the Creative Commons Attribution 4.0 License.
the Creative Commons Attribution 4.0 License.
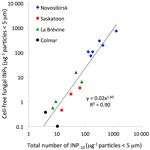
Soils rich in biological ice-nucleating particles abound in ice-nucleating macromolecules likely produced by fungi
Mikhail V. Yakutin
Soil organic matter carries ice-nucleating particles (INPs) the origin of which is hard to define and that are active at slight supercooling. The discovery and characterization of INPs produced by the widespread soil fungus Mortierella alpina permits a more targeted investigation of the likely origin of INPs in soils. We searched for INPs with characteristics similar to those reported for M. alpina in 20 soil samples from four areas in the northern midlatitudes and one area in the tropics. In the 15 samples where we could detect such INPs, they constituted between 1 and 94 % (median 11 %) of all INPs active at −10 ∘C or warmer (INP−10) associated with soil particles < 5 µm. Their concentration increased overproportionately with the concentration of INP−10 in soil and seems to be greater in colder climates. Large regional differences and prevalently high concentrations allow us to make inferences regarding their potential role in the atmosphere and the soil.
- Article
(535 KB) - Full-text XML
- BibTeX
- EndNote
Soils could be a relevant source of ice-nucleating particles (INPs) found in the atmosphere, and INPs from soils are also found in precipitation (Creamean et al., 2013, 2014) and in rivers (Moffett, 2016; Larsen et al., 2017). Organic matter, or biological residues, associated with soil particles may contribute a major share to atmospheric INPs active at temperatures warmer than −10 ∘C (Schnell and Vali, 1976; Szyrmer and Zawadzki, 1997; Conen et al., 2011; O'Sullivan et al., 2014; Creamean et al., 2013; Tobo et al., 2014; Hill et al., 2016). Recent progress in this field of research has been made by the detailed characterization of INPs produced by the widespread soil fungus Mortierella alpina (Fröhlich-Nowoisky et al., 2015). Plating and cultivation have allowed (Fröhlich-Nowoisky et al., 2015) to identify M. alpina as an INP-producing organism through DNA sequencing followed by phylogenetic analysis. Together with the earlier discovery of Fusarium avenaceum and Fusarium acuminatum as sources of INPs with similar characteristics (Pouleur et al., 1992), this new INP source raises the question of the more general relevance of cell-free INPs produced by fungi in soils. Trying to identify and count or determine the mass of these fungi in soil could be one approach. However, this approach would not account for the fact that INPs produced by the organisms can be washed off, may be preserved, may accumulate in the soil, and may be exported from a watershed during intense rainfall (Larsen et al., 2017). In a first attempt to gauge the potential relevance of cell-free, ice-nucleating macromolecules likely derived from fungi, we looked for INPs in soils that match the challenge tests described for M. alpina (Fröhlich-Nowoisky et al., 2015).
Table 1Details of sample origin, including mean annual temperature (MAT) and precipitation (MAP). In each area, between three and six samples (N) were collected. A sample consisted of 100 to 300 g of soil collected from the surface within a radius of a few metres. The maximum distance between samples (Dmax) ranged from 4 to 106 km.
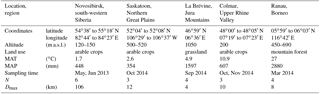
We collected grab samples (100 to 300 g of material per sample) from the surface of arable soils (Table 1) in Novosibirsk (Western Siberia), Saskatoon (Saskatchewan) and Colmar (France), from grasslands in La Brévine (Switzerland), and from tropical mountain forests around Ranau (Borneo). Where present, aboveground vegetation and litter were removed before sampling. Samples were air-dried and dry-sieved (< 63 µm). From each sample, 1 g of dry particles (< 63 µm) was weighed into a 50 mL centrifuge tube containing 20 mL of 0.1 % NaCl, was shaken for 2 min by hand, and was allowed to settle for another 10 min. About 10 mL suspension was withdrawn from the top of the suspension and passed through a syringe filter with 5 µm pore size (sterile cellulose acetate filter; Sterlitech Corporation, Kent, USA); 9 mL of it was put into a pre-weighed aluminium tray; 1.0 to 1.5 mL was put into another tube together with the proper amount of 0.1 % NaCl to create a 1:20 dilution of the suspension. The tray and its content were dried at 80 ∘C and reweighed, and the mass of particles < 5 µm was determined from the difference to a control tray prepared with only an NaCl solution. The tube containing the 1:20 dilution of the suspension was analysed for INPs on a freezing nucleation apparatus (Stopelli et al., 2014) in 52 aliquots of 100 µL in 0.5 mL tubes and, if necessary, further diluted to a concentration at which most, but not all, of the 52 tubes were frozen at −10 ∘C. Final concentrations of particles < 5 µm ranged from 0.02 to 15.5 µg mL−1, with a median of 1.0 µg mL−1. The remainder of the suspension with the final concentration was then passed through a 0.22 µm syringe filter (same material and supplier as above) and partitioned into three portions. One portion was analysed for INPs without further treatment; the other two portions were either heated to 60 or 95 ∘C for 15 min in a water bath before being analysed in the same way. From the original suspension and a 6 M solution of guanidinium chloride (> 99.5 %; Roth GmbH + Co. KG, Karlsruhe, Germany) we prepared a similarly diluted suspension of particles < 0.22 µm and analysed it for INPs after 1 to 2 h of storage at room temperature. Guanidinium chloride deactivates bacterial and fungal INPs but not pollen (Pummer et al., 2012, 2015). Blank samples of 0.1 % NaCl solution did not freeze at −10 ∘C. Our criteria for what we presume are cell-free fungal INPs were an activation temperature of −6.5 ∘C or warmer (INP−6.5) that is retained after heating to 60 ∘C but which is deactivated by heating to 95 ∘C and by 6 M guanidinium chloride. For practical reasons (smallest mesh filter size available), we relaxed the size criterion (< 300 kDa) in Fröhlich-Nowoisky et al. (2015) to < 0.22 µm. This may seem generous, but it still excludes other potential INP−6.5 that are associated with cells and are not detached macromolecules. However, bacterial INPs have been found to not withstand heating to 60 ∘C, with the exception of ice-nucleating entities resistant to boiling produced by Lysinibacillus sp. (Failor et al., 2017). Pollen-derived INP macromolecules are not sensitive to guanidinium chloride or boiling (Pummer et al., 2012, 2015). Thus, the only INPs that are currently known to match the applied test criteria are from fungal sources.
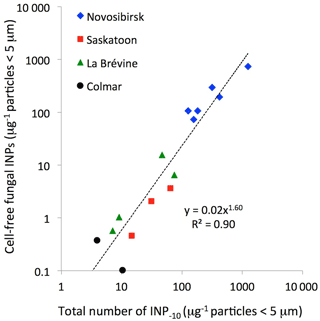
Figure 1Ice-nucleating particles with characteristics of macromolecules released by certain fungi as a function of the total number of INPs active at −10 ∘C in soil particles < 5 µm. The trend line was fitted to all data in the plot. Not plotted are four samples from tropical Ranau (INP−10 < 0.1 µg−1) and one (of three) from the wine-growing area around Colmar (INP µg−1) in which we could not detect any INPs resembling macromolecules released by fungi.
We found what we presume are cell-free fungal INPs in all samples with more than one INP µg−1 particles < 5 µm active at −10 ∘C (INP−10) (Figs. 1, 2). There might also have been a contribution of cell-free fungal INPs in samples with less than 1 INP−10 µg−1, but it was too small to be detected. The latter applies to all four samples from tropical Ranau (INP−10 < 0.1 µg−1) and one (of three) from the wine-growing area around Colmar (INP 0.3 µg−1). Higher concentrations of INPs in cold compared to warm regions were previously reported by Schnell and Vali (1976) and Augustin et al. (2013). Guanidinium chloride reduced the number of INP−10 in all suspensions of particles < 0.22 µm to below the detection limit (to 2 % or less of what we found in suspensions prepared with 0.1 % NaCl), as did heating to 95 ∘C. What we presume are cell-free fungal INPs were therefore not derived from pollen (Pummer et al., 2012, 2015) or Lysinibacillus sp. (Failor et al., 2017), otherwise they would still have been active after heating to 95 ∘C. Averaged over all samples, 97 % (±9 %) of INP−6.5 associated with particles < 5 µm passed through the 0.22 µm filter and 86 % (±10 %) of those remained active after heating to 60 ∘C. Consequently, about five-sixths (0.97 × 0.86 = 0.83) of all INP−6.5 found in soil particles < 5 µm matched characteristics of cell-free fungal INPs. There might have been a small contribution by Isaria farinosa (Huffman et al., 2013) to the number of INP−6.5 determined before heat treatment. However, these INPs would have been deactivated after heating to 60 ∘C (Pummer et al., 2015) and would not have contributed to the number of cell-free fungal INPs considered in the present study. Smaller fractions of INP−10 passed the challenge tests. On average 81 % (±6 %) passed through 0.22 µm and only half (51 %, ±9 %) of all INP−10 were also active after heating to 60 ∘C.
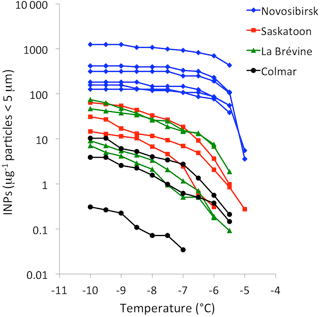
Figure 2Cumulative freezing spectra of the (untreated) samples shown in Fig. 1 and a third sample from Colmar in which we could not detect INPs resembling macromolecules released by fungi (lowermost curve).
Cell-free fungal INPs made up only 1∕20th of INP−10 around Colmar but 2∕3 of INP−10 around Novosibirsk. Regression analysis of the ensemble of 15 samples with detectable cell-free fungal INPs from all four areas on three continents (Fig. 1) suggests that a doubling of INP−10 may be associated with a tripling of the number of cell-free fungal INPs (21.6=3). This trend not only applies across the different areas investigated but also within certain areas (Saskatoon, La Brévine). We speculate that the great plasticity in the contribution of cell-free fungal INPs results from their property of being macromolecules that can be washed off from the mycelium. In principle, the production of a macromolecule requires less resources than the production of a complete cell carrying an ice-nucleation-active entity. Hence, an organism capable of releasing ice-nucleation-active macromolecules has a greater range over which it can potentially modify its surrounding in terms of ice nucleation.
4.1 Atmosphere
The frequency of clouds containing ice particles at moderate supercooling is greater above fertile land than downwind of a desert (Kanitz et al., 2011). One cause of this difference could be higher concentrations of INPs in fertile soils compared to desert soils (Conen et al., 2011). Above fertile land, however, atmospheric INP concentrations do not seem to vary with INP concentration in soils. The 1 to 2 orders of magnitude larger concentrations of INPs in soils at Novosibirsk, compared to soils in La Brévine and Colmar (Fig. 1) leave little or no trace in the atmosphere. At Chaumont, a site 30 km to the east of La Brévine and 120 km to the south-west of Colmar, we observed similar concentrations of atmospheric INPs active at −8 ∘C or warmer to those we observed in Novosibirsk (Conen et al., 2017). During April and May, when arable soils are prepared for seeding and wind erosion is most prevalent (Selegey et al., 2011), median values were four INPs and seven INPs per cubic metre at Chaumont and Novosibirsk, respectively. Thus, soils are unlikely the dominant source of biological INPs in the atmosphere above the cropland belt stretching from western Europe eastward all the way to Novosibirsk. Still, the influence of soils as a source of atmospheric INPs might appear unduly small in this comparison because of efficient atmospheric mixing within the latitudinal band. Nevertheless, it is likely that vegetation and leaves decaying at the soil surface make a larger contribution to atmospheric INP−10 (Schnell and Vali, 1976; Conen et al., 2017). We conclude that cell-free fungal INPs associated with soil dust probably have a minor influence on ice formation in supercooled clouds, and regional differences between soils are masked by atmospheric mixing and relatively larger contributions of INPs from vegetation and decaying leaves.
4.2 Soil
Postulated potential advantages to an organism capable of catalysing ice formation at slight supercooling include the cleavage of structures by ice formation to access otherwise occluded resources (Paul and Ayres, 1991) and the accumulation of water through growing ice from vapour in the surrounding air (Kiefft, 1988). However, there is little experimental evidence to support these ideas in the context of soil. To our knowledge, the most convincing evidence for an accumulation of water in the form of ice was described by Hofmann et al. (2015). Fascinating sculptures of hair ice can form on the surface of dead wood infected by the fungus Exidiopsis effusa through the mechanism of ice segregation. This mechanism transports slightly supercooled water from inside the wood to a body of ice growing on the outside of it. The heat released by the phase transition stabilizes the front between liquid and ice, as long as water is supplied to the growing ice at a sufficient rate. Although fungal activity is responsible for shaping hair ice, ice segregation proceeds under the same conditions without the fungus but then results in an ice crust. Temperatures recorded by Hofmann et al. (2015) inside and outside of wood samples showed that hair ice formation started when temperatures had decreased to about −0.5 ∘C in one experiment and to −2.5 ∘C in another experiment. In both cases, temperature inside the wood increased sharply after the onset of ice formation and stabilized near −0.2 ∘C through the heat released by ice formation, while temperature outside the wood continued to decrease. In one of the experiments, ice growth stopped when outside temperature had decreased to −4 ∘C.
The same process of ice segregation as described by Hofmann et al. (2015) may also take place at the surface or within the porous structure of soil, where larger pores are typically air-filled and water is held in finer capillaries, similar to those supplying water to the hair ice growing on wood. Visible phenomena of water accumulating through ice segregation at or near the soil surface include ice needles and ice lenses (Dash et al., 2006). For a soil fungus to benefit from ice segregation, it has to produce INPs active as close as possible to 0 ∘C. We think that INP−6.5 do not provide much of an advantage in this context. Even in a very small volume of soil, pore water is unlikely to supercool to that temperature. Further, the volume of water that might be harvested through ice segregation would be irrelevantly small if there are other INPs active at the same temperature nearby, which is definitively the case for all samples shown in Fig. 1. It can only be the much rarer INPs active closer to 0 ∘C that potentially provide the advantage of ice segregation to an INP-producing fungus in soil. Pouleur et al. (1992) found about 1 in 104 INP−6.5 was already active at −2.5 ∘C. The large numbers of cell-free fungal INPs found in our samples may just be a proxy for the soil-ecologically relevant INPs active closer to 0 ∘C. The detection of the latter would require larger volumes of soil (e.g. millimetre-size aggregates) tested under conditions where temperature can be controlled with great stability and high precision (e.g. within a dry-block temperature calibrator).
All data is is available upon request from the corrensonding author.
The authors declare that they have no conflict of interest.
The collaboration between the authors on the issue of ice nuclei in soils was
supported by the Swiss National Science Foundation through grant number
IZK0Z2-142484/1 to Mikhail V. Yakutin for a short visit to Basel in summer 2012, during
which much of the method applied in this study was developed. We thank Kirk Blomquist, Vitali Petrovich Baranov, and Simon Tresch for providing samples from Saskatoon, Ranau, and La Brévine,
respectively. This manuscript has benefitted a lot from comments and
suggestions made by Cindy Morris and a second, anonymous referee.
Edited by: Kees Jan van
Groenigen
Reviewed by: Cindy Morris and one anonymous referee
Augustin, S., Wex, H., Niedermeier, D., Pummer, B, Grothe, H., Hartmann, S., Tomsche, L., Clauss, T., Voigtländer, J., Ignatius, K., and Stratmann, F.: Immersion freezing of birch pollen washing water, Atmos. Chem. Phys., 13, 10989–11003, https://doi.org/10.5194/acp-13-10989-2013, 2013.
Conen, F., Morris, C. E., Leifeld, J., Yakutin, M. V., and Alewell, C.: Biological residues define the ice nucleation properties of soil dust, Atmos. Chem. Phys., 11, 9643–9648, https://doi.org/10.5194/acp-11-9643-2011, 2011.
Conen, F., Yakutin, M. V., Yttri, K. E., and Hüglin, C.: Ice nucleating particle concentrations increase when leaves fall in autumn, Atmosphere, 8, 202, https://doi.org/10.3390/atmos8100202, 2017.
Creamean, J. M., Suski, K. J., Rosenfeld, D., Cazorla, A., DeMott, P. J., Sullivan, R. C., White, A. B., Ralph, F. M., Minnis, P., Comstock, J. M., Tomlinson, J. M., and Prather, K. A.: Dust and biological aerosols from the Sahara and Asia influence precipitation in the Western US, Science, 339, 1572–1578, https://doi.org/10.1126/science.1227279, 2013.
Creamean, J. M., Lee, C., Hill, T. C., Ault, A. P., DeMott, P. J., White, A. B., Ralph, F. M., and Prather, K. A.: Chemical properties of insoluble precipitation residue particles, J. Aerosol Sci., 76, 13–27, https://doi.org/10.1016/j.jaerosci.2014.05.005, 2014.
Dash, J. G., Rempel, A. W., and Wettlaufer, J. S.: The physics of premelted ice and its geophysical consequences, Rev. Mod. Phys., 78, 695–741, https://doi.org/10.1103/RevModPhys.78.695, 2006.
Failor, K. C., Schmale III, D. G., Vinatzer, B. A., and Monteil, C. L.: Ice nucleation active bacteria in precipitation are genetically diverse and nucleate ice by employing different mechanisms, ISME J., 11, 2740–2753, https://doi.org/10.1038/ismej.2017.124, 2017.
Fröhlich-Nowoisky, J., Hill, T. C. J., Pummer, B. G., Franc, G. D., and Pöschl, U.: Ice nucleation activity in the widespread soil fungus Mortierella alpine, Biogeosciences, 12, 1057–1071, https://doi.org/10.5194/bg-12-1057-2015, 2015.
Hill. T. C. J., DeMott, P. J., Tobo, Y., Fröhlich Nowoisky, J., Moffett, B. F., Franc, G. D., and Kreidenweis, S. M.: Sources of ice nucleating particles in soils, Atmos. Chem. Phys., 16, 7195–7211, https://doi.org/10.5194/acp-16-7195-2016, 2016.
Hofmann, D., Preuss, G., and Mätzler, C.: Evidence for biological shaping of hair ice, Biogeosciences, 12, 4261–4273, https://doi.org/10.5194/bg-12-4261-2015, 2015.
Huffman, J. A., Prenni, A. J., DeMott, P. J., Pöhlker, C., Mason, R. H., Robinson, N. H., Fröhlich-Nowoisky, J., Tobo, Y., Després, V. R., Garcia, E., Gochis, D. J., Harris, E., Müller-Germann, I., Ruzene, C., Schmer, B., Sinha, B., Day, D. A., Andreae, M. O., Jimenez, J. L., Gallagher, M., Kreidenweis, S. M., Bertram, A. K., and Pöschl, U.: High concentrations of biological aerosol particles and ice nuclei during and after rain, Atmos. Chem. Phys., 13, 6151–6164, https://doi.org/10.5194/acp-13-6151-2013, 2013.
Kanitz, T., Seifert, P., Ansman, A., Engelmann, R., Althausen, D., Casiccia, C., and Rohwer, E. G.: Contrasting the impact of aerosol at northern and southern midlatitudes on heterogeneous ice formation, Geophys. Res. Lett., 38, L17802, https://doi.org/10.1029/2011GL048532, 2011.
Kiefft, T. L.: Ice nucleation activity in lichens, Appl. Environ. Microbiol., 54, 1678–1681, 1988.
Larsen, J. A., Conen, F., and Alewell, C.: Export of ice nucleating particles from a watershed, R. Soc. Open Sci., 4, 170213, https://doi.org/10.1098/rsos.170213, 2017.
Moffett, B. F.: Fresh water ice nuclei, Fund. Appl. Limnol., 188, 19–23, https://doi.org/10.1127/fal/2016/0851, 2016.
O'Sullivan, D., Murray, B. J., Malkin, T. L., Whale, T. F., Umo, N. S., Atkinson, J. D., Price, H. C., Baustian, K. J., Browse, J., and Webb, M. E.: Ice nucleation by fertile soil dusts: relative importance of mineral and biogenic components, Atmos. Chem. Phys., 14, 1853–1867, https://doi.org/10.5194/acp-14-1853-2014, 2014.
Paul, N. D. and Ayres, P. G.: Changes in tissue freezing in Senecio vulgaris infected by rust (Puccinia lagenophorae), Ann. Bot., 68, 129–133, 1991.
Pouleur, S., Richard, C., Martin, J.-G., and Antoun, H.: Ice nucleation activity in Fusarium acuminatum and Fusarium avenaceum, Appl. Environ. Microbiol., 58, 2960–2964, 1992.
Pummer, B. G., Bauer, H., Bernardi, J., Bleicher, S., and Grothe, H.: Suspendable macromolecules are responsible for ice nucleation activity of birch and conifer pollen, Atmos. Chem. Phys., 12, 2541–2550, https://doi.org/10.5194/acp-12-2541-2012, 2012.
Pummer, B. G., Bundke, C., Augustin-Bauditz, S., Niedermeier, D., Felgitsch, L., Kampf, C. J., Huber, R. G., Liedl, K. R., Loerting, T., Moschen, T., Schauperl, M., Tollinger, M., Morris, C. E., Wex, H., Grothe, H., Pöschl, U., Koop, T., and Fröhlich-Nowoisky, J.: Ice nucleation by water-soluble macromolecules, Atmos. Chem. Phys., 15, 4077–4091, https://doi.org/10.5194/acp-15-4077-2015, 2015.
Schnell, R. and Vali, G.: Biogenic ice nuclei: Part I. Terrestrial and marine sources, J. Atmos. Sci., 33, 1554–1564, 1976.
Selegey, T. S., Koutsenogii, K. P., Filonenko, N. N., Popova, S. A., and Lenkovskaya, T. N.: Space-time variability of the characteristics of aerosol in the city-suburbs system (for Novosibirsk as example), Chemistry for Sustainable Development, 19, 289–293, 2011.
Stopelli, E., Conen, F., Zimmermann, L., Alewell, C., and Morris, C. E.: Freezing nucleation apparatus puts new slant on study of biological ice nucleators in precipitation, Atmos. Meas. Tech., 7, 129–134, https://doi.org/10.5194/amt-7-129-2014, 2014.
Szyrmer, W., and Zawadzki, I.: Biogenic and anthropogenic sources of ice-forming nuclei: A review, Bull. Am. Meteorol. Soc., 78, 209–228, 1997.
Tobo, Y., DeMott, P. J., Hill, T. C. J., Prenni, A. J., Swoboda-Colberg, N. G., Franc, G. D., and Kreidenweis, S. M.: Organic matter matters for ice nuclei of agricultural soil origin, Atmos. Chem. Phys., 14, 8521–8531, https://doi.org/10.5194/acp-14-8521-2014, 2014.