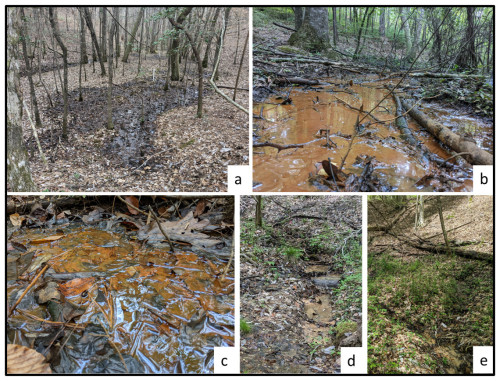
Figure 3Examples of variable inundation along hillslopes: (a) looking downslope at an inundated slope, (b) ponding with no flow due to microtopography, (c) sheet wash with directional flow across the surface of a hillslope, (d) rill formation with turbid water from erosion, and (e) vegetation community change on a slope due to differences in soil moisture. All photos taken by Corianne Tatariw at Tanglewood Forest, Alabama.
Surface runoff and inundation on hillslopes can result in the export of soil nutrients, salinization of soil from groundwater seeps, erosion, and landslides. There is a balance between the effects of variable inundation on hillslope vegetation and erosion. In water-limited systems, inundation can increase plant productivity and diversity, as well as increasing the rooting strength of soils (Zhao et al., 2022) (Fig. 3e). However, increased inundation can also lead to increased chemical weathering and lower shear strength in hillslope soils during storms, leading to higher erosion and landslide potential. Along with erosion, landslides and soil compaction are inherent to many hillslopes, which also can create areas ripe for inundation (Bogaard and Greco, 2016). At shoulder and midslope positions, increased overland flow due to saturation- or infiltration excess increases sediment detachment, which is then deposited on foot and toe slopes (Huang et al., 2002). The transport of particles also leads to the transport of nutrients that are sorbed to the particles, such as phosphorus. Erosion can be concentrated in rills and gullies or can spread out across a slope as “sheet wash” that impacts large areas of hillslopes (Fig. 3c, d). Impacts of erosion are dependent on interactions between the persistence of inundation and soil properties (Thomas et al., 2020).
The aqueous chemistry of water that is transported over hillslope surfaces reflects the chemistries of contributing water sources such as precipitation, shallow soil water, and exfiltrating groundwater. Shallow soils on hillslopes have abundant organic materials and nutrients (Herndon et al., 2015). Whereas organic matter decreases with depth, solutes derived from the parent rock material increase with depth (Brantley et al., 2017). These stratifications collectively regulate source water chemistry on hillslopes. Dry-to-wet transitions shift flow paths from groundwater to soil water dominance in streams, therefore shaping stream chemistry (Stewart et al., 2022; Zhi and Li, 2020). Dry-to-wet transitions also shift water content and pore space oxygen concentrations (Jarecke et al., 2016; Smyth et al., 2019), often triggering the release of a cascade of solutes produced under anaerobic conditions (Schlesinger and Bernhardt, 2020). These entangled, complex interactions among hydrological and biogeochemical processes often challenge the differentiation of individual processes and mechanistic understanding of how variable inundation regulates flow paths, reactions, stream chemistry, and solute and gas export fluxes (Li et al., 2021).
Investigations of variably inundated hillslopes present significant and challenging research opportunities due to their inherently dynamic nature. One key challenge is quantifying the occurrence and spatial extent of hillslope VIEs across the globe. Remote sensing could be used to identify and quantify these areas, spatially and temporally, based on sky-visible vegetation (e.g., plant morphologies, leaf nutrient contents) (Hwang et al., 2012; Tai et al., 2020) and topographic signatures (e.g., erosional patterns) (Trochim et al., 2016) caused by variable inundation. To fully understand the ecological and biogeochemical impacts of variable inundation on hillslopes, research needs to focus on shallow subsurface physical properties, hydrology, and their linkage to biogeochemical processes. This can be pursued via environmental geophysics to map and characterize the influence of subsurface restrictive layers (Fan et al., 2019, p. 201). Understanding the subsurface soil architecture is key to predicting variable inundation from bottom-up and top-down water sources, along with the follow-on impacts on ecology and biogeochemistry.
2.2 Non-perennial streams
Non-perennial streams, defined as rivers and streams that cease to flow at some point in either space or time (Busch et al., 2020), are ubiquitous and comprise 50 %–60 % of the global river length (Messager et al., 2021). These systems occur across all continents and biomes (Messager et al., 2021). Streamflow in non-perennial streams ranges from nearly perennial (year-round) flow to seasonal flow, responding to drivers like snowmelt, to daily or sub-daily flow events, responding to rainfall events or evapotranspiration (Price et al., 2021). At the reach scale, non-perennial streams shift between three main states – flowing, ponded/pooled, or no surface water present (Fig. 4). As reaches become hydrologically connected (or disconnected), the spatial footprint/extent of the connected stream network can grow (or shrink) over sub-daily to seasonal to interannual timescales (Xiao et al., 2019). Spatial and temporal shifts among the three hydrologic states strongly influence the network's capacity to process, transport, and export material to downstream systems (Allen et al., 2020).
The high variability in the spatial and temporal scales of streamflow intermittency is indicative of the complex set of interacting drivers that induce stream drying. At the global and regional scales, the degree of aridity is a primary control on the abundance of non-perennial streams (Hammond et al., 2021; Zipper et al., 2021). At smaller scales, catchment properties exert strong control over both the capacity of water delivery to the channel and the subsequent balance between the channel and near-subsurface capacity to transport water (Hammond et al., 2021; Price et al., 2021; Zipper et al., 2021). Non-perennial flow can occur anywhere in the steam network, from headwaters to higher-order rivers. While some networks display longitudinal transitions from non-perennial to perennial flow (or vice versa), other networks exhibit more complex patterns in surface water flow and connectivity, which may be driven by topography, geology, vegetation, or groundwater abstraction/use (Costigan et al., 2015, 2016).
The variable inundation dynamics in non-perennial streams have cascading implications for biogeochemical cycling, water quality, ecosystem function, and community ecology. Under non-flowing conditions, riverbeds are characterized by dry conditions or discontinuous and stagnant water pools, often with high temperatures, low dissolved oxygen levels, and long residence times, functioning more like soils (Arce et al., 2019), as described also in the “Hillslopes with surface runoff” section. Pooled, non-flowing conditions can lead to steep redox gradients in the shallow subsurface that drive nutrient processing (Datry and Larned, 2008; DelVecchia et al., 2022; Gómez-Gener et al., 2021). During dry/non-flowing states, terrestrial organic matter accumulates in the channel and is subjected to varying degrees of breakdown (Datry et al., 2018b; Del Campo et al., 2021). Rewetting of accumulated substrates can stimulate microbial activity and nutrient attenuation (Saltarelli et al., 2022) and generate pulses of greenhouse gases such as CO2 and N2O (Datry et al., 2018c; Song et al., 2018). During rewetting and resumption of flow, non-perennial streams can contain large numbers of terrestrial and aquatic organisms that can be flushed downstream (Corti and Datry, 2012; Rosado et al., 2015), with high sediment, dissolved organic carbon, and solutes (Blaurock et al., 2021; Fortesa et al., 2021; Herndon et al., 2018; Hladyz et al., 2011; Laronne and Reid, 1993; Wen et al., 2020).
Biological responses to rewetting depend on the distribution of habitats and biota at the watershed scale and the duration of the preceding dry phase. In highly dynamic river systems, such as braided rivers, drying and wetting cycles can be spatially patchy and short-lived but frequent, and thus ecological recovery following wetting can be very rapid due to the very active hyporheic zones characterizing these systems (Arscott et al., 2002; Vorste et al., 2016). In other systems, recovery can be slow, depending on the proximity of refuges, such as springs, isolated pools, and perennial reaches (Fournier et al., 2023; Sarremejane et al., 2021). Systems with frequent and severe drying events are more likely to be colonized by aerial or other overland dispersers than by aquatic dispersers (Bogan et al., 2017a; Bonada et al., 2007; Sarremejane et al., 2021). Life-history events of some species coincide with predictable rewetting events, such as post-snowmelt fish spawning (Hooley-Underwood et al., 2019) and amphibian and insect life histories (Bogan et al., 2017a). Rewetting also partly determines the germination success and establishment of riparian vegetation (Merritt and Wohl, 2002).
Compared to their perennial counterparts, non-perennial streams have received less research and monitoring attention and tend to be undervalued relative to the ecological/functional performance of perennial streams (Palmer and Hondula, 2014). As a consequence, many of the pressing research needs in non-perennial streams are limited by data availability (Van Meerveld et al., 2020; Zimmer et al., 2022). Non-perennial streams are systematically under-represented in global gaging networks (Krabbenhoft et al., 2022; Messager et al., 2021), leading to major gaps in our understanding of the timing, magnitude, and duration of flow in diverse non-perennial streams. In addition, our ability to predict the onset or cessation of flowing periods is limited by a lack of gaging. Infrequent grab sampling for water chemistry tends to undersample non-perennial streams specifically, leading to an even greater paucity of biogeochemical data from these systems, particularly during rapid rewetting events. Spatially explicit data on streamflow intermittency and subsurface conditions at fine spatial scales (tens of meters) remain limited to a few intensively studied catchments (e.g., Zimmer and McGlynn, 2017). While some global-scale datasets on streamflow intermittency have been developed (Messager et al., 2021), the resolution of these products necessarily omits smaller headwater reaches, hindering our ability to quantify hydrologic and biogeochemical processes in non-perennial streams broadly (Benstead and Leigh, 2012).
Major challenges and opportunities include accurate mapping of non-perennial streams and accurate predictions of flow timing at annual, seasonal, and shorter timescales while also accounting for spatial scales. Headwaters, which are small, numerous, and often non-perennial (Kampf et al., 2021), are difficult to map and understand hydrologically, leading to knowledge gaps in the hydrological integrity of ecosystems at regional scales (Benstead and Leigh, 2012; Dugdale et al., 2022). While challenges remain, the use of drones and thermal infrared remote sensing could connect field observations with modeling for better understanding of the hydrology of these valuable systems (Dugdale et al., 2022). In addition to mapping issues, limited time series data make predictions of flow in terms of duration, frequency, and spatial extent challenging. How the timing and frequency of flow will change under climate change remains an open question. It is expected that an increased frequency and duration of droughts will shift streams towards more non-perennial flow states (Döll and Schmied, 2012). In contrast, flow permanence may increase in select areas where streams are fed by melting glaciers or snowpack or where anthropogenic intervention occurs (Datry et al., 2023). The changing frequency of extreme flow events and rapid no-flow–high-flow oscillations also have the potential to further alter streamflow, biogeochemical processes, and organismal ecology in non-perennial streams, necessitating further integrated hydro-biogeochemical studies in these dynamic systems.
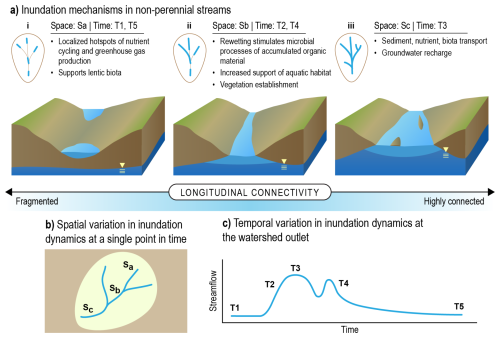
Figure 4Conceptual model of variable inundation in non-perennial streams. (a) Water connections between groundwater, near-surface, and surface regions at locations within a given network result in varying degrees of longitudinal connectivity with associated biogeochemical processes. (b) At a single snapshot in time, water connections result in spatial variation in surface water inundation. (c) Under time-varying flow states, the extent of surface inundation will also vary at a given location. Inundation mechanisms depicted in (a) represent a losing system that is transitioning to a flowing state. We acknowledge that in some systems, a low-flow fragmented state also occurs in gaining streams with locally connected groundwater. Spatial variation is signified by Sa–Sc, and temporal variation is signified by T1–T5. Credit: Nathan Johnson.
2.3 Floodplains and parafluvial zones
Rivers, both perennial and non-perennial, create two types of VIEs, floodplains and parafluvial zones (Fig. 5). Floodplains are alluvial landforms generated by river erosion and deposition and hydrologically connected to the contemporary active channel but outside the active river channel (Nanson and Croke, 1992). Parafluvial zones are areas in the active channel without surface water at low flow, i.e., at higher-elevation areas within an active channel that contains perennial flow (Goldman et al., 2017). Nearly all rivers have parafluvial zones and adjacent floodplains, although these may be longitudinally discontinuous (e.g., absent where the river flows through a narrow bedrock gorge or descends into the subsurface). Consequently, the global distribution of these environments is extensive, as few terrestrial surfaces do not include a river network.
Spatial scales of inundation in floodplains and parafluvial zones are variable between rivers and through time along a river. Fundamentally, spatial scales are governed by the interaction between the magnitude of flow and available space as defined by topography (Nardi et al., 2006). Floodplains of the world's largest rivers such as the Amazon, Congo, or Mississippi can extend laterally for kilometers on both sides of the active channel (Arnesen et al., 2013). In contrast, the floodplain of a headwater channel in high-relief terrain may be only 1–2 m wide on each side of the channel (Adams and Spotila, 2005).
Temporal scales of inundation (e.g., frequency, periodicity, intensity) vary substantially across climates, topographic regions, and river network positions. A snowmelt-dominated or monsoon-fed river will have regular annual inundation that lasts for weeks, whereas a small stream dominated by convective rainfall or tropical depressions may have irregular floods that only last for hours. Although precipitation driven over bank flow from the main and tributary channels is the primary driver of inundation on floodplains and parafluvial zones, inundation also results from direct precipitation, rising water tables, and overland flow from adjacent uplands (Mertes, 2011). Thus, inundation of floodplains may be directly related to their proximity to variably inundated hillslopes and streams.
The nature of floodplain/parafluvial inundation affects the dynamics of surface and subsurface water, solutes, particulate organic matter, sediment, and biota (Junk et al., 1989). Dynamics include the volume and duration of storage; the rate of movement; the direction of movement between the surface, hyporheic zone, and groundwater; and biogeochemical alterations that in turn impact river water quality, greenhouse gas emissions, plant function, and organismal ecology. The duration, frequency, and areal extent of floodplain/parafluvial inundation control ecosystem function and the types and abundances of organismal communities, including both aquatic and terrestrial species (Ward et al., 1999). Species distribution, movement, and biological interactions such as predator–prey are intricately tied to these inundation patterns (Robinson et al., 2002; Stanford et al., 2005). Fish species, for example, can migrate from dry-season refugia into floodplains during inundation, influencing food web structure and ecosystem productivity (Crook et al., 2020).
Among the primary challenges in answering questions regarding the variation in floodplain/parafluvial inundation are limited monitoring data and a lack of numerical models that integrate knowledge across disciplines and processes. Measurements and models of hydrology commonly treat floodplains as flat, impermeable surfaces, which ignores surface–subsurface water exchanges that influence hydrology and ecosystem function (Wohl, 2021). Models also often ignore the micro-heterogeneities that influence spatially and temporally variable patterns of inundation, biogeochemical cycling, and ecology in both floodplains and parafluvial zones. The degree of physical detail represented in models often involves tradeoffs in spatiotemporal extent; a one-dimensional model might ignore microtopography that influences important inundation details, whereas a more representative two-dimensional or three-dimensional model becomes computationally intensive for larger spatial extents. This problem gives rise to the challenges of and opportunities for (i) designing measurement campaigns across disciplines that can create integrative data for diverse floodplains and parafluvial zones to adequately represent the physical complexity of variable inundation processes at broad scales and (ii) developing floodplain/parafluvial functional groups (e.g., Fryirs and Brierley, 2022) that can facilitate understanding of scaling and transferability of data.
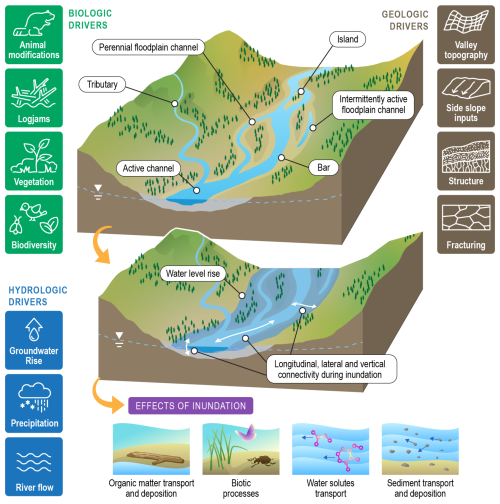
Figure 5Conceptual model of variable inundation in floodplain and parafluvial systems. Across floodplains and parafluvial zones a suite of biological, hydrologic, and geologic factors drive inundation regimes in terms of spatiotemporal duration, timing, depth, flow rate, etc. These systems include diverse subsystems, as summarized in the top panel. Rising water levels, due to one or more drivers, can inundate these subsystems, as shown in the middle section, resulting in a variety of biogeochemical, ecological, and physical effects (bottom sub-panels). Credit: Nathan Johnson.
2.4 Variably inundated wetlands
While not all wetlands are variably inundated, variable inundation is a common feature of many wetland ecosystems (Arias-Real et al., 2024). Here we focus primarily on wetlands that are similar to swamps, marshes, and bogs (Fig. 6). Wetlands cover about 10 % of the global land area, and nearly half of global wetland area (46 %) is temporarily inundated (Davidson et al., 2018). Generally, wetland inundation regimes are shaped by the wetland's connectivity to surface and subsurface hydrologic sources and landscape position (Åhlén et al., 2022). The landscape position of wetlands is a first-order indicator of the water source and chemistry, ranging from headwater depressional locally fed wetlands to flow-through and fringing wetlands to groundwater-fed low-lying wetlands (Fan and Miguez-Macho, 2011; Tiner, 2013). Wetland typologies applied in several national inventories generally rely on a combination of three criteria: soil type, hydrophytic vegetation and hydrology (Cowardin and Golet, 1995). Alternatively, hydrogeomorphic classification systems propose to exclusively draw on physical drivers, such as geomorphology, hydrology, and substrate, to allow for cross-site comparisons of biota and to serve functional assessments (Brinson, 1993; Davis et al., 2013; Semeniuk and Semeniuk, 1995, 2011).
While changes to inundation extent and depth can occur at timescales ranging from days to decades, the most conspicuous inundation patterns occur on event (e.g., following rain events), seasonal (e.g., snowmelt or wet–dry seasons), and interannual timescales. Primary drivers of inundation in unmanaged wetlands come from subsurface groundwater discharge and surface flows including rainfall or snowmelt runoff that occur when antecedent soil moisture conditions are high, preventing quick infiltration of water (Rasmussen et al., 2016). Many wetlands are actively managed, such as to provide bird habitat, so that inundation can vary based on management decisions (see below and Fredrickson and Taylor, 1982).
The spatial scales of variable inundation are shaped by both wetland size and geomorphology. Wetlands can be shallow over large spatial scales, and thus the size of variably inundated wetland area can range from microtopographic (i.e., hummock–hollow, ∼ m2 scales) to larger ecosystem scales. Large wetland areas, especially in the tropics, experience strong seasonal inundation cycles which depend on changes in water balance and local topography (Zhang et al., 2021). While the largest variably inundated wetlands are connected to floodplains, like the 130 000 km2 Pantanal located in Brazil and extending into Bolivia and Paraguay (Ivory et al., 2019), non-floodplain wetlands surrounded by upland (also known as geographically isolated wetlands) as large as ∼6 ha may also experience whole-system drying and rewetting (Lane and D'Amico, 2016).
Embedded within wetland ecosystems, microtopographic structures can create within-system mosaics of inundation regimes. Microtopography in peaty wetlands is particularly pronounced, ranging from several tens of meters (e.g., ridges and sloughs; Larsen et al., 2011) to meters (e.g., hummocks–hollows; Shi et al., 2015). These spatial patterns result from dynamic feedbacks between ecological processes (e.g., peat accumulation) and hydrology that reinforce these patterns (Belyea and Baird, 2006; Eppinga et al., 2008; Larsen et al., 2011).
Wetlands are widely acknowledged to be biogeochemical hotspots and ecosystem control points (Bernhardt et al., 2017; McClain et al., 2003) because of the confluence in space and time of allochthonous substrates moving into reactive environments (e.g., nitrate produced under oxic conditions entering anaerobic environments where denitrification can occur). In addition, variable inundation is associated with nutrient influx into wetlands that replenishes nutrient pools and can drive productivity and organic matter decomposition (Venterink et al., 2002). The depth and duration of inundation shape the wetland vegetation community by controlling germination success, modifying oxygen availability, and changing concentrations of toxins and nutrients, by desiccating aquatic plants or inundating terrestrial plants, and by changing the light availability (Casanova and Brock, 2000). Wetland vegetation is structurally adapted to low-oxygen environments; for example, some vegetation has developed air channels in leaves, stems, and roots to transport oxygen belowground (Tiner, 2016). Alternatively, wetland vegetation can also respond to shifts in oxygen levels physiologically on shorter timescales (Colmer, 2003).
Variable inundation provides an environmental filter for biota adapted to live under either dry or inundated conditions, resulting in distinct communities including wetland obligate and facultative species (Gleason and Rooney, 2018). The temporal duration of inundation (i.e., hydroperiod) indirectly controls the bird community composition through the absence and presence of wetland vegetation and availability of aquatic macroinvertebrate prey (Daniel and Rooney, 2021). Amphibian communities are particularly impacted by the hydroperiod: it needs to be long enough for eggs to hatch and tadpoles to reach metamorphosis but should not allow the establishment of many predator species (Resetarits, 1996).
Predicting how complex inundation patterns in wetlands will change under changing climate is a major research challenge. Predictions span the range from a decrease in inundation in some regions (Londe et al., 2022) to an increase in others (Watts et al., 2014), with uncertain consequences for wetland persistence overall. To improve regional or global predictions, accurate maps of wetland extent on different scales that can be incorporated into mechanistic models will be necessary (Melton et al., 2013). This is particularly challenging for non-permanent wetlands, which are hard to reliably map and are generally understudied (Calhoun et al., 2017; Gallant, 2015) but which are, by definition, VIEs. As climate change alters wetland inundation regimes, the net impacts on carbon storage and greenhouse gas fluxes are of particular concern (Moomaw et al., 2018) because together they will determine the net climatic impact of changes in wetland area and dynamics (Neubauer and Megonigal, 2015).
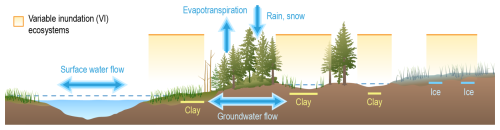
Figure 6Conceptual model of variable inundation in wetland systems. Different wetland types are influenced and shaped by variable inundation. The absence and presence of surface water is driven by (e.g., seasonally) changing water supply and the hydrologic function of the wetland in the landscape. Sediment characteristics (e.g., clay or ice) and topographic positions of wetlands in the landscape influence water loss to infiltration or gain from groundwater. Credit: Nathan Johnson.
2.5 Freshwater ponds
Freshwater ponds are among the most abundant and common freshwater ecosystems worldwide, with estimates of between 500 million and 3.2 billion ponds globally (Davidson et al., 2018; Hill et al., 2021). Ponds are generally small (less than 5 ha) and shallow (less than 5 m) and, consequently, are highly sensitive to changes in water levels that can result in highly variable inundation regimes (Gendreau et al., 2021; Richardson et al., 2022a). Pond ecosystems are extremely diverse and include arctic thermokarst ponds, prairie potholes, vernal pools, playas, rock pools, and agricultural dugouts. The numbers of ponds globally are likely underestimated, as their size and ephemeral/temporary nature have meant they are often excluded from physical inventories and mean they are below the resolution of many remote sensing techniques (Calhoun et al., 2017; Hayashi et al., 2016; Hill et al., 2021).
As in many other VIEs, inundation of freshwater ponds can be highly variable, and the timing, duration, and frequency of inundation can vary considerably (Williams, 2006). Many temporary or ephemeral ponds can become intermittently or seasonally inundated (Fig. 7). For some ponds, particularly vernal pools, seasonal inundation is relatively predictable, as these systems become inundated following snowmelt or spring runoff and are subsequently drawn down with increasing summer evapotranspiration (Brooks, 2004; Zedler, 2003). Variation in the hydroperiod can alter the composition of biotic communities (Brooks, 2004; Gleason and Rooney, 2018), as well as impact biogeochemical and hydrological processes (Bam et al., 2020; Hondula et al., 2021a). In more temperate regions, the timing of inundation is often driven by heavy rainfall, and periods of inundation can be highly variable, with inundation durations lasting from days to months and sometimes occurring intermittently as ephemeral systems dry and rewet multiple times a year (Florencio et al., 2020; Kneitel, 2014; Ripley and Simovich, 2009). For nearly permanent ponds, the pattern of wet and dry periods are more predictable, but the initiation and length of the hydroperiod can vary spatially as water levels fluctuate, inundating and exposing shallower areas (Brendonck et al., 2017). Freshwater ponds often demonstrate both high interannual and high intra-annual variability, and diurnal, annual, and multi-decadal periods of inundation can occur due to changes in evapotranspiration, drought, drainage, and/or the hydrologic function of the pond acting on the landscape (Brooks, 2004; Gendreau et al., 2021). Modifications to ponds by humans (e.g., irrigation ponds, urban stormwater ponds; see the “Human-engineered systems” section) or other organisms, such as beavers, can also impact the hydroperiod and inundation regimes (Brazier et al., 2021; Renwick et al., 2006).
Like many of the other ecosystems that experience variable inundation, freshwater ponds are also considered biodiversity and biogeochemical hotspots, providing many critical ecosystem services (Capps et al., 2014; Marton et al., 2015). Despite their relatively small size, ponds can have considerable variability in both community composition and biogeochemical processes, in part due to differences in inundation regimes, where pond margins are more likely than central regions to be more frequently desiccated for longer periods (Reverey et al., 2018). Models that explicitly incorporate remotely sensed variable inundation predict that ephemeral systems with shorter hydroperiods retain nitrogen at greater rates than larger systems with less variable inundation and longer hydroperiods, particularly in semi-arid regions like the Prairie Pothole Region of the North American northern Great Plains and playas in the south-central United States (Cheng et al., 2023). In addition, research suggests reproduction is largely impacted by inundation. Salamanders, for example, tend to lay more eggs during years with greater rainfall, while hatching success decreases with desiccation (Della Rocca et al., 2005). Variable inundation across ponds can result in ecosystem heterogeneity at the landscape scale, increasing local abiotic and biotic variation (Jeffries, 2008), but the number and distribution of inundated ponds can also impact regional biodiversity through processes like dispersal (Brendonck et al., 2017).
Climate change will likely alter the inundation regimes in freshwater ponds in terms of timing, frequency, duration, and extent. Decreases in precipitation and increases in extreme drought can result in shortened hydroperiods, and increasing temperatures can alter water temperatures and evaporation rates (Matthews, 2010). The persistence of freshwater ponds may, therefore, be reduced with climate change (Londe et al., 2022). Understanding how future changes in inundation regimes impact freshwater ponds will be critical. Similarly to wetland ecosystems, improved remote sensing methods, including incorporating multispectral imagery and radar along with finer-spatial-resolution mapping approaches, may improve the mapping, counting, and inclusion of small ponds in freshwater inventories (Bie et al., 2020; Hofmeister et al., 2022; Rosentreter et al., 2021). As inundation regimes become more variable, increasing conservation and protection efforts for maintaining ephemeral and temporary ponds will become more essential.
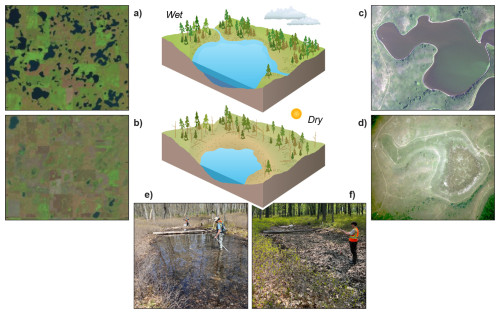
Figure 7Examples of variable inundation across scales in pond systems. Satellite imagery of the Prairie Pothole Region, North Dakota, USA, illustrating decadal variable inundation at a landscape scale on (a) 2 September 1992 and (b) 23 May 2013 (modified from Anon, 2015) and at the pond scale – aerial imagery of Pond P1, Cottonwood Lake Study Area, North Dakota, on (c) September 2002 and (d) September 1992 (images from the US Geological Survey, 2017). Seasonal changes in a vernal pond in Moshannon State Forest, Pennsylvania, USA, (e) inundated (11 May 2023) and (f) non-inundated (23 May 2023) (Jon N. Sweetman). Conceptual drawings by Nathan Johnson.
2.6 Storm-impacted coastal zones
The coastal zone includes ecosystems and communities (cities/towns) that are adjacent and hydrologically connected to a large waterbody (e.g., ocean, Great Lakes). These systems influence, are impacted by, and are dependent on coastal-zone hydrologic processes, such as inundation, that occur at the interface between terrestrial and aquatic domains. Unlike tidal environments, inundation that affects the coastal zone is driven by temporary, often stochastic, events including storms, seiches, and king tides. The impact and areal extent of coastal inundation vary across events, depending on topography, infrastructure, and event size (Fig. 8). The frequency of these events ranges from multiple times a season to decadal (Fig. 8). Tropical storms and cyclones develop in tropical regions during seasonal periods of warm water each year. Due to their high energy and movement, they influence more temperate regions as well (Colbert and Soden, 2012). In temperate or cold regions, storms develop in the wintertime due to large temperature differences between land and ocean (Liberato et al., 2013). Natural systems will display some form of resilience to and recovery from storm impacts (Lugo, 2008; Wang et al., 2016), but human settlements and infrastructure are vulnerable to both intense winds and inundation (Braswell et al., 2022; Hinkel et al., 2014; Lane et al., 2013). Land use development also alters the natural resilience of coastal environments through the proliferation of gray infrastructure such as jetties and seawalls (Gittman et al., 2015). Systems in low-lying regions as opposed to rocky shores with steep slopes are particularly vulnerable to inundation. While regional or global datasets based on elevation data exist, the extent at any given time of storm surges, king tides, and other high-water episodes depends locally/regionally on where the event hits, infrastructure, and the topography of the area.
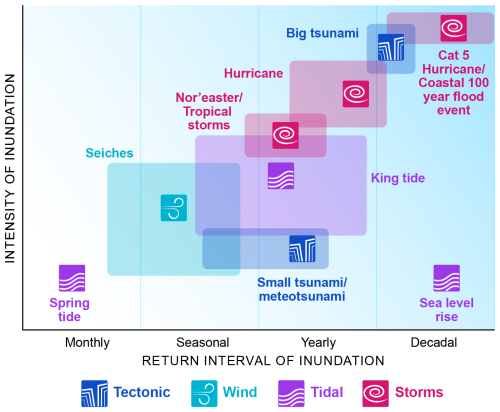
Figure 8Coastal VIEs experience inundation events with different frequencies and intensities. Some events occur rarely but are of very high intensity (category 5 hurricanes; large tsunamis), increasing the area of inundation and affecting areas that seldom experience inundation. The impacted systems are often less adapted to inundation, increasing the extent of destruction or reorganization of the system. Other events occur more regularly and/or are lower in intensity (spring tide, seiches), leading to less extensive inundation and impacting coastal systems that are more adapted to inundation. Credit: Nathan Johnson.
Inundation in the coastal zone impacts sediment transport, solute and nutrient mobilization, vegetation distribution, biological diversity, and biogeochemical processes. Erosion and sediment deposition alter ecosystem geomorphology (e.g., dune shape, marsh accretion) (Dissanayake et al., 2015; Houser and Hamilton, 2009) and ecosystem nutrient pools (e.g., Castañeda-Moya et al., 2020; O'Mara et al., 2019). In coastal zones adjacent to marine and estuarine waters, saltwater intrusion changes surface (Schaffer-Smith et al., 2020) and groundwater (Cantelon et al., 2022) quality and mobilizes nutrients through porewater ionic exchange processes (Herbert et al., 2018). Coastal-zone inundation as a natural process alters dune systems, which generates a mosaic of habitats that increase biodiversity (Smith et al., 2021) and alter distributions of vegetation and animals. For example, the frequency of overwash events affects plant composition and diversity on sand dunes (Stallins and Parker, 2003) and regular inundation is thought to provide necessary habitats for some insects and birds (Smith et al., 2021). Increased salinity and associated geochemical changes alter microbial community diversity and population heterogeneity (Nelson et al., 2015), shifting to more specialized communities as an adaptation to anaerobic conditions, redox fluctuation, and salt stress. Previous studies have found high variability in relationships between salinity and ecosystem carbon dioxide fluxes (Dang et al., 2019; van Dijk et al., 2015; Hopple et al., 2022; Morrissey and Franklin, 2015).
Human communities within the coastal zone are impacted by inundation events as well. Inundation of coastal agricultural lands from storm surge and sea level rise reduces agricultural productivity (Lei et al., 2016). In particular, risk is high for coastal-zone communities in developing nations, where inundation events can lead to food insecurity, loss of livelihood, and increased transmission of waterborne diseases. As climate change alters the magnitude and frequency of inundation in the coastal zone, it will be necessary to integrate both natural and human adaptations, such as enabling salt marsh transgression (marsh migration upland) to mitigate storm surge impacts on crop yield (Guimond and Michael, 2021).
While we understand many of the linkages between the ecology, biogeochemistry, hydrology, and geomorphology that regulate ecosystem structure and function in coastal systems (Braswell and Heffernan, 2019; Cantelon et al., 2022; Fagherazzi et al., 2012; Hinshaw et al., 2017), we know little about how to predict the future effects of the interacting stressors associated with climate change (Arrigo et al., 2020; O'Meara et al., 2017; Ward et al., 2020). Our ability to predict is reliant on our understanding of shifting inundation regimes in the context of elevated CO2, nutrient pollution, and coastal development, which can generate antagonistic, synergistic, or additive effects. These knowledge gaps stem from the dynamic and unpredictable nature of events that drive coastal inundation. Observational data to inform mechanistic models are limited and governed by where and when events happen (not necessarily within monitored sites), funding periods, and accessible coastlines. This difficulty is exacerbated by the fact that 40 % of the world's population lives within 100 km of the coast (Maul and Duedall, 2019), which heightens social impacts of variable inundation while also adding logistical difficulty to coastal monitoring. When events do overlap with instrumented sites, the extreme nature of inundation events threatens the physical integrity of instrumentation. Lastly, high-latitude coastlines are also susceptible to coastal inundation, yet few models incorporate the physical, biogeochemical, and ecological implications of inundation on permafrost-bound coastlines and environments (Bevacqua et al., 2020; Ekici et al., 2019). Opportunities for critical knowledge advancement exist in (1) monitoring events through in situ or remotely sensed monitoring data, (2) model development that integrates more robust process-based understanding, and (3) expansion into urban and permafrost-bound coastlines.
2.7 Tidally driven coastal zones
Tidally influenced coastal zones exist at the intersection of terrestrial and marine environments and encompass diverse intertidal ecosystems such as marshes, mangroves, ghost forests, and beaches (Fig. 9). Globally, tidal wetlands exist on six of the seven continents and are spread across tropical, temperate, and polar latitudes (Murray et al., 2022a). Tidal flats are predominantly found along low-sloping coastlines, with approximately 70 % of global tidal flat area existing in Asia, North America, and South America (Murray et al., 2022b), while beaches encompass 31 % of ice-free shorelines (Luijendijk et al., 2018).
Tidally driven coastal zones are inundated semi-diurnally (i.e., twice a day) or diurnally (i.e., once a day). Unlike VIE systems summarized above, where inundation events may be difficult to predict, inundation in tidally driven coastal zones varies primarily based on predictable drivers. For example, high-tide and low-tide water levels dictate the spatial extent and duration of inundation. In addition, intra-annual tidal dynamics are largely controlled by lunar cycles which drive, approximately monthly, the highest (spring) and lowest (neap) tides, as well as annual high (king) and low tides. Interannual tidal dynamics are linked to sea level rise, which is shifting the zone of variable inundation inland (Ensign and Noe, 2018; Tagestad et al., 2021). We note that while the timing of king tides is predictable (perigean spring tide), their impacts can be difficult to predict, as mentioned in the “Storm-impacted coastal zones” section. In addition, topography (e.g., slope) and other natural physical factors, including wind speed and direction, waves, and even localized high- and low-pressure events, mediate the lateral extent of surface water inundation in tidal ecosystems. Human modifications further alter both the vertical and the longitudinal extent of tidal inundation via control structures which may exclude tides (gates, weirs, etc.) and channels that transport tidal waters well inland of the natural intertidal zone.
The extent of tidal influence, which spans microtidal (<2 m tidal range) to macrotidal scales (>10 m tidal range in some locations), controls water quality, terrestrial–aquatic interactions, and resulting biogeochemical and ecological responses (e.g., Tweedley, 2016). Estuaries, where tides mix saltwater and freshwater, are dynamic biogeochemical mixing zones characterized by sharp chemical gradients that regulate biological activity (e.g., Crump et al., 2017). Shifts in tidal zones associated with sea level rise are predicted to alter the extent of key intertidal habitats, with potential disruptions to coastal food webs (Rullens et al., 2022). Changes in the duration and extent of inundation associated with tides control soil saturation and salinity, which influence redox dynamics and the hydrologically driven exchange of carbon, nutrients, and pollutants (Bogard et al., 2020; Pezeshki and DeLaune, 2012; Regier et al., 2021). Biological activity, including crab burrows that alter hydrologic flow paths (Crotty et al., 2020), also influences tidal exchanges across the coastal terrestrial–aquatic interface (Crotty et al., 2020). Increased saltwater exposure due to shifting tidal ranges can alter the stability of coastal soils (e.g., Chambers et al., 2019), which represent a globally important carbon sink (Mcleod et al., 2011). In addition, tidal regimes structure vegetation gradients, where salt-sensitive communities including low-lying forests and freshwater marsh species are replaced by salt-tolerant communities including mangroves and salt marsh species (Kirwan and Gedan, 2019; Lovelock and Reef, 2020). This shift in tidal range leads to the creation of ghost forests (Kirwan and Gedan, 2019), which can impact coastal biogeochemical cycles (e.g., Cawley et al., 2014). Similarly, sea level rise may lead to mangrove or marsh retreat as inundation patterns change (Xie et al., 2020).
Due to the frequency of inundation, tidally inundated ecosystems are hydrologically, biogeochemically, and geomorphologically dynamic, creating challenges for scientists and land managers seeking accurate estimations of land surface area, elevation, and carbon storage. These challenges are exacerbated by sea level rise, which exerts heterogeneous and nonlinear influences on tidal ranges (Du et al., 2018). Methodological approaches to assess tidal ecosystem area and elevation that are based on satellite imagery will be critical for present and future management and decision-making. Similarly, complex feedbacks exist among hydrology, biogeochemistry, ecology, and geomorphology (Xin et al., 2022); these dynamics may need to be considered in future ecosystem projections. Thus, a deeper understanding of feedbacks and their variability in space and time in response to tidal activity is needed (Ward et al., 2020). Lastly, with sea level rise, tidal constituents may change, with nonlinear impacts on tidal range and inundation extent (Pickering et al., 2017). Tidally inundated VIEs represent the interface between marine and terrestrial ecosystems, and to predict their future will require understanding bi-directional connections among physical, chemical, and biological system components.
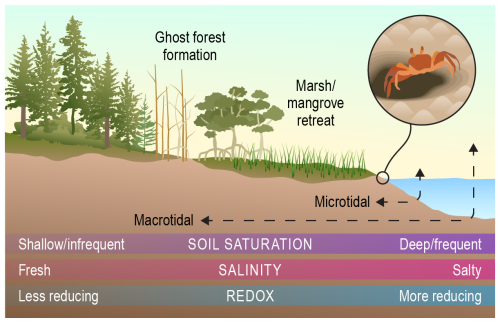
Figure 9Conceptual model of variable inundation in tidal systems. Tidally driven coastal zones span sediments exposed at low tide to marshes and coastal forests inundated at high tide. This lateral gradient of tidal exposure across micro- to macrotidal systems (dashed black lines) alters physical (e.g., particle deposition), biological (e.g., species composition), and chemical (e.g., nutrient transformations) factors. Organisms can impact conditions along the gradient, such as flow path alteration by crab burrowing. Credit: Nathan Johnson.
2.8 Human-engineered systems
Human-engineered systems are environments where inundation magnitude, frequency, timing, and duration either are actively managed or have been dramatically altered by structural modifications to the landscape (Fig. 10). Human-engineered VIEs rival natural systems in area and extent (Clifford and Heffernan, 2018), yet the significance of engineered VIEs in influencing landscape processes is relatively unexplored compared to natural systems (Koschorreck et al., 2020) and they have been historically excluded from water and nutrient budgets (Abbott et al., 2019). The primary drivers of human-engineered VIE formation explored here are land use change and restoration (including those for nature-based solutions), though hydrologic modifications impact inundation regimes of the natural VIEs explored earlier in the paper. Examples of land-use-driven human-engineered VIEs include, but are not limited to, croplands irrigated to the point of inundation (e.g., rice paddies, cranberry bogs); canals for irrigation, drainage, and stormwater (e.g., roadside ditches, retention ponds); and unintentional VIE formation following landscape modification, e.g., “accidental” urban wetlands (Palta et al., 2017) and ponds in agricultural fields (Saadat et al., 2020). Whereas the purpose of land-use-driven engineered VIEs is to redistribute water for human purposes, the goal of VIEs engineered for restoration is to either replace or enhance ecosystems lost or damaged as a result of human activity. VIE restoration efforts vary in scope and form, spanning local (e.g., residential living shorelines, individual stream reaches, agricultural ditch wetlands) to ecosystem (e.g., adding sediment to degrading marshes) to regional (e.g., dam removal) scales (Baptist et al., 2021; VanZomeren et al., 2018).
While the full extent of human-engineered VIEs is difficult to quantify, key examples highlight their significance in the landscape. Agriculture covers nearly 40 % of Earth's land surface (Siebert et al., 2010), and nearly a quarter of that is variably inundated by flood irrigation (Wu et al., 2023). In urban systems, the extent of stormwater control networks rivals the extents of natural systems. For example, the total linear length of residential canals in North America nearly equals that of the Mississippi River (Waltham and Connolly, 2011). While restoration efforts are not as widely distributed as land use change, restoration still contributes to extensive VIE creation. For example, restoration accounts for 14 % of areal gain of tidal wetlands globally (Murray et al., 2022b). Inundation regimes in human-engineered VIEs can be driven by natural hydrologic processes, such as connectivity with the water table or tidal inputs. This is particularly important in VIEs built for restoration, as establishing natural inundation regimes enhances landscape connectivity and mediates ecosystem functions (Jones et al., 2018; Reis et al., 2017). However, unlike the previously discussed natural systems, the drivers and duration of inundation in human-engineered VIEs may be decoupled from natural hydrologic processes. Controlling drainage, such as for stormwater management, land reclamation, or effluent releases, is a key motivation for VIE construction and system design, resulting in inundation periods largely driven by precipitation that persist at event to seasonal scales depending on local hydrology and climate. Inundation duration may also occur on longer timescales, such as seasonally in paddy systems (De Vries et al., 2010). Finally, direct human interventions, such as floodgates, weirs, and dams, may affect water residence time at timescales that are asynchronous with natural drivers, such as seasonality or tides.
Human-engineered VIEs fundamentally alter the landscape, changing the spatial and temporal patterns of ecosystem processes. Agricultural inundation, such as flood irrigation or ponding, alters redox conditions, greenhouse gas emissions, groundwater recharge, evapotranspiration fluxes, plant growth, and pollutant export to natural waterbodies (Buszka and Reeves, 2021; Hale et al., 2015; Pan et al., 2017; Pool et al., 2021). For example, a recent study showed that variably inundated depressions in agricultural fields can account for ∼30 % of nitrous oxide emissions across cultivated areas despite comprising ∼1 % of the land surface (Elberling et al., 2023). The creation of drainage canals increases waterborne carbon fluxes from VIEs by producing a newly decomposed stock of labile soil carbon to be leached as well as by increasing the hydrological runoff rate through the soil and receiving canals and ditches (Stanley et al., 2012). Human-engineered VIEs can also provide ecosystem services that supplement or replace those of natural VIEs in the landscape (Clifford and Heffernan, 2018). For example, they can enhance habitat (Connolly, 2003; Herzon and Helenius, 2008), nitrogen removal (Bettez and Groffman, 2012; Reisinger et al., 2016), and recreation (Beckingham et al., 2019). Further, the services these systems provide can be improved through targeted management (e.g., vegetation composition; Castaldelli et al., 2015) or restoration practices (i.e., two-stage ditches; Speir et al., 2020).
Including human-engineered systems in our conceptualization of VIEs emphasizes the growing significance of these systems as human landscape modifications continue to alter and eliminate natural VIEs. Recent efforts have synthesized the role and impacts of human-engineered VIEs at large scales (Li et al., 2022b; Peacock et al., 2021), but, as with many natural systems, the majority of studies on human-engineered VIEs are based in North America and Europe (Bertolini and da Mosto, 2021; González et al., 2015; Zhang et al., 2018). Thus, our knowledge may not reflect the social, political, and economic challenges of developing areas where the highest rates of VIE modification are occurring (Wantzen et al., 2019). The knowledge gaps surrounding human-engineered VIEs will become increasingly important to address as global change continues to alter the spatial and temporal patterns of inundation. Given that human-engineered VIEs can enhance or disrupt hydrologic connectivity, they potentially magnify the effects of human-driven changes such as sea level rise and impacts of contamination from anthropogenic “chemical cocktails” (Kaushal et al., 2022). We lack a baseline standard for how human-engineered VIEs function in the landscape, even as global change continues to shift existing baselines (e.g., Palmer et al., 2014). A baseline understanding would also enable the restoration and repurposing of engineered VIEs as nature-based solutions (Clifford and Heffernan, 2023). Addressing these knowledge gaps will require the incorporation of human-engineered VIEs into large-scale syntheses and modeling efforts, particularly those that address hydrologic and biogeochemical fluxes. Conclusive definitions and inventories of human-engineered VIEs are essential for estimating the ecological and biogeochemical roles of VIEs at the global scale. Finally, human-engineered VIEs need to be conceptualized within an ecological, rather than managerial, context for comparison with natural systems and to be integrated into a more continuum-based approach for VIE science. Human-engineered VIEs rival the range of natural VIEs in structure, inundation regime, and global distribution. Understanding their role in the Earth system is, therefore, critical for understanding both the impacts of and the potential solutions to global change.
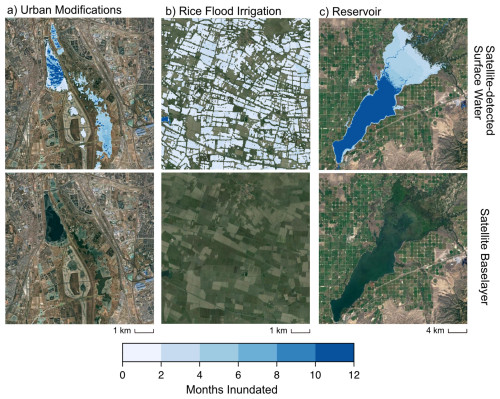
Figure 10Examples of human-engineered variably inundated ecosystems: (a) Yongding River in Beijing, China; (b) paddy rice fields in northern Italy; (c) American Falls Reservoir on the Snake River in Idaho, United States. These three examples emphasize significant variation in the degree of variable inundation across human-engineered VIEs, with some regions being perennially inundated. Top row: satellite-derived map data on months inundated are derived from the “seasonality” product in the Global Surface Water Mapping Layers v1.4 (Pekel et al., 2016). Credit: Jillian Deines.
VIEs span broad spatiotemporal scales of variable inundation, from small wetlands and vernal ponds to the floodplains of the world's largest rivers. While examples in the mini-reviews focus on eight different ecosystems, variably inundated ecosystems are even broader, including for example mosses and pore spaces that are periodically covered by droplets of water and vast endorheic lakes and rivers. Inundation volumes and surface areas of VIEs vary by at least 16 orders of magnitude, from under 10−3 to over 1013 L (Bonython and Mason, 1953) and from 10−6 to over 1010 m2 (Hess et al., 2015), respectively. The duration of inundation varies by up to 8 orders of magnitude, spanning a few seconds, in the case of droplets, to decades, in the case of endorheic lakes, and centuries, in the case of sea level rise. Non-inundated periods likewise span seconds to centuries and longer. This variability in spatial and temporal extent has profound consequences for the ecology and biogeochemistry of VIEs. This section highlights the importance of considering scale and explores hypotheses regarding how scale drives variability in drivers, processes, and impacts across VIEs and how we study them.
Spatial and temporal scales of VIEs can be categorized along two axes – extent and granularity. Extent comprises the total size of the spatial domain or time duration of a defined system, while granularity pertains to the spatial or temporal intervals of system transitions (Ladau and Eloe-Fadrosh, 2019). For example, the dynamics of water droplets across North America would represent a large extent with fine granularity, relative to the inundation dynamics of a desert playa of several square meters (smaller extent but coarser grain). The impacts of variable inundation are dependent “on the scale of the beholder” relative to the extent and grain of variable inundation, where a “beholder” may be a molecule, organism, population, community, land manager, or something/someone else (Fig. 11). The expressed metabolism of an individual microbe will be influenced by inundation down to the spatial scale of water films and on hourly or shorter timescales. An individual microbe may not, however, be influenced by whether variable inundation occurs only within a square meter or across many square kilometers because it does not perceive these larger scales. In contrast, macroinvertebrate behavior is influenced by variable inundation down to scales of meters and days and is likely further influenced by larger and longer scales of stream network connectivity (Bogan et al., 2017b; Sarremejane et al., 2017).
VIEs can be viewed as habitat patches of different sizes that vary in how long they persist in a given state and that have dynamic connectivity among patches. Terrestrial and aquatic biota respond on ecological and evolutionary timescales to the expansion and contraction cycles of inundation (Bornette et al., 1998; Ward et al., 2002). Biotic diversity is influenced by productivity, connectivity, disturbance severity, and disturbance frequency, all of which operate at hierarchical scales (Ward et al., 1999). Biogeographical and ecological theories posit that patch size (e.g., species area scaling) and disturbance regimes (e.g., intermediate disturbance hypothesis) are strong determinants of community composition (Adler et al., 2005; Svensson et al., 2012), suggesting that VIE community composition may vary predictably with these factors. The duration, predictability, and frequency of inundation likely have consistent community-level consequences that vary predictably with VIE extent and grain. Different extents and grains of inundation have the potential to change habitat connectivity in addition to directly selecting for different groups of organisms. Isolated marshes may, for example, become merged during a flood, thereby enhancing dispersal of aquatic organisms. The scale of variable inundation has numerous influences over ecological processes and dynamics that need to be understood.
From a biogeochemical perspective, variable inundation generates spatial and temporal variation in rates and patterns of biogeochemical processes. This variability is important for scaling biogeochemical rates because of process nonlinearity and Jensen's inequality (Ruel and Ayres, 1999). That is, a rate based on average conditions differs systematically from the average rate across variable conditions. This is important because the scales of processes (e.g., microbial activity occurring within pore channels) are typically not aligned with the scales of measurements and models (e.g., core scale or above). The lack of clear understanding of how variable inundation influences variation in biogeochemical processes and how these relationships change with the extent and grain of inundation can, therefore, lead to unreliable predictions for the scaling of biogeochemical processes.
Understanding the biogeochemical influences of variable inundation across a broad range of scales is important for informing a diverse suite of needs across models, decision-makers, and other interested parties. Our ability to inform these needs depends on our ability to rigorously understand and predict influences of variable inundation across scales. This is a challenge as variable inundation likely has direct, but unknown, influences over the scaling of biogeochemical function. For example, cumulative metabolism in streams is predicted to increase faster than their upstream drainage areas for perennial stream networks (Wollheim et al., 2022). The influence of variable inundation on biogeochemical processes cannot yet be accounted for in such scaling theory. More generally, perturbations like variable inundation can drive systems away from steady-state assumptions from which scaling relationships are derived (McCarthy et al., 2019); therefore, we expect significant changes in scaling behavior across inundation regimes. A research frontier is to quantify the direction, magnitude, and duration of changes in scaling patterns in response to variable inundation and to modified variable inundation regimes wrought by climate, land use, and other environmental changes.
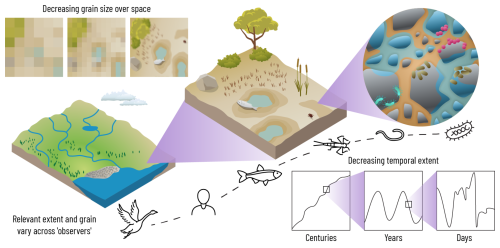
Figure 11Variable inundation can be observed at different spatiotemporal granularities and extents. Upper left: granularity is based on the resolution of observations in space or time. Lower right: extent is based on the cumulative breadth of observations in space or time. Middle illustrations: granularity and extent of observations are often correlated, such as barely resolving individual trees when extent spans a watershed and resolving individual microbes when extent spans a few soil particles. A given beholder observes variable inundation at a given scale and will, in turn, make changes to behavior, physiology, and/or aspects of life history. For example, migratory waterfowl select habitats based on the inundation state as they move across watersheds, humans plan cities based on regional patterns, fish move across stream reaches based on the continuity of inundation, macroinvertebrates lay eggs on individual rocks based on the inundation state, nematodes experience variable inundation as they move through porous media, and soil microbes separated by micrometers likely experience vastly different inundation dynamics linked to water films on soil particles. Credit: Nathan Johnson.
The multi-scale nature of VIE systems has led to experimental and observational studies that span point-scale lab-based characterization to reach- or watershed-scale monitoring networks and to regional- and global-scale remote sensing. Point-scale measurements at the smallest scales help reveal processes that underlie larger-scale dynamics. For example, point measurements of water presence, water absence, and low-flow detection within a watershed are increasingly available with the development of small, inexpensive, and easily deployable sensors, meters, and time-lapse cameras (e.g., Chapin et al., 2014; Costigan et al., 2017; Soupir et al., 2009; Zimmer et al., 2020) (Fig. 12). While these measurements are easy to take and can provide a long temporal dataset for little effort, they are not always detailed and require regular calibrations.
A broad range of methods can be used to link the hydrologic dynamics to ecological and biogeochemical responses. Standardized field surveys and biomolecular methods (e.g., isotopic ratios, including compound specific analyses) are commonly used to study organismal, population, and community ecology across multiple taxa (e.g., Gates et al., 2020; Ode et al., 2016) and can be standardized for both inundated and non-inundated states. There is increasing use of crowdsourcing for biogeochemical characterization to consistently obtain samples across diverse systems (Garayburu-Caruso et al., 2020; von Schiller et al., 2019). Sample collection can be followed by a variety of laboratory measurements of properties (e.g., carbon content, redox potential and redox-active elements, microbial genetic potential, sediment grain size) and processes, such as CO2 production and methanogenesis related to variable inundation. Point-scale measurements often operate at instantaneous to daily scales. Conversely, larger-scale measurements are integrated across finer-scale processes to quantify ecosystem dynamics and properties but without necessarily revealing what governs those processes. Spatially distributed monitoring networks using in situ sensors (e.g., the United States Geological Survey, USGS, gage network) can connect event-scale responses across hydrologically linked locations as well as revealing long-term trends (e.g., Zipper et al., 2021). Long-term field manipulations are another complementary in situ technique that can reveal mechanisms underlying system responses to changes in inundation state. There are numerous configurations of such experiments that directly or indirectly impact inundation dynamics, such as intentional inundation (Hopple et al., 2023), water exclusion (Kundel et al., 2018), and heating (Hanson et al., 2017). Despite the plethora of data produced by such large-scale projects, these are expensive and require deep buy-in of researchers and landowners.
Remote sensing can complement in situ measurements to facilitate more spatially continuous characterization of surface water dynamics and their impacts. There are different types of remote sensing techniques, from drones to satellites and optical to microwave sensors, that can capture different aspects of VIEs. For example, soil surface saturation may be captured by a passive microwave radiometer as well as C- and L-band radar backscatter, which can also penetrate through thin canopies, clouds, and the top few centimeters of the soil (Schumann and Moller, 2015). Recent satellite missions such as the Surface Water and Ocean Topography (SWOT) mission provide increased capabilities for monitoring changes in surface water over time with radar data (Biancamaria et al., 2016), while NASA's forthcoming NISAR mission will allow for the detection of inundation even under tree canopy. Thermal infrared measurements can indirectly reveal saturation at very high spatiotemporal resolutions, as well as evapotranspiration associated with water table depth, soil moisture, and rooting depth (Fisher et al., 2020; Lalli et al., 2022). Long time series from moderate-resolution (∼30 m) optical satellites can document multi-decadal open-water trends and seasonal regimes across the globe (Pekel et al., 2016), while some combinations of indices have shown success in detecting mixed vegetation and inundation cover (Jones, 2019). Commercial satellite constellations provide daily global imagery at <4 m resolution, enabling monitoring of more dynamic waterbodies (e.g., Arctic lakes, Cooley et al., 2017, and forested wetlands, Hondula et al., 2021b). Deep groundwater and changes in the total water column storage are detectable through measurements of gravitational anomalies at very high precision levels but low spatial resolution (Bloom et al., 2010, 2017; Pascolini-Campbell et al., 2021; Richey et al., 2015). Fine-scale inundation dynamics, which have been historically hard to measure, can be captured using unoccupied aerial vehicles (UAVs), which are often useful during or immediately after a significant inundation event (Perks et al., 2016) for capturing small-scale spatial dynamics that are difficult to detect with satellite or airborne methods (Dugdale et al., 2022; Manfreda et al., 2018) or for deriving detailed data for input into hydrologic models and surface water calculations (Acharya et al., 2021).
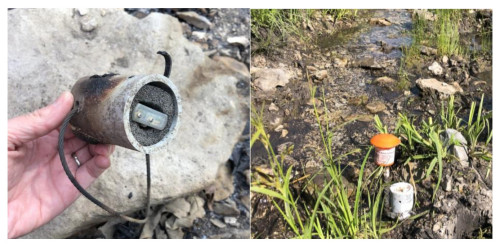
Figure 12Monitoring inundation regimes is increasingly possible via in situ sensors. Pictured are stream temperature, intermittency, and conductivity sensors (STICs) (Chapin et al., 2014), one of the types of increasingly available sensors to measure water presence/absence in an inexpensive and easily deployable manner. These sensors can be used across all types of VIEs. Credit: Amy Burgin.
To advance predictive understanding requires the integration of data with models. Process-based models can be used to simulate hydrological and biogeochemical processes under dry and wet conditions (Fatichi et al., 2016; Li et al., 2017). These models are often built upon mass conservation principles, with ordinary or partial differential equations that describe coupled hydrological, ecological, and biogeochemical processes. They rely on existing knowledge of processes, including, for example, theories or empirical relationships between discharge and water storage (Wittenberg, 1999), biogeochemical reaction rate dependence on temperature and water content (Davidson et al., 1998; Mahecha et al., 2010), and redox reactions (Borch et al., 2010). Among process-based models, there are spatially distributed models that couple surface and subsurface flow dynamics explicitly (Coon et al., 2020; Kollet and Maxwell, 2006). This class of models has recently been extended to include reactive transport (Wu et al., 2021), which may be considered a set of tools to understand the biogeochemical effects of variable inundation (Molins et al., 2022). However, spatial resolution and data requirements for the integrated surface and subsurface models are high, which places practical limits on the spatial scales that can be addressed. Semi-distributed or fully distributed models with coarse spatial resolution are able to work at larger scales but require theories or empirical relationships to represent processes and impacts at subgrid scales. Data-driven machine learning methods present new opportunities to blend models with various levels of mechanistic representations into hybrid models (Reichstein et al., 2019). Increases in the volume of observational datasets combined with advances in high-performance computing have triggered a shift towards machine learning applications for capturing inundation dynamics. More recently, integration of physics-based models with machine learning has improved the interpretability of machine learning methods and increased their ability to model complex ecosystem processes (Sun et al., 2022b). These hybrid approaches have the potential to optimize the characterization and prediction of inundation dynamics by incorporating the strengths of multiple models to achieve predictions with minimized uncertainty and greater accuracy than either model alone.
Coordinated integration (Patel et al., 2024) between model development and data generation is key to deepening our understanding of VIEs and increasing our ability to predict their future ecosystem function and ecological integrity. More specifically, we promote iterating between model-guided data generation and observation-informed model development. This iterative cycle between models and “experiments” (i.e., real-world data generation) has previously been termed “ModEx” (Atchley et al., 2015) and is similar to approaches used in “ecological forecasting” (Dietze et al., 2017, 2018). It also aligns generally with the scientific method based on continuous iteration between conjectures (hypotheses/models) and refutation (falsification of hypothesis using observations and data) to drive scientific discovery and knowledge growth (Popper, 2014). The ModEx approach often starts with using experimental or field data to parameterize and calibrate models and/or generate new data based on known model input needs. This can be expanded, whereby models generate hypotheses via in silico experiments, and field or lab studies can be designed to test those hypotheses. Models can also be used to optimize the design of real-world experiments by indicating when, where, and what to measure to provide the strongest hypothesis evaluation.
In the context of VIEs, we expect ModEx to touch scales ranging from molecular microbiology to landscape ecology to regional ecosystem function to Earth system elemental cycles. As a landscape-scale example of ModEx, physical models could first be used to predict variable inundation across a watershed. Spatial and/or temporal uncertainty in those predictions could then be used to optimize the collection of commercial remote sensing data. Those data would, in turn, be used to evaluate model predictions, leading to updated guidance from the model on where/when to collect additional remote sensing data. Further cycles could be pursued, and model uncertainties could also guide the collection of in situ data on variable inundation, organismal ecology, and/or biogeochemical processes. Many other examples across a variety of scales can be envisioned, and key to enabling this approach is the further development of models and measurement techniques that can capture system states in both inundated and non-inundated conditions. Techniques/models designed for specific kinds of ecosystems (e.g., perennial rivers) may be difficult to adapt. This emphasizes a need to apply ModEx using models and measurements intentionally designed to span inundated and non-inundated system states.
Across the continuum of ModEx, it is important to consider the scales at which models and measurements operate, as discussed above. The issues around scale could, in part, be addressed by “integrated, coordinated, open, networked” (ICON) science principles (Goldman et al., 2022). ICON is based on the intentional design of research efforts to be integrated across disciplines and scales, coordinated across research efforts via consistent methods, open throughout the research life cycle, and networked across stakeholders to understand collective needs. We propose using ICON principles for in situ data generation and remote sensing, jointly guided by model-generated predictions (i.e., ModEx). Embedding ICON throughout the research life cycle can help to ensure that new data are at the right scale and can be used to link disciplines (e.g., hydrology, biogeochemistry, and community ecology). This can also ensure that data are interoperable across VIEs, are available to everyone and connected to deep metadata, and are useful to a broad range of stakeholders with interests spanning different types and locations of VIEs. The use of ICON in cross-VIE science could bridge existing data across multiple spatial and temporal scales and could potentially bridge gaps among VIEs.
We propose that a key goal for VIE science is the development and open sharing of knowledge, models, algorithms, and data that transcend individual system types. Knowledge that crosses VIE systems will inherently span scales and levels of certainty from predictable sub-daily inundation regimes to rare extreme events; integrating perspectives of these dynamic systems can aid in understanding and anticipating tipping points of physical, chemical, and biological components across VIEs. Development of such knowledge should be done via ModEx approaches coupled with ICON principles, which can generate models that can be used across VIEs. Similarly to the perspectives of Arias-Real et al. (2024), we suggest this can be facilitated through the development of conceptual models based on continuous environmental axes that modulate system responses to re-inundation (e.g., greenhouse gas production and changes in biological diversity).
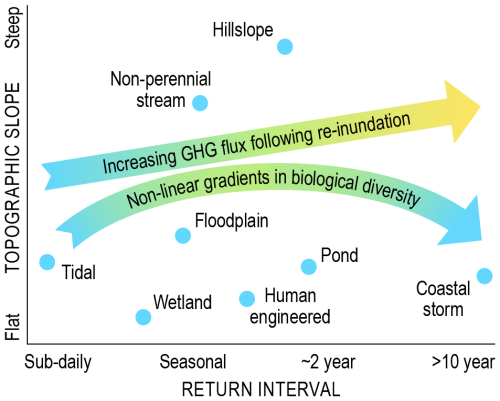
Figure 13We encourage unifying conceptual models of VIEs based on hypotheses linked to continuous environmental axes, across which these systems can be studied without regard for what system names may be attached to a given studied place and time. In our proposed conceptual model, two key axes are the topographic slope and inundation return interval. Points represent approximate locations of where each VIE type may lie. Each VIE type spans a range of slopes and inundation return intervals, but we do not define these ranges as the conceptual model is based on how study systems fall across the environmental space represented here, rather than within specific nomenclature. Two priority research directions are greenhouse gas (GHG) fluxes and biological diversity, and the arrows represent possible hypotheses that could be evaluated with cross-VIE studies. We propose that knowledge and models that are transferable across VIEs can be achieved through evaluation of such hypotheses across broad ranges in the slope and return interval. Credit: Nathan Johnson.
Such continuum-based conceptual models necessitate going beyond discrete VIE categories by treating key physical characteristics as continuous variables that influence all VIE systems. One realization of such a conceptual model is summarized in Fig. 13. Related approaches that are based on a suite of temporally variable ecological and geomorphological characteristics have proven useful for wetlands (Euliss et al., 2004; Lisenby et al., 2019). These wetland frameworks have improved the understanding of human impacts on wetlands and led to more effective management (Mandishona and Knight, 2022; Wierzbicki et al., 2020). These successes emphasize the potential effectiveness of continuum-based conceptual models for cross-VIE science.
The impacts of variable inundation depend on multiple characteristics of inundation regimes (e.g., return interval and duration) and factors that influence those regimes (e.g., subsurface permeability, topography, climate, and vegetation) (Banach et al., 2009; De Jager et al., 2012). Furthermore, there are dynamic attributes such as water residence time and hydrologic connectivity that influence process rates (Covino, 2017). We hypothesize that despite this complexity, cross-VIE science can make progress towards transferable understanding through the evaluation of conceptual models that focus on impacts of variable inundation across relatively simple physical variables that can be easily measured. Two such variables are the inundation return interval and topographic slope (Fig. 13). As suggested above, we encourage studies that examine responses to variable inundation (e.g., biogeochemical rates and ecological community composition) across VIEs that collectively span a broad range of return intervals and slopes.
While many environmental variables could be used in this conceptual model (Fig. 13), here we propose using the inundation return interval and topographic slope, as both are well known to impact ecological communities. For example, the inundation return interval has been shown to alter plant composition (Arim et al., 2023) and biogeochemical function such as CH4 fluxes (Batson et al., 2015). We view it as an integrated proxy for variables with direct impacts (e.g., desiccation) that are linked to the temporal scale of non-inundated conditions. The other axis of our conceptual model is the topographic slope (Fig. 13), which we also view as an integrated proxy but for variables linked to how much time water spends in a system (Anderson and Burt, 1978; McGuire et al., 2005). The slope and the variables it represents (e.g., water residence time and velocity) are also well known for influencing ecological communities (e.g., by altering fish composition, as in Bain et al., 1988) and biogeochemistry (e.g., by altering nitrate reductions, as in Gomez et al., 2012).
At a high level, the return interval and slope are two key dimensions of temporal scale: how long it takes water to return and how long a parcel of water spends in the system. Similarly, these variables encompass differences across spatial scales, capturing differences in the timing of inundation and how water flows through and is connected to different components of VIEs (e.g., differences in drying across branches of a river network). While these two components should jointly influence nearly every physical, chemical, and biological aspect of VIEs through time and across space, we do not imply that these two variables will capture all relevant processes. Other variables such as sediment/soil mineralogy and climate also have strong influences over the biogeochemistry and community ecology of VIEs (e.g., Pumo et al., 2016). We may learn that additional axes are needed and these may be linked to other conceptual models, such as whether inundation emerges through infiltration excess (Hortonian flow generation) or through saturation excess (Dunnian flow generation) (Freeze, 1974). Nonetheless, we propose that significant progress can be made towards cross-VIE understanding of the controls over biogeochemistry and ecology by further developing and testing the high-level conceptual model proposed here linked to the inundation return interval and topographic slope. In doing so, we encourage paying careful attention to the spatial and temporal scales of modeling and data generation efforts linked to the return interval and slope.
Our conceptual model can be used to frame and study questions representing science challenges that span all VIEs, such as how greenhouse gas fluxes and biological diversity respond to variable inundation (Fig. 13). Similarly, metabolism research has suggested using a continuum of flow predictability and light availability to better unify river metabolism research (Bernhardt et al., 2022). In this approach, there is no need to bin VIEs into discrete categories (Euliss et al., 2004), many of which have varying definitions and levels of overlap. A given system may also not fit clearly into a single VIE category and/or may transition across categories through time and across space. Rather, we can observe and study continuous response surfaces across multiple physical axes and identify patterns within this quantitative space.
In addition to generating transferable understanding, bringing all VIEs together via studies focused on unifying conceptual models could help raise awareness of VIE diversity, importance, vulnerabilities, and how they may change in the future. This may, in turn, help address the fact that VIEs are often overlooked in terms of conservation and monitoring efforts (Calhoun et al., 2017; Hill et al., 2018; Krabbenhoft et al., 2022; Zimmer et al., 2022). Studying diverse VIEs across broad ranges of key environmental axes can also be used to learn where, along environmental continuums, functional thresholds exist that could help with categorizations important for policy and management (Richardson et al., 2022b).
Cross-VIE understanding of the drivers, patterns, and processes linking inundation to system responses can greatly improve with increased collaboration and communication across scientific fields and systems. Our experience is that communities working in VIEs are scattered across different societies and funding programs. Studying VIEs together via unifying conceptual models tied to environmental continuums can bring these science communities together. To this end, we encourage training and collaborations focused on consistent data generation methods that may be adopted across the VIE community and in pursuit of conceptual unification. In addition, disciplinary conferences could also recognize VIE commonalities with special sessions to bring people together from across the VIE continuum to discuss research needs.
Cross-VIE knowledge and models are needed to address human impacts on environments across the globe. Humans both directly (i.e., dams, weirs, surface water and groundwater abstraction, channelization, draining, invasive species introduction and spread, etc.) and indirectly (i.e., climate change) alter VIEs (Kiss et al., 2019; Maris et al., 2016; Pumo et al., 2016). As climate change and other anthropogenic impacts increasingly alter these already dynamic systems, it is imperative that knowledge and models transcend VIEs. Future environmental change can alter the position of a given VIE within environmental space, including what is depicted in our conceptual model (Fig. 13) (e.g., increasing frequency of storm surges changing the inundation return interval). The ability to predict impacts of such environmental change can be facilitated by mechanistic knowledge that is transferable across the environmental space occupied by VIEs. We hypothesize that unifying VIEs across environmental continuums can help achieve this mechanistic, transferable knowledge.
No data sets were used in this article.
JS contributed to conceptualization, investigation, project administration, visualization, and writing (original draft, review and editing). AJB contributed to conceptualization, investigation, and writing (original draft, review and editing). MHB and AB contributed to investigation, visualization, and writing (original draft, review and editing). JA, EKPB, TD, JMD, JBF, JAG, EH, KLJ, ML, AM, TO, SP, PR, JNS, KR, and RVV contributed to writing (original draft, review and editing). JL contributed to conceptualization, investigation, project administration, visualization, and writing (original draft, review and editing). XC, LKC, and ES contributed to conceptualization, visualization, and writing (original draft, review and editing). NM contributed to investigation and writing (original draft, review and editing). KB, KH, LL, BAM, CT, and EW contributed to conceptualization and writing (original draft, review and editing). KR contributed to conceptualization, supervision, visualization, and writing (original draft, review and editing). EFC, KFP, and MZ contributed to conceptualization and writing (review and editing). IF and JS contributed to visualization and writing (original draft, review and editing). JZ contributed to conceptualization, investigation, and writing (original draft, review and editing). DCA contributed to conceptualization, project administration, and writing (review and editing).
The contact author has declared that none of the authors has any competing interests.
Any use of trade, firm, or product names is for descriptive purposes only and does not imply endorsement by the US Government.
Publisher's note: Copernicus Publications remains neutral with regard to jurisdictional claims made in the text, published maps, institutional affiliations, or any other geographical representation in this paper. While Copernicus Publications makes every effort to include appropriate place names, the final responsibility lies with the authors.
We thank Jon Chorover, Sarah Godsey, Jesus Gomez-Velez, Wei Huang, Roser Matamala, Hyun Song, and Kristen Underwood for contributions to the conceptual directions of this paper. This paper was an outgrowth of the VIE Workshop, and we sincerely thank the participants for their contributions.
James Stegen, Xingyuan Chen, Nate McDowell, Jillian M. Deines, Peter Regier, Kenton Rod, Etienne Fluet-Chouinard, Tim Scheibe, Jianqiu Zheng, Maggi Laan, and Kaizad F. Patel were supported by the Earth & Biological Sciences Program Development Office at Pacific Northwest National Laboratory, a multiprogram national laboratory operated by Battelle for the US Department of Energy. Joshua B. Fisher was supported by NASA's ECOSTRESS Science and Applications Team (ESAT) (80NSSC23K0309). Teri O'Meara was supported by COMPASS-FME, a multi-institutional project supported by the US Department of Energy's Environmental System Science program. Inke Forbrich was supported by US Department of Energy award DE-SC0022108. Michelle H. Busch, Amy J. Burgin, and Erin Seybold were supported by NSF EPSCoR Aquatic Intermittency effects of Microbiomes on Streams: OSF 2019603. Kristin Boye was supported by the Watershed Function Science Focus Area at the SLAC National Accelerator Laboratory funded by the US Department of Energy, Office of Science, Biological and Environmental Research, under contract no. DE-AC02-76SF00515. Joshua Ladau was supported by Arva Intelligence. Thibault Datry was supported by the DRYvER project, which has received funding from the European Union's Horizon 2020 research and innovation program under grant agreement no. 869226. Elizabeth Herndon was supported by the Watershed Dynamics and Evolution Science Focus Area funded through the Department of Energy Office of Science Biological and Environmental Research program.
This paper was edited by Paul Stoy and reviewed by Cedric Laize, Bree Whitehead, and Robert Payn.
Abbott, B. W., Bishop, K., Zarnetske, J. P., Minaudo, C., Chapin, F. S., Krause, S., Hannah, D. M., Conner, L., Ellison, D., Godsey, S. E., Plont, S., Marçais, J., Kolbe, T., Huebner, A., Frei, R. J., Hampton, T., Gu, S., Buhman, M., Sara Sayedi, S., Ursache, O., Chapin, M., Henderson, K. D., and Pinay, G.: Human domination of the global water cycle absent from depictions and perceptions, Nat. Geosci., 12, 533–540, https://doi.org/10.1038/s41561-019-0374-y, 2019.
Acharya, B. S., Bhandari, M., Bandini, F., Pizarro, A., Perks, M., Joshi, D. R., Wang, S., Dogwiler, T., Ray, R. L., Kharel, G., and Sharma, S.: Unmanned aerial vehicles in hydrology and water management: Applications, challenges, and perspectives, Water Resour. Res., 57, e2021WR029925, https://doi.org/10.1029/2021WR029925, 2021.
Adams, R. K. and Spotila, J. A.: The form and function of headwater streams based on field and modeling investigations in the southern Appalachian Mountains, Earth Surf. Proc. Land., 30, 1521–1546, https://doi.org/10.1002/esp.1211, 2005.
Adler, P. B., White, E. P., Lauenroth, W. K., Kaufman, D. M., Rassweiler, A., and Rusak, J. A.: Evidence for a General Species-Time-Area Relationship, Ecology, 86, 2032–2039, https://doi.org/10.1890/05-0067, 2005.
Åhlén, I., Thorslund, J., Hambäck, P., Destouni, G., and Jarsjö, J.: Wetland position in the landscape: Impact on water storage and flood buffering, Ecohydrology, 15, e2458, https://doi.org/10.1002/eco.2458, 2022.
Allen, D. C., Datry, T., Boersma, K. S., Bogan, M. T., Boulton, A. J., Bruno, D., Busch, M. H., Costigan, K. H., Dodds, W. K., Fritz, K. M., Godsey, S. E., Jones, J. B., Kaletova, T., Kampf, S. K., Mims, M. C., Neeson, T. M., Olden, J. D., Pastor, A. V., Poff, N. L., Ruddell, B. L., Ruhi, A., Singer, G., Vezza, P., Ward, A. S., and Zimmer, M.: River ecosystem conceptual models and non-perennial rivers: A critical review, WIREs Water, 7, e1473, https://doi.org/10.1002/wat2.1473, 2020.
Anderson, M. G. and Burt, T. P.: The role of topography in controlling throughflow generation, Earth Surf. Process., 3, 331–344, https://doi.org/10.1002/esp.3290030402, 1978.
Angle, J. C., Morin, T. H., Solden, L. M., Narrowe, A. B., Smith, G. J., Borton, M. A., Rey-Sanchez, C., Daly, R. A., Mirfenderesgi, G., Hoyt, D. W., Riley, W. J., Miller, C. S., Bohrer, G., and Wrighton, K. C.: Methanogenesis in oxygenated soils is a substantial fraction of wetland methane emissions, Nat. Commun., 8, 1567, https://doi.org/10.1038/s41467-017-01753-4, 2017.
Anon: Scientific Investigations Report, 2015.
Appels, W. M., Bogaart, P. W., and van der Zee, S. E. A. T. M.: Surface runoff in flat terrain: How field topography and runoff generating processes control hydrological connectivity, J. Hydrol., 534, 493–504, https://doi.org/10.1016/j.jhydrol.2016.01.021, 2016.
Arce, M. I., Mendoza-Lera, C., Almagro, M., Catalán, N., Romaní, A. M., Martí, E., Gómez, R., Bernal, S., Foulquier, A., Mutz, M., Marcé, R., Zoppini, A., Gionchetta, G., Weigelhofer, G., Del Campo, R., Robinson, C. T., Gilmer, A., Rulik, M., Obrador, B., Shumilova, O., Zlatanović, S., Arnon, S., Baldrian, P., Singer, G., Datry, T., Skoulikidis, N., Tietjen, B., and Von Schiller, D.: A conceptual framework for understanding the biogeochemistry of dry riverbeds through the lens of soil science, Earth-Sci. Rev., 188, 441–453, https://doi.org/10.1016/j.earscirev.2018.12.001, 2019.
Arias-Real, R., Delgado-Baquerizo, M., Sabater, S., Gutiérrez-Cánovas, C., Valencia, E., Aragón, G., Cantón, Y., Datry, T., Giordani, P., Medina, N. G., de los Ríos, A., Romaní, A. M., Weber, B., and Hurtado, P.: Unfolding the dynamics of ecosystems undergoing alternating wet-dry transitional states, Ecol. Lett., 27, e14488, https://doi.org/10.1111/ele.14488, 2024.
Arim, M., Pinelli, V., Rodríguez-Tricot, L., Ortiz, E., Illarze, M., Fagúndez-Pachón, C., and Borthagaray, A. I.: Chance and necessity in the assembly of plant communities: Stochasticity increases with size, isolation and diversity of temporary ponds, J. Ecol., 111, 1641–1655, https://doi.org/10.1111/1365-2745.14119, 2023.
Arnesen, A. S., Silva, T. S. F., Hess, L. L., Novo, E. M. L. M., Rudorff, C. M., Chapman, B. D., and McDonald, K. C.: Monitoring flood extent in the lower Amazon River floodplain using ALOS/PALSAR ScanSAR images, Remote Sens. Environ., 130, 51–61, https://doi.org/10.1016/j.rse.2012.10.035, 2013.
Arnold, W., Salazar, J. Z., Carlino, A., Giuliani, M., and Castelletti, A.: Operations eclipse sequencing in multipurpose dam planning, Earth's Future, 11, e2022EF003186, https://doi.org/10.1029/2022EF003186, 2023.
Arrigo, K. R., Van Dijken, G. L., Cameron, M. A., Van Der Grient, J., Wedding, L. M., Hazen, L., Leape, J., Leonard, G., Merkl, A., Micheli, F., Mills, M. M., Monismith, S., Ouellette, N. T., Zivian, A., Levi, M., and Bailey, R. M.: Synergistic interactions among growing stressors increase risk to an Arctic ecosystem, Nat. Commun., 11, 6255, https://doi.org/10.1038/s41467-020-19899-z, 2020.
Arscott, D. B., Tockner, K., Van Der Nat, D., and Ward, J. V.: Aquatic habitat dynamics along a braided Alpine river ecosystem (Tagliamento River, Northeast Italy), Ecosystems, 5, 0802–0814, https://doi.org/10.1007/s10021-002-0192-7, 2002.
Atchley, A. L., Painter, S. L., Harp, D. R., Coon, E. T., Wilson, C. J., Liljedahl, A. K., and Romanovsky, V. E.: Using field observations to inform thermal hydrology models of permafrost dynamics with ATS (v0.83), Geosci. Model Dev., 8, 2701–2722, https://doi.org/10.5194/gmd-8-2701-2015, 2015.
Bain, M. B., Finn, J. T., and Booke, H. E.: Streamflow regulation and fish community structure, Ecology, 69, 382–392, https://doi.org/10.2307/1940436, 1988.
Bam, E. K. P., Ireson, A. M., Kamp, G., and Hendry, J. M.: Ephemeral ponds: Are they the dominant source of depression-focused groundwater recharge?, Water Resour. Res., 56, e2019WR02664, https://doi.org/10.1029/2019WR026640, 2020.
Banach, A. M., Banach, K., Visser, E. J. W., Stępniewska, Z., Smits, A. J. M., Roelofs, J. G. M., and Lamers, L. P. M.: Effects of summer flooding on floodplain biogeochemistry in Poland; implications for increased flooding frequency, Biogeochemistry, 92, 247–262, https://doi.org/10.1007/s10533-009-9291-2, 2009.
Baptist, M. J., Dankers, P., Cleveringa, J., Sittoni, L., Willemsen, P. W. J. M., van Puijenbroek, M. E. B., de Vries, B. M. L., Leuven, J. R. F. W., Coumou, L., Kramer, H., and Elschot, K.: Salt marsh construction as a nature-based solution in an estuarine social-ecological system, Nature-Based Solutions, 1, 100005, https://doi.org/10.1016/j.nbsj.2021.100005, 2021.
Barczok, M., Smith, C., Di Domenico, N., Kinsman-Costello, L., and Herndon, E.: Variability in soil redox response to seasonal flooding in a vernal pond, Front. Environ. Sci., 11, 1114814, https://doi.org/10.3389/fenvs.2023.1114814, 2023.
Batson, J., Noe, G. B., Hupp, C. R., Krauss, K. W., Rybicki, N. B., and Schenk, E. R.: Soil greenhouse gas emissions and carbon budgeting in a short-hydroperiod floodplain wetland, J. Geophys. Res.-Biogeo., 120, 77–95, https://doi.org/10.1002/2014JG002817, 2015.
Beckingham, B., Callahan, T., and Vulava, V.: Stormwater Ponds in the Southeastern U.S. Coastal Plain: Hydrogeology, Contaminant Fate, and the Need for a Social-Ecological Framework, Front. Environ. Sci., 7, 1–14, https://doi.org/10.3389/fenvs.2019.00117, 2019.
Belyea, L. R. and Baird, A. J.: Beyond The limits to peat bog growth: cross-scale feedback in peatland development, Ecol. Monogr., 76, 299–322, https://doi.org/10.1890/0012-9615(2006)076[0299:BTLTPB]2.0.CO;2, 2006.
Benstead, J. P. and Leigh, D. S.: An expanded role for river networks, Nat. Geosci., 5, 678–679, https://doi.org/10.1038/ngeo1593, 2012.
Bernhardt, E. S., Blaszczak, J. R., Ficken, C. D., Fork, M. L., Kaiser, K. E., and Seybold, E. C.: Control points in ecosystems: Moving beyond the hot spot hot moment concept, Ecosystems, 20, 665–682, https://doi.org/10.1007/s10021-016-0103-y, 2017.
Bernhardt, E. S., Savoy, P., Vlah, M. J., Appling, A. P., Koenig, L. E., Hall, R. O., Arroita, M., Blaszczak, J. R., Carter, A. M., Cohen, M., Harvey, J. W., Heffernan, J. B., Helton, A. M., Hosen, J. D., Kirk, L., McDowell, W. H., Stanley, E. H., Yackulic, C. B., and Grimm, N. B.: Light and flow regimes regulate the metabolism of rivers, P. Natl. Acad. Sci. USA, 119, e2121976119, https://doi.org/10.1073/pnas.2121976119, 2022.
Bertolini, C. and da Mosto, J.: Restoring for the climate: a review of coastal wetland restoration research in the last 30 years, Restor. Ecol., 29, e13438, https://doi.org/10.1111/rec.13438, 2021.
Betson, R. P. and Marius, J. B.: Source areas of storm runoff, Water Resour. Res., 5, 574–582, https://doi.org/10.1029/WR005i003p00574, 1969.
Bettez, N. D. and Groffman, P. M.: Denitrification potential in stormwater control structures and natural riparian zones in an urban landscape, Environ. Sci. Technol., 46, 10909–10917, https://doi.org/10.1021/es301409z, 2012.
Bevacqua, E., Vousdoukas, M. I., Zappa, G., Hodges, K., Shepherd, T. G., Maraun, D., Mentaschi, L., and Feyen, L.: More meteorological events that drive compound coastal flooding are projected under climate change, Commun. Earth Environ., 1, 47, https://doi.org/10.1038/s43247-020-00044-z, 2020.
Biancamaria, S., Lettenmaier, D. P., and Pavelsky, T. M.: The SWOT Mission and its capabilities for land hydrology, Surv. Geophys., 37, 307–337, https://doi.org/10.1007/s10712-015-9346-y, 2016.
Bie, W., Fei, T., Liu, X., Liu, H., and Wu, G.: Small water bodies mapped from Sentinel-2 MSI (MultiSpectral Imager) imagery with higher accuracy, Int. J. Remote Sens., 41, 7912–7930, https://doi.org/10.1080/01431161.2020.1766150, 2020.
Blaurock, K., Garthen, P., Gilfedder, B. S., Fleckenstein, J. H., Peiffer, S., and Hopp, L.: Elucidating sources and pathways of dissolved organic carbon in a small, forested catchment – A qualitative assessment of stream, soil and shallow groundwater, EGU General Assembly 2021, online, 19–30 Apr 2021, EGU21-15785, https://doi.org/10.5194/egusphere-egu21-15785, 2021.
Bloom, A. A., Palmer, P. I., Fraser, A., Reay, D. S., and Frankenberg, C.: Large-scale controls of methanogenesis inferred from methane and gravity spaceborne data, Science, 327, 322–325, https://doi.org/10.1126/science.1175176, 2010.
Bloom, A. A., Bowman, K. W., Lee, M., Turner, A. J., Schroeder, R., Worden, J. R., Weidner, R., McDonald, K. C., and Jacob, D. J.: A global wetland methane emissions and uncertainty dataset for atmospheric chemical transport models (WetCHARTs version 1.0), Geosci. Model Dev., 10, 2141–2156, https://doi.org/10.5194/gmd-10-2141-2017, 2017.
Bogaard, T. A. and Greco, R.: Landslide hydrology: from hydrology to pore pressure, WIREs Water, 3, 439–459, https://doi.org/10.1002/wat2.1126, 2016.
Bogan, M. T., Chester, E. T., Datry, T., Murphy, A. L., Robson, B. J., Ruhi, A., Stubbington, R., and Whitney, J. E.: Resistance, Resilience, and Community Recovery in Intermittent Rivers and Ephemeral Streams, in: Intermittent Rivers and Ephemeral Streams, Elsevier, 349–376, https://doi.org/10.1016/B978-0-12-803835-2.00013-9, 2017a.
Bogan, M. T., Chester, E. T., Datry, T., Murphy, A. L., Robson, B. J., Ruhi, A., Stubbington, R., and Whitney, J. E.: Resistance, Resilience, and Community Recovery in Intermittent Rivers and Ephemeral Streams, in: Intermittent Rivers and Ephemeral Streams, Elsevier, 349–376, https://doi.org/10.1016/B978-0-12-803835-2.00013-9, 2017b.
Bogard, M. J., Bergamaschi, B. A., Butman, D. E., Anderson, F., Knox, S. H., and Windham-Myers, L.: Hydrologic export is a major component of coastal wetland carbon budgets, Global Biogeochem. Cy., 34, e2019GB006430, https://doi.org/10.1029/2019GB006430, 2020.
Bonada, N., Rieradevall, M., and Prat, N.: Macroinvertebrate community structure and biological traits related to flow permanence in a Mediterranean river network, Hydrobiologia, 589, 91–106, https://doi.org/10.1007/s10750-007-0723-5, 2007.
Bonython, C. W. and Mason, B.: The Filling and Drying of Lake Eyre, Geogr. J., 119, 321–330, https://doi.org/10.2307/1790646, 1953.
Borch, T., Kretzchmar, R., Kappler, A., Van Cappellen, P., Ginder-Vogel, M., and Campbell, K.: Biogeochemical redox processes and their impact on contaminant dynamics, Environ. Sci. Technol., 44, 15–23, 2010.
Bornette, G., Amoros, C., Piegay, H., Tachet, J., and Hein, T.: Ecological complexity of wetlands within a river landscape, Biol. Conserv., 85, 35–45, https://doi.org/10.1016/S0006-3207(97)00166-3, 1998.
Bourke, S. A., Shanafield, M., Hedley, P., Chapman, S., and Dogramaci, S.: A hydrological framework for persistent pools along non-perennial rivers, Hydrol. Earth Syst. Sci., 27, 809–836, https://doi.org/10.5194/hess-27-809-2023, 2023.
Brantley, S. L., Lebedeva, M. I., Balashov, V. N., Singha, K., Sullivan, P. L., and Stinchcomb, G.: Toward a conceptual model relating chemical reaction fronts to water flow paths in hills, Geomorphology, 277, 100–117, https://doi.org/10.1016/j.geomorph.2016.09.027, 2017.
Braswell, A. E. and Heffernan, J. B.: Coastal wetland distributions: Delineating domains of macroscale drivers and local feedbacks, Ecosystems, 22, 1256–1270, https://doi.org/10.1007/s10021-018-0332-3, 2019.
Braswell, A. E., Leyk, S., Connor, D. S., and Uhl, J. H.: Creeping disaster along the U.S. coastline: Understanding exposure to sea level rise and hurricanes through historical development, PLoS ONE, 17, e0269741, https://doi.org/10.1371/journal.pone.0269741, 2022.
Brazier, R. E., Puttock, A., Graham, H. A., Auster, R. E., Davies, K. H., and Brown, C. M. L.: Beaver: Nature's ecosystem engineers, WIREs Water, 8, e1494, https://doi.org/10.1002/wat2.1494, 2021.
Brendonck, L., Pinceel, T., and Ortells, R.: Dormancy and dispersal as mediators of zooplankton population and community dynamics along a hydrological disturbance gradient in inland temporary pools, Hydrobiologia, 796, 201–222, https://doi.org/10.1007/s10750-016-3006-1, 2017.
Brinson, M.: A Hydrogeomorphic classification for wetlands, U.S. Army Corps of Engineers, Washington, DC, Technical report WRP-DE-4, NSN 7540-01-280-5500, 1993.
Brooks, R. T.: Weather-related effects on woodland vernal pool hydrology and hydroperiod, Wetlands, 24, 104–114, https://doi.org/10.1672/0277-5212(2004)024[0104:WEOWVP]2.0.CO;2, 2004.
Burt, T. P. and Swank, W. T.: Hursh CR and Brater EF (1941) Separating storm-hydrographs from small drainage-areas into surface- and subsurface-flow. Transactions, American Geophysical Union 22: 863–871, Progress in Physical Geography: Earth and Environment, 34, 719–726, https://doi.org/10.1177/0309133310377040, 2010.
Busch, M. H., Costigan, K. H., Fritz, K. M., Datry, T., Krabbenhoft, C. A., Hammond, J. C., Zimmer, M., Olden, J. D., Burrows, R. M., Dodds, W. K., Boersma, K. S., Shanafield, M., Kampf, S. K., Mims, M. C., Bogan, M. T., Ward, A. S., Perez Rocha, M., Godsey, S., Allen, G. H., Blaszczak, J. R., Jones, C. N., and Allen, D. C.: What's in a name? Patterns, trends, and suggestions for defining non-perennial rivers and streams, Water, 12, 1980, https://doi.org/10.3390/w12071980, 2020.
Buszka, T. T. and Reeves, D. M.: Pathways and timescales associated with nitrogen transport from septic systems in coastal aquifers intersected by canals, Hydrogeol. J., 29, 1953–1964, https://doi.org/10.1007/s10040-021-02362-8, 2021.
Calhoun, A. J. K., Mushet, D. M., Bell, K. P., Boix, D., Fitzsimons, J. A., and Isselin-Nondedeu, F.: Temporary wetlands: challenges and solutions to conserving a “disappearing” ecosystem, Biol. Conserv., 211, 3–11, https://doi.org/10.1016/j.biocon.2016.11.024, 2017.
Cantelon, J. A., Guimond, J. A., Robinson, C. E., Michael, H. A., and Kurylyk, B. L.: Vertical saltwater intrusion in coastal aquifers driven by episodic flooding: A review, Water Resour. Res., 58, e2022WR032614, https://doi.org/10.1029/2022WR032614, 2022.
Capps, K. A., Rancatti, R., Tomczyk, N., Parr, T. B., Calhoun, A. J. K., and Hunter, M.: Biogeochemical hotspots in forested landscapes: The role of vernal pools in denitrification and organic matter processing, Ecosystems, 17, 1455–1468, https://doi.org/10.1007/s10021-014-9807-z, 2014.
Casanova, M. T. and Brock, M. A.: How do depth, duration and frequency of flooding influence the establishment of wetland plant communities?, Plant Ecol., 147, 237–250, https://doi.org/10.1023/A:1009875226637, 2000.
Castaldelli, G., Soana, E., Racchetti, E., Vincenzi, F., Fano, E. A., and Bartoli, M.: Vegetated canals mitigate nitrogen surplus in agricultural watersheds, Agr. Ecosyst. Environ., 212, 253–262, https://doi.org/10.1016/j.agee.2015.07.009, 2015.
Castañeda-Moya, E., Rivera-Monroy, V. H., Chambers, R. M., Zhao, X., Lamb-Wotton, L., Gorsky, A., Gaiser, E. E., Troxler, T. G., Kominoski, J. S., and Hiatt, M.: Hurricanes fertilize mangrove forests in the Gulf of Mexico (Florida Everglades, USA), P. Natl. Acad. Sci. USA, 117, 4831–4841, https://doi.org/10.1073/pnas.1908597117, 2020.
Cawley, K. M., Yamashita, Y., Maie, N., and Jaffé, R.: Using optical properties to quantify fringe mangrove inputs to the dissolved organic matter (DOM) pool in a subtropical estuary, Estuar. Coast., 37, 399–410, https://doi.org/10.1007/s12237-013-9681-5, 2014.
Celi, J. E. and Hamilton, S. K.: Measuring Floodplain Inundation Using Diel Amplitude of Temperature, Sensors, 20, 6189, https://doi.org/10.3390/s20216189, 2020.
Chambers, L. G., Steinmuller, H. E., and Breithaupt, J. L.: Toward a mechanistic understanding of “peat collapse” and its potential contribution to coastal wetland loss, Ecology, 100, e02720, https://doi.org/10.1002/ecy.2720, 2019.
Chapin, T. P., Todd, A. S., and Zeigler, M. P.: Robust, low-cost data loggers for stream temperature, flow intermittency, and relative conductivity monitoring, Water Resour. Res., 50, 6542–6548, https://doi.org/10.1002/2013WR015158, 2014.
Chen, B. and Wise, D. H.: Bottom-up limitation of predaceous arthropods in a detritus-based terrestrial food web, Ecology, 80, 761–772, https://doi.org/10.1890/0012-9658(1999)080[0761:BULOPA]2.0.CO;2, 1999.
Cheng, F. Y., Park, J., Kumar, M., and Basu, N. B.: Disconnectivity matters: the outsized role of small ephemeral wetlands in landscape-scale nutrient retention, Environ. Res. Lett., 18, 024018, https://doi.org/10.1088/1748-9326/acab17, 2023.
Choularton, T. W. and Perry, S. J.: A model of the orographic enhancement of snowfall by the seeder-feeder mechanism, Q. J. Roy. Meteor. Soc., 112, 335–345, https://doi.org/10.1002/qj.49711247204, 1986.
Clark, K. E., Torres, M. A., West, A. J., Hilton, R. G., New, M., Horwath, A. B., Fisher, J. B., Rapp, J. M., Robles Caceres, A., and Malhi, Y.: The hydrological regime of a forested tropical Andean catchment, Hydrol. Earth Syst. Sci., 18, 5377–5397, https://doi.org/10.5194/hess-18-5377-2014, 2014.
Clementson, L. A., Richardson, A. J., Rochester, W. A., Oubelkheir, K., Liu, B., D'Sa, E. J., Gusmão, L. F. M., Ajani, P., Schroeder, T., Ford, P. W., Burford, M. A., Saeck, E., and Steven, A. D. L.: Effect of a once in 100-year flood on a subtropical coastal phytoplankton community, Front. Mar. Sci., 8, 580516, https://doi.org/10.3389/fmars.2021.580516, 2021.
Clifford, C. and Heffernan, J.: Artificial aquatic ecosystems, Water, 10, 1096, https://doi.org/10.3390/w10081096, 2018.
Clifford, C. C. and Heffernan, J. B.: North Carolina coastal plain ditch types support distinct hydrophytic communities, Wetlands, 43, 56, https://doi.org/10.1007/s13157-023-01703-5, 2023.
Colbert, A. J. and Soden, B. J.: Climatological variations in North Atlantic tropical cyclone tracks, J. Climate, 25, 657–673, https://doi.org/10.1175/JCLI-D-11-00034.1, 2012.
Coles, A. E., McConkey, B. G., and McDonnell, J. J.: Climate change impacts on hillslope runoff on the northern Great Plains, 1962–2013, J. Hydrol., 550, 538–548, https://doi.org/10.1016/j.jhydrol.2017.05.023, 2017.
Colmer, T. D.: Long-distance transport of gases in plants: A perspective on internal aeration and radial oxygen loss from roots: Gas transport in plants, Plant Cell Environ., 26, 17–36, https://doi.org/10.1046/j.1365-3040.2003.00846.x, 2003.
Connolly, R. M.: Differences in trophodynamics of commercially important fish between artificial waterways and natural coastal wetlands, Estuar. Coast. Shelf Sci., 58, 929–936, https://doi.org/10.1016/j.ecss.2003.06.003, 2003.
Cooley, S., Smith, L., Stepan, L., and Mascaro, J.: Tracking dynamic northern surface water changes with high-frequency planet CubeSat imagery, Remote Sens., 9, 1306, https://doi.org/10.3390/rs9121306, 2017.
Coon, E. T., Moulton, J. D., Kikinzon, E., Berndt, M., Manzini, G., Garimella, R., Lipnikov, K., and Painter, S. L.: Coupling surface flow and subsurface flow in complex soil structures using mimetic finite differences, Adv. Water Resour., 144, 103701, https://doi.org/10.1016/j.advwatres.2020.103701, 2020.
Corti, R. and Datry, T.: Invertebrates and sestonic matter in an advancing wetted front travelling down a dry river bed (Albarine, France), Freshwater Sci., 31, 1187–1201, https://doi.org/10.1899/12-017.1, 2012.
Costigan, K. H., Daniels, M. D., and Dodds, W. K.: Fundamental spatial and temporal disconnections in the hydrology of an intermittent prairie headwater network, J. Hydrol., 522, 305–316, https://doi.org/10.1016/j.jhydrol.2014.12.031, 2015.
Costigan, K. H., Jaeger, K. L., Goss, C. W., Fritz, K. M., and Goebel, P. C.: Understanding controls on flow permanence in intermittent rivers to aid ecological research: integrating meteorology, geology and land cover: Integrating Science to Understand Flow Intermittence, Ecohydrol., 9, 1141–1153, https://doi.org/10.1002/eco.1712, 2016.
Costigan, K. H., Kennard, M. J., Leigh, C., Sauquet, E., Datry, T., and Boulton, A. J.: Flow regimes in intermittent rivers and ephemeral streams, in: Intermittent Rivers and Ephemeral Streams, Elsevier, 51–78, https://doi.org/10.1016/B978-0-12-803835-2.00003-6, 2017.
Covino, T.: Hydrologic connectivity as a framework for understanding biogeochemical flux through watersheds and along fluvial networks, Geomorphology, 277, 133–144, https://doi.org/10.1016/j.geomorph.2016.09.030, 2017.
Cowardin, L. M. and Golet, F. C.: US Fish and Wildlife Service 1979 wetland classification: A review, Vegetatio, 118, 139–152, https://doi.org/10.1007/BF00045196, 1995.
Crook, D. A., Buckle, D. J., Morrongiello, J. R., Allsop, Q. A., Baldwin, W., Saunders, T. M., and Douglas, M. M.: Tracking the resource pulse: Movement responses of fish to dynamic floodplain habitat in a tropical river, J. Anim. Ecol., 89, 795–807, https://doi.org/10.1111/1365-2656.13146, 2020.
Crotty, S. M., Ortals, C., Pettengill, T. M., Shi, L., Olabarrieta, M., Joyce, M. A., Altieri, A. H., Morrison, E., Bianchi, T. S., Craft, C., Bertness, M. D., and Angelini, C.: Sea-level rise and the emergence of a keystone grazer alter the geomorphic evolution and ecology of southeast US salt marshes, P. Natl. Acad. Sci. USA, 117, 17891–17902, https://doi.org/10.1073/pnas.1917869117, 2020.
Crump, B. C., Fine, L. M., Fortunato, C. S., Herfort, L., Needoba, J. A., Murdock, S., and Prahl, F. G.: Quantity and quality of particulate organic matter controls bacterial production in the Columbia River estuary, Limnol. Oceanogr., 62, 2713–2731, https://doi.org/10.1002/lno.10601, 2017.
Cubley, E. S., Cooper, D. J., and Merritt, D. M.: Are riparian vegetation flow response guilds transferable between rivers?, Freshwater Biol., 68, 406–424, https://doi.org/10.1111/fwb.14034, 2023.
Culley, S., Noble, S., Yates, A., Timbs, M., Westra, S., Maier, H. R., Giuliani, M., and Castelletti, A.: A bottom-up approach to identifying the maximum operational adaptive capacity of water resource systems to a changing climate, Water Resour. Res., 52, 6751–6768, https://doi.org/10.1002/2015WR018253, 2016.
Dang, C., Morrissey, E. M., Neubauer, S. C., and Franklin, R. B.: Novel microbial community composition and carbon biogeochemistry emerge over time following saltwater intrusion in wetlands, Glob. Change Biol., 25, 549–561, https://doi.org/10.1111/gcb.14486, 2019.
Daniel, J. and Rooney, R. C.: Wetland hydroperiod predicts community structure, but not the magnitude of cross-community congruence, Sci. Rep., 11, 429, https://doi.org/10.1038/s41598-020-80027-4, 2021.
Datry, T. and Larned, S. T.: River flow controls ecological processes and invertebrate assemblages in subsurface flowpaths of an ephemeral river reach, Can. J. Fish. Aquat. Sci., 65, 1532–1544, https://doi.org/10.1139/F08-075, 2008.
Datry, T., Foulquier, A., Corti, R., von Schiller, D., Tockner, K., Mendoza-Lera, C., Clément, J. C., Gessner, M. O., Moleón, M., Stubbington, R., Gücker, B., Albariño, R., Allen, D. C., Altermatt, F., Arce, M. I., Arnon, S., Banas, D., Banegas-Medina, A., Beller, E., Blanchette, M. L., Blanco-Libreros, J. F., Blessing, J. J., Boëchat, I. G., Boersma, K. S., Bogan, M. T., Bonada, N., Bond, N. R., Brintrup Barría, K. C., Bruder, A., Burrows, R. M., Cancellario, T., Canhoto, C., Carlson, S. M., Cauvy-Fraunié, S., Cid, N., Danger, M., de Freitas Terra, B., De Girolamo, A. M., de La Barra, E., del Campo, R., Diaz-Villanueva, V. D., Dyer, F., Elosegi, A., Faye, E., Febria, C., Four, B., Gafny, S., Ghate, S. D., Gómez, R., Gómez-Gener, L., Graça, M. a. S., Guareschi, S., Hoppeler, F., Hwan, J. L., Jones, J. I., Kubheka, S., Laini, A., Langhans, S. D., Leigh, C., Little, C. J., Lorenz, S., Marshall, J. C., Martín, E., McIntosh, A. R., Meyer, E. I., Miliša, M., Mlambo, M. C., Morais, M., Moya, N., Negus, P. M., Niyogi, D. K., Papatheodoulou, A., Pardo, I., Pařil, P., Pauls, S. U., Pešić, V., Polášek, M., Robinson, C. T., Rodríguez-Lozano, P., Rolls, R. J., Sánchez-Montoya, M. M., Savić, A., Shumilova, O., Sridhar, K. R., Steward, A. L., Storey, R., Taleb, A., Uzan, A., Vander Vorste, R., Waltham, N. J., Woelfle-Erskine, C., Zak, D., Zarfl, C., and Zoppini, A.: A global analysis of terrestrial plant litter dynamics in non-perennial waterways, Nat. Geosci., 11, 497–503, https://doi.org/10.1038/s41561-018-0134-4, 2018a.
Datry, T., Foulquier, A., Corti, R., Von Schiller, D., Tockner, K., Mendoza-Lera, C., Clément, J. C., Gessner, M. O., Moleón, M., Stubbington, R., Gücker, B., Albariño, R., Allen, D. C., Altermatt, F., Arce, M. I., Arnon, S., Banas, D., Banegas-Medina, A., Beller, E., Blanchette, M. L., Blanco-Libreros, J. F., Blessing, J. J., Boëchat, I. G., Boersma, K. S., Bogan, M. T., Bonada, N., Bond, N. R., Brintrup Barría, K. C., Bruder, A., Burrows, R. M., Cancellario, T., Canhoto, C., Carlson, S. M., Cauvy-Fraunié, S., Cid, N., Danger, M., De Freitas Terra, B., De Girolamo, A. M., De La Barra, E., Del Campo, R., Diaz-Villanueva, V. D., Dyer, F., Elosegi, A., Faye, E., Febria, C., Four, B., Gafny, S., Ghate, S. D., Gómez, R., Gómez-Gener, L., Graça, M. A. S., Guareschi, S., Hoppeler, F., Hwan, J. L., Jones, J. I., Kubheka, S., Laini, A., Langhans, S. D., Leigh, C., Little, C. J., Lorenz, S., Marshall, J. C., Martín, E., McIntosh, A. R., Meyer, E. I., Miliša, M., Mlambo, M. C., Morais, M., Moya, N., Negus, P. M., Niyogi, D. K., Papatheodoulou, A., Pardo, I., Pařil, P., Pauls, S. U., Pešić, V., Polášek, M., Robinson, C. T., Rodríguez-Lozano, P., Rolls, R. J., Sánchez-Montoya, M. M., Savić, A., Shumilova, O., Sridhar, K. R., Steward, A. L., Storey, R., Taleb, A., Uzan, A., Vander Vorste, R., Waltham, N. J., Woelfle-Erskine, C., Zak, D., Zarfl, C., and Zoppini, A.: A global analysis of terrestrial plant litter dynamics in non-perennial waterways, Nat. Geosci., 11, 497–503, https://doi.org/10.1038/s41561-018-0134-4, 2018b.
Datry, T., Boulton, A. J., Bonada, N., Fritz, K., Leigh, C., Sauquet, E., Tockner, K., Hugueny, B., and Dahm, C. N.: Flow intermittence and ecosystem services in rivers of the Anthropocene, J. Appl. Ecol., 55, 353–364, https://doi.org/10.1111/1365-2664.12941, 2018c.
Datry, T., Truchy, A., Olden, J. D., Busch, M. H., Stubbington, R., Dodds, W. K., Zipper, S., Yu, S., Messager, M. L., Tonkin, J. D., Kaiser, K. E., Hammond, J. C., Moody, E. K., Burrows, R. M., Sarremejane, R., DelVecchia, A. G., Fork, M. L., Little, C. J., Walker, R. H., Walters, A. W., and Allen, D.: Causes, responses, and implications of anthropogenic versus natural flow intermittence in river networks, BioScience, 73, 9–22, https://doi.org/10.1093/biosci/biac098, 2023.
Davidson, EriC. A., Belk, E., and Boone, R. D.: Soil water content and temperature as independent or confounded factors controlling soil respiration in a temperate mixed hardwood forest, Glob. Change Biol., 4, 217–227, https://doi.org/10.1046/j.1365-2486.1998.00128.x, 1998.
Davidson, N. C., Fluet-Chouinard, E., and Finlayson, C. M.: Global extent and distribution of wetlands: trends and issues, Mar. Freshwater Res., 69, 620–627, https://doi.org/10.1071/MF17019, 2018.
Davidson, T. A., Mackay, A. W., Wolski, P., Mazebedi, R., Murray-Hudson, M., and Todd, M.: Seasonal and spatial hydrological variability drives aquatic biodiversity in a flood-pulsed, sub-tropical wetland, Freshwater Biology, 57, 1253–1265, https://doi.org/10.1111/j.1365-2427.2012.02795.x, 2012.
Davis, C. A., Dvorett, D., and Bidwell, J. R.: Hydrogeomorphic classification and functional assessment, in: Wetland Techniques: Volume 3: Applications and Management, edited by: Anderson, J. T. and Davis, C. A., Springer Netherlands, Dordrecht, 29–68, https://doi.org/10.1007/978-94-007-6907-6_2, 2013.
Day, J. A., Malan, H. L., Malijani, E., and Abegunde, A. P.: Review: Water quality in non-perennial rivers (with erratum), Water SA, 45, 487–500, https://doi.org/10.17159/wsa/2019.v45.i3.6746, 2019.
De Jager, N. R., Thomsen, M., and Yin, Y.: Threshold effects of flood duration on the vegetation and soils of the Upper Mississippi River floodplain, USA, Forest Ecol. Manag., 270, 135–146, https://doi.org/10.1016/j.foreco.2012.01.023, 2012.
De Sassi, C., Lewis, O. T., and Tylianakis, J. M.: Plant-mediated and nonadditive effects of two global change drivers on an insect herbivore community, Ecology, 93, 1892–1901, https://doi.org/10.1890/11-1839.1, 2012.
De Vries, M. E., Rodenburg, J., Bado, B. V., Sow, A., Leffelaar, P. A., and Giller, K. E.: Rice production with less irrigation water is possible in a Sahelian environment, Field Crop. Res., 116, 154–164, https://doi.org/10.1016/j.fcr.2009.12.006, 2010.
Dee, M. M. and Tank, J. L.: Inundation time mediates denitrification end products and carbon limitation in constructed floodplains of an agricultural stream, Biogeochemistry, 149, 141–158, https://doi.org/10.1007/s10533-020-00670-x, 2020.
Del Campo, R., Corti, R., and Singer, G.: Flow intermittence alters carbon processing in rivers through chemical diversification of leaf litter, Limnol. Oceanogr., 6, 232–242, https://doi.org/10.1002/lol2.10206, 2021.
Della Rocca, F., Vignoli, L., and Bologna, M. A.: The reproductive biology of Salamandrina terdigitata (Caudata, Salamandridae), Herpetol. J., 15, 273–278, 2005.
DelVecchia, A. G., Shanafield, M., Zimmer, M. A., Busch, M. H., Krabbenhoft, C. A., Stubbington, R., Kaiser, K. E., Burrows, R. M., Hosen, J., Datry, T., Kampf, S. K., Zipper, S. C., Fritz, K., Costigan, K., and Allen, D. C.: Reconceptualizing the hyporheic zone for nonperennial rivers and streams, Freshwater Sci., 41, 167–182, https://doi.org/10.1086/720071, 2022.
Dietze, M. C., Averill, C., Foster, J., and Wheeler, K.: Ecological Forecasting, Princeton University Press, https://doi.org/10.1093/OBO/97801998300600205, 2017.
Dietze, M. C., Fox, A., Beck-Johnson, L. M., Betancourt, J. L., Hooten, M. B., Jarnevich, C. S., Keitt, T. H., Kenney, M. A., Laney, C. M., Larsen, L. G., Loescher, H. W., Lunch, C. K., Pijanowski, B. C., Randerson, J. T., Read, E. K., Tredennick, A. T., Vargas, R., Weathers, K. C., and White, E. P.: Iterative near-term ecological forecasting: Needs, opportunities, and challenges, P. Natl. Acad. Sci. USA, 115, 1424–1432, https://doi.org/10.1073/pnas.1710231115, 2018.
van Dijk, G., Smolders, A. J. P., Loeb, R., Bout, A., Roelofs, J. G. M., and Lamers, L. P. M.: Salinization of coastal freshwater wetlands; effects of constant versus fluctuating salinity on sediment biogeochemistry, Biogeochemistry, 126, 71–84, https://doi.org/10.1007/s10533-015-0140-1, 2015.
Dissanayake, P., Brown, J., Wisse, P., and Karunarathna, H.: Effects of storm clustering on beach/dune evolution, Mar. Geol., 370, 63–75, https://doi.org/10.1016/j.margeo.2015.10.010, 2015.
Döll, P. and Schmied, H. M.: How is the impact of climate change on river flow regimes related to the impact on mean annual runoff? A global-scale analysis, Environ. Res. Lett., 7, 014037, https://doi.org/10.1088/1748-9326/7/1/014037, 2012.
Du, J., Shen, J., Zhang, Y. J., Ye, F., Liu, Z., Wang, Z., Wang, Y. P., Yu, X., Sisson, M., and Wang, H. V.: Tidal response to sea-level rise in different types of estuaries: The importance of length, bathymetry, and geometry, Geophys. Res. Lett., 45, 227–235, https://doi.org/10.1002/2017GL075963, 2018.
Dube, K., Nhamo, G., and Chikodzi, D.: Flooding trends and their impacts on coastal communities of Western Cape Province, South Africa, GeoJournal, 87, 453–468, https://doi.org/10.1007/s10708-021-10460-z, 2021.
Dugdale, S. J., Klaus, J., and Hannah, D. M.: Looking to the Skies: Realising the Combined Potential of Drones and Thermal Infrared Imagery to Advance Hydrological Process Understanding in Headwaters, Water Resour. Res., 58, e2021WR031168, https://doi.org/10.1029/2021WR031168, 2022.
Ekici, A., Lee, H., Lawrence, D. M., Swenson, S. C., and Prigent, C.: Ground subsidence effects on simulating dynamic high-latitude surface inundation under permafrost thaw using CLM5, Geosci. Model Dev., 12, 5291–5300, https://doi.org/10.5194/gmd-12-5291-2019, 2019.
Elberling, B., Askaer, L., Jørgensen, C. J., Joensen, H. P., Kühl, M., Glud, R. N., and Lauritsen, F. R.: Linking Soil O2, CO2, and CH4 Concentrations in a Wetland Soil: Implications for CO2 and CH4 Fluxes, Environ. Sci. Technol., 45, 3393–3399, https://doi.org/10.1021/es103540k, 2011.
Elberling, B. B., Kovács, G. M., Hansen, H. F. E., Fensholt, R., Ambus, P., Tong, X., Gominski, D., Mueller, C. W., Poultney, D. M. N., and Oehmcke, S.: High nitrous oxide emissions from temporary flooded depressions within croplands, Commun. Earth Environ., 4, 463, https://doi.org/10.1038/s43247-023-01095-8, 2023.
Ensign, S. H. and Noe, G. B.: Tidal extension and sea-level rise: recommendations for a research agenda, Front. Ecol. Environ., 16, 37–43, https://doi.org/10.1002/fee.1745, 2018.
Eppinga, M. B., Rietkerk, M., Borren, W., Lapshina, E. D., Bleuten, W., and Wassen, M. J.: Regular surface patterning of peatlands: Confronting theory with field data, Ecosystems, 11, 520–536, https://doi.org/10.1007/s10021-008-9138-z, 2008.
Euliss, N. H., LaBaugh, J. W., Fredrickson, L. H., Mushet, D. M., Laubhan, M. K., Swanson, G. A., Winter, T. C., Rosenberry, D. O., and Nelson, R. D.: The wetland continuum: A conceptual framework for interpreting biological studies, Wetlands, 24, 448–458, https://doi.org/10.1672/0277-5212(2004)024[0448:TWCACF]2.0.CO;2, 2004.
Fagherazzi, S., Kirwan, M. L., Mudd, S. M., Guntenspergen, G. R., Temmerman, S., D'Alpaos, A., Van De Koppel, J., Rybczyk, J. M., Reyes, E., Craft, C., and Clough, J.: Numerical models of salt marsh evolution: Ecological, geomorphic, and climatic factors, Rev. Geophys., 50, RG1002, https://doi.org/10.1029/2011RG000359, 2012.
Fan, Y. and Miguez-Macho, G.: A simple hydrologic framework for simulating wetlands in climate and earth system models, Clim. Dynam., 37, 253–278, https://doi.org/10.1007/s00382-010-0829-8, 2011.
Fan, Y., Clark, M., Lawrence, D. M., Swenson, S., Band, L. E., Brantley, S. L., Brooks, P. D., Dietrich, W. E., Flores, A., Grant, G., Kirchner, J. W., Mackay, D. S., McDonnell, J. J., Milly, P. C. D., Sullivan, P. L., Tague, C., Ajami, H., Chaney, N., Hartmann, A., Hazenberg, P., McNamara, J., Pelletier, J., Perket, J., Rouholahnejad-Freund, E., Wagener, T., Zeng, X., Beighley, E., Buzan, J., Huang, M., Livneh, B., Mohanty, B. P., Nijssen, B., Safeeq, M., Shen, C., Verseveld, W., Volk, J., and Yamazaki, D.: Hillslope hydrology in global change research and earth system modeling, Water Resour. Res., 55, 1737–1772, https://doi.org/10.1029/2018WR023903, 2019.
Fatichi, S., Vivoni, E. R., Ogden, F. L., Ivanov, V. Y., Mirus, B., Gochis, D., Downer, C. W., Camporese, M., Davison, J. H., Ebel, B., Jones, N., Kim, J., Mascaro, G., Niswonger, R., Restrepo, P., Rigon, R., Shen, C., Sulis, M., and Tarboton, D.: An overview of current applications, challenges, and future trends in distributed process-based models in hydrology, J. Hydrol., 537, 45–60, https://doi.org/10.1016/j.jhydrol.2016.03.026, 2016.
Finlayson, C. M. and Van Der Valk, A. G. (Eds.): Classification and Inventory of the World's Wetlands, Dordrecht, https://doi.org/10.1007/978-94-011-0427-2, 1995.
Fisher, J. B., Lee, B., Purdy, A. J., Halverson, G. H., Dohlen, M. B., Cawse-Nicholson, K., Wang, A., Anderson, R. G., Aragon, B., Arain, M. A., Baldocchi, D. D., Baker, J. M., Barral, H., Bernacchi, C. J., Bernhofer, C., Biraud, S. C., Bohrer, G., Brunsell, N., Cappelaere, B., Castro-Contreras, S., Chun, J., Conrad, B. J., Cremonese, E., Demarty, J., Desai, A. R., De Ligne, A., Foltýnová, L., Goulden, M. L., Griffis, T. J., Grünwald, T., Johnson, M. S., Kang, M., Kelbe, D., Kowalska, N., Lim, J., Maïnassara, I., McCabe, M. F., Missik, J. E. C., Mohanty, B. P., Moore, C. E., Morillas, L., Morrison, R., Munger, J. W., Posse, G., Richardson, A. D., Russell, E. S., Ryu, Y., Sanchez-Azofeifa, A., Schmidt, M., Schwartz, E., Sharp, I., Šigut, L., Tang, Y., Hulley, G., Anderson, M., Hain, C., French, A., Wood, E., and Hook, S.: ECOSTRESS: NASA's next generation mission to measure evapotranspiration from the international space station, Water Resour. Res., 56, e2019WR02605, https://doi.org/10.1029/2019WR026058, 2020.
Flick, R. E., Chadwick, D. B., Briscoe, J., and Harper, K. C.: “Flooding” versus “inundation,” Eos, Transactions American Geophysical Union, 93, 365–366, https://doi.org/10.1029/2012EO380009, 2012.
Florencio, M., Fernández-Zamudio, R., Lozano, M., and Díaz-Paniagua, C.: Interannual variation in filling season affects zooplankton diversity in Mediterranean temporary ponds, Hydrobiologia, 847, 1195–1205, https://doi.org/10.1007/s10750-019-04163-3, 2020.
Fortesa, J., Ricci, G. F., García-Comendador, J., Gentile, F., Estrany, J., Sauquet, E., Datry, T., and De Girolamo, A. M.: Analysing hydrological and sediment transport regime in two Mediterranean intermittent rivers, Catena, 196, 104865, https://doi.org/10.1016/j.catena.2020.104865, 2021.
Fournier, R. J., De Mendoza, G., Sarremejane, R., and Ruhi, A.: Isolation controls reestablishment mechanisms and post-drying community structure in an intermittent stream, Ecology, 104, e3911, https://doi.org/10.1002/ecy.3911, 2023.
Fredrickson, L. and Taylor, T. S.: Management of seasonally flooded impoundments for wildlife. Resource Publication 148, U.S. Fish and Wildlife Service, 29 pp., 1982.
Freeze, R. A.: Streamflow generation, Rev. Geophys., 12, 627–647, https://doi.org/10.1029/RG012i004p00627, 1974.
Fryirs, K. and Brierley, G.: Assemblages of geomorphic units: A building block approach to analysis and interpretation of river character, behaviour, condition and recovery, Earth Surf. Proc. Land., 47, 92–108, https://doi.org/10.1002/esp.5264, 2022.
Gallant, A.: The challenges of remote monitoring of wetlands, Remote Sens., 7, 10938–10950, https://doi.org/10.3390/rs70810938, 2015.
Garayburu-Caruso, V. A., Danczak, R. E., Stegen, J. C., Renteria, L., Mccall, M., Goldman, A. E., Chu, R. K., Toyoda, J., Resch, C. T., Torgeson, J. M., Wells, J., Fansler, S., Kumar, S., and Graham, E. B.: Using community science to reveal the global chemogeography of river metabolomes, Metabolites, 10, 518, https://doi.org/10.3390/metabo10120518, 2020.
Gates, J. B., Chittaro, P. M., and Veggerby, K. B.: Standard operating procedures for measuring bulk stable isotope values of nitrogen and carbon in marine biota by isotope ratio mass spectrometry (IRMS), U.S. Department of Commerce, NOAA Processed Report NMFS-NWFSC-PR-2020-04, https://doi.org/10.25923/3MWP-CE02, 2020.
Gendreau, K. L., Buxton, V., Moore, C. E., and Mims, M.: Temperature loggers capture intraregional variation of inundation timing for intermittent ponds, Water Resour. Res., 57, e2021WR029958, https://doi.org/10.1029/2021WR029958, 2021.
Gittman, R. K., Fodrie, F. J., Popowich, A. M., Keller, D. A., Bruno, J. F., Currin, C. A., Peterson, C. H., and Piehler, M. F.: Engineering away our natural defenses: an analysis of shoreline hardening in the US, Front. Ecol. Environ., 13, 301–307, https://doi.org/10.1890/150065, 2015.
Glaser, B., Hopp, L., Partington, D., Brunner, P., Therrien, R., and Klaus, J.: Sources of surface water in space and time: Identification of delivery processes and geographical sources with hydraulic mixing-cell modeling, Water Resour. Res., 57, e2021WR030332, https://doi.org/10.1029/2021WR030332, 2021.
Gleason, J. E. and Rooney, R. C.: Pond permanence is a key determinant of aquatic macroinvertebrate community structure in wetlands, Freshw. Biol., 63, 264–277, https://doi.org/10.1111/fwb.13057, 2018.
Goldman, A. E., Graham, E. B., Crump, A. R., Kennedy, D. W., Romero, E. B., Anderson, C. G., Dana, K. L., Resch, C. T., Fredrickson, J. K., and Stegen, J. C.: Biogeochemical cycling at the aquatic–terrestrial interface is linked to parafluvial hyporheic zone inundation history, Biogeosciences, 14, 4229–4241, https://doi.org/10.5194/bg-14-4229-2017, 2017.
Goldman, A. E., Emani, S. R., Pérez-Angel, L. C., Rodríguez-Ramos, J. A., and Stegen, J. C.: Integrated, coordinated, open, and networked (ICON) science to advance the geosciences: Introduction and synthesis of a special collection of commentary articles, Earth Space Sci., 9, e2021EA002099, https://doi.org/10.1029/2021EA002099, 2022.
Gomez, J. D., Wilson, J. L., and Cardenas, M. B.: Residence time distributions in sinuosity-driven hyporheic zones and their biogeochemical effects, Water Resour. Res., 48, W09533, https://doi.org/10.1029/2012WR012180, 2012.
Gómez-Gener, L., Siebers, A. R., Arce, M. I., Arnon, S., Bernal, S., Bolpagni, R., Datry, T., Gionchetta, G., Grossart, H.-P., Mendoza-Lera, C., Pohl, V., Risse-Buhl, U., Shumilova, O., Tzoraki, O., Von Schiller, D., Weigand, A., Weigelhofer, G., Zak, D., and Zoppini, A.: Towards an improved understanding of biogeochemical processes across surface-groundwater interactions in intermittent rivers and ephemeral streams, Earth-Sci. Rev., 220, 103724, https://doi.org/10.1016/j.earscirev.2021.103724, 2021.
González, E., Sher, A. A., Tabacchi, E., Masip, A., and Poulin, M.: Restoration of riparian vegetation: A global review of implementation and evaluation approaches in the international, peer-reviewed literature, J. Environ. Manage., 158, 85–94, https://doi.org/10.1016/j.jenvman.2015.04.033, 2015.
Guimond, J. A. and Michael, H. A.: Effects of marsh migration on flooding, saltwater intrusion, and crop yield in coastal agricultural land subject to storm surge inundation, Water Res., 57, e2020WR028326, https://doi.org/10.1029/2020WR028326, 2021.
Hale, R. L., Turnbull, L., Earl, S. R., Childers, D. L., and Grimm, N. B.: Stormwater infrastructure controls runoff and dissolved material export from arid urban watersheds, Ecosystems, 18, 62–75, https://doi.org/10.1007/s10021-014-9812-2, 2015.
Hamilton, S. K., Sippel, S. J., and Melack, J. M.: Comparison of inundation patterns among major South American floodplains, J. Geophys. Res.-Atmos., 107, LBA 5-1–LBA 5-14, https://doi.org/10.1029/2000JD000306, 2002.
Hammond, J. C., Zimmer, M., Shanafield, M., Kaiser, K., Godsey, S. E., Mims, M. C., Zipper, S. C., Burrows, R. M., Kampf, S. K., Dodds, W., Jones, C. N., Krabbenhoft, C. A., Boersma, K. S., Datry, T., Olden, J. D., Allen, G. H., Price, A. N., Costigan, K., Hale, R., Ward, A. S., and Allen, D. C.: Spatial patterns and drivers of nonperennial flow regimes in the contiguous United States, Geophys. Res. Lett., 48, e2020GL090794, https://doi.org/10.1029/2020GL090794, 2021.
Hanson, P. J., Riggs, J. S., Nettles, W. R., Phillips, J. R., Krassovski, M. B., Hook, L. A., Gu, L., Richardson, A. D., Aubrecht, D. M., Ricciuto, D. M., Warren, J. M., and Barbier, C.: Attaining whole-ecosystem warming using air and deep-soil heating methods with an elevated CO2 atmosphere, Biogeosciences, 14, 861–883, https://doi.org/10.5194/bg-14-861-2017, 2017.
Hayashi, M., Van Der Kamp, G., and Rosenberry, D. O.: Hydrology of prairie wetlands: Understanding the integrated surface-water and groundwater processes, Wetlands, 36, 237–254, https://doi.org/10.1007/s13157-016-0797-9, 2016.
Herbert, E. R., Schubauer-Berigan, J., and Craft, C. B.: Differential effects of chronic and acute simulated seawater intrusion on tidal freshwater marsh carbon cycling, Biogeochemistry, 138, 137–154, https://doi.org/10.1007/s10533-018-0436-z, 2018.
Herndon, E. M., Dere, A. L., Sullivan, P. L., Norris, D., Reynolds, B., and Brantley, S. L.: Landscape heterogeneity drives contrasting concentration–discharge relationships in shale headwater catchments, Hydrol. Earth Syst. Sci., 19, 3333–3347, https://doi.org/10.5194/hess-19-3333-2015, 2015.
Herndon, E. M., Steinhoefel, G., Dere, A. L. D., and Sullivan, P. L.: Perennial flow through convergent hillslopes explains chemodynamic solute behavior in a shale headwater catchment, Chem. Geol., 493, 413–425, https://doi.org/10.1016/j.chemgeo.2018.06.019, 2018.
Herzon, I. and Helenius, J.: Agricultural drainage ditches, their biological importance and functioning, Biol. Conserv., 141, 1171–1183, https://doi.org/10.1016/j.biocon.2008.03.005, 2008.
Hess, L. L., Melack, J. M., Affonso, A. G., Barbosa, C., Gastil-Buhl, M., and Novo, E. M. L. M.: Wetlands of the Lowland Amazon Basin: Extent, vegetative cover, and dual-season inundated area as mapped with JERS-1 synthetic aperture radar, Wetlands, 35, 745–756, https://doi.org/10.1007/s13157-015-0666-y, 2015.
Hill, M. J., Hassall, C., Oertli, B., Fahrig, L., Robson, B. J., Biggs, J., Samways, M. J., Usio, N., Takamura, N., Krishnaswamy, J., and Wood, P. J.: New policy directions for global pond conservation, Conserv. Lett., 11, e12447, https://doi.org/10.1111/conl.12447, 2018.
Hill, M. J., Greaves, H. M., Sayer, C. D., Hassall, C., Milin, M., Milner, V. S., Marazzi, L., Hall, R., Harper, L. R., Thornhill, I., Walton, R., Biggs, J., Ewald, N., Law, A., Willby, N., White, J. C., Briers, R. A., Mathers, K. L., Jeffries, M. J., and Wood, P. J.: Pond ecology and conservation: research priorities and knowledge gaps, Ecosphere, 12, e03853, https://doi.org/10.1002/ecs2.3853, 2021.
Hinkel, J., Lincke, D., Vafeidis, A. T., Perrette, M., Nicholls, R. J., Tol, R. S. J., Marzeion, B., Fettweis, X., Ionescu, C., and Levermann, A.: Coastal flood damage and adaptation costs under 21st century sea-level rise, P. Natl. Acad. Sci. USA, 111, 3292–3297, https://doi.org/10.1073/pnas.1222469111, 2014.
Hinshaw, S. E., Tatariw, C., Flournoy, N., Kleinhuizen, A., Taylor, C., Sobecky, P. A., and Mortazavi, B.: Vegetation loss decreases salt marsh denitrification capacity: Implications for marsh erosion, Environ. Sci. Technol., 51, 8245–8253, https://doi.org/10.1021/acs.est.7b00618, 2017.
Hladyz, S., Watkins, S. C., Whitworth, K. L., and Baldwin, D. S.: Flows and hypoxic blackwater events in managed ephemeral river channels, J. Hydrol., 401, 117–125, https://doi.org/10.1016/j.jhydrol.2011.02.014, 2011.
Hofmeister, K. L., Eggert, S. L., Palik, B. J., Morley, D., Creighton, E., Rye, M., and Kolka, R. K.: The identification, mapping, and management of seasonal ponds in forests of the Great Lakes Region, Wetlands, 42, 1–23, https://doi.org/10.1007/s13157-021-01526-2, 2022.
Hondula, K. L., Jones, C. N., and Palmer, M. A.: Effects of seasonal inundation on methane fluxes from forested freshwater wetlands, Environ. Res. Lett., 16, 084016, https://doi.org/10.1088/1748-9326/ac1193, 2021a.
Hondula, K. L., DeVries, B., Jones, C. N., and Palmer, M. A.: Effects of using high resolution satellite-based inundation time series to estimate methane fluxes from forested wetlands, Geophys. Res. Lett., 48, e2021GL092556, https://doi.org/10.1029/2021GL092556, 2021b.
Hooley-Underwood, Z. E., Stevens, S. B., Salinas, N. R., and Thompson, K. G.: An intermittent stream supports extensive spawning of large-river native fishes, T. Am. Fish. Soc., 148, 426–441, https://doi.org/10.1002/tafs.10141, 2019.
Hopple, A. M., Pennington, S. C., Megonigal, J. P., Bailey, V., and Bond-Lamberty, B.: Disturbance legacies regulate coastal forest soil stability to changing salinity and inundation: A soil transplant experiment, Soil Biol. Biochem., 169, 108675, https://doi.org/10.1016/j.soilbio.2022.108675, 2022.
Hopple, A. M., Doro, K. O., Bailey, V. L., Bond-Lamberty, B., McDowell, N., Morris, K. A., Myers-Pigg, A., Pennington, S. C., Regier, P., Rich, R., Sengupta, A., Smith, R., Stegen, J., Ward, N. D., Woodard, S. C., and Megonigal, J. P.: Attaining freshwater and estuarine-water soil saturation in an ecosystem-scale coastal flooding experiment, Environ. Monit. Assess., 195, 425, https://doi.org/10.1007/s10661-022-10807-0, 2023.
Horton, R. E.: An approach toward a physical interpretation of infiltration capacity, Soil Sci. Soc. Am. J., 5, 339–417, https://doi.org/10.2136/sssaj1941.036159950005000C0075x, 1940.
Houser, C. and Hamilton, S.: Sensitivity of post-hurricane beach and dune recovery to event frequency, Earth Surf. Proc. Land., 34, 613–628, https://doi.org/10.1002/esp.1730, 2009.
Huang, C., Gascuel-Odoux, C., and Cros-Cayot, S.: Hillslope topographic and hydrologic effects on overland flow and erosion, Catena, 46, 177–188, https://doi.org/10.1016/S0341-8162(01)00165-5, 2002.
Huang, W., Wang, K., Ye, C., Hockaday, W. C., Wang, G., and Hall, S. J.: High carbon losses from oxygen-limited soils challenge biogeochemical theory and model assumptions, Glob. Change Biol., 27, 6166–6180, https://doi.org/10.1111/gcb.15867, 2021.
Hutchinson, G. E.: Introduction to population ecology, Yale University Press, New Haven, CT, USA, ISBN-10 0300021550, 1978.
Hwang, T., Band, L. E., Vose, J. M., and Tague, C.: Ecosystem processes at the watershed scale: Hydrologic vegetation gradient as an indicator for lateral hydrologic connectivity of headwater catchments, Water Resour. Res., 48, W06514, https://doi.org/10.1029/2011WR011301, 2012.
Ivory, S. J., McGlue, M. M., Spera, S., Silva, A., and Bergier, I.: Vegetation, rainfall, and pulsing hydrology in the Pantanal, the world's largest tropical wetland, Environ. Res. Lett., 14, 124017, https://doi.org/10.1088/1748-9326/ab4ffe, 2019.
Jarecke, K. M., Loecke, T. D., and Burgin, A. J.: Coupled soil oxygen and greenhouse gas dynamics under variable hydrology, Soil Biol. Biochem., 95, 164–172, https://doi.org/10.1016/j.soilbio.2015.12.018, 2016.
Jeffries, M.: The spatial and temporal heterogeneity of macrophyte communities in thirty small, temporary ponds over a period of ten years, Ecography, 31, 765–775, https://doi.org/10.1111/j.0906-7590.2008.05487.x, 2008.
Jones, C. N., Evenson, G. R., McLaughlin, D. L., Vanderhoof, M. K., Lang, M. W., McCarty, G. W., Golden, H. E., Lane, C. R., and Alexander, L. C.: Estimating restorable wetland water storage at landscape scales, Hydrol. Process., 32, 305–313, https://doi.org/10.1002/hyp.11405, 2018.
Jones, J.: Improved automated detection of subpixel-scale inundation: Revised dynamic surface water extent (DSWE) partial surface water tests, Remote Sens., 11, 374, https://doi.org/10.3390/rs11040374, 2019.
Junk, W., Bayley, P., and Sparks, R.: The flood pulse concept in river-floodplain systems, in: Proceedings of the International Large River Symposium (LARS), edited by: Dodge, D. P., Canadian Journal of Fisheries and Aquatic Sciences Special Publication 106, NRC research press, Ottawa, 110–127, 1989.
Kampf, S. K., Dwire, K. A., Fairchild, M. P., Dunham, J., Snyder, C. D., Jaeger, K. L., Luce, C. H., Hammond, J. C., Wilson, C., Zimmer, M. A., and Sidell, M.: Managing nonperennial headwater streams in temperate forests of the United States, Forest Ecol. Manag., 497, 119523, https://doi.org/10.1016/j.foreco.2021.119523, 2021.
Kaushal, S. S., Reimer, J. E., Mayer, P. M., Shatkay, R. R., Maas, C. M., Nguyen, W. D., Boger, W. L., Yaculak, A. M., Doody, T. R., Pennino, M. J., Bailey, N. W., Galella, J. G., Weingrad, A., Collison, D. C., Wood, K. L., Haq, S., Newcomer-Johnson, T. A., Duan, S., and Belt, K. T.: Freshwater salinization syndrome alters retention and release of chemical cocktails along flowpaths: From stormwater management to urban streams, Freshwater Sci., 41, 420–441, https://doi.org/10.1086/721469, 2022.
Kirkby, M., Bracken, L., and Reaney, S.: The influence of land use, soils and topography on the delivery of hillslope runoff to channels in SE Spain, Earth Surf. Proc. Land., 27, 1459–1473, https://doi.org/10.1002/esp.441, 2002.
Kirwan, M. L. and Gedan, K. B.: Sea-level driven land conversion and the formation of ghost forests, Nat. Clim. Chang., 9, 450–457, https://doi.org/10.1038/s41558-019-0488-7, 2019.
Kiss, T., Nagy, J., Fehérváry, I., and Vaszkó, C.: (Mis) management of floodplain vegetation: The effect of invasive species on vegetation roughness and flood levels, Sci. Total Environ., 686, 931–945, https://doi.org/10.1016/j.scitotenv.2019.06.006, 2019.
Kneitel, J. M.: Inundation timing, more than duration, affects the community structure of California vernal pool mesocosms, Hydrobiologia, 732, 71–83, https://doi.org/10.1007/s10750-014-1845-1, 2014.
Kollet, S. J. and Maxwell, R. M.: Integrated surface–groundwater flow modeling: A free-surface overland flow boundary condition in a parallel groundwater flow model, Adv. Water Resour., 29, 945–958, https://doi.org/10.1016/j.advwatres.2005.08.006, 2006.
Konapala, G., Mishra, A. K., Wada, Y., and Mann, M. E.: Climate change will affect global water availability through compounding changes in seasonal precipitation and evaporation, Nat. Commun., 11, 3044, https://doi.org/10.1038/s41467-020-16757-w, 2020.
Koschorreck, M., Downing, A. S., Hejzlar, J., Marcé, R., Laas, A., Arndt, W. G., Keller, P. S., Smolders, A. J. P., van Dijk, G., and Kosten, S.: Hidden treasures: Human-made aquatic ecosystems harbour unexplored opportunities, Ambio, 49, 531–540, https://doi.org/10.1007/s13280-019-01199-6, 2020.
Krabbenhoft, C. A., Allen, G. H., Lin, P., Godsey, S. E., Allen, D. C., Burrows, R. M., DelVecchia, A. G., Fritz, K. M., Shanafield, M., Burgin, A. J., Zimmer, M. A., Datry, T., Dodds, W. K., Jones, C. N., Mims, M. C., Franklin, C., Hammond, J. C., Zipper, S., Ward, A. S., Costigan, K. H., Beck, H. E., and Olden, J. D.: Assessing placement bias of the global river gauge network, Nat. Sustain., 5, 586–592, https://doi.org/10.1038/s41893-022-00873-0, 2022.
Kundel, D., Meyer, S., Birkhofer, H., Fliessbach, A., Mäder, P., Scheu, S., van Kleunen, M., and Birkhofer, K.: Design and manual to construct rainout-shelters for climate shange experiments in agroecosystems, Front. Environ. Sci., 6, 14, https://doi.org/10.3389/fenvs.2018.00014, 2018.
Ladau, J. and Eloe-Fadrosh, E. A.: Spatial, temporal, and phylogenetic scales of microbial ecology, Trends Microbiol., 27, 662–669, https://doi.org/10.1016/j.tim.2019.03.003, 2019.
Lalli, K., Soenen, S., Fisher, J. B., McGlinchy, J., Kleynhans, T., Eon, R., and Moreau, L. M.: VanZyl-1: demonstrating SmallSat measurement capabilities for land surface temperature and evapotranspiration, in: CubeSats and SmallSats for Remote Sensing VI, CubeSats and SmallSats for Remote Sensing VI, San Diego, United States, 8, https://doi.org/10.1117/12.2632565, 2022.
Lane, C. R. and D'Amico, E.: Identification of putative geographically isolated wetlands of the conterminous United States, J. Am. Water Resour. Assoc., 52, 705–722, https://doi.org/10.1111/1752-1688.12421, 2016.
Lane, K., Charles-Guzman, K., Wheeler, K., Abid, Z., Graber, N., and Matte, T.: Health effects of coastal storms and flooding in urban areas: A review and vulnerability assessment, J. Environ. Pub. He., 2013, 913064, https://doi.org/10.1155/2013/913064, 2013.
Laronne, J. B. and Reid, L.: Very high rates of bedload sediment transport by ephemeral desert rivers, Nature, 366, 148–150, https://doi.org/10.1038/366148a0, 1993.
Larsen, L., Aumen, N., Bernhardt, C., Engel, V., Givnish, T., Hagerthey, S., Harvey, J., Leonard, L., McCormick, P., Mcvoy, C., Noe, G., Nungesser, M., Rutchey, K., Sklar, F., Troxler, T., Volin, J., and Willard, D.: Recent and historic drivers of landscape change in the Everglades Ridge, slough, and tree island mosaic, Crit. Rev. Env. Sci. Tec., 41, 344–381, https://doi.org/10.1080/10643389.2010.531219, 2011.
Lei, Y., Liu, C., Zhang, L., and Luo, S.: How smallholder farmers adapt to agricultural drought in a changing climate: A case study in southern China, Land Use Policy, 55, 300–308, https://doi.org/10.1016/j.landusepol.2016.04.012, 2016.
Li, L., Maher, K., Navarre-Sitchler, A., Druhan, J., Meile, C., Lawrence, C., Moore, J., Perdrial, J., Sullivan, P., Thompson, A., Jin, L., Bolton, E. W., Brantley, S. L., Dietrich, W. E., Mayer, K. U., Steefel, C. I., Valocchi, A., Zachara, J., Kocar, B., Mcintosh, J., Tutolo, B. M., Kumar, M., Sonnenthal, E., Bao, C., and Beisman, J.: Expanding the role of reactive transport models in critical zone processes, Earth-Sci. Rev., 165, 280–301, https://doi.org/10.1016/j.earscirev.2016.09.001, 2017.
Li, L., Sullivan, P. L., Benettin, P., Cirpka, O. A., Bishop, K., Brantley, S. L., Knapp, J. L. A., Meerveld, I., Rinaldo, A., Seibert, J., Wen, H., and Kirchner, J. W.: Toward catchment hydro-biogeochemical theories, WIREs Water, 8, e1495, https://doi.org/10.1002/wat2.1495, 2021.
Li, S., Wang, G., Zhu, C., Lu, J., Ullah, W., Hagan, D. F. T., Kattel, G., and Peng, J.: Attribution of global evapotranspiration trends based on the Budyko framework, Hydrol. Earth Syst. Sci., 26, 3691–3707, https://doi.org/10.5194/hess-26-3691-2022, 2022a.
Li, Z., Gao, S., Chen, M., Gourley, J. J., and Hong, Y.: Spatiotemporal characteristics of US floods: Current status and forecast under a future warmer climate, Earth's Future, 10, e2022EF002700, https://doi.org/10.1029/2022EF002700, 2022b.
Liberato, M. L. R., Pinto, J. G., Trigo, R. M., Ludwig, P., Ordóñez, P., Yuen, D., and Trigo, I. F.: Explosive development of winter storm Xynthia over the subtropical North Atlantic Ocean, Nat. Hazards Earth Syst. Sci., 13, 2239–2251, https://doi.org/10.5194/nhess-13-2239-2013, 2013.
Lisenby, P. E., Tooth, S., and Ralph, T. J.: Product vs. process? The role of geomorphology in wetland characterization, Sci. Total Environ., 663, 980–991, https://doi.org/10.1016/j.scitotenv.2019.01.399, 2019.
Lohse, K. A., Brooks, P. D., McIntosh, J. C., Meixner, T., and Huxman, T. E.: Interactions between biogeochemistry and hydrologic systems, Annu. Rev. Env. Resour., 34, 65–96, https://doi.org/10.1146/annurev.environ.33.031207.111141, 2009.
Londe, D. W., Dvorett, D., Davis, C. A., Loss, S. R., and Robertson, E. P.: Inundation of depressional wetlands declines under a changing climate, Clim. Change, 172, 1–19, https://doi.org/10.1007/s10584-022-03386-z, 2022.
Lovelock, C. E. and Reef, R.: Variable impacts of climate change on blue carbon, One Earth, 3, 195–211, https://doi.org/10.1016/j.oneear.2020.07.010, 2020.
Lugo, A. E.: Visible and invisible effects of hurricanes on forest ecosystems: an international review, Austral. Ecol., 33, 368–398, https://doi.org/10.1111/j.1442-9993.2008.01894.x, 2008.
Luijendijk, A., Hagenaars, G., Ranasinghe, R., Baart, F., Donchyts, G., and Aarninkhof, S.: The state of the world's beaches, Sci. Rep., 8, 6641, https://doi.org/10.1038/s41598-018-24630-6, 2018.
Mahecha, M. D., Reichstein, M., Carvalhais, N., Lasslop, G., Lange, H., Seneviratne, S. I., Vargas, R., Ammann, C., Arain, M. A., Cescatti, A., Janssens, I. A., Migliavacca, M., Montagnani, L., and Richardson, A. D.: Global convergence in the temperature sensitivity of respiration at ecosystem level, Science, 329, 838–840, https://doi.org/10.1126/science.1189587, 2010.
Mandishona, E. and Knight, J.: Inland wetlands in Africa: A review of their typologies and ecosystem services, Progress in Physical Geography: Earth and Environment, 46, 547–565, https://doi.org/10.1177/03091333221075328, 2022.
Manfreda, S., McCabe, M. F., Miller, P. E., Lucas, R., Pajuelo Madrigal, V., Mallinis, G., Ben Dor, E., Helman, D., Estes, L., Ciraolo, G., Müllerová, J., Tauro, F., De Lima, M. I., De Lima, J. L. M. P., Maltese, A., Frances, F., Caylor, K., Kohv, M., Perks, M., Ruiz-Pérez, G., Su, Z., Vico, G., and Toth, B.: On the use of unmanned aerial systems for environmental monitoring, Remote Sens., 10, 641, https://doi.org/10.3390/rs10040641, 2018.
Maris, S. C., Teira-Esmatges, M. R., and Català, M. M.: Influence of irrigation frequency on greenhouse gases emission from a paddy soil, Paddy Water Environ., 14, 199–210, https://doi.org/10.1007/s10333-015-0490-2, 2016.
Marton, J. M., Creed, I. F., Lewis, D. B., Lane, C. R., Basu, N. B., Cohen, M. J., and Craft, C. B.: Geographically isolated wetlands are important biogeochemical reactors on the landscape, BioScience, 65, 408–418, https://doi.org/10.1093/biosci/biv009, 2015.
Matthews, J.: Anthropogenic climate change impacts on ponds: a thermal mass perspective, BioRisk, 5, 193–209, https://doi.org/10.3897/biorisk.5.849, 2010.
Maul, G. A. and Duedall, I. W.: Demography of coastal populations, in: Encyclopedia of Coastal Science, edited by: Finkl, C. W. and Makowski, C., Springer International Publishing, Cham, 692–700, https://doi.org/10.1007/978-3-319-93806-6_115, 2019.
McCarthy, J. K., Dwyer, J. M., and Mokany, K.: A regional-scale assessment of using metabolic scaling theory to predict ecosystem properties, P. Roy. Soc. B., 286, 20192221, https://doi.org/10.1098/rspb.2019.2221, 2019.
McClain, M. E., Boyer, E. W., Dent, C. L., Gergel, S. E., Grimm, N. B., Groffman, P. M., Hart, S. C., Harvey, J. W., Johnston, C. A., Mayorga, E., McDowell, W. H., and Pinay, G.: Biogeochemical hot spots and hot moments at the interface of terrestrial and aquatic ecosystems, Ecosystems, 6, 301–312, https://doi.org/10.1007/s10021-003-0161-9, 2003.
McDaniel, P. A., Regan, M. P., Brooks, E., Boll, J., Barndt, S., Falen, A., Young, S. K., and Hammel, J. E.: Linking fragipans, perched water tables, and catchment-scale hydrological processes, Catena, 73, 166–173, https://doi.org/10.1016/j.catena.2007.05.011, 2008.
McDonnell, J. J., Hewlett, J. D., and Hibbert, A. R.: Factors affecting the response of small watersheds to precipitation in humid areas, in: Forest hydrology, edidet by: Sopper, W. E. and Lull, H. W., New York, Pergamon Press, 275—290, Progress in Physical Geography: Earth and Environment, 33, 288–293, https://doi.org/10.1177/0309133309338118, 2009.
McDowell, N. G., Ball, M., Bond-Lamberty, B., Kirwan, M. L., Krauss, K. W., Megonigal, J. P., Mencuccini, M., Ward, N. D., Weintraub, M. N., and Bailey, V.: Processes and mechanisms of coastal woody-plant mortality, Glob. Change Biol., 28, 5881–5900, https://doi.org/10.1111/gcb.16297, 2022.
McDowell, N. G., Anderson-Teixeira, K., Biederman, J. A., Breshears, D. D., Fang, Y., Fernández-de-Uña, L., Graham, E. B., Mackay, D. S., McDonnell, J. J., Moore, G. W., Nehemy, M. F., Stevens Rumann, C. S., Stegen, J., Tague, N., Turner, M. G., and Chen, X.: Ecohydrological decoupling under changing disturbances and climate, One Earth, 6, 251–266, https://doi.org/10.1016/j.oneear.2023.02.007, 2023.
McGrane, S. J.: Impacts of urbanisation on hydrological and water quality dynamics, and urban water management: a review, Hydrolog. Sci. J., 61, 2295–2311, https://doi.org/10.1080/02626667.2015.1128084, 2016.
McGuire, K. J., McDonnell, J. J., Weiler, M., Kendall, C., McGlynn, B. L., Welker, J. M., and Seibert, J.: The role of topography on catchment-scale water residence time, Water Resour. Res., 41, W05002, https://doi.org/10.1029/2004WR003657, 2005.
Mcleod, E., Chmura, G. L., Bouillon, S., Salm, R., Björk, M., Duarte, C. M., Lovelock, C. E., Schlesinger, W. H., and Silliman, B. R.: A blueprint for blue carbon: toward an improved understanding of the role of vegetated coastal habitats in sequestering CO2, Front. Ecol. Environ., 9, 552–560, https://doi.org/10.1890/110004, 2011.
McVicar, T. R., Van Niel, T. G., Li, L., Hutchinson, M. F., Mu, X., and Liu, Z.: Spatially distributing monthly reference evapotranspiration and pan evaporation considering topographic influences, J. Hydrol., 338, 196–220, https://doi.org/10.1016/j.jhydrol.2007.02.018, 2007.
Melton, J. R., Wania, R., Hodson, E. L., Poulter, B., Ringeval, B., Spahni, R., Bohn, T., Avis, C. A., Beerling, D. J., Chen, G., Eliseev, A. V., Denisov, S. N., Hopcroft, P. O., Lettenmaier, D. P., Riley, W. J., Singarayer, J. S., Subin, Z. M., Tian, H., Zürcher, S., Brovkin, V., van Bodegom, P. M., Kleinen, T., Yu, Z. C., and Kaplan, J. O.: Present state of global wetland extent and wetland methane modelling: conclusions from a model inter-comparison project (WETCHIMP), Biogeosciences, 10, 753–788, https://doi.org/10.5194/bg-10-753-2013, 2013.
Merritt, D. M. and Wohl, E. E.: Processes governing hydrochory along rivers: Hydraulics, hydrology and dispersal phenology, Ecol. Appl., 12, 1071–1087, https://doi.org/10.1890/1051-0761(2002)012[1071:PGHARH]2.0.CO;2, 2002.
Mertes, L. A. K.: Inland flood hazards: Human, riparian, and aquatic communities, in: Inundation hydrology, Cambridge University Press, Cambridge, UK, 145–166, 2011.
Messager, M. L., Lehner, B., Cockburn, C., Lamouroux, N., Pella, H., Snelder, T., Tockner, K., Trautmann, T., Watt, C., and Datry, T.: Global prevalence of non-perennial rivers and streams, Nature, 594, 391–397, https://doi.org/10.1038/s41586-021-03565-5, 2021.
Mikac, S., Žmegač, A., Trlin, D., Paulić, V., Oršanić, M., and Anić, I.: Drought-induced shift in tree response to climate in floodplain forests of Southeastern Europe, Sci. Rep., 8, 16495, https://doi.org/10.1038/s41598-018-34875-w, 2018.
Molins, S., Svyatsky, D., Xu, Z., Coon, E. T., and Moulton, J. D.: A multicomponent reactive transport model for integrated surface-subsurface hydrology problems, Water Resour. Res., 58, e2022WR032074, https://doi.org/10.1029/2022WR032074, 2022.
Moomaw, W. R., Chmura, G. L., Davies, G. T., Finlayson, C. M., Middleton, B. A., Natali, S. M., Perry, J. E., Roulet, N., and Sutton-Grier, A. E.: Wetlands In a changing climate: Science, policy and management, Wetlands, 38, 183–205, https://doi.org/10.1007/s13157-018-1023-8, 2018.
Morrissey, E. M. and Franklin, R. B.: Evolutionary history influences the salinity preference of bacterial taxa in wetland soils, Front. Microbiol., 6, 1013, https://doi.org/10.3389/fmicb.2015.01013, 2015.
Murray, N. J., Bunting, P., Canto, R. F., Hilarides, L., Kennedy, E. V., Lucas, R. M., Lyons, M. B., Navarro, A., Roelfsema, C. M., Rosenqvist, A., Spalding, M. D., Toor, M., and Worthington, T. A.: coastTrain: A global feference library for coastal ecosystems, Remote Sens., 14, 5766, https://doi.org/10.3390/rs14225766, 2022a.
Murray, N. J., Worthington, T. A., Bunting, P., Duce, S., Hagger, V., Lovelock, C. E., Lucas, R., Saunders, M. I., Sheaves, M., Spalding, M., Waltham, N. J., and Lyons, M. B.: High-resolution mapping of losses and gains of Earth's tidal wetlands, Science, 376, 744–749, https://doi.org/10.1126/science.abm9583, 2022b.
Nanson, G. and Croke, J.: A genetic classification of floodplains, Geomorphology, 4, 459–486, 1992.
Nardi, F., Vivoni, E. R., and Grimaldi, S.: Investigating a floodplain scaling relation using a hydrogeomorphic delineation method, Water Resour. Res., 42, https://doi.org/10.1029/2005WR004155, 2006.
Nelson, T. M., Streten, C., Gibb, K. S., and Chariton, A. A.: Saltwater intrusion history shapes the response of bacterial communities upon rehydration, Sci. Total Environ., 502, 143–148, https://doi.org/10.1016/j.scitotenv.2014.08.109, 2015.
Neubauer, S. C. and Megonigal, J. P.: Moving beyond global warming potentials to quantify the climatic role of ecosystems, Ecosystems, 18, 1000–1013, https://doi.org/10.1007/s10021-015-9879-4, 2015.
Ode, P. R., Fetscher, A. E., and Busse, L. B.: Standard operating procedures for the collection of field data for bioassessments of California wadeable streams: Benthic macroinvertebrates, algae, and physical habitat, California State Water Resources Control Board Surface Water Ambient Monitoring Program (SWAMP) Bioassessment SOP 004, SCCWRP Technical Report 835, 2016.
O'Mara, K., Olley, J. M., Fry, B., and Burford, M.: Catchment soils supply ammonium to the coastal zone – Flood impacts on nutrient flux in estuaries, Sci. Total Environ., 654, 583–592, https://doi.org/10.1016/j.scitotenv.2018.11.077, 2019.
O'Meara, T. A., Hillman, J. R., and Thrush, S. F.: Rising tides, cumulative impacts and cascading changes to estuarine ecosystem functions, Sci. Rep., 7, 10218, https://doi.org/10.1038/s41598-017-11058-7, 2017.
Orozco-López, E., Muñoz-Carpena, R., Gao, B., and Fox, G. A.: Riparian Vadose Zone preferential flow: Review of concepts, limitations, and perspectives, Vadose Zone J., 17, 180031, https://doi.org/10.2136/vzj2018.02.0031, 2018.
Palmer, M. A. and Hondula, K. L.: Restoration as mitigation: Analysis of stream mitigation for coal mining impacts in Southern Appalachia, Environ. Sci. Technol., 48, 10552–10560, https://doi.org/10.1021/es503052f, 2014.
Palmer, M. A., Hondula, K. L., and Koch, B. J.: Ecological restoration of streams and rivers: Shifting strategies and shifting goals, Annu. Rev. Ecol. Evol. Syst., 45, 247–269, https://doi.org/10.1146/annurev-ecolsys-120213-091935, 2014.
Palta, M. M., Ehrenfeld, J. G., and Groffman, P. M.: “Hotspots” and “Hot Moments” of denitrification in urban Brownfield Wetlands, Ecosystems, 17, 1121–1137, https://doi.org/10.1007/s10021-014-9778-0, 2014.
Palta, M. M., Grimm, N. B., and Groffman, P. M.: “Accidental” urban wetlands: Ecosystem functions in unexpected places, Front. Ecol. Environ., 15, 248–256, https://doi.org/10.1002/fee.1494, 2017.
Pan, J., Liu, Y., Zhong, X., Lampayan, R. M., Singleton, G. R., Huang, N., Liang, K., Peng, B., and Tian, K.: Grain yield, water productivity and nitrogen use efficiency of rice under different water management and fertilizer-N inputs in South China, Agr. Water Manag., 184, 191–200, https://doi.org/10.1016/j.agwat.2017.01.013, 2017.
Pascolini-Campbell, M., Fisher, J. B., and Reager, J. T.: GRACE-FO and ECOSTRESS synergies constrain fine-scale impacts on the water balance, Geophys. Res. Lett., 48, e2021GL093984, https://doi.org/10.1029/2021GL093984, 2021.
Patel, K. F., Tatariw, C., MacRae, J. D., Ohno, T., Nelson, S. J., and Fernandez, I. J.: Snowmelt periods as hot moments for soil N dynamics: a case study in Maine, USA, Environ. Monit. Assess., 192, 777, https://doi.org/10.1007/s10661-020-08733-0, 2020.
Patel, K. F., Rod, K. A., Zheng, J., Regier, P. J., Machado-Silva, F., Bond-Lamberty, B., Chen, X., Day, D., Doro, K. O., Kaufman, M., Kovach, M., McDowell, N., McKever, S. A., Megonigal, P. J., Norris, C. G., O’Meara, T., Rich, R., Thornton, P., Kemner, K. M., Ward, N. D., Weintraub, M. N., and Bailey, V. L.: Time to anoxia: Observations and predictions of oxygen drawdown following coastal flood events, Geoderma, 444, 116854, https://doi.org/10.1016/j.geoderma.2024.116854, 2024.
Patel, N., Gahlaud, S., Saxena, A., Thakur, B., Bharti, N., Dabhi, A., Bhushan, R., and Agnihotri, R.: Revised chronology and stable isotopic (carbon and nitrogen) characterization of Lahuradewa lake sediment (Ganga-plain, India): Insights into biogeochemistry leading to peat formation in the lake, J. Palaeontol. Soc. Ind., 67, 113–125, 2022.
Peacock, M., Audet, J., Bastviken, D., Futter, M. N., Gauci, V., Grinham, A., Harrison, J. A., Kent, M. S., Kosten, S., Lovelock, C. E., Veraart, A. J., and Evans, C. D.: Global importance of methane emissions from drainage ditches and canals, Environ. Res. Lett., 16, 044010, https://doi.org/10.1088/1748-9326/abeb36, 2021.
Pedersen, O., Sauter, M., Colmer, T. D., and Nakazono, M.: Regulation of root adaptive anatomical and morphological traits during low soil oxygen, New Phytol., 229, 42–49, https://doi.org/10.1111/nph.16375, 2021.
Pekel, J.-F., Cottam, A., Gorelick, N., and Belward, A. S.: High-resolution mapping of global surface water and its long-term changes, Nature, 540, 418–422, https://doi.org/10.1038/nature20584, 2016.
Peng, S., Lin, X., Thompson, R. L., Xi, Y., Liu, G., Hauglustaine, D., Lan, X., Poulter, B., Ramonet, M., Saunois, M., Yin, Y., Zhang, Z., Zheng, B., and Ciais, P.: Wetland emission and atmospheric sink changes explain methane growth in 2020, Nature, 612, 477–482, https://doi.org/10.1038/s41586-022-05447-w, 2022.
Perks, M. T., Russell, A. J., and Large, A. R. G.: Technical Note: Advances in flash flood monitoring using unmanned aerial vehicles (UAVs), Hydrol. Earth Syst. Sci., 20, 4005–4015, https://doi.org/10.5194/hess-20-4005-2016, 2016.
Peruccacci, S., Brunetti, M. T., Gariano, S. L., Melillo, M., Rossi, M., and Guzzetti, F.: Rainfall thresholds for possible landslide occurrence in Italy, Geomorphology, 290, 39–57, https://doi.org/10.1016/j.geomorph.2017.03.031, 2017.
Pezeshki, S. R. and DeLaune, R. D.: Soil oxidation-reduction in wetlands and Its impact on plant functioning, Biology (Basel), 1, 196–221, https://doi.org/10.3390/biology1020196, 2012.
Pickering, M. D., Horsburgh, K. J., Blundell, J. R., Hirschi, J. J.-M., Nicholls, R. J., Verlaan, M., and Wells, N. C.: The impact of future sea-level rise on the global tides, Cont. Shelf Res., 142, 50–68, https://doi.org/10.1016/j.csr.2017.02.004, 2017.
Plum, N.: Terrestrial invertebrates in flooded grassland: A literature review, Wetlands, 25, 721–737, https://doi.org/10.1672/0277-5212(2005)025[0721:TIIFGA]2.0.CO;2, 2005.
Pool, S., Francés, F., Garcia-Prats, A., Pulido-Velazquez, M., Sanchis-Ibor, C., Schirmer, M., Yang, H., and Jiménez-Martínez, J.: From flood to drip irrigation under climate change: Impacts on evapotranspiration and groundwater recharge in the mediterranean region of Valencia (Spain), Earth's Future, 9, e2020EF001859, https://doi.org/10.1029/2020EF001859, 2021.
Popper, K. R.: Conjectures and refutations: the growth of scientific knowledge, Repr. Routledge, London, 608 pp., ISBN 9780415285940, 2014.
Price, A. N., Jones, C. N., Hammond, J. C., Zimmer, M. A., and Zipper, S. C.: The drying regimes of non-perennial rivers and streams, Geophys. Res. Lett., 48, e2021GL093298, https://doi.org/10.1029/2021GL093298, 2021.
Pumo, D., Caracciolo, D., Viola, F., and Noto, L. V.: Climate change effects on the hydrological regime of small non-perennial river basins, Sci. Total Environ., 542, 76–92, https://doi.org/10.1016/j.scitotenv.2015.10.109, 2016.
Quinn, J. D., Reed, P. M., Giuliani, M., Castelletti, A., Oyler, J. W., and Nicholas, R. E.: Exploring how changing monsoonal dynamics and human pressures challenge multireservoir management for flood protection, hydropower production, and agricultural water supply, Water Resour. Res., 54, 4638–4662, https://doi.org/10.1029/2018WR022743, 2018.
Rameshwaran, P., Bell, V. A., Davies, H. N., and Kay, A. L.: How might climate change affect river flows across West Africa?, Clim. Change, 169, 1–27, https://doi.org/10.1007/s10584-021-03256-0, 2021.
Ramsar Secretariat: An Introduction to the Convention on Wetlands (previously The Ramsar Convention Manual), 7th ed., Ramsar Convention Secretariat, Gland, Switzerland, 100 pp., 2016.
Rasmussen, T. C., Deemy, J. B., and Long, S. L.: Wetland Hydrology, in: The Wetland Book, edited by: Finlayson, C. M., Everard, M., Irvine, K., McInnes, R. J., Middleton, B. A., Van Dam, A. A., and Davidson, N. C., Springer Netherlands, Dordrecht, 1–16, https://doi.org/10.1007/978-94-007-6172-8_71-1, 2016.
Regier, P., Ward, N. D., Indivero, J., Wiese Moore, C., Norwood, M., and Myers-Pigg, A.: Biogeochemical control points of connectivity between a tidal creek and its floodplain, Limnol. Oceanogr., 6, 134–142, https://doi.org/10.1002/lol2.10183, 2021.
Reichstein, M., Camps-Valls, G., Stevens, B., Jung, M., Denzler, J., Carvalhais, N., and Prabhat: Deep learning and process understanding for data-driven Earth system science, Nature, 566, 195–204, https://doi.org/10.1038/s41586-019-0912-1, 2019.
Reis, V., Hermoso, V., Hamilton, S. K., Ward, D., Fluet-Chouinard, E., Lehner, B., and Linke, S.: A global assessment of inland wetland conservation status, BioScience, 67, 523–533, https://doi.org/10.1093/biosci/bix045, 2017.
Reisinger, A. J., Groffman, P. M., and Rosi-Marshall, E. J.: Nitrogen cycling process rates across urban ecosystems, FEMS Microbiol. Ecol., 92, fiw198, https://doi.org/10.1093/femsec/fiw198, 2016.
Renwick, W., Sleezer, R., Buddemeier, R., and Smith, S.: Small artificial ponds in the United States: Impacts on sedimentation and carbon budget, in: Proceedings of the Eighth Federal Interagency Sedimentation Conference, 2–6 April 2006, Reno, NV, USA, 738–744, 2006.
Resetarits, W. J.: Oviposition site choice and life history evolution, Am. Zool., 36, 205–215, https://doi.org/10.1093/icb/36.2.205, 1996.
Reverey, F., Ganzert, L., Lischeid, G., Ulrich, A., Premke, K., and Grossart, H.-P.: Dry-wet cycles of kettle hole sediments leave a microbial and biogeochemical legacy, Sci. Total Environ., 627, 985–996, https://doi.org/10.1016/j.scitotenv.2018.01.220, 2018.
Ribolzi, O., Patin, J., Bresson, L. M., Latsachack, K. O., Mouche, E., Sengtaheuanghoung, O., Silvera, N., Thiébaux, J. P., and Valentin, C.: Impact of slope gradient on soil surface features and infiltration on steep slopes in northern Laos, Geomorphology, 127, 53–63, https://doi.org/10.1016/j.geomorph.2010.12.004, 2011.
Richardson, D. C., Holgerson, M. A., Farragher, M. J., Hoffman, K. K., King, K. B. S., Alfonso, M. B., Andersen, M. R., Cheruveil, K. S., Coleman, K. A., Farruggia, M. J., Fernandez, R. L., Hondula, K. L., López Moreira Mazacotte, G. A., Paul, K., Peierls, B. L., Rabaey, J. S., Sadro, S., Sánchez, M. L., Smyth, R. L., and Sweetman, J. N.: A functional definition to distinguish ponds from lakes and wetlands, Sci. Rep., 12, 10472, https://doi.org/10.1038/s41598-022-14569-0, 2022a.
Richardson, D. C., Holgerson, M. A., Farragher, M. J., Hoffman, K. K., King, K. B. S., Alfonso, M. B., Andersen, M. R., Cheruveil, K. S., Coleman, K. A., Farruggia, M. J., Fernandez, R. L., Hondula, K. L., López Moreira Mazacotte, G. A., Paul, K., Peierls, B. L., Rabaey, J. S., Sadro, S., Sánchez, M. L., Smyth, R. L., and Sweetman, J. N.: A functional definition to distinguish ponds from lakes and wetlands, Sci. Rep., 12, 10472, https://doi.org/10.1038/s41598-022-14569-0, 2022b.
Richey, A. S., Thomas, B. F., Lo, M., Reager, J. T., Famiglietti, J. S., Voss, K., Swenson, S., and Rodell, M.: Quantifying renewable groundwater stress with GRACE, Water Resour. Res., 51, 5217–5238, https://doi.org/10.1002/2015WR017349, 2015.
Ripley, B. J. and Simovich, M. A.: Species richness on islands in time: Variation in ephemeral pond crustacean communities in relation to habitat duration and size, Hydrobiologia, 617, 181–196, https://doi.org/10.1007/s10750-008-9548-0, 2009.
Robinson, C. T., Tockner, K., and Ward, J. V.: The fauna of dynamic riverine landscapes: Fauna of riverine landscapes, Freshwater Biol., 47, 661–677, https://doi.org/10.1046/j.1365-2427.2002.00921.x, 2002.
Rosado, J., Morais, M., and Tockner, K.: Mass dispersal of terrestrial organisms during first flush events in a temporary stream: Mass dispersal of terrestrial organisms, River Res. Appl., 31, 912–917, https://doi.org/10.1002/rra.2791, 2015.
Rosentreter, J. A., Borges, A. V., Deemer, B. R., Holgerson, M. A., Liu, S., Song, C., Melack, J., Raymond, P. A., Duarte, C. M., Allen, G. H., Olefeldt, D., Poulter, B., Battin, T. I., and Eyre, B. D.: Half of global methane emissions come from highly variable aquatic ecosystem sources, Nat. Geosci., 14, 225–230, https://doi.org/10.1038/s41561-021-00715-2, 2021.
Ruel, J. J. and Ayres, M. P.: Jensen's inequality predicts effects of environmental variation, Trends Ecol. Evol., 14, 361–366, https://doi.org/10.1016/s0169-5347(99)01664-x, 1999.
Rullens, V., Mangan, S., Stephenson, F., Clark, D. E., Bulmer, R. H., Berthelsen, A., Crawshaw, J., Gladstone-Gallagher, R. V., Thomas, S., Ellis, J. I., and Pilditch, C. A.: Understanding the consequences of sea level rise: the ecological implications of losing intertidal habitat, New Zeal. J. Mar. Fresh., 56, 353–370, https://doi.org/10.1080/00288330.2022.2086587, 2022.
Saadat, S., Frankenberger, J., Bowling, L., and Ale, S.: Evaluation of surface ponding and runoff generation in a seasonally frozen drained agricultural field, J. Hydrol., 588, 124985, https://doi.org/10.1016/j.jhydrol.2020.124985, 2020.
Saltarelli, W. A., Cunha, D. G. F., Freixa, A., Perujo, N., López-Doval, J. C., Acuña, V., and Sabater, S.: Nutrient stream attenuation is altered by the duration and frequency of flow intermittency, Ecohydrology, 15, e2351, https://doi.org/10.1002/eco.2351, 2022.
Sarremejane, R., Mykrä, H., Bonada, N., Aroviita, J., and Muotka, T.: Habitat connectivity and dispersal ability drive the assembly mechanisms of macroinvertebrate communities in river networks, Freshwater Biol., 62, 1073–1082, https://doi.org/10.1111/fwb.12926, 2017.
Sarremejane, R., Stubbington, R., England, J., Sefton, C. E. M., Eastman, M., Parry, S., and Ruhi, A.: Drought effects on invertebrate metapopulation dynamics and quasi-extinction risk in an intermittent river network, Glob. Change Biol., 27, 4024–4039, https://doi.org/10.1111/gcb.15720, 2021.
Schaffer-Smith, D., Myint, S. W., Muenich, R. L., Tong, D., and DeMeester, J. E.: Repeated hurricanes reveal risks and opportunities for social-ecological resilience to flooding and water quality problems, Environ. Sci. Technol., 54, 7194–7204, https://doi.org/10.1021/acs.est.9b07815, 2020.
Schimel, D. S., Kittel, T. G. F., and Parton, W. J.: Terrestrial biogeochemical cycles: global interactions with the atmosphere and hydrology, Tellus A, 43, 188–203, https://doi.org/10.1034/j.1600-0870.1991.00017.x, 1991.
Schimel, J. P.: Life in dry soils: Effects of drought on soil microbial communities and processes, Annu. Rev. Ecol. Evol. S., 49, 409–432, https://doi.org/10.1146/annurev-ecolsys-110617-062614, 2018.
Schlesinger, W. H. and Bernhardt, E. S.: The atmosphere, in: Biogeochemistry, Elsevier, 51–97, https://doi.org/10.1016/B978-0-12-814608-8.00003-7, 2020.
Schumann, G. J.-P. and Moller, D. K.: Microwave remote sensing of flood inundation, Phys. Chem. Earth, 83–84, 84–95, https://doi.org/10.1016/j.pce.2015.05.002, 2015.
Schuwirth, N., Borgwardt, F., Domisch, S., Friedrichs, M., Kattwinkel, M., Kneis, D., Kuemmerlen, M., Langhans, S. D., Martínez-López, J., and Vermeiren, P.: How to make ecological models useful for environmental management, Ecol. Model., 411, 108784, https://doi.org/10.1016/j.ecolmodel.2019.108784, 2019.
Semeniuk, C. and Semeniuk, V.: A comprehensive classification of inland wetlands of Western Australia using the geomorphic-hydrologic approach, Journal of the Royal Society of Western Australia, 94, 449–464, 2011.
Semeniuk, C. A. and Semeniuk, V.: A geomorphic approach to global classification for inland wetlands, in: Advances in Vegetation Science, Vegetatio, 118, 103–124, https://doi.org/10.1007/BF00045193, 1995.
Shaeri Karimi, S., Saintilan, N., Wen, L., and Cox, J.: Spatio-temporal effects of inundation and climate on vegetation greenness dynamics in dryland floodplains, Ecohydrology, 15, e2378, https://doi.org/10.1002/eco.2378, 2022.
Shanafield, M., Bourke, S. A., Zimmer, M. A., and Costigan, K. H.: An overview of the hydrology of non-perennial rivers and streams, WIREs Water, 8, e1504, https://doi.org/10.1002/wat2.1504, 2021.
Shi, X., Thornton, P. E., Ricciuto, D. M., Hanson, P. J., Mao, J., Sebestyen, S. D., Griffiths, N. A., and Bisht, G.: Representing northern peatland microtopography and hydrology within the Community Land Model, Biogeosciences, 12, 6463–6477, https://doi.org/10.5194/bg-12-6463-2015, 2015.
Shumilova, O., Zak, D., Datry, T., von Schiller, D., Corti, R., Foulquier, A., Obrador, B., Tockner, K., Allan, D. C., Altermatt, F., Arce, M. I., Arnon, S., Banas, D., Banegas-Medina, A., Beller, E., Blanchette, M. L., Blanco-Libreros, J. F., Blessing, J., Boëchat, I. G., Boersma, K., Bogan, M. T., Bonada, N., Bond, N. R., Brintrup, K., Bruder, A., Burrows, R., Cancellario, T., Carlson, S. M., Cauvy-Fraunié, S., Cid, N., Danger, M., de Freitas Terra, B., Girolamo, A. M. D., del Campo, R., Dyer, F., Elosegi, A., Faye, E., Febria, C., Figueroa, R., Four, B., Gessner, M. O., Gnohossou, P., Cerezo, R. G., Gomez-Gener, L., Graça, M. A. S., Guareschi, S., Gücker, B., Hwan, J. L., Kubheka, S., Langhans, S. D., Leigh, C., Little, C. J., Lorenz, S., Marshall, J., McIntosh, A., Mendoza-Lera, C., Meyer, E. I., Miliša, M., Mlambo, M. C., Moleón, M., Negus, P., Niyogi, D., Papatheodoulou, A., Pardo, I., Paril, P., Pešić, V., Rodriguez-Lozano, P., Rolls, R. J., Sanchez-Montoya, M. M., Savić, A., Steward, A., Stubbington, R., Taleb, A., Vorste, R. V., Waltham, N., Zoppini, A., and Zarfl, C.: Simulating rewetting events in intermittent rivers and ephemeral streams: A global analysis of leached nutrients and organic matter, Glob. Change Biol., 25, 1591–1611, https://doi.org/10.1111/gcb.14537, 2019.
Siebert, S., Portmann, F. T., and Döll, P.: Global patterns of cropland use intensity, Remote Sens., 2, 1625–1643, https://doi.org/10.3390/rs2071625, 2010.
Siev, S., Paringit, E. C., Yoshimura, C., and Hul, S.: Modelling inundation patterns and sediment dynamics in the extensive floodplain along the Tonle Sap River, River Res. Appl., 35, 1387–1401, https://doi.org/10.1002/rra.3491, 2019.
Slater, L., Villarini, G., Archfield, S., Faulkner, D., Lamb, R., Khouakhi, A., and Yin, J.: Global Changes in 20-Year, 50-Year, and 100-Year River Floods, Geophys. Res. Lett., 48, e2020GL091824, https://doi.org/10.1029/2020GL091824, 2021.
Smith, A. P., Bond-Lamberty, B., Benscoter, B. W., Tfaily, M. M., Hinkle, C. R., Liu, C., and Bailey, V. L.: Shifts in pore connectivity from precipitation versus groundwater rewetting increases soil carbon loss after drought, Nat. Commun., 8, 1335, https://doi.org/10.1038/s41467-017-01320-x, 2017.
Smith, J. A. M., Rossner, K. J., and Duran, D. P.: New opportunities for conservation of a rare tiger beetle on developed barrier island beaches, J. Insect. Conserv., 25, 733–745, https://doi.org/10.1007/s10841-021-00339-2, 2021.
Smith, K. A., Ball, T., Conen, F., Dobbie, K. E., Massheder, J., and Rey, A.: Exchange of greenhouse gases between soil and atmosphere: interactions of soil physical factors and biological processes, Eur. J. Soil Sci., 69, 10–20, https://doi.org/10.1111/ejss.12539, 2018.
Smyth, A. R., Loecke, T. D., Franz, T. E., and Burgin, A. J.: Using high-frequency soil oxygen sensors to predict greenhouse gas emissions from wetlands, Soil Biol. Biochem., 128, 182–192, https://doi.org/10.1016/j.soilbio.2018.10.020, 2019.
Song, X., Chen, X., Stegen, J., Hammond, G., Song, H., Dai, H., Graham, E., and Zachara, J. M.: Drought Conditions Maximize the Impact of High-Frequency Flow Variations on Thermal Regimes and Biogeochemical Function in the Hyporheic Zone, Water Resour. Res., 54, 7361–7382, https://doi.org/10.1029/2018WR022586, 2018.
Soupir, M. L., Mostaghimi, S., and Mitchem Jr., C. E.: A comparative study of stream-gaging techniques for low-flow measurements in two Virginia tributaries, J. Am. Water Resour. As., 45, 110–122, https://doi.org/10.1111/j.1752-1688.2008.00264.x, 2009.
Speir, S. L., Tank, J. L., and Mahl, U. H.: Quantifying denitrification following floodplain restoration via the two-stage ditch in an agricultural watershed, Ecol. Eng., 155, 105945, https://doi.org/10.1016/j.ecoleng.2020.105945, 2020.
Stallins, J. A. and Parker, A. J.: The influence of complex systems interactions on barrier island dune vegetation pattern and process, Ann. Assoc. Am. Geogr., 93, 13–29, 2003.
Stanford, J. A., Lorang, M. S., and Hauer, F. R.: The shifting habitat mosaic of river ecosystems, SIL Proceedings, 1922–2010, 29, 123–136, https://doi.org/10.1080/03680770.2005.11901979, 2005.
Stanley, E. H., Powers, S. M., Lottig, N. R., Buffam, I., and Crawford, J. T.: Contemporary changes in dissolved organic carbon (DOC) in human-dominated rivers: is there a role for DOC management?, Freshwater Biol., 57, 26–42, https://doi.org/10.1111/j.1365-2427.2011.02613.x, 2012.
Stewart, B., Shanley, J. B., Kirchner, J. W., Norris, D., Adler, T., Bristol, C., Harpold, A. A., Perdrial, J. N., Rizzo, D. M., Sterle, G., Underwood, K. L., Wen, H., and Li, L.: Streams as mirrors: Reading subsurface water chemistry from stream chemistry, Water Resour. Res., 58, e2021WR029931, https://doi.org/10.1029/2021WR029931, 2022.
Stewart, R. D., Bhaskar, A. S., Parolari, A. J., Herrmann, D. L., Jian, J., Schifman, L. A., and Shuster, W. D.: An analytical approach to ascertain saturation-excess versus infiltration-excess overland flow in urban and reference landscapes, Hydrol. Process., 33, 3349–3363, https://doi.org/10.1002/hyp.13562, 2019.
Sullivan, P., Rains, M. C., and Rodewald, A. D.: The proposed change to the definition of “waters of the United States” flouts sound science, P. Natl. Acad. Sci. USA, 116, 11558–11561, https://doi.org/10.1073/pnas.1907489116, 2019.
Sun, B., Jiang, M., Han, G., Zhang, L., Zhou, J., Bian, C., Du, Y., Yan, L., and Xia, J.: Experimental warming reduces ecosystem resistance and resilience to severe flooding in a wetland, Sci. Adv., 8, eabl9526, https://doi.org/10.1126/sciadv.abl9526, 2022a.
Sun, Z., Sandoval, L., Crystal-Ornelas, R., Mousavi, S. M., Wang, J., Lin, C., Cristea, N., Tong, D., Carande, W. H., Ma, X., Rao, Y., Bednar, J. A., Tan, A., Wang, J., Purushotham, S., Gill, T. E., Chastang, J., Howard, D., Holt, B., Gangodagamage, C., Zhao, P., Rivas, P., Chester, Z., Orduz, J., and John, A.: A review of Earth artificial intelligence, Comput. Geosci., 159, 105034, https://doi.org/10.1016/j.cageo.2022.105034, 2022b.
Svensson, J. R., Lindegarth, M., Jonsson, P. R., and Pavia, H.: Disturbance–diversity models: what do they really predict and how are they tested?, P. Roy. Soc. B, 279, 2163–2170, https://doi.org/10.1098/rspb.2011.2620, 2012.
Sweet, W., Park, J., Marra, J., Zervas, C., and Gill, S.: Sea level rise and nuisance flood frequency changes around the United States, NOAA technical report NOS CO-OPS, https://repository.library.noaa.gov/view/noaa/30823 (last access: 5 February 2025), 2014.
Swenson, L. J., Zipper, S., Peterson, D. M., Jones, C. N., Burgin, A. J., Seybold, E., Kirk, M. F., and Hatley, C.: Changes in Water Age During Dry-Down of a Non-Perennial Stream, Water Resour. Res., 60, e2023WR034623, https://doi.org/10.1029/2023WR034623, 2024.
Tagestad, J., Ward, N. D., Butman, D., and Stegen, J.: Small streams dominate US tidal reaches and will be disproportionately impacted by sea-level rise, Sci. Total Environ., 753, 141944, https://doi.org/10.1016/j.scitotenv.2020.141944, 2021.
Tai, X., Anderegg, W. R. L., Blanken, P. D., Burns, S. P., Christensen, L., and Brooks, P. D.: Hillslope hydrology influences the spatial and temporal patterns of remotely sensed ecosystem productivity, Water Resour. Res., 56, e2020WR027630, https://doi.org/10.1029/2020WR027630, 2020.
Thomas, M. A., Mirus, B. B., and Smith, J. B.: Hillslopes in humid-tropical climates aren't always wet: Implications for hydrologic response and landslide initiation in Puerto Rico, Hydrol. Process., 34, 4307–4318, https://doi.org/10.1002/hyp.13885, 2020.
Tiner, R. W.: Tidal wetlands primer: An introduction to their ecology, natural history, status, and conservation, University of Massachusetts Press, Amherst, 560 pp., ISBN-10 1625340222, 2013.
Tiner, R. W.: Wetland indicators: A guide to wetland identification, delineation, classification, and mapping, Second edition, Taylor & Francis, Boca Raton, https://doi.org/10.1201/9781315374710, 2016.
Trochim, E. D., Prakash, A., Kane, D. L., and Romanovsky, V. E.: Remote sensing of water tracks, Earth Space Sci., 3, 106–122, https://doi.org/10.1002/2015EA000112, 2016.
Tromp-van Meerveld, H. J. and McDonnell, J. J.: Threshold relations in subsurface stormflow: 2. The fill and spill hypothesis: Threshold flow relations, Water Resour. Res., 42, W02411, https://doi.org/10.1029/2004WR003800, 2006.
Tsoi, W., Growns, I., Southwell, M., Mika, S., Lewis, S., Ryder, D., and Frazier, P.: Effects of inundation on water quality and invertebrates in semiarid floodplain wetlands, Inland Waters, 12, 397–406, https://doi.org/10.1080/20442041.2022.2057164, 2022.
Tweedley, J.: The contrasting ecology of temperate macrotidal and microtidal estuaries, Oceanography and Marine Biology, 1st Edition, CRC Press, 100 pp., ISBN 9781315368597, 2016.
U.S. Geological Survey: Cottonwood Lake Study Area – Aerial Imagery: U.S. Geological Survey data release, https://doi.org/10.5066/F7DZ06GR, 2017.
US Army Corps of Engineers: Definitions of Terms https://www.nap.usace.army.mil/Missions/Regulatory/Definitions/, last access: 14 August 2024.
Valett, H. M., Baker, M. A., Morrice, J. A., Crawford, C. S., Molles Jr., M. C., Dahm, C. N., Moyer, D. L., Thibault, J. R., and Ellis, L. M.: Biogeochemical and metabolic responses to the flood pulse in a semiarid floodplain, Ecology, 86, 220–234, https://doi.org/10.1890/03-4091, 2005.
Van Appledorn, M., De Jager, N. R., and Rohweder, J. J.: Quantifying and mapping inundation regimes within a large river-floodplain ecosystem for ecological and management applications, River Res. Appl., 37, 241–255, https://doi.org/10.1002/rra.3628, 2021.
Van Meerveld, H. J. I., Sauquet, E., Gallart, F., Sefton, C., Seibert, J., and Bishop, K.: Aqua temporaria incognita, Hydrol. Process., 34, 5704–5711, https://doi.org/10.1002/hyp.13979, 2020.
VanZomeren, C. M., Berkowitz, J. F., Piercy, C. D., and White, J. R.: Restoring a degraded marsh using thin layer sediment placement: Short term effects on soil physical and biogeochemical properties, Ecol. Eng., 120, 61–67, https://doi.org/10.1016/j.ecoleng.2018.05.012, 2018.
Venterink, H. O., Pieterse, N. M., Belgers, J. D. M., Wassen, M. J., and De Ruiter, P. C.: N, P, and K budgets along nutrient availability and productivity gradients in wetlands, Ecol. Appl., 12, 1010–1026, https://doi.org/10.1890/1051-0761(2002)012[1010:NPAKBA]2.0.CO;2, 2002.
Vitousek, S., Barnard, P. L., Fletcher, C. H., Frazer, N., Erikson, L., and Storlazzi, C. D.: Doubling of coastal flooding frequency within decades due to sea-level rise, Sci. Rep., 7, 1399, https://doi.org/10.1038/s41598-017-01362-7, 2017.
Vorste, R. V., Corti, R., Sagouis, A., and Datry, T.: Invertebrate communities in gravel-bed, braided rivers are highly resilient to flow intermittence, Freshwater Sci., 35, 164–177, https://doi.org/10.1086/683274, 2016.
von Schiller, D., Datry, T., Corti, R., Foulquier, A., Tockner, K., Marcé, R., García-Baquero, G., Odriozola, I., Obrador, B., Elosegi, A., Mendoza-Lera, C., Gessner, M. O., Stubbington, R., Albariño, R., Allen, D. C., Altermatt, F., Arce, M. I., Arnon, S., Banas, D., Banegas-Medina, A., Beller, E., Blanchette, M. L., Blanco-Libreros, J. F., Blessing, J., Boëchat, I. G., Boersma, K. S., Bogan, M. T., Bonada, N., Bond, N. R., Brintrup, K., Bruder, A., Burrows, R. M., Cancellario, T., Carlson, S. M., Cauvy-Fraunié, S., Cid, N., Danger, M., de Freitas Terra, B., Dehedin, A., De Girolamo, A. M., del Campo, R., Díaz-Villanueva, V., Duerdoth, C. P., Dyer, F., Faye, E., Febria, C., Figueroa, R., Four, B., Gafny, S., Gómez, R., Gómez-Gener, L., Graça, M. a. S., Guareschi, S., Gücker, B., Hoppeler, F., Hwan, J. L., Kubheka, S., Laini, A., Langhans, S. D., Leigh, C., Little, C. J., Lorenz, S., Marshall, J., Martín, E. J., McIntosh, A., Meyer, E. I., Miliša, M., Mlambo, M. C., Moleón, M., Morais, M., Negus, P., Niyogi, D., Papatheodoulou, A., Pardo, I., Pařil, P., Pešić, V., Piscart, C., Polášek, M., Rodríguez-Lozano, P., Rolls, R. J., Sánchez-Montoya, M. M., Savić, A., Shumilova, O., Steward, A., Taleb, A., Uzan, A., Vander Vorste, R., Waltham, N., Woelfle-Erskine, C., Zak, D., Zarfl, C., and Zoppini, A.: Sediment respiration pulses in intermittent rivers and ephemeral streams, Global Biogeochem. Cy., 33, 1251–1263, https://doi.org/10.1029/2019GB006276, 2019.
Vousdoukas, M. I., Voukouvalas, E., Mentaschi, L., Dottori, F., Giardino, A., Bouziotas, D., Bianchi, A., Salamon, P., and Feyen, L.: Developments in large-scale coastal flood hazard mapping, Nat. Hazards Earth Syst. Sci., 16, 1841–1853, https://doi.org/10.5194/nhess-16-1841-2016, 2016.
Waltham, N. J. and Connolly, R. M.: Global extent and distribution of artificial, residential waterways in estuaries, Estuar. Coast. Shelf Sci., 94, 192–197, https://doi.org/10.1016/j.ecss.2011.06.003, 2011.
Wang, X., Wang, W., and Tong, C.: A review on impact of typhoons and hurricanes on coastal wetland ecosystems, Acta Ecologica Sinica, 36, 23–29, https://doi.org/10.1016/j.chnaes.2015.12.006, 2016.
Wantzen, K., Alves, C., Badiane, S., Bala, R., Blettler, M., Callisto, M., Cao, Y., Kolb, M., Kondolf, G., Leite, M., Macedo, D., Mahdi, O., Neves, M., Peralta, M., Rotgé, V., Rueda-Delgado, G., Scharager, A., Serra-Llobet, A., Yengué, J.-L., and Zingraff-Hamed, A.: Urban stream and wetland restoration in the Global South–A DPSIR analysis, Sustainability, 11, 4975, https://doi.org/10.3390/su11184975, 2019.
Ward, J. V., Tockner, K., and Schiemer, F.: Biodiversity of floodplain river ecosystems: ecotones and connectivity1, Regul. Rivers Res. Mgmt., 15, 125–139, https://doi.org/10.1002/(SICI)1099-1646(199901/06)15:1/3<125::AID-RRR523>3.0.CO;2-E, 1999.
Ward, J. V., Tockner, K., Arscott, D. B., and Claret, C.: Riverine landscape diversity, Freshwater Biol., 47, 517–539, https://doi.org/10.1046/j.1365-2427.2002.00893.x, 2002.
Ward, N. D., Megonigal, J. P., Bond-Lamberty, B., Bailey, V. L., Butman, D., Canuel, E. A., Diefenderfer, H., Ganju, N. K., Goñi, M. A., Graham, E. B., Hopkinson, C. S., Khangaonkar, T., Langley, J. A., McDowell, N. G., Myers-Pigg, A. N., Neumann, R. B., Osburn, C. L., Price, R. M., Rowland, J., Sengupta, A., Simard, M., Thornton, P. E., Tzortziou, M., Vargas, R., Weisenhorn, P. B., and Windham-Myers, L.: Representing the function and sensitivity of coastal interfaces in Earth system models, Nat. Commun., 11, 2458, https://doi.org/10.1038/s41467-020-16236-2, 2020.
Watts, J. D., Kimball, J. S., Bartsch, A., and McDonald, K. C.: Surface water inundation in the boreal-Arctic: potential impacts on regional methane emissions, Environ. Res. Lett., 9, 075001, https://doi.org/10.1088/1748-9326/9/7/075001, 2014.
Weird Bristol [WeirdBristol]: “With a difference of 15-metres/49-foot between high and low tide, the River Avon has the second largest tidal range in the world. Only the Bay of Fundy in Canada has a higher tide, with an average of 16.8 metres/55-foot, #Bristol”, Twitter https://twitter.com/WeirdBristol/status/1015732213730758658 (last access: 5 February 2025), 7 July 2018.
Wen, H., Perdrial, J., Abbott, B. W., Bernal, S., Dupas, R., Godsey, S. E., Harpold, A., Rizzo, D., Underwood, K., Adler, T., Sterle, G., and Li, L.: Temperature controls production but hydrology regulates export of dissolved organic carbon at the catchment scale, Hydrol. Earth Syst. Sci., 24, 945–966, https://doi.org/10.5194/hess-24-945-2020, 2020.
Weyman, D. R.: Measurements of the downslope flow of water in a soil, J. Hydrol., 20, 267–288, https://doi.org/10.1016/0022-1694(73)90065-6, 1973.
Whitworth, K. L., Kerr, J. L., Mosley, L. M., Conallin, J., Hardwick, L., and Baldwin, D. S.: Options for managing hypoxic blackwater in river systems: case studies and framework, Environ. Manage., 52, 837–850, https://doi.org/10.1007/s00267-013-0130-9, 2013.
Wierzbicki, G., Ostrowski, P., and Falkowski, T.: Applying floodplain geomorphology to flood management (The Lower Vistula River upstream from Plock, Poland), Open Geosci., 12, 1003–1016, https://doi.org/10.1515/geo-2020-0102, 2020.
Williams, D. D.: The biology of temporary waters, Oxford University Press, Oxford, New York, 337 pp., https://doi.org/10.1093/acprof:oso/9780198528128.001.0001, 2006.
Wittenberg, H.: Baseflow recession and recharge as nonlinear storage processes, Hydrol. Process., 13, 715–726, https://doi.org/10.1002/(SICI)1099-1085(19990415)13:5<715::AID-HYP775>3.0.CO;2-N, 1999.
Wohl, E.: An integrative conceptualization of floodplain storage, Rev. Geophys., 59, e2020RG000724, https://doi.org/10.1029/2020RG000724, 2021.
Wollheim, W. M., Harms, T. K., Robison, A. L., Koenig, L. E., Helton, A. M., Song, C., Bowden, W. B., and Finlay, J. C.: Superlinear scaling of riverine biogeochemical function with watershed size, Nat. Commun., 13, 1230, https://doi.org/10.1038/s41467-022-28630-z, 2022.
Wu, B., Tian, F., Nabil, M., Bofana, J., Lu, Y., Elnashar, A., Beyene, A. N., Zhang, M., Zeng, H., and Zhu, W.: Mapping global maximum irrigation extent at 30m resolution using the irrigation performances under drought stress, Glob. Environ. Change, 79, 102652, https://doi.org/10.1016/j.gloenvcha.2023.102652, 2023.
Wu, R., Chen, X., Hammond, G., Bisht, G., Song, X., Huang, M., Niu, G.-Y., and Ferre, T.: Coupling surface flow with high-performance subsurface reactive flow and transport code PFLOTRAN, Environ. Model. Softw., 137, 104959, https://doi.org/10.1016/j.envsoft.2021.104959, 2021.
Xiao, D., Shi, Y., Brantley, S. L., Forsythe, B., DiBiase, R., Davis, K., and Li, L.: Streamflow generation from catchments of contrasting lithologies: The role of soil properties, topography, and catchment size, Water Resour. Res., 55, 9234–9257, https://doi.org/10.1029/2018WR023736, 2019.
Xie, D., Schwarz, C., Brückner, M. Z. M., Kleinhans, M. G., Urrego, D. H., Zhou, Z., and Van Maanen, B.: Mangrove diversity loss under sea-level rise triggered by bio-morphodynamic feedbacks and anthropogenic pressures, Environ. Res. Lett., 15, 114033, https://doi.org/10.1088/1748-9326/abc122, 2020.
Xin, P., Wilson, A., Shen, C., Ge, Z., Moffett, K. B., Santos, I. R., Chen, X., Xu, X., Yau, Y. Y. Y., Moore, W., Li, L., and Barry, D. A.: Surface water and groundwater interactions in salt marshes and their impact on plant ecology and coastal biogeochemistry, Rev. Geophys., 60, e2021RG000740, https://doi.org/10.1029/2021RG000740, 2022.
Zedler, P. H.: Vernal pools and the concept of “isolated wetlands”, Wetlands, 23, 597–607, https://doi.org/10.1672/0277-5212(2003)023[0597:VPATCO]2.0.CO;2, 2003.
Zhang, Y. S., Cioffi, W. R., Cope, R., Daleo, P., Heywood, E., Hoyt, C., Smith, C. S., and Silliman, B. R.: A Global Synthesis Reveals Gaps in Coastal Habitat Restoration Research, Sustainability, 10, 1040, https://doi.org/10.3390/su10041040, 2018.
Zhang, Z., Zimmermann, N. E., Stenke, A., Li, X., Hodson, E. L., Zhu, G., Huang, C., and Poulter, B.: Emerging role of wetland methane emissions in driving 21st century climate change, P. Natl. Acad. Sci. USA, 114, 9647–9652, https://doi.org/10.1073/pnas.1618765114, 2017.
Zhang, Z., Fluet-Chouinard, E., Jensen, K., McDonald, K., Hugelius, G., Gumbricht, T., Carroll, M., Prigent, C., Bartsch, A., and Poulter, B.: Development of the global dataset of Wetland Area and Dynamics for Methane Modeling (WAD2M) , Earth Syst. Sci. Data, 13, 2001–2023, https://doi.org/10.5194/essd-13-2001-2021, 2021.
Zhao, Y., Wang, X., Jiang, S., Xiao, J., Li, J., Zhou, X., Liu, H., Hao, Z., and Wang, K.: Soil development mediates precipitation control on plant productivity and diversity in alpine grasslands, Geoderma, 412, 115721, https://doi.org/10.1016/j.geoderma.2022.115721, 2022.
Zhi, W. and Li, L.: The shallow and deep hypothesis: Subsurface vertical chemical contrasts shape nitrate export patterns from different land uses, Environ. Sci. Technol., 54, 11915–11928, https://doi.org/10.1021/acs.est.0c01340, 2020.
Zimmer, M. A. and McGlynn, B. L.: Ephemeral and intermittent runoff generation processes in a low relief, highly weathered catchment, Water Resour. Res., 53, 7055–7077, https://doi.org/10.1002/2016WR019742, 2017.
Zimmer, M. A., Kaiser, K. E., Blaszczak, J. R., Zipper, S. C., Hammond, J. C., Fritz, K. M., Costigan, K. H., Hosen, J., Godsey, S. E., Allen, G. H., Kampf, S., Burrows, R. M., Krabbenhoft, C. A., Dodds, W., Hale, R., Olden, J. D., Shanafield, M., DelVecchia, A. G., Ward, A. S., Mims, M. C., Datry, T., Bogan, M. T., Boersma, K. S., Busch, M. H., Jones, C. N., Burgin, A. J., and Allen, D. C.: Zero or not? Causes and consequences of zero-flow stream gage readings, WIREs Water, 7, e1436, https://doi.org/10.1002/wat2.1436, 2020.
Zimmer, M. A., Burgin, A. J., Kaiser, K., and Hosen, J.: The unknown biogeochemical impacts of drying rivers and streams, Nat. Commun., 13, 7213, https://doi.org/10.1038/s41467-022-34903-4, 2022.
Zipper, S. C., Hammond, J. C., Shanafield, M., Zimmer, M., Datry, T., Jones, C. N., Kaiser, K. E., Godsey, S. E., Burrows, R. M., Blaszczak, J. R., Busch, M. H., Price, A. N., Boersma, K. S., Ward, A. S., Costigan, K., Allen, G. H., Krabbenhoft, C. A., Dodds, W. K., Mims, M. C., Olden, J. D., Kampf, S. K., Burgin, A. J., and Allen, D. C.: Pervasive changes in stream intermittency across the United States, Environ. Res. Lett., 16, 084033, https://doi.org/10.1088/1748-9326/ac14ec, 2021.
- Abstract
- Introduction
- Divergent drivers, common responses, and VIE mini-reviews
- Inundation processes are relevant at the scale of the beholder
- Summary of primary methods used to study VIEs
- Towards cross-VIE transferable understanding
- Data availability
- Author contributions
- Competing interests
- Disclaimer
- Acknowledgements
- Financial support
- Review statement
- References
- Abstract
- Introduction
- Divergent drivers, common responses, and VIE mini-reviews
- Inundation processes are relevant at the scale of the beholder
- Summary of primary methods used to study VIEs
- Towards cross-VIE transferable understanding
- Data availability
- Author contributions
- Competing interests
- Disclaimer
- Acknowledgements
- Financial support
- Review statement
- References