the Creative Commons Attribution 4.0 License.
the Creative Commons Attribution 4.0 License.
Calcification in a marginal sea – influence of seawater [Ca2+] and carbonate chemistry on bivalve shell formation
Jörn Thomsen
Kirti Ramesh
Trystan Sanders
Markus Bleich
Frank Melzner
In estuarine coastal systems such as the Baltic Sea, mussels suffer from low salinity which limits their distribution. Anthropogenic climate change is expected to cause further desalination which will lead to local extinctions of mussels in the low saline areas. It is commonly accepted that mussel distribution is limited by osmotic stress. However, along the salinity gradient, environmental conditions for biomineralization are successively becoming more adverse as a result of reduced [Ca2+] and dissolved inorganic carbon (CT) availability. In larvae, calcification is an essential process starting during early development with formation of the prodissoconch I (PD I) shell, which is completed under optimal conditions within 2 days.
Experimental manipulations of seawater [Ca2+] start to impair PD I formation in Mytilus larvae at concentrations below 3 mM, which corresponds to conditions present in the Baltic at salinities below 8 g kg−1. In addition, lowering dissolved inorganic carbon to critical concentrations (< 1 mM) similarly affected PD I size, which was well correlated with calculated ΩAragonite and [Ca2+][HCO] ∕ [H+] in all treatments. Comparing results for larvae from the western Baltic with a population from the central Baltic revealed a significantly higher tolerance of PD I formation to lowered [Ca2+] and [Ca2+][HCO] ∕ [H+] in the low saline adapted population. This may result from genetic adaptation to the more adverse environmental conditions prevailing in the low saline areas of the Baltic.
The combined effects of lowered [Ca2+] and adverse carbonate chemistry represent major limiting factors for bivalve calcification and can thereby contribute to distribution limits of mussels in the Baltic Sea.
- Article
(1977 KB) - Full-text XML
- BibTeX
- EndNote
Salinity is one of the most important environmental parameters limiting the distribution of aquatic species. One the one hand, many marine organisms exhibit little tolerance to reduced salinity and are thus not able to thrive in brackish water environments influenced by riverine inputs (Whitfield et al., 2012). On the other hand, some animals, such as bivalves and crustaceans, tolerate the dilution of the ambient seawater and are able to inhabit brackish estuarine water habitats (Westerbom et al., 2002). However, within these habitats, organisms need to tolerate a number of environmental stressors which are changing concomitantly.
Generally, lowered ambient ion concentrations affect an organism's ability to maintain cellular homeostasis. In response, some organisms such as crustaceans actively regulate the ionic composition of their extracellular fluids. However, mytilid mussels do not control haemolymph osmolarity and ionic composition mostly corresponds to that of ambient seawater (Thomsen et al., 2010). Thus tissues are subjected to a diluted medium in brackish water but the inorganic composition of the intracellular space needs to be regulated in order to maintain enzymatic functions. At moderately lowered salinity, intracellular [K+] and [Na+] are kept relatively stable at about 200 and 100 mM, respectively, but [K+] drops rapidly under strong hypoosmotic stress to avoid cell swelling (Willmer, 1978; Wright et al., 1989; Silva and Wright, 1994). In order to stay isoosmotic with their environment following long-term acclimation to lowered salinity, intracellular [K+] and [Na+] are maintained at lower concentrations (Willmer, 1978; Natochin et al., 1979). In addition, bivalves reduce the concentration of intracellular compatible organic osmolytes, such as certain amino acids, taurine and betaine during the acclimation phase (Silva and Wright, 1994; Kube et al., 2006). However, at a certain critical salinity threshold (Scrit), the intracellular organic osmolyte pools are depleted, which has been suggested to eventually limit species fitness (Kube et al., 2006; Podbielski et al., 2016).
At the same time, bivalves produce an external shell composed of CaCO3 and an organic matrix (Falini et al., 1996). The shell enables adult bivalves to live in intertidal habitats and is an effective protection against predation, but shell formation has been shown to be sensitive to lowered salinity (Malone and Dodd, 1967). Under favourable environmental conditions, calcification begins already in early development and the first larval shell (prodissoconch I, PD I) is completed within the first 48 h after fertilization. PD I formation is an important prerequisite for the successful development of bivalve larvae as larvae seem to commence feeding only after completion of the shell, which provides structural support (e.g. muscle attachment site) for the functional velum (Lucas and Rangel, 1983; Cragg, 1985). However, PD I formation is highly sensitive to chemical and environmental stressors (Williams and Hall, 1999) and initiation of feeding is delayed under adverse carbonate chemistry (Waldbusser et al., 2015).
Recently, a number of studies investigated how changes in seawater carbonate chemistry affect marine calcifiers. Those studies were mostly motivated by the ongoing input of anthropogenic CO2 into the oceans that results in a drop of pH and lowered [CO], a process called ocean acidification. Bivalve shell formation is highly sensitive to modifications of carbonate chemistry and therefore negatively affected by ocean acidification (Gazeau et al., 2013; Waldbusser et al., 2014; Thomsen et al., 2015). The exact reason for the sensitivity of calcification to adverse carbonate chemistry is still under debate (Cyronak et al., 2015). Lowered saturation of seawater with respect to calcium carbonate (Ω, [Ca2+][CO] ∕ K*sp; with K*sp being the stoichiometric solubility product; Mucci, 1983) could affect the kinetics of shell formation (according to , with r being mineral precipitation rate, k being the rate constant and n being the reaction order; Waldbusser et al., 2014) and undersaturation leads to dissolution of existing calcium carbonate structures (Thomsen et al., 2010; Melzner et al., 2011; Haynert et al., 2014). Alternatively, the substrate inhibitor ratio (SIR) defined as the availability of the substrate for calcification in the form of dissolved inorganic carbon (CT) or HCO and the inhibitory effect of lowered seawater pH (increased [H+]) could restrict calcification rates (Bach, 2015; Thomsen et al., 2015; Fassbender et al., 2016).
Independent of the exact mode of action, larval bivalve calcification is driven by uptake of seawater Ca2+ and inorganic carbon (CT), whereas metabolic carbon is only of minor importance and contributes to less than 10 % in larvae and adults (McConnaughey and Gillikin, 2008; Waldbusser et al., 2015). Oceanic [Ca2+] is about 10 mM, but necessarily linearly related with seawater salinity and thus reduced in estuaries. Freshwater [Ca2+] is in general much lower (< 1–2 mM [Ca2+]; Ohlson and Anderson, 1990; Juhna and Klavins, 2001). Oceanic CT is about 2 mM whereby HCO and CO contribute about 90 and 8 % to the CT pool, respectively. CT of seawater equilibrated with the atmosphere is directly proportional to salinity as it is depending on seawater total alkalinity (AT). Therefore, calcifiers are facing abiotic conditions in brackish water habitats that most likely affect their ability to form a shell.
The Baltic Sea is an example of a brackish water habitat which is substantially influenced by precipitation and riverine input (Gustafsson et al., 2014), which results in a salinity gradient from 25 g kg−1 in the Kattegat transition zone to basically freshwater in the gulfs of Riga, Finland and Bothnia. As a consequence, [Ca2+], AT and CT decline linearly along the salinity gradient (Kremling and Wilhelm, 1997; Beldowski et al., 2010). However, varying the composition of riverine freshwater results in differing AT–salinity correlations and in the Gulf of Riga, AT and thus CT even increases with lowered salinity (Beldowski et al., 2010).
The Baltic Sea is among the coastal ecosystems which are most heavily influenced by anthropogenic activity. Eutrophication enhanced hypoxia or even anoxia events in the benthic ecosystem. As respiratory oxygen consumption is coupled to CO2 production, hypoxia is always accompanied by a pronounced increase in pCO2 and thus affects the carbonate system simultaneously (Melzner et al., 2013). Furthermore, climate change is expected to increase precipitation in the Baltic catchment area, which may cause increased riverine runoff leading to reduced salinity (0–45 % reduction) in particular in the northeastern and central Baltic Sea (Meier et al., 2006; Gräwe et al., 2013). This shift in salinity will most likely induce a substantial retreat of the marine fauna and flora and expansion of limnic species into the formerly brackish water habitats (Johannesson et al., 2011).
Mytilid mussels (Mytilus spp.) are among the most abundant organisms of the Baltic Sea (1013 individuals) contributing up to 90 % to local hard bottom biomass, and thus are important habitat builders (Enderlein and Wahl, 2004; Johannesson et al., 2011). Their distribution along the Finnish, Swedish and Estonian coasts is limited by salinities of about 4.5 g kg−1 when abundance, biomass and growth drastically decline (Westerbom et al., 2002; Martin et al., 2013; Riisgard et al., 2014). As growth combines both somatic growth and shell formation, it is unclear which physiological mechanism exactly limits performance and therefore the distribution of mussels (Riisgard et al., 2014).
Currently, distribution limits of marine bivalves in estuaries are commonly related to the inability of intracellular osmoregulatory adjustment at lowered salinity (Maar et al., 2015). However, as [Ca2+] and CT availability decline along the Baltic Sea salinity gradient it is likely that the calcification process is negatively affected as well. This process has not been previously considered as a factor contributing to distribution limits of mussels. In this study, we investigated the effects of seawater [Ca2+] independently of salinity in combination with lowered CT availability on the calcification performance of larval Mytilus spp. and correlated the experimental data with environmental conditions present in the Baltic Sea.
2.1 Animal collection and spawning
Adult mussels were collected from subtidal depths at the pier of GEOMAR in Kiel Fjord (shell length: 4–6 cm; 54∘19.8′ N, 010∘09.0′ E) and at the wooden groynes close to Koserow on the island of Usedom (shell length: 2–3 cm; 54∘03.4′ N, 014∘00.4′ E) between May and June 2016 (Fig. 1). Median salinity for Kiel Fjord and Usedom, located ∼ 350 km east of Kiel, are ∼ 17 and 7 g kg−1, respectively (Table 1).
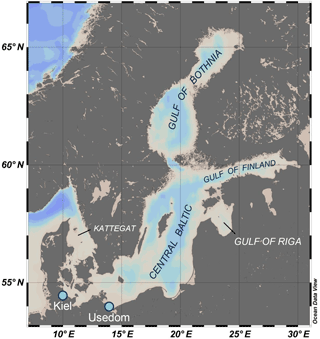
Figure 1Bathymetric map of the Baltic Sea and its subregions which are characterized by specific carbonate chemistry. Sampling spots for mussel populations used in the experiments are indicated by light blue dots.
Mussels in the Baltic Sea represent hybrids of Mytilus edulis and trossulus with increasing trossulus allele frequency towards the less saline eastern Baltic (Stuckas et al., 2017). Thus mussels collected in Kiel represent the Baltic M. edulis-like and animals from Usedom belong to the M. trossulus-like genotype (Stuckas et al., 2017).
Specimens were either used for spawning immediately after collection or kept in cold storage (9 ∘C) in order to delay gonad maturation for up to 3 months. Stored mussels (ca. 500 g mussel wet biomass per 20 L tank, 12 tanks) were fed 6 times a week with 500 mL of Rhodomonas solution (ca. 2 × 106 cells mL−1) supplemented with a commercial bivalve diet (Acuinuga, Spain) and water was exchanged twice a week (Thomsen et al., 2010). Rhodomonas spp. were cultured in PES (Provasoli-enriched seawater) medium as described previously with the exception of using 40 L cylinders (Thomsen et al., 2010).
All experiments were performed at 17 ∘C. Spawning was induced by exposing the animals to rapidly elevated water temperatures between 18–25 ∘C using heaters. Spawning specimens were separated from the remaining animals and eggs and sperms were collected individually in beakers filled with 0.2 µm filtered seawater (FSW). Subsequently, eggs were pooled and fertilized with a pooled sperm solution. For the Kiel population, 5 individual experimental runs were performed with varying number of dams and sires used for crossings in each run. In total 16 dams and 18 sires were used. For the Usedom population 1 run with 4 replicates was performed for which gonads from 5 dams and 4 sires were pooled. Fertilization success was determined by verifying the presence of a polar body and first and second cell division of zygotes and was above 90 % in all runs. Embryos (4–8 cell stage) and non-calcified trochophora (in one experimental run of the Kiel population) from all parents were transferred in equal numbers into the experimental units (volume: 25 or 50 mL in round plastic beakers) at a density of 10 embryos or larvae per millilitre.
Three days post fertilization, animals were removed from the experimental units by filtering the full water volume through a filter with a mesh size of 20 µm or by collecting larvae individually using a pipette in treatments with low survival. Subsequently, larvae were fixed using 40 % paraformaldehyde (PFA, pH 8.0) resulting in a final PFA concentration of 4 %.
Pictures of larvae were taken using a stereo microscope (Leica M165 FC) equipped with a Leica DFC 310 FX camera and LAS V4.2 software. Calcification was assessed by measuring the larval shell length. PD I shell length was assessed using Image J 1.50i by measuring the maximal shell length in parallel to the hinge or the maximal shell diameter for larvae that had not developed a complete PD I shell.
2.2 Experimental manipulation of seawater [Ca2+] and carbonate chemistry
Artificial seawater (ASW) was prepared according to Kester (1967) for salinities of 14 and 7 g kg−1 for experiments with M. edulis-like and trossulus-like, respectively, by adding NaCl, NaSO4, KCl, NaHCO3, KBr, H3BO3, MgCl2, CaCl2 and SrCl2 to deionized water. Ca2+ free artificial seawater (CFSW) was prepared by omitting CaCl2 and adjusting osmolarity similar to ASW by increasing NaCl concentrations. pHNBS was adjusted to 8.0 using NaOH. All experimental treatments comprised 5 % of 0.2 µm filtered seawater (FSW) from Kiel Fjord which was adjusted to salinity 7 g kg−1 for the Usedom population experiment to ensure that trace elements were present. The comparison of shell sizes of larvae kept in control ASW + 5 % FSW or 100 % FSW yielded no significant differences (p > 0.05). Varying seawater [Ca2+] treatments were prepared by mixing ASW and CFSW (lowered [Ca2+]) or by addition of CaCl2 from a 500 mM stock solution to ASW (elevated [Ca2+]). Following mixing, water samples were taken and seawater [Ca2+] was measured using a flame photometer (EFOX 5053, Eppendorf, Germany) calibrated with urine standards (Biorapid GmbH, Germany).
Table 2Experimental conditions during larval experiments, N: 1–10 determinations, ΩAragonite and [Ca2+][HCO] ∕ [H+] are calculated from measured [Ca2+], CT and pHNBS.
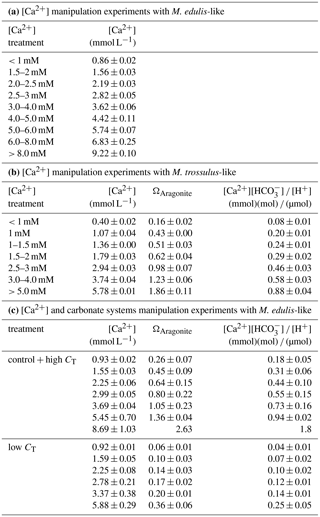
Seawater carbonate chemistry was manipulated by increasing alkalinity by addition of [NaHCO3] to ASW or by lowering alkalinity by adding 1M HCl to the experimental units. Excess CO2 was removed by aeration of the experimental units for 30 min and embryos were only added after pH had increased again to stable values (∼ 7.8). Seawater pH was determined on the NBS scale (National Bureau of Standards) using a WTW 3310 pH meter equipped with a Sentix 81 electrode. Seawater CT was determined using an AIRICA CO2 analyzer and verified by measuring a certified reference material (Dickson et al., 2003). Seawater carbonate system parameters (HCO, CO, Ωaragonite) were calculated using the CO2SYS program with KHSO4, K1 and K2 dissociation constants after Dickson et al. (1990) and Roy et al. (1993), respectively. pHNBS was converted to total scale pH. Ωaragonite and [Ca2+][HCO] ∕ [H+] were linearly adjusted according to measured seawater [Ca2+] (Table 2).
2.3 Microelectrode measurements of [Ca2+] in the calcifying space of D-stage veliger
Using ion-selective electrodes, Ca2+ gradients were measured in seawater and in the calcification space (CS) below the surface of the shell in veliger larvae 3 days after fertilization. The experimental set-up and hardware was identical to that of Stumpp et al. (2013), except for the addition of a metal plate connected to a water cooling system for temperature control.
Borosilicate glass capillary tubes (inner diameter 1.2 mm, outer diameter, 1.5 mm) with filament were pulled on a DMZ-Universal puller (Zeitz Instruments, Germany) to micropipettes with tip diameters of 1–3 µm. Micropipettes were silanized with dimethyl chlorosilane (Sigma-Aldrich, USA) in an oven at 200 ∘C for 1 h. Calcium-sensitive liquid ion exchangers (LIX) and LIX-PVC membranes were prepared according to de Beer et al. (2000) with Ca2+ ionophore II (Sigma-Aldrich). The microelectrodes were back filled with a KCl-based electrolyte (200 mM KCl, 2 mM CaCl2.2H2O) and thereafter front loaded with LIX and finally LIX-PVC at a length of 150 and 50 µm, respectively. To measure calcium in the CS, larvae were placed into the temperature controlled perfusion chamber mounted on an inverted microscope (Axiovert 135, Zeiss, Germany) at a density of 100 mL−1 and were held in position using a holding pipette. The ion-selective probe was mounted on a remote-controlled micro-manipulator and was introduced beneath the shell from the side of the growing edge, where stable measurements were obtained within 5–10 s. Microelectrode calibration was verified by measuring [Ca2+] of seawater standards as described above and analogue outputs were channelled through an amplifier (WPI Instruments, USA) to a chart recorder (Gould Instruments, USA).
2.4 Seawater [Ca2+] and carbonate chemistry of the Baltic Sea
Seawater [Ca2+] (mM kg−1) was calculated for salinities between 3 and 20 g kg−1 using the correlation for chlorinities < 4.5 and > 4.5 g kg−1 provided by Kremling and Wilhelm (1997) and a salinity–chlorinity conversion after Millero (1984). [Ca2+] was calculated for salinity values measured in Kiel Fjord (N=4250, weekly measurements 2005–2009, 0–18 m; 54∘19.8′ N, 10∘9.0′ E; Clemmesen et al., unpublished data; Casties et al., 2015) and at the Oder Bank (N=260 000, hourly measurements, 2000–2015, 3 + 12 m water depths; 54∘4.6′ N, 14∘9.6′ E; ∼ 8 km off the M. trossulus-like collection site at Usedom (BSH, 2000–2015, Table 1). As the distribution of mytilid bivalves is limited by salinities below 4.5 g kg−1 the calculation covers the full [Ca2+] range relevant for mussels in this estuary (Westerbom et al., 2002). Carbonate chemistry calculations are based on the salinity–alkalinity correlation published by Beldowski et al. (2010) for salinities between 3 and 20 g kg−1 and a seawater surface pCO2 of 400 µatm assuming equilibrium with current atmospheric CO2 concentrations of ∼ 400 ppm. Calculations were performed for seawater temperatures of 15 ∘C, which corresponds to average conditions experienced by larvae during the natural reproductive period from April to June. The Baltic Sea has four sub-areas which are differentially impacted by the inflow of riverine freshwater and their respective chemical properties: the central Baltic Sea with the Kattegat transition area, the Gulf of Riga, the Gulf of Finland and the Bothnian Sea with Gulf of Bothnia. Depending on the chemical properties of the riverine input, seawater carbonate chemistry can differ substantially for similar salinity values between the four regions. The same calculations were performed for predicting future conditions using atmospheric CO2 concentration of 800 ppm.
2.5 Statistical analysis
All statistical analyses (t test, Kruskal–Wallis test followed by Dunn's test, regression analysis, linear and nonlinear model parameter fitting) were performed using R and the mosaic package. Population comparisons were performed by fitting linear models for log transformed data. Each experimental unit was considered as a replicate. Values in text and figures are replicate means ± standard error.
Table 3Model parameters (a, b, c) describing PD I size as a function of experimental seawater conditions for Mytilus edulis-like and trossulus-like: shell length (µm) = .
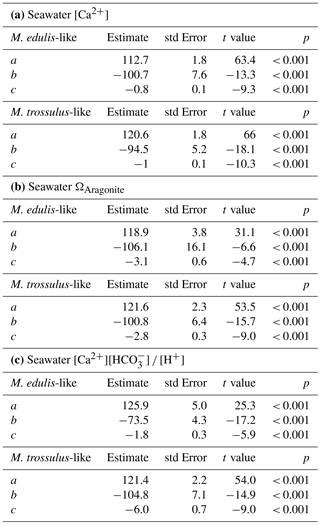
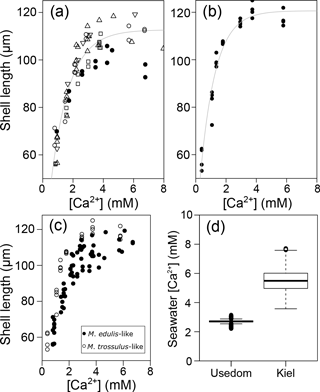
Figure 2Prodissoconch I length of mussel larvae as a function of seawater [Ca2+]. (a) M. edulis-like, different symbols represent different experimental runs (1–5), (b) M. trossulus-like, (c) comparison of M. edulis-like and trossulus-like and (d) box plots of seawater [Ca2+] at the collection site in Kiel Fjord and at Usedom depicting median, 25 and 75 % percentiles and outliers.
3.1 PD I shell formation and CS [Ca2+] under varying seawater [Ca2+]
Larval development until PD I formation was investigated for M. edulis-like collected in Kiel Fjord. The lowest seawater [Ca2+] tested in the experiment was 0.51 mM, which did not allow successful development of larvae to the trochophore stage in the Kiel population and was thus not considered in subsequent experiments. At all other [Ca2+] treatments, early development was not adversely affected and larvae started to calcify prodissoconch I. However, at [Ca2+] of < 2 mM larvae were not able to produce a complete PD I shell. Even after 7 days, shell size did not increase above a mean diameter of 63.7 ± 6.0 µm although larvae stayed viable and continued to actively swim. In all other treatments, shells were fully developed within 72 h post fertilization, but shell length declined linearly at [Ca2+] below 3 mM ranging between 104.5 ± 2.1 µm at 2.8 mM and 82.1 ± 1.5 µm at 1.6 mM, with significant reductions below 2.5 mM [Ca2+] (H: 50.3, p<0.001, Dunn's test). Specimens kept at control [Ca2+] of 4–5 mM had mean lengths of 108.2 ± 2.5 µm. Modifications of seawater [Ca2+] in the range 4–10 mM had only minor impacts on lengths and elevated [Ca2+] did not cause a further increase in shell lengths above control size (Fig. 2a, Table 3a).
Table 4Results for linear models fitted on log transformed data of shell length and seawater parameters, significant results in bold. CHH is [Ca2+][HCO]∕[H+].
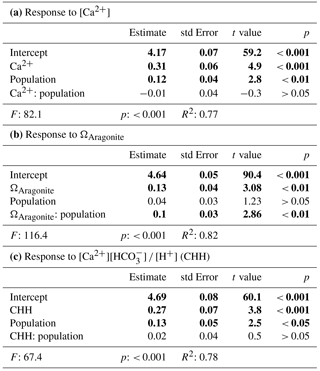
Results for shell formation rates of M. edulis-like larvae were compared with the M. trossulus-like population from Usedom. Larvae were exposed to [Ca2+] between 0.4 and 5.8 mM (Fig. 2b, c). Overall, the response curve for M. trossulus-like was similar to M. edulis-like (Table 3b). Maximal shell sizes observed at 3.7 mM were 120 ± 1.5 µm and shell lengths started to decline at lower [Ca2+]. Nevertheless, at comparable [Ca2+] shell sizes were larger compared to M. edulis-like and larvae were able to calcify a full PD I even at 1.1 mM [Ca2+] with an average size of 81.9 ± 3.2 µm. In contrast, PD I formation was not completed at 0.4 mM, yet larvae started to calcify. A linear model of the calcification response revealed a significant effect of [Ca2+] and population on shell size but no interaction (Table 4a, Fig. 2c).
Microelectrode measurements of [Ca2+] in the CS of M. edulis-like revealed that CS [Ca2+] drops with seawater [Ca2+], (H: 21.2, p<0.01, Fig. 3a). However, larvae kept at 3.5 mM [Ca2+] (above the critical [Ca2+] threshold) are characterized by CS [Ca2+] of 0.1 ± 0.01 mM above seawater concentrations (paired t test: t=16.9, p<0.01, Fig. 3b). In larvae raised at 2.6 and 2.3 mM [Ca2+], the difference between seawater and CS [Ca2+] declined to 0.06 ± 0.03 and 0.03 ± 0.02 mM, which was not significantly enriched compared to the ambient seawater. In contrast, the gradient between CS and seawater increased to 0.28 ± 0.02 mM in larvae grown at 1.5 mM.
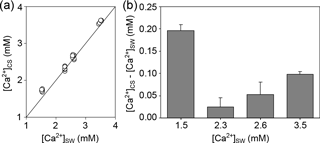
Figure 3[Ca2+] in the calcifying space (CS) of M. edulis-like larvae reared under control conditions (3.5 mM) and lower seawater [Ca2+]. (a) CS [Ca2+] as a function of seawater [Ca2+], the line indicates the isoline. (b) Difference between CS [Ca2+] and seawater [Ca2+] at four [Ca2+] treatments expressed as [Ca2+]CS-[Ca2+]SW. Bar chart depicts mean ± standard error of the mean (N=6).
3.2 Combined effects of seawater [Ca2+] and carbonate chemistry on larval calcification
M. edulis-like larvae were exposed to a range of seawater [Ca2+] between 1 and 10 mM and CT concentrations between 880–3520 µM. PD I size was not modulated by increased seawater CT of 2900–3520 µM compared to control conditions (CT: 1773 µM) and shell length was only negatively affected by seawater [Ca2+] below 3 mM (Fig. 4a). In contrast, lowered seawater CT (975 µM) significantly affected shell formation and PD I length declined to 72.5 ± 2.7 µm at control [Ca2+]. Within these treatments shell length was marginally positively correlated with seawater [Ca2+] but shell length remained reduced in all [Ca2+] treatments (linear regression: 63 (±2.2) µm + 2.9 (±0.7) × [Ca2+], F: 18.6, p<0.01, R2=0.47, Fig. 4a).
However, the correlation of shell length against [Ca2+] under reduced CT differed significantly from the three higher CT treatments. Plotting PD I sizes against seawater ΩAragonite and [Ca2+][HCO] ∕ [H+] revealed a similar correlation of calcification in all treatments (Fig. 4b, c). Calcification of larvae started to decline at ΩAragonite below 1 with significant reductions in the treatments with ΩAragonite below 0.5 (H: 44.5, p<0.001, Dunn's test). Similarly, PD I size declined at [Ca2+][HCO] ∕ [H+] values below 0.7 and shells were significantly smaller below 0.3 (H: 42.5, p<0.01, Dunn' test). In addition, the shell formation responses of M. edulis-like and M. trossulus-like to combined manipulations of [Ca2+] and carbonate chemistry were more similar compared to the effects of lowered seawater [Ca2+] alone (Figs. 2c, 4b, c; Table 3b, c). Nevertheless, whereas the response to ΩAragonite was similar for both hybrid populations, they differed significantly in their response to [Ca2+][HCO] ∕ [H+] (Table 4c, d).
3.3 Calculation of seawater [Ca2+], Ω and [Ca2+][HCO] ∕ [H+] for the Baltic Sea
Calculations of seawater [Ca2+] were performed for the salinity range observed at the collections sites of M. edulis-like and trossulus-like in Kiel Fjord and Usedom, respectively. In Kiel Fjord, salinity fluctuated substantially between 10.5 and 24.7 g kg−1 in the period 2005–2009 which resulted in simultaneous strong variations in seawater [Ca2+] between 3.6 and 7.7 mM with a mean of 5.6 mM (Table 1, Fig. 1d). In contrast, salinity in Usedom was lower with mean salinity of 7.1 g kg−1 and, in absolute numbers, more stable (3.4–9.1 g kg−1, Table 1). Thus, seawater [Ca2+] in Usedom was ranging between 1.5 and 3.2 mM with an average of 2.7 mM (Table 1, Fig. 2d).
Calculation of [Ca2+] along the Baltic salinity gradient revealed that the critical concentrations of 3 and 2.5 mM at which calcification is negatively affected are reached at a salinity of about 7–8 g kg−1, respectively, in all four subregions (Fig. 5a). In contrast, calculated values for [HCO] ∕ [H+] are above 0.13 in almost all regions within the distribution range of mussels as long as the seawater is in equilibrium with current atmospheric CO2 concentrations (Fig. 5b). Only in the Gulf of Bothnia are critical values lower than 0.1 observed for salinities of 4.5 g kg−1 and below. For ΩAragonite, undersaturation is observed at a salinity of 9 g kg−1 for the central Baltic. The gulfs of Bothnia and Finland are always undersaturated for ΩAragonite, but the Gulf of Riga seawater is supersaturated (Fig. 5c), and strong negative effects on larval calcification can be expected for salinities of about 5 g kg−1. Similarly, critical values for [Ca2+][HCO] ∕ [H+] of 0.3 at which PD I formation is significantly affected are reached at a salinity of 5 g kg−1 in most regions of the Baltic excluding the Gulf of Riga (Fig. 5d).
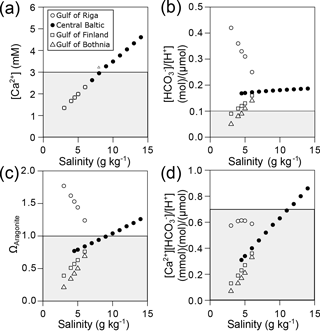
Figure 5Environmental parameters relevant for calcification in the Baltic Sea calculated for current salinity–AT correlations and atmospheric CO2 concentration (400 ppm). (a) [Ca2+], (b) [HCO] ∕ [H+], (c) ΩAragonite and (d) [Ca2+][HCO] ∕ [H+] plotted against salinity for the four subregions of the Baltic Sea. Grey areas indicate conditions of incipient reduction of larval calcification rates.
Conditions for calcification will become more adverse in the future as atmospheric CO2 concentrations are going to reach 800 ppm. In this scenario, critical values for [HCO] ∕ [H+] will be observed in most areas of the Baltic at salinities below 10 g kg−1 (Fig. 6b). In particular, [Ca2+][HCO] ∕ [H+] and ΩAragonite will be below the critical threshold in all areas of the Baltic Sea (Fig. 6c, d).
This study investigated the impact of modifications of seawater [Ca2+] and carbonate chemistry on shell formation of bivalve larvae. The experimental results were compared to the environmental conditions prevailing in the Baltic Sea.
The laboratory experiments revealed that seawater [Ca2+] is a critical factor for shell formation in marine bivalves. Similarly, Ca2+ deposition into the shells of Crassostrea gigas larvae following PD I formation was similar at seawater [Ca2+] of 10 and 16.8 mM but reduced by 40 % at 6.1 mM (Maeda-Martinez, 1987). Thus, where high oceanic [Ca2+] of ∼ 10 mM is not limiting bivalve calcification, the low concentrations present in estuaries, such as the Baltic, significantly affect biomineralization.
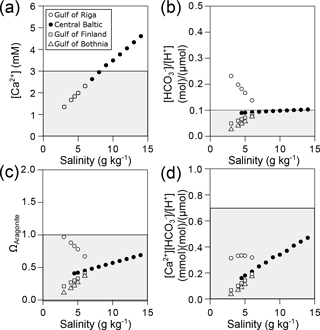
Figure 6Predicted environmental parameters relevant for calcification in the Baltic Sea calculated for current salinity–AT correlations and future atmospheric CO2 concentration (800 ppm). (a) [Ca2+], (b) [HCO] ∕ [H+], (c) ΩAragonite and (d) [Ca2+][HCO] ∕ [H+] plotted against salinity for the four subregions of the Baltic Sea. Grey areas indicate conditions of incipient reduction of larval calcification rates.
In both tested populations, M. edulis-like and M. trossulus-like, the overall response curve was similar and both populations become calcium limited at [Ca2+] below 3 mM. M. trossulus-like appeared to be slightly more tolerant to lowered [Ca2+] as larvae maintained larger PD I lengths at similar [Ca2+] and PD I formation was successfully accomplished at 1.1 mM. The response matches seawater [Ca2+] observed in the respective habitats of the tested populations and may result from either phenotypic plasticity or genetic adaptation. High plasticity of PD I formation has been observed in transgenerational experiments when parental animals were pre-exposed to elevated pCO2 (Thomsen et al., 2017a). Alternatively, it is possible that M. edulis-like living in the western brackish Baltic may have already adapted to lower [Ca2+] compared to populations and species living in habitats characterized by higher [Ca2+] (Maeda-Martinez, 1987). Gene flow between both tested populations is limited since larval drift does not allow direct exchange (Stuckas et al., 2017). As PD I formation is a crucial but sensitive stage during larval life, impaired calcification by low [Ca2+] can have significant effects on larval performance and fitness. As the distribution of bivalves is depending on successful larval dispersal, low [Ca2+] can be an important factor which determines the distribution limits of mussels and represents a strong selective force. Additionally, the strong [Ca2+] gradient observed between the western Baltic–Kattegat transition zone and the central Baltic Sea can be one explanation for the simultaneously observed allele frequency shift from M. edulis-like to trossulus-like (Larsson et al., 2017; Stuckas et al., 2017).
Nevertheless, larval shell formation of Baltic mytilids starts to become Ca2+-limited at concentrations of about 3 mM and was significantly affected at 2.5 mM. Consequently, in areas of the Baltic with salinities below 7–8 g kg−1 and corresponding [Ca2+] < 3 mM, reduced shell formation starts to compromise overall larval performance. At moderately lowered [Ca2+] > 2 mM, shells were not malformed but calcification was only slowed down by a few hours and larvae completed normal D-shell formation at a later age. At the critical salinity of 4.5 g kg−1, which delineates the distribution boundary of mussels in the Baltic (Westerbom et al., 2002), [Ca2+] is as low as 1.8 mM whereby concentration below 2 mM substantially impaired PD I formation in our experiments and did not allow production of a normal PD I. Importantly, even under these adverse conditions larvae were viable and continued active swimming for up to 7 days. Thus impaired calcification in low [Ca2+] seawater can result from two mechanisms acting independently or in combination: (i) continuous dissolution of existing calcium carbonate crystals under highly corrosive conditions may prevent further net calcification or (ii) larvae only use a pre-determined fraction of the energy stored in the egg for calcification. If this amount is not sufficient to sustain full PD I formation under low [Ca2+] the budget does not seem to be adjusted to provide additional energy to complete calcification. Instead larvae do not continue calcification and may switch to an energy saving mode to stay alive. In our experiments, M. trossulus-like apparently developed a higher tolerance to low [Ca2+] compared to M. edulis-like but incipient impairment of calcification at about 3 mM was similar in both populations, which suggests relatively conserved [Ca2+] transport mechanisms in both populations.
The impact of external [Ca2+] on calcification has previously been studied mostly in corals for which a significant correlation was observed in a number of studies (e.g. Chalker, 1976; Ip and Krishnaveni, 1991). Whereas cytosolic calcium concentrations are tightly regulated and kept constantly low, calcifiers obviously developed a mechanism to accumulate high [Ca2+] in specialized compartments within or outside the cell for biomineralization. In corals, Ca2+ uptake and transport to the site of calcification is driven by a combination of diffusive and active transport and involves active transport by plasma membrane Ca2+-ATPase (PMCA; Tambutte et al., 1996; Barott et al., 2015). In bivalves, calcification is performed by the outer mantle epithelium or the shell field in adults and larvae, respectively (Kniprath, 1980), and a PMCA homolog has been localized in the outer mantle epithelium of oysters and its inhibition negatively impacted shell growth in freshwater clams, which might suggest a conserved function in bivalve calcification as well (Wang et al., 2008; Zhao et al., 2016).
Early studies suggested that the extrapallial fluid (EPF) of bivalves provides the microhabitat for calcification (Crenshaw, 1972). However, [Ca2+] and the acid–base status of bulk EPF of adult mussels corresponds to seawater and haemolymph conditions, respectively, which supports excretion of CO2 via passive diffusion into the ambient seawater (Thomsen et al., 2010; Heinemann et al., 2012). In M. edulis-like larvae, kept above the critical threshold of 3 mM, CS [Ca2+] was marginally but significantly elevated compared to seawater [Ca2+]. At lowered environmental [Ca2+] between 2 and 3 mM CS [Ca2+] was not significantly enriched compared to seawater concentration. At these seawater [Ca2+], calcification rates were significantly reduced but larvae were still able to produce a smaller but complete PD I. At even lower ambient [Ca2+] of 1.5 mM, CS [Ca2+] was again significantly elevated compared to seawater which was accompanied by strongly reduced PD I formation. The incapacity of larvae to maintain transmembrane Ca2+ transport at lowered [Ca2+] potentially indicates a significant contribution of diffusion or involvement of a low affinity Ca2+ transporter (e.g. Na+/Ca2+ Exchanger) in this process (Blaustein and Lederer, 1999). Thus, larvae may actively enrich CS [Ca2+] to increase ΩAragonite and support the structural integrity of the shell under corrosive conditions. Alternatively, CS [Ca2+] only increased secondarily as a result of drastically reduced calcification rates caused by negative effects of low seawater [Ca2+] on larval physiology.
In addition to the sole effects of low Ca2+ availability, studies documented an influence of varying Mg : Ca ratio on calcification for a range of marine organisms independent of absolute [Ca2+] (reviewed in Ries, 2010). Considering 20–25 mM [Mg2+] present in the brackish Baltic Sea, the Mg : Ca ratio in the experimental [Ca2+] treatments varied between about 2 and 20. Consequently not only Ca2+ limitation but also adverse Mg : Ca ratios may have had an effect on PD I formation. Future research needs to perform manipulations of both ions under controlled conditions in order to investigate potential synergistic effects on larval calcification.
In the present study, the effect of lowered [Ca2+] was most pronounced under conditions when seawater carbonate chemistry was not a limiting parameter for calcification. Lowering of seawater CT, which has a similar effect on ΩAragonite and [HCO] ∕ [H+] as acidification, significantly affects the rate of PD I formation. Under these CT ∕ HCO limiting conditions, seawater [Ca2+] had only a minor, yet slightly positive, linear effect on shell formation. Presumably the effect was smaller as Ca2+ uptake was not any longer the only rate-limiting process but rather HCO uptake and/or H+ extrusion (Bach, 2015) or impaired kinetics of crystal formation (Waldbusser et al., 2014).
Importantly, the applied experimental seawater manipulations of calcium and carbonate chemistry can be integrated by calculation of ΩAragonite or extending the SIR term to [Ca2+][HCO] ∕ [H+], which also takes lowered availability of [Ca2+] into account (Bach, 2015; Fassbender et al., 2016). Plotting shell length against these two parameters revealed a similar response for all manipulations independent of whether they were manipulated by lowered [Ca2+] or CT. The correlation of calcification with these parameters corresponded to previously observed shell formation performance of mussels and oysters resulting from modifications of seawater carbonate chemistry only (Waldbusser et al., 2014, 2015; Thomsen et al., 2015). As salinity and temperature were not changed in the experiments performed with M. edulis-like ΩAragonite and [Ca2+][HCO] ∕ [H+] are linearly correlated and it is not possible to distinguish whether shell formation is modified by the changed kinetics of crystal formation (Waldbusser et al., 2015), higher dissolution due to undersaturation of the EPF with respect to calcium carbonate (Miller et al., 2009; Thomsen et al., 2010; Melzner et al., 2011; Frieder et al., 2017) or by lowered substrate availability and impaired H+ removal from the calcifying fluids (Thomsen et al., 2015; Bach, 2015; Fassbender et al., 2016). However, the calcification response of M. trossulus-like was similar to M. edulis-like when plotted against ΩAragonite, but differed significantly for [Ca2+][HCO] ∕ [H+] in accordance with the higher tolerance to lowered [Ca2+]. This could indicate local adaptation of M. trossulus-like to the adverse environment in the low saline areas of the Baltic. In contrast, the response to ΩAragonite was similar in animals from both populations, which may indicate that shell dissolution under corrosive conditions impacts net shell formation to the same extent.
Our experimental data revealed that larval calcification is substantially compromised by environmental conditions encountered in the Baltic Sea. Calculation of Baltic seawater [Ca2+] suggests [Ca2+] limitation of calcification at salinities of about 8 g kg−1. Thus, with the exception of the western Baltic Sea with its higher salinity values, mussels inhabiting most areas of the Baltic suffer from low Ca2+ availability. Interestingly, studies measuring Baltic Sea [Ca2+] revealed increasing concentrations over the last decades, which may have a beneficial effect on calcification for a given salinity (Kremling and Wilhelm, 1997). Nevertheless, the expected overall reduction of salinity will most likely exceed the minor positive effect of [Ca2+] enrichment and negatively affect overall fitness by osmotic stress and secondarily calcification (Gräwe et al., 2013).
In contrast to [Ca2+], estimating current carbonate chemistry for the four Baltic subregions suggests that the influence is of less importance for limitation of calcification. The calculated [HCO] ∕ [H+] and ΩAragonite for seawater in equilibrium with current atmospheric CO2 concentrations remain above the critical thresholds of 0.1–0.13 and 1, respectively (Thomsen et al., 2015, this study). However, this conclusion does not consider the substantial variability in carbonate chemistry in the surface water of the Baltic, which is modified by biogeochemical processes such as riverine composition, photosynthesis and upwelling on a seasonal and spatial scale. Seawater carbonate chemistry can be substantially modified by phytoplankton blooms in spring and early summer causing a draw down of seawater pCO2 to 150 µatm thereby causing elevated pH, [CO] and [HCO] ∕ [H+] for several weeks (Schneider and Kuss, 2004). Consequently, larvae can be exposed to environmental conditions which are beneficial for calcification. In contrast, local upwelling phenomena have the opposite effect leading to lowered pH and [CO], [HCO] ∕ [H+] and elevated pCO2 (Thomsen et al., 2010; Saderne et al., 2013). Upwelling events are common in the Baltic Sea in particular along the western coastlines (Myrberg and Andrejev, 2003). However, research mostly focused on the effect of upwelling on temperature and nutrient supply but neglected the local impacts on carbonate chemistry (e.g. Haapala, 1994). As upwelling causes rapid elevation of pCO2 within a short period of hours but can last for several days to a few weeks, thus for a significant part of a larval life time, its impact on calcification and performance of larvae can be substantial (Barton et al., 2012; Thomsen et al., 2015, 2017a).
In addition to the present carbonate system variability, the successive increase in atmospheric CO2 concentrations and coupled pH decline in the Baltic will result in progressively adverse conditions for calcification. This process is particularly critical for mussel populations inhabiting the low saline areas of the Baltic where conditions for calcification are less favourable already today and will become more adverse in the future. Nevertheless, it has recently been shown that increasing AT (from an unaccounted source) may partly and even completely compensate the negative effects of CO2 uptake (Müller et al., 2016). Consequently, bivalve calcification may benefit from higher AT and thus favourable carbonate chemistry in future, but lowered salinity might still affect performance.
Both substrates relevant for calcification, Ca2+ and inorganic carbon are integrated in the terms Ω and the SIR extended to [Ca2+][HCO] ∕ [H+]. In fact the calcification response of bivalve larvae in our experiments was accurately described by both terms for a given salinity and temperature. Nevertheless, calculations of the environmental conditions in the four Baltic subregions revealed important differences. ΩAragonite remains favourable for calcification (> 1) in most parts of the central Baltic and in the Gulf of Riga caused by high alkaline riverine runoff and therefore prohibits dissolution of shell crystals (Juhna and Klavins, 2001). In contrast, calculated values for [Ca2+][HCO] ∕ [H+] are below the critical threshold of 0.7 in all subregions at a salinity of 11 g kg−1 caused by low [Ca2+]. Thus, it is of high ecological relevance whether bivalve calcification is sensitive to the reduced kinetics of shell formation and dissolution depending on Ω or lowered substrate availability and inhibition by [H+]. According to our experimental data a combination of both parameters is most likely determining sensitivity. However, compared to M. edulis-like, M. trossulus-like seems to have evolved a slightly higher tolerance to low [Ca2+][HCO] ∕ [H+], but not to low ΩAragonite. A similar response has been observed in a comparison between Baltic and North Sea mussels under simulated ocean acidification (Thomsen et al., 2017a).
In conclusion, this study reveals strong impacts of lowered [Ca2+] and carbonate chemistry, which are naturally changing along the Baltic salinity gradient, on the early calcification of mussel larvae. Strong delays and impairment of complete shell formation most likely affect the energy budget and overall physiology of mussels in the low saline areas. Consequently, low [Ca2+] and adverse carbonate chemistry impact mussel fitness substantially and therefore likely seem to contribute significantly to determining the distribution of marine mussels in estuarine environment such as the Baltic Sea.
All data are available from the following link https://doi.org/10.1594/PANGAEA.871804 (Thomsen et al., 2017b).
JT conceived the study and led the writing of the manuscript; JT, KR, TS and FM collected data; JT, KR, MB and FM analysed the data. All authors contributed to the various manuscript drafts.
The authors declare that they have no conflict of interest.
The authors thank Thomas Stegmann for performing Ca2+ measurements,
Marian Hu for supporting Ca2+-microelectrode measurements and
Ulrike Panknin for maintaining Rhodomonas cultures. Furthermore,
Detlev Machoczek and Rainer Kiko are acknowledged for providing and
supporting processing of Oder Bank salinity data, respectively. This study
was funded by the BMBF program BIOACID subproject 2.3 and CACHE, a Marie
Curie Initial Training Network (ITN) funded by the People Programme (Marie
Curie Actions) of the European Union's Seventh Framework Programme
FP7/2007-2013/ under REA grant agreement no. [605051]13.
The article processing charges for this open-access
publication were covered by a Research
Centre
of the Helmholtz Association.
Edited by:
Lennart de Nooijer
Reviewed by: two anonymous referees
Bach, L. T.: Reconsidering the role of carbonate ion concentration in calcification by marine organisms, Biogeosciences, 12, 4939–4951, https://doi.org/10.5194/bg-12-4939-2015, 2015.
Barott, K. L., Perez, S. O., Linsmayer, L. B., and Tresguerres, M.: Differential localization of ion transporters suggests distinct cellular mechanisms for calcification and photosynthesis between two coral species, Am. J. Physiol.-Reg. I., 309, R235–R246, 2015.
Barton, A., Hales, B., Waldbusser, G. G., Langdon, C., and Felly, R. A.: The Pacific oyster Crassostrea gigas, shows negative correlation to naturally elevated carbon dioxide levels: Implications for near-term ocean acidification effects, Limnol. Oceanogr., 57, 698–710, 2012.
Beldowski, J., Löffler, A., and Joensuu, L.: Distribution and biogeochemical control of total CO2 and total alkalinity in the Baltic Sea, J. Marine Syst., 81, 252–259, 2010.
Blaustein, M. P. and Lederer, W. J.: Sodium/Calcium Exchange: Its physiological implications, Physiol. Rev., 79, 763–854, 1999.
BSH: Hourly meteorological observations at Station Oder Bank 2000–2015, Bundesamt für Seeschiffahrt und Hydrographie, Hamburg, 2000–2015.
Casties, I., Clemmensen, C., Melzner, F., and Thomsen, J.: Salinity dependence of recruitment success of the sea star Asterias rubens in the brackish western Baltic Sea, Helgoland Mar. Res., 69, 169–175, 2015.
Chalker, B. E.: Calcium transport during skeletogenesis in hermatypiccorals, Comp. Biochem. Phys. A, 54, 455–459, 1976.
Cragg, S. M.: The adductor and retractor muscles of the veliger of Pecten maximus (L.) (Bivalvia), J. Mollus. Stud., 51, 276–283, 1985.
Crenshaw, M. A.: The inorganic composition of molluscan extrapallial fluid, Biol. Bull., 143, 506–512, 1972.
Cyronak, T., Schulz, K. G., and Jokiel, P. L.: The Omega myth: what really drives lower calcification rates in an acidifying ocean, ICES J. Mar. Sci., 73, 558–562, 2015.
De Beer, D., Kühl, M., Stambler, N., and Vaki, L.: A mirosensor study of light enhanced Ca2+ uptake and photosynthesis in the reef-building hematypic coral Favia sp., Mar. Ecol.-Prog. Ser., 194, 75–85, 2000.
Dickson, A. G.: Standard potential of the reaction – AgClS + 1/2 H2 = AgS + HClAq and the standard acidity constant of the ion HSO4 – in synthetic sea-water from 273.15-K to 318.15-K, J. Chem. Thermodyn., 22, 113–127, 1990.
Dickson, A. G., Afghan, J. D., and Anderson, G. G.: Reference materials for oceanic CO2 analysis: A method for the certification of total alkalinity, Mar. Chem., 80, 185–197, 2003.
Enderlein, P. and Wahl, M.: Dominance of blue mussels versus consumer-mediated enhancement of benthic diversity, J. Sea Res., 51, 145–155, 2004.
Falini, G., Albeck, S., Weiner, S., and Addadi, L.: Control of aragonite or calcite polymorphism by mollusk shell macromolecules, Science, 271, 67–69, 1996.
Fassbender, A. J., Sabine, C. L., and Feifel, K. M.: Consideration of coastal carbonate chemistry in understanding biological calcification, Geophys. Res. Lett., 43, 4467–4476, 2016.
Frieder, C. A., Applebaum, S. L., Pan, T. C. F., Hedgecock, D., and Manahan, D.: Metabolic cost of calcification in bivalve larvae under experimental ocean acidification, ICES J. Mar. Sci., 74, 941–954, 2017.
Gazeau, F., Parker, L. M., Comeau, S., Gattuso, J. P., O`Connor, W. A., Martin, S., Pörtner, H. O., and Ross, P. M.: Impacts of ocean acidification on marine shelled molluscs, Mar. Biol., 160, 2207–2245, 2013.
Gräwe, U., Freidland, R., and Burchard, H.: The future of the western Baltic Sea: two possible scenarios, Ocean Dynam., 63, 901–921, 2013.
Gustafsson, E., Wällstedt, T., Humborg, C., Mörth, C. M., and Gustafsson, B. G.: External total alkalinity loads versus internal generation: The influence of nonriverine alkalinity sources in the Baltic Sea, Global Biochem. Cy., 28, 1358–1370, 2014.
Haapala, J.: Upwelling and its influence on nutrient concentration in the coastal area of the Hanko Peninsula, Entrance of the Gulf of Finland, Estuar. Coast. Shelf S., 38, 507–521, 1994.
Haynert, K., Schönfeld, J., Schiebel, R., Wilson, B., and Thomsen, J.: Response of benthic foraminifera to ocean acidification in their natural sediment environment: a long-term culturing experiment, Biogeosciences, 11, 1581–1597, https://doi.org/10.5194/bg-11-1581-2014, 2014.
Heinemann, A., Fietzke, J., Melzner, F., Böhm, F., Thomsen, J., Garbe-Schönberg, D., and Eisenhauer, A.: Conditions of Mytilus edulis extracellular body fluids and shell composition in a pH-treatment experiment: Acid-base status, trace elements and δ11B, Geochem. Geophy. Geosy., 13, Q01005, https://doi.org/10.1029/2011GC003790, 2012.
Ip, Y. K. and Krishnaveni, P.: Incorporation of Strontium (90Sr2+) into the skeleton of the hermatypic coral Galaxea fascicularis, J. Exp. Zool., 258, 273–276, 1991.
Johannesson, K., Smolarz, K., Grahn, M., and Andre, C.: The Future of Baltic Sea Populations: Local Extinction or Evolutionary Rescue?, AMBIO, 40, 179–190, 2011.
Juhna, T. and Klavins, M.: Water-quality changes in Latvian and Riga 1980–2000: Possibilities and Problems, AMBIO, 30, 306–314, 2001.
Kester, D. R., Duedall, I. W., Connors, D. N., and Pytkowicz, R. M.: Preparation of artificial seawater, Limnol. Oceanogr., 12, 176–179, 1967.
Kniprath, E.: Larval development of the shell and the shell gland in Mytilus (Bivalvia), Roux. Arch. Dev. Biol., 188, 201–204, 1980.
Kremling, K. and Wilhelm, G.: Recent increase of the calcium concentrations in Baltic Sea waters, Mar. Pollut. Bull., 34, 763–767, 1997.
Kube, S., Gerber, A., Jansen, J. M., and Schiedek, D.: Patterns of organic osmolytes in two marine bivalves, Macoma baltica, and Mytilus spp., along their European distribution, Mar. Biol., 149, 1387–1396, 2006.
Larsson, J., Lind, E. E., Corell, H., Smolarz, K., and Lönn, M.: Regional genetic differentiation in the blue mussel from the Baltic Sea, Estuar. Coast. Shelf S., 295, 98–109, 2017.
Lucas, A. and Rangel, C.: Detection of the first larval feeding in Crassostrea gigas using the epifluorescence microscope, Aquaculture, 30, 369–374, 1983.
Maar, M., Saurel, C., Landes, A., Dolmer, P., and Petersen, J. K.: Growth potential of blue mussels (M. edulis) exposed to different salinities evaluated by a Dynamic Energy Budget model, J. Marine Syst., 148, 48–55, 2015.
Maeda-Martinez, A. N.: The rates of calcium deposition in shells of molluscan larvae, Comp. Biochem. Phys. A, 86, 21–28, 1987.
Malone, P. G. and Dodd, J. R.: Temperature and salinity effects on calcification rate in Mytilus edulis and its paleoecological implications, Limnol. Oceanogr., 12, 432–436, 1967.
Martin, G., Kotta, J., Möller, T., and Herkül, K.: Spatial distribution of marine benthic habitats in the Estonian coastal sea, northeastern Baltic Sea, Est. J. Ecol., 62, 165–191, 2013.
McConnaughey, T. A. and Gillikin, D. P.: Carbon isotopes in mollusk shell carbonates, Geo-Mar. Lett., 28, 287–299, 2008.
Meier, H. E. M., Kjellström, E., and Graham, L. P.: Estimating uncertainties of projected Baltic Sea salinity in the late 21st century, Geophys. Res. Lett., 33, L15705, https://doi.org/10.1029/2006GL026488, 2006.
Melzner, F., Stange, P., Trübenbach, K., Thomsen, J., Casties, I., Panknin, U., Gorb, S., and Gutowska, M. A.: Food supply and seawater pCO2 impact calcification and internal shell dissolution in the Blue Mussel Mytilus edulis, PLOS ONE, 6, e24223, https://doi.org/10.1371/journal.pone.0024223, 2011.
Melzner, F., Thomsen, J., Koeve, W., Oschlies, A., Gutowska, M. A., Bange, H. W., Hansen, H. P., and Körtzinger, A.: Future ocean acidification will be amplified by hypoxia in coastal habitats, Mar. Biol., 160, 1875–1888, 2013.
Miller, A. W., Reynolds, A. C., Sobrino, C., and Riedel, G. F.: Shellfish face uncertain future in high CO2 world: Influence of acidification on oyster larvae calcification and growth in estuaries, PLOS ONE, 4, e5661, https://doi.org/10.1371/journal.pone.0005661, 2009.
Millero, F.: The conductivity-density-salinity-chlorinity relationships for estuarine water, Limnol. Oceanogr., 29, 1317–1321, 1984.
Mucci, A.: The solubility of calcite and aragonite in seawater at various salinities, temperatures, and one atmosphere total pressure, Am. J. Sci., 28, 780–799, 1983.
Müller, J., Schneider, B., and Rehder, G.: Long-term alkalinity trends in the Baltic Sea and implications for Co2-induced acidification, Limnol. Oceanogr., 61, 1984–2002, 2016.
Myrberg, K. and Andrejev, O.: Main upwelling regions in the Baltic Sea – a statistical analysis based on three-dimensional modelling, Boreal Environ. Res., 8, 97–112, 2003.
Natochin, Y. V., Berger, V. Y., Khlebovich, V. V., Lavrova, E. A., and Michailova, O. Y.: The participation of electrolytes in adaptation mechanisms of intertidal molluscs' cells to altered salinity, Comp. Biochem. Phys. A, 63, 115–119, 1979.
Ohlson, M. and Anderson, L.: Recent investigation of total carbonate in the Baltic Sea: changes from the past as a result of acid rain?, Mar. Chem., 30, 259–267, 1990.
Podbielski, I., Bock, C., Lenz, M., and Melzner, F.: Using the critical salinity (Scrit) concept to predict invasion potential of the anemone Diadumene lineata in the Baltic Sea, Mar. Biol., 163, 227, https://doi.org/10.1007/s00227-016-2989-5, 2016.
Ries, J. B.: Review: geological and experimental evidence for secular variation in seawater Mg ∕ Ca (calcite-aragonite seas) and its effects on marine biological calcification, Biogeosciences, 7, 2795–2849, https://doi.org/10.5194/bg-7-2795-2010, 2010.
Riisgard, H. U., Larsen, P. S., Turja, R., and Lundgreen, K.: Dwarfism of blue mussels in the lower saline Baltic Sea – growth to the lower salinity limit, Mar. Ecol.-Prog. Ser., 517, 181–192, 2014.
Roy, R. N., Roy, L. N., Vogel, K. M., Porter-Moore, C., Pearson, T., Good, C. E., Millero, F. J., and Campbell, D.: The dissociation constants of carbonic acid in seawater at salinities 5 to 45 and temperatures 0 to 45 ∘C, Mar. Chem., 44, 249–267, 1993.
Saderne, V., Fietzek, P., and Herman, P. M. J.: Extreme variations of pCO2 and pH in a macrophyte meadow of the Baltic Sea in summer: Evidence of the effect of photosynthesis and local upwelling, PLOS ONE, 8, e62689, https://doi.org/10.1371/journal.pone.0062689, 2013.
Schneider, B. and Kuss, J.: Past and present productivity of the Baltic Sea inferred from pCO2 data, Cont. Shelf Res., 24, 1611–1622, 2004.
Silva, A. L. and Wright, S. H.: Short-term cell volume regulation in Mytilus californianus gill, J. Exp. Biol., 194, 47–68, 1994.
Stuckas, H., Knobel, L., Schade, H., Breusing, C., Hinrichsen, H.-H., Bartel, M., Langguth, K., and Melzner, F.: Combining hydrodynamic modelling with genetics: Can passive larval drift shape the genetic structure of Baltic Mytilus populations?, Mol. Ecol., 26, 2765–2782, 2017.
Stumpp, M., Hu, M., Casties, I., Saborowski, R., Bleich, M., Melzner, F., and Dupont, S.: Digestion in sea urchin larvae impaired under ocean acidification, Nat. Clim. Change, 3, 1044–1049, 2013.
Tambutte, E., Allemand, D., Mueller, E., and Jaubert, J.: A compartimental approach to the mechanism of calcification in hermatypic corals, J. Exp. Biol., 199, 1029–1041, 1996.
Thomsen, J., Gutowska, M. A., Saphörster, J., Heinemann, A., Trübenbach, K., Fietzke, J., Hiebenthal, C., Eisenhauer, A., Körtzinger, A., Wahl, M., and Melzner, F.: Calcifying invertebrates succeed in a naturally CO2-rich coastal habitat but are threatened by high levels of future acidification, Biogeosciences, 7, 3879–3891, https://doi.org/10.5194/bg-7-3879-2010, 2010.
Thomsen, J., Haynert, K., Wegner, K. M., and Melzner, F.: Impact of seawater carbonate chemistry on the calcification of marine bivalves, Biogeosciences, 12, 4209–4220, https://doi.org/10.5194/bg-12-4209-2015, 2015.
Thomsen, J., Stapp, L. S., Haynert, K., Schade, H., Danelli, M., Lannig, G., Wegner, K. M., and Melzner, F.: Naturally acidified habitat selects for ocean acidification–tolerant mussels, Sci. Adv., 3, e1602411, https://doi.org/10.1126/sciadv.1602411, 2017a.
Thomsen, J., Ramesh, K., Sanders, T., Bleich, M., and Melzner, F.: Effects of seawater calcium on calcification in mussel larvae, PANGAEA, https://doi.org/10.1594/PANGAEA.871804, 2017b.
Waldbusser, G. G., Hales, B., Langdon, C. J., Haley, B. A., Schrader, P., Brunner, E. L., Gray, M. W., Miller, C. A., and Gimenez, I.: Saturation-state sensitivity of marine bivalve larvae to ocean acidification, Nat. Clim. Change, 5, 273–280, 2014.
Waldbusser, G. G., Hales, B., Langdon, C. J., Haley, B. A., Schrader, P., Brunner, E. L., Gray, M. W., Miller, C. A., Gimenez, I., and Hutchinson, G.: Ocean acidification has multiple modes of action in bivalve larvae, PLOS ONE, 10, e0128376, https://doi.org/10.1371/journal.pone.0128376, 2015.
Wang, X., Fan, W., Xie, L., and Zhang, R.: Molecular cloning and distribution of a plasma membane calcium ATPase homolog from the pearl oyster Pinctada fucata, Tsinghua Sci. Technol., 13, 439–446, 2008.
Westerbom, M., Kilpi, M., and Mustonen, O.: Blue mussels, Mytilus edulis, at the edge of the range: population structure, growth and biomass along a salinity gradient in the north-eastern Baltic Sea, Mar. Biol., 140, 991–999, 2002.
Whitfield, A. K., Elliott, M., Basset, A., Blaber, S. J. M., and West, R. J.: Paradigms in estuarine ecology – A review of the Remane diagram with a suggested revised model for estuaries, Estuar. Coast. Shelf S., 97, 78–90, 2012.
Williams, E. K. and Hall, J. A.: Seasonal and geographic variability in toxicant sensitivity of Mytilus galloprovinicialis, Australas. J. Ecotox., 5, 1–10, 1999.
Willmer, P. G.: Sodium fluxes and exchange pumps: Further correlates of osmotic conformity in the nerves of an estuarine bivalve (Mytilus edulis), J. Exp. Biol., 77, 207–223, 1978.
Wright, S. H., Moon, D. A., and Silva, A. L.: Intracellular Na+ and the control of amino acid fluxes in the integumental epithelium of a marine bivalve, J. Exp. Biol., 142, 293–310, 1989.
Zhao, L., Schöne, B. R., and Mertz-Kruas, R.: Delineating the role of calcium in shell formation and elemental composition of Corbicula fluminea (Bivalvia), Hydrobiologica, 790, 259–270, 2016.