the Creative Commons Attribution 4.0 License.
the Creative Commons Attribution 4.0 License.
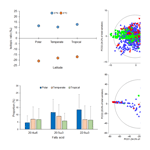
Reviews and syntheses: Insights into deep-sea food webs and global environmental gradients revealed by stable isotope (δ15N, δ13C) and fatty acid trophic biomarkers
Camilla Parzanini
Christopher C. Parrish
Jean-François Hamel
Annie Mercier
Biochemical markers developed initially for food-web studies of terrestrial and shallow-water environments have only recently been applied to deep-sea ecosystems (i.e., in the early 2000s). For the first time since their implementation, this review took a close look at the existing literature in the field of deep-sea trophic ecology to synthesize current knowledge. Furthermore, it provided an opportunity for a preliminary analysis of global geographic (i.e., latitudinal, along a depth gradient) trends in the isotopic (δ15N, δ13C) and fatty acid composition of deep-sea macro- and megafauna from heterotrophic systems. Results revealed significant relationships along the latitudinal and bathymetric gradients. Deep-sea animals sampled at temperate and polar latitudes displayed lower isotopic ratios and greater proportions of essential ω3 long-chain polyunsaturated fatty acids (LC-PUFAs) than did tropical counterparts. Furthermore, δ15N and δ13C ratios as well as proportions of arachidonic acid increased with increasing depth. Since similar latitudinal trends in the isotopic and fatty acid composition were found in surface water phytoplankton and particulate organic matter, these results highlight the link across latitudes between surface primary production and deep-water communities. Because global climate change may affect quantity and quality (e.g., levels of essential ω3 PUFAs) of surface primary productivity, and by extension those of its downward flux, the dietary intake of deep-sea organisms may likely be altered. In addition, because essential ω3 PUFAs play a major role in the response to temperature variations, climate change may interfere with the ability of deep-sea species to cope with potential temperature shifts. Importantly, methodological disparities were highlighted that prevented in-depth analyses, indicating that further studies should be conducted using standardized methods in order to generate more reliable global predictions.
- Article
(1269 KB) -
Supplement
(198 KB) - BibTeX
- EndNote
- Included in Encyclopedia of Geosciences
1.1 Historical background of biochemical biomarkers in deep-sea food-web studies
While the use of biochemical biomarkers in marine food-web studies has a long and successful tradition in shallow-water ecosystems, starting from the 1970s with the use of stable isotopes (McConnaughey and McRoy, 1979) and lipids (Lee et al., 1971), their application in deep-water environments is relatively new (e.g., Iken et al., 2001; Polunin et al., 2001; Howell et al., 2003). Undoubtedly, technological advances made over the past few decades have allowed the exploration of ever deeper ecosystems with more refined techniques. Iken et al. (2001) were among the first to provide a comprehensive analysis of a deep-sea food web, which was sampled at a depth of ∼ 4840 m at the Porcupine Abyssal Plain (PAP, northeast Atlantic), by using bulk stable N and C isotope ratios (δ15N and δ13C, respectively) as trophic markers. In the same year, Polunin et al. (2001) used the same approach to study the trophic relationships of a slope megafaunal assemblage collected off the Balearic Islands (western Mediterranean). Since these first two investigations, several others have been carried out across different oceanic regions and climes, such as the Canadian Arctic (Iken et al., 2005), the Arabian Sea (Jeffreys et al., 2009), and the Sea of Japan (Kharlamenko et al., 2013). Furthermore, over the past decade, it has become evident that the simultaneous use of different trophic markers (e.g., δ15N, δ13C, and fatty acids, FAs) and techniques (e.g., bulk or compound specific isotope analysis, as well as FAs, gut content, and morphometric analyses) provides a more complete picture of trophic structure and dynamics. Indeed, while the first investigations relied on a single method (Iken et al., 2001; Polunin et al., 2001; Howell et al., 2003), the latest trend in deep-sea food-web studies favors an integrative approach, which maximizes the efficiency of each technique, while increasing the resolution of the investigation (e.g., Stowasser et al., 2009; Parzanini et al., 2017).
For the first time since the implementation of trophic markers in studies of deep-sea food webs, this review synthesizes current knowledge in this growing field of research, mainly focusing on heterotrophic ecosystems (i.e., relying on photosynthetic primary production). In addition, it provides a preliminary overview of large-scale geographic trends from the analysis of isotopic and FA data for macro- and megafauna, along with guidance for future investigations. In particular, the present contribution (i) briefly defines various trophic biomarkers and their respective advantages; (ii) describes deep-sea food webs, based on examples from the literature; (iii) lists the sources of variation among the different studies to highlight pitfalls and gaps; and (iv) provides a preliminary quantitative analysis across studies by using relevant data sets.
1.2 Comparison of major trophic markers
The analysis of gut contents was among the first techniques (together with in situ observation of feeding behaviors) applied in trophic ecology and food-web studies in aquatic systems (Gartner et al., 1997; Michener and Kaufman, 2007). Subsequently, other methods were developed as alternative or supplementary means of studying diet and feeding behaviors within the same ecosystems. Among them, the use of biochemical markers as trophic tracers rapidly grew in popularity in food-web ecology since it is relatively simple and should overcome many of the issues ascribed to gut content analysis (Michener and Kaufman, 2007). In this regard, Table 1 lists strengths and drawbacks of gut content analysis and of the two most popular biochemical techniques, i.e., bulk stable isotope and FA analyses. For instance, bulk stable isotope and FA analyses may, theoretically, be performed on any species, regardless of feeding mode and food sources, whereas gut content analysis can only be applied to those organisms characterized by a sufficiently large and full stomach. Except in cases where individuals are too small and have to be analyzed whole, biochemical analyses are typically conducted on target tissues (e.g., muscle) that provide long-term dietary data and reduce intra-individual variability (Table 1). In addition, the use of biochemical tracers requires shorter processing times than gut content analysis. Thanks to this integrative approach and faster output, the application of food-web tracers has been particularly helpful in deep-sea studies, which are often plagued by financial and logistical constraints. Furthermore, due to its relative ease of use, it has favored the analysis of wider sets of taxa/feeding guilds, primary producers included, rather than focusing on one or a few focal groups. However, the interpretation of isotopic and FA data is complex, and both techniques require dedicated and sophisticated instrumentation (e.g., gas chromatograph, mass spectrometer) and knowledge of intrinsic sources of variations (see Sect. 1.4). Although each method needs a sufficient sample size, only gut content analysis may provide direct and clear taxonomic evidence of the diet (Table 1). Therefore, as stated above, the latest trend in trophic ecology advocates a multifaceted approach, on the understanding that each technique may offer unique and valuable data.
Table 1Comparison outlining the major strengths and drawbacks of gut content, stable isotope, and FA analysis.
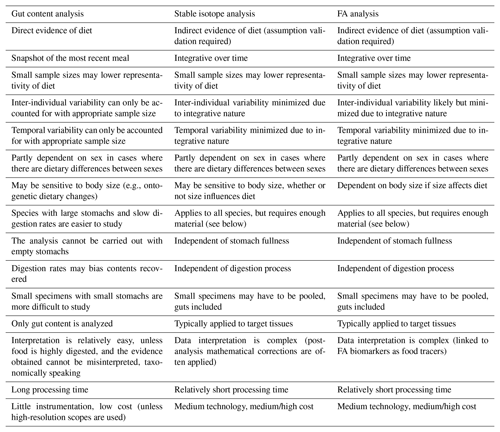
The principle behind the use of food-web tracers is that the biochemical signature of consumers reflects that of their diet. Among them, δ15N and δ13C are the most popular. While the former is used to study trophic positions and dietary sources, with an enrichment factor of 2 ‰–4 ‰ between a consumer and its food (Minagawa and Wada, 1984), the latter undergoes little fractionation (< 1 ‰) and, therefore, is used to distinguish primary food sources (McConnaughey and McRoy, 1979). For further details, refer to Sulzman (2007) and Michener and Kaufman (2007), who have provided extensive reviews on the chemistry behind stable isotopes and their use as food-web tracers, respectively. In addition, sterols, FAs, and amino acids, which are important constituents of lipids (for the first two) and proteins (for the last), have successfully been used to study trophic relationships and dietary sources in deep-water systems (Howell et al., 2003; Drazen et al., 2008a, b). Their use is based on the principle that certain FAs and amino acids are considered essential for animals, being required for optimal fitness. However, most species cannot synthesize these essential compounds de novo and, therefore, they must gain them through their diet. Indeed, only primary producers and a few consumers possess the enzymatic apparatus to synthesize essential FAs and amino acids de novo. Conversely, a few taxa are unable to synthesize sterols de novo, which are critical for them; therefore, they have to acquire these essential sterols through diet (Martin-Creuzburg and Von Elert, 2009). Because sterols, FAs, and amino acids undergo little or no alteration when consumed, it is possible to detect dietary sources within the consumers' tissues (Parrish et al., 2000). The isotopic signature of amino acids can also be used to study trophic position through compound specific analysis (δ15N), as some of these acids show trophic enrichment (Bradley et al., 2015). Detailed information about FA analysis was outside the scope of this study, and is provided by Parrish (2009) and Iverson (2009), whereas the use of sterols as food-web tracers was outlined in Martin-Creuzburg and Von Elert (2009) and Parrish et al. (2000). McClelland and Montoya (2002) and Larsen et al. (2009) discuss the use of amino acids as trophic biomarkers.
1.3 Understanding deep-sea food webs through biochemical markers
As there is no photosynthetically derived primary production in the deep sea, deep-water ecosystems are mostly heterotrophic (Gage, 2003), and may hence largely rely on particulate organic matter (POM) that passively sinks from the surface waters as a primary source of nutrients (Hudson et al., 2004). Nonetheless, food can also be actively transported down by those animals that carry out vertical diel migrations through the water column (Trueman et al., 2014); it can also be provided by the occasional fall of large animal carcasses (Smith and Baco, 2003), and/or by lateral inputs, from inland and shelf areas towards abyssal offshore regions (Pfannkuche, 2005). Although most of the deep-water ecosystems are heterotrophic, a few, such as hydrothermal vents and cold seeps, are fueled by chemical energy (e.g., methane, hydrogen sulfide) and rely on chemosynthetic microorganisms for the production of organic matter. Each of these primary food sources has a specific isotopic composition and biochemical signature, resulting from a combination of chemical and physical processes reflective of its origin. By knowing the composition of the food source(s) that fuel(s) a given food web, it is possible to reconstruct its trophic structure and dynamics. Conversely, by measuring the signatures of the food-web components, it is possible to assess food sources on which they rely. For instance, Iken et al. (2001) showed that phytodetritus was the primary energy input of the deep-sea benthic community at PAP, and also defined two different trophic pathways: a pelagic and isotopically lighter one in which sinking POM and small pelagic prey constituted the main food sources, and a benthic and more isotopically enriched trophic pathway, fueled by degraded sedimented POM. In fact, once POM settles on the seafloor, it undergoes continuous degradation by microbes and is reworked through bioturbation and feeding activities, thus leading to a more isotopically enriched material relative to the sinking one (Iken et al., 2001). Depending on the primary food source they relied on, benthic organisms at PAP were thus characterized by either lower or higher values of δ15N. Similar scenarios of dual trophic pathways characterizing benthic systems were also found by Iken et al. (2005) in the Canadian Arctic, Drazen et al. (2008b) in the North Pacific, Reid et al. (2012) within the benthic community sampled on the mid-Atlantic Ridge, Valls et al. (2014) in the western Mediterranean, and Parzanini et al. (2017) in the northwest Atlantic. Moreover, Kharlamenko et al. (2013) used both stable isotopes and FAs to study the dietary sources of benthic invertebrates collected along the continental slope (500–1600 m depth) in the Sea of Japan. The authors recognized different trophic pathways (i.e., planktonic, benthic, microbial) and dietary sources by using biochemical tracers, and they proposed a strong link with the primary production of the surface waters, as the FA composition of the deep-sea echinoderms and mollusks was similar to that of the shallow-water counterparts.
As POM sinks through the water column, its δ15N increases, reflecting the preferential assimilation of the lighter isotope, 14N, by microbes; in particular, a gradient in POM δ15N has been detected with depth, where POM at greater depths is more enriched (Altabet et al., 1999). For this reason, Mintenbeck et al. (2007) carried out a study in the high-Antarctic Weddell Sea to assess whether this gradient was reflected in the isotopic signature of POM consumers sampled at 50–1600 m. In this regard, only those organisms feeding directly on sinking POM (e.g., suspension feeders) showed increasing values of δ15N with depth, whereas the increase was less evident for the deposit feeders (Mintenbeck et al., 2007). Similar results for suspension feeders were obtained by Bergmann et al. (2009), who analyzed a benthic food web sampled at the deep-water observatory HAUSGARTEN, west of Svalbard (Arctic), between 1300 and 5600 m depth. Conversely, deposit feeders exhibited a negative trend along the bathymetric gradient in terms of δ15N, and predator/scavengers were not affected. In another study, Sherwood et al. (2008) did not detect any relationships with depth in the δ15N values measured from cold-water corals collected on a slope environment in the northwest Atlantic. Among the explanations suggested for these inconsistencies and differences among feeding groups, Mintenbeck et al. (2007) and Sherwood et al. (2008) included feeding preferences with respect to the size and sinking velocity of POM. According to these authors, only those organisms feeding on small particles of sinking POM should reflect a bathymetric gradient in δ15N. In fact, small-sized particles sink at a lower velocity and, therefore, experience high rates of degradation, with more evident changes in δ15N (Mintenbeck et al., 2007). Based on these findings, depth-stratified sampling should ideally be conducted when studying a system characterized by a bathymetric gradient, as it would prevent biases in the interpretation of the isotopic data.
Table 2Sources of variations across studies, distinguished by type (i.e., biological, environmental, analytical).
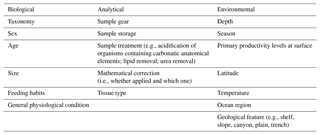
Deep-water systems are generally characterized by a limited food supply, as the quantity of food being transferred from the surface to the bottom diminishes with increasing depth (Gage, 2003). In addition, in temperate areas, food arrives as intermittent pulses, following the spring and late summer blooms of primary (and secondary) productivity. For this reason, deep-water benthic communities can only rely on fresh, high-quality phytodetritus within short temporal windows following algal blooms; whereas reworked and resuspended POM fuels these communities for the rest of the year (Lampitt, 1985). Deep-sea benthic organisms have hence developed adaptations and strategies to increase their feeding success and minimize competition for food, including trophic niche expansion and specialization. In this regard, certain benthic taxa (e.g., pennatulacean corals, hexactinellid sponges) and/or feeding groups (e.g., suspension and deposit feeders) at PAP showed vertical extension of their trophic niches (i.e., omnivory), which, according to Iken et al. (2001), was most likely driven by a strong competition for food. In other words, some species belonging to the same taxon or feeding guild shared similar food sources (i.e., exhibiting similar δ13C values), but they were located at different trophic levels (i.e., exhibiting a wide range of δ15N). Similarly, Jeffreys et al. (2009) reported trophic niche expansion among and within feeding guilds sampled between 140 and 1400 m depth, at the Pakistan margin (Arabian Sea). Pennatulacean corals and other sestonivorous cnidarians, for example, displayed the greatest niche expansion; they fed on not only POM, but also small invertebrates (e.g., zooplankton). Moreover, ophiuroids, which are typically selective deposit feeders, switched to an omnivorous diet under food-limited conditions (Jeffreys et al., 2009). Apart from trophic niche expansion, Iken et al. (2001) proposed that specialization on certain food items represented another adaptation developed by benthic organisms at PAP to mitigate competition for food. Holothuroid echinoderms, for instance, were thought to accomplish food specialization through a combination of different factors involving changes in morphology, mobility, and digestive abilities (Iken et al., 2001). Further examples of trophic niche segregation and food partitioning, as strategies to minimize competition, were also reported for deep-sea demersal fishes in the northwest Mediterranean Sea (Papiol et al., 2013) and for asteroid echinoderms in the northwest Atlantic (Gale et al., 2013). Howell et al. (2003) detected trophic niche expansion across different species of deep-sea asteroids (1053–4840 m) by analyzing their FA composition. In particular, multivariate analysis of FA proportions discriminated three different feeding guilds among the asteroids analyzed, including mud ingesters, predators and scavengers, and suspension feeders.
1.4 Sources of variation across studies
When comparing studies relying on biochemical analysis, there are numerous sources of variation, which may influence results and findings and also prevent the detection of similarities and general trends. However, their importance may depend on the scale of the investigation (i.e., local, regional, or global). In this section, the main sources of variation are illustrated and explained by type (Table 2).
1.4.1 Biological sources
Age, size, and sex, whether related to diet, determine natural intraspecific variability in the isotopic and FA compositions of organisms, which may affect data interpretation of small-spatial-scale investigations. At a basic level, sessile and sedentary taxa typically experience a transition from a pelagic to a benthic lifestyle between the larval and the juvenile stage (Rieger, 1994). Research has also shown that certain deep-sea fish experience changes in diet with age, typically with younger individuals preying upon benthic organisms and adults feeding on prey that are larger and of benthopelagic origin (Mauchline and Gordon, 1984; Eliassen and Jobling, 1985). Stowasser et al. (2009) combined stable isotope analysis (SIA) and FA analysis to detect ontogenetic shifts in the diet of the fish Coryphaenoides armatus and Antimora rostrata, collected at depths between 785 and 4814 m at PAP (northeast Atlantic). By looking at their biochemical composition, the two species switched from active predation to scavenging with increasing size. Similar results are reported in Drazen et al. (2008c) for macrourid fish species from the eastern North Pacific. Conversely, although Reid et al. (2013) detected size-related trends in the δ13C of deep-water fish collected from the Mid-Atlantic Ridge at 2400–2750 m depth, the authors were not able to distinguish whether these results were due to ontogenetic changes in diet or merely to an effect of increasing size, within the size range sampled. Moreover, δ15N and trophic position may increase with body size in adult shallow-water fish, as larger predatory fish ingest larger, more isotopically enriched prey (Badalamenti et al., 2002; Galván et al., 2010).
The potential influence of sex as a source of variation in biomarker studies has not received as much attention and remains ambiguous. Nonetheless, Boyle et al. (2012) studied whether diet and trophic position varied between sexes in deep-sea fish species collected at 55–1280 m depth in the eastern North Pacific using gut content and stable isotope analysis of muscle tissue. The authors did not detect any difference between sexes, but variations in trophic position were encountered when analyzing fish of different sizes (Boyle et al., 2012). An investigation of the oceanic squid Todarodes filippovae sampled within a depth range of 13–380 m in the southwestern Indian Ocean by Cherel et al. (2009) revealed that females had higher values of δ15N, and thus occupied a higher trophic position. However, because T. filippovae exhibits sexual dimorphism in body size, this difference was ultimately shown to be driven by size, i.e., no δ15N variations were detected when females and males of similar sizes were compared (Cherel et al., 2009). Sex may constitute a source of variation in relation to diet in those species that exhibit extreme cases of sexual dimorphism, as in deep-sea anglerfish (Shine, 1989). However, investigation of the role of sex in intraspecific variability will need to be carried out across a broader taxonomic scope before drawing generalizations.
1.4.2 Environmental sources
Larger-scale (e.g., regional, global) comparative studies among deep-sea habitats are complicated by the wide bathymetric ranges they may occupy, anywhere between 200 and ∼ 11 000 m depth. Depth may constitute a major driver of variation in δ15N and δ13C in deep-sea organisms for two main reasons. First, as mentioned earlier, biodegradation processes occurring within the water column may favor the enrichment of POM as it sinks, thus influencing the stable isotope composition of those organisms that directly feed on it (Mintenbeck et al., 2007; Bergmann et al., 2009). Second, size-based trends and shifts in diet, and hence in the isotopic composition, with depth have been reported for deep-sea demersal fish (Collins et al., 2005; Mindel et al., 2016a, b). Likewise, deep-sea species may exhibit different lipid and FA compositions along a bathymetric gradient, reflecting physiological adaptations to changing temperature and pressure with depth (Parzanini et al., 2018b).
Geographic location (e.g., latitude) and season, linked to level and type of surface primary production, nitrogen supply dynamics, and temperature, are also important factors to consider when comparing studies, as large-scale temporal and spatial differences may be detected in the organisms' isotopic composition. Stowasser et al. (2009), for instance, combined stable isotope and FA analyses to study seasonal variations in the diet of five species of demersal fish collected between 785 and 4814 m in the northeast Atlantic. The authors found overall that stable isotope and FA composition of fish varied temporally, and that these differences most likely reflected timing and strength of food inputs sinking from surface waters. However, not all the species (e.g., Coryphaenoides armatus) exhibited a strong seasonality in their biochemical composition, probably due to the high trophic position of the species and the length of the food web analyzed, obscuring the effects of the seasonal POM inputs (Stowasser et al., 2009). Colombo et al. (2016) detected a latitudinal gradient in the FA composition of marine species, with higher levels of ω3-polyunsaturated fatty acids in organisms collected at polar and temperate regions in comparison to tropical ones. Large-scale geographic effects will be further explored below, in the exploratory analytical section; however, Fig. 1 shows where food-web studies accomplished via biochemical tracers have been carried out in heterotrophic ecosystems, highlighting important geographic heterogeneity, especially the limited number of investigations in the Southern Hemisphere.
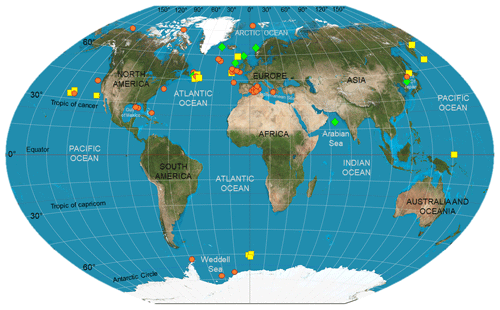
Figure 1Deep-sea biomarker studies in the world ocean. Symbols indicate where the studies listed in Table 2 have been carried out. In detail, red circles represent those investigations that have used stable isotopes as food-web tracers; whereas yellow squares and green diamonds indicate those which used lipids and a combination of SIA and FA analysis, respectively. This map is a derivative of “Creative Commons The world on Winkel tripel projection” by Strebe (2011), used under CC-BY-SA 3.0.
1.4.3 Analytical sources
Several aspects of the SIA methodology can generate variability among studies, including type(s) of tissue chosen for analysis, as well as sample treatment and storage, thus influencing interpretation of small-scale investigations. For instance, lipids have lower 13C in comparison to proteins and carbohydrates (DeNiro and Epstein, 1977); lipid-rich tissues hence display lower δ13C values. In addition, there are tissues, such as liver in fish and gonads in other taxa, which are characterized by higher turnover rates of lipids than others (e.g., white muscle), and hence incorporate information only on the recent diet. To avoid biases caused by the presence of lipids in tissues, several approaches may be used. Stowasser et al. (2009) and Boyle et al. (2012), for example, opted to extract lipid from the tissues prior to analysis, whereas Sherwood et al. (2008), Fanelli et al. (2011a, b), and Papiol et al. (2013) applied a mathematical correction to their δ13C data, based on the elemental C-to-N ratio (C : N) characterizing the samples. Other authors, such as Polunin et al. (2001) and Carlier et al. (2009), did not apply any treatment. In the case of mathematical corrections, two equations are currently used for deep-sea organisms, those proposed by Post et al. (2007) and Hoffman and Sutton (2010). Since lipid extraction increases values of δ15N in deep-sea fish muscle tissue (Hoffman and Sutton, 2010), this practice is not recommended. Conversely, mathematical corrections seem to be preferable when dealing with lipids, and they have already been applied in several studies, including those mentioned above.
Some marine organisms, such as corals and echinoderms, contain carbonate skeletal elements. Since inorganic carbonate has higher δ13C values than other fractions (Pinnegar and Polunin, 1999), it is a widespread practice to acidify these types of samples. Variations occur when acidification is executed on samples that are simultaneously run for δ15N and δ13C, as the treatment may affect δ15N data (Bunn et al., 1995). Whenever feasible, depending on both financial constraints and the sizes of the organisms, processing samples separately for each isotope would therefore be advisable, as in Carlier et al. (2009), Sherwood et al. (2008), and Papiol et al. (2013).
The tissues of elasmobranchs (e.g., sharks, rays) contain urea and trimethylamine oxide, which are both 15N-depleted; therefore, their presence may affect stable isotope data (Hussey et al., 2012; Kim and Koch, 2012; Churchill et al., 2015). As for the inorganic carbonate issue, there is no agreement among studies. Nonetheless, the removal of urea prior to analysis or the use of arithmetic corrections is among the most common solutions applied to deal with the presence of these compounds. In addition, the former seems to be the more commonly recommended and performed, as the application of mathematical corrections requires the calculation of species-specific discrimination factors, which is not always feasible (Hussey et al., 2012).
Sample storage is also crucial to obtain reliable data since nonoptimal preservation methods may compromise the outcome of the investigation. Regarding the storage temperature, while biological samples for gut content and stable isotope analysis are commonly frozen at −20 ∘C, if not processed soon after their collection, those for lipid analysis are stored at either −80 ∘C (recommended) or −20 ∘C prior to further processing in the lab. Since storage at −20 ∘C might not completely prevent lipid degradation, especially if samples are analyzed after several years, rapid initial processing of samples and vacuum packing may reduce potential issues when freezing at −80 ∘C is not logistically feasible. In addition, freezing is highly recommended over chemical storage for SIA, as there is evidence that formalin/ethanol considerably alters the isotopic ratios in biological tissues (Arrington and Winemiller, 2002; Syväranta et al., 2011; Xu et al., 2011).
The study of large-scale trends in biological variables (e.g., distribution, biochemical composition, biodiversity) may not only help understand general functioning and structure of ecosystems, but it may also allow us to make predictions and support conservation initiatives. While several studies already exist on large-scale distribution and biodiversity patterns of deep-sea species (Rex et al., 1993; Stuart et al., 2003; Ramirez-Llodra et al., 2010), a similar approach has yet to be applied to trophodynamics. This preliminary analysis detected global spatial trends (i.e., along latitudinal and depth gradients) in the isotopic and FA composition of deep-water animals for the first time since the application of biochemical tracers to the study of trophic ecology in the deep sea.
Latitudinal gradients have been detected in δ13C of plankton and POM collected from surface waters in both the Southern Hemisphere and Northern Hemisphere, with decreasing values towards the polar regions (Sackett et al., 1965; Rau et al., 1982; Francois et al., 1993). Both environmental (e.g., temperature, nutrient supply) and biological (e.g., plankton metabolism) factors have been proposed to explain such trends (Rau et al., 1982; Francois et al., 1993). The stable N isotope signature of surface primary production may also vary regionally, depending on the nutrient (mainly N) supply to the phytoplankton, as well as its community structure and cell size (Choy et al., 2015; Hetherington et al., 2017). Oligotrophic areas, characterized by marked oxygen minimum zones and by high denitrification rates, such as the eastern tropical Pacific Ocean, typically have higher δ15N values (Hetherington et al., 2017). In addition, latitudinal trends have been detected in the FA composition of marine organisms, which tend to have higher levels of essential ω3 long-chain polyunsaturated fatty acids (LC-PUFA) in the polar and temperate regions in comparison to the tropical ones (Colombo et al., 2016). As POM is the main food source of most deep-sea food webs (Gage, 2003; Hudson et al., 2004), we hypothesized that (a) similar latitudinal gradients exist in the isotopic and essential PUFA composition of deep-water organisms and that (b) the strength of these trends varies among organisms from different habitats, i.e., pelagic, demersal, and benthic, as diversely dependant on POM. Furthermore, as both isotopic and lipid composition of POM and as deep-sea taxa varied along a depth gradient in the deep North Pacific (Lewis, 1967; Altabet et al., 1999), North Atlantic (Polunin et al., 2001; Parzanini et al., 2018a, b, 2017), and Arctic oceans (Bergmann et al., 2008), we hypothesized that similar trends could be extended to the global scale.
2.1 Materials and methods
2.1.1 Data set
This analysis focused on studies that used either bulk stable isotope or FA analysis, or a combination of them, to infer trophic relationships of deep-water macro- and megafauna, as well as to study deep-sea food webs, from heterotrophic ecosystems. Experimental studies as well as investigations on chemosynthetic habitats (e.g., hydrothermal vents) were excluded a priori to avoid possible biases. In fact, these habitats are fueled by primary dietary sources, e.g., methane, whose isotopic and FA composition is substantially different than that of POM (Rau and Hedges, 1979; Saito and Osako, 2007). Table 3 outlines the full data set collated for the present analysis, which includes 52 different studies. The literature search was carried out through Scopus and Google Scholar portals using the following key words: stable isotopes, fatty acids, food webs, deep sea, trophic ecology, and trophic relationships. Additional sources provided by an anonymous referee were also included. These studies were used to analyze global trends in δ15N, δ13C, and the essential arachidonic (ARA, 20 : 4ω6), eicosapentaenoic (EPA; 20 : 5ω3), and docosahexaenoic (DHA, 22 : 6ω3) acids across deep-water communities. ARA, EPA, and DHA are the most important nutrients in aquatic ecosystems, required by organisms for optimal health (Parrish, 2009), as well as being excellent trophic biomarkers. In fact, whereas EPA and DHA are typically used as biomarkers in diatoms and dinoflagellates, respectively (Parrish, 2013), in the deep sea, ARA is associated with microorganisms from the sediment (Howell et al., 2003). Our study focused on these three FAs since they are present in all the organisms under analysis.
Table 3List of trophic ecology studies in deep-sea heterotrophic systems, carried out using stable isotopes (bulk) and lipids (including FA) as food-web tracers. Experimental studies were excluded a priori. Reference, method(s) applied, latitude, sampling depth, ocean region, and taxa analyzed are reported for each study. Polar latitudes include investigations between 60 and 90∘ N or S, whereas temperate and tropical latitudes represent studies carried out within 0–30 and 30–60∘ N, respectively. References are ordered according to sampling depth(s).
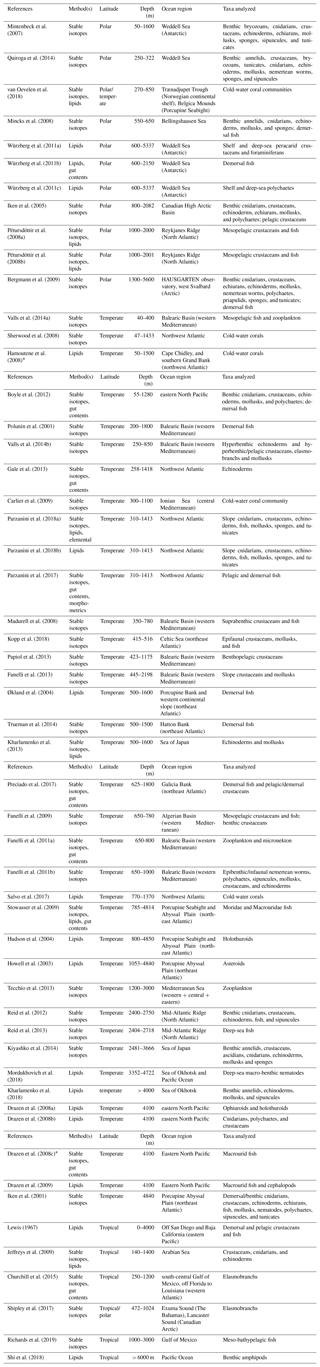
* The study was excluded from analyses because it did not meet the criteria outlined in Sect. 2.1.1 or did not include any data.
2.1.2 Variables considered
Each species from each investigation was sorted by latitude (i.e., tropical, 0–30∘; temperate, 30–60∘; and polar, 60–90∘), habitat (i.e., pelagic, demersal, and benthic), depth at collection (i.e., mesopelagic, 200–1000 m; bathypelagic, 1000–4000 m; and abyssopelagic, > 4000 m, for pelagic species; bathyal 200–4000 m; abyssal, 4000–6000 m; and hadal, > 6000 m, for benthic species), and phylum (i.e., Annelida, Arthropoda, Brachiopoda, Bryozoa, Chaetognatha, Chordata, Cnidaria, Hemichordata, Echinodermata, Mollusca, Nematoda, Nemertea, Porifera, and Sipuncula). Information about species habitat was either obtained through WoRMS and FishBase online databases or was already included in the source paper. In addition, species were labeled as “meso-bathypelagic” and “bathyal-abyssal”, if the depth at collection was not specified further, but the whole set of samples for a study was collected within those zones. In the current analysis, tissue type, acidification treatment, sampling season, sex, and age were not considered as variables because (i) they were assumed to not play a major role in global-scale investigations and/or (ii) this information was not always provided. In addition, tests were performed on lipid-corrected and uncorrected δ13C data pooled together. For analyses regarding stable isotope composition (δ15N, δ13C), data were obtained from Iken et al. (2005), Mincks et al. (2008), Bergmann et al. (2009), Quiroga et al. (2014), and van Oevelen et al. (2018), for polar regions; Iken et al. (2001), Madurell et al. (2008), Sherwood et al. (2008), Carlier et al. (2009), Fanelli et al. (2009), Stowasser et al. (2009), Fanelli et al. (2011a, b), Boyle et al. (2012), Reid et al. (2012), Fanelli et al. (2013), Gale et al. (2013), Kharlamenko et al. (2013), Papiol et al. (2013), Reid et al. (2013), Tecchio et al. (2013), Kiyashko et al. (2014), Trueman et al. (2014), Valls et al. (2014a, b), Kopp et al. (2018), Parzanini et al. (2017), Preciado et al. (2017), and Parzanini et al. (2018a) for temperate latitudes; and Jeffreys et al. (2009), Churchill et al. (2015), Shipley et al. (2017), and Richards et al. (2019) for tropical regions (Table S1). FA composition (ARA, EPA, and DHA) data were collected from Pétursdóttir et al. (2008a, b) and Würzberg et al. (2011a, b, c) for polar areas; Lewis (1967), Howell et al. (2003), Hudson et al. (2004), Økland et al. (2005), Drazen et al. (2008a, b), Stowasser et al. (2009), Murdukhovich et al. (2018), Parzanini et al. (2018a), Salvo et al. (2018), van Oevelen et al. (2018), and Kharlamenko et al. (2018) for temperate regions; and Jeffreys et al. (2009) and Shi et al. (2018) for tropical regions (Table S2).
2.2 Statistical analysis
Comparisons among multiple groups of deep-sea organisms were run through t tests and one-way analysis of variance (ANOVA). In particular, isotopic (i.e., δ15N, δ13C) and FA (i.e., ARA, EPA, and DHA) data were compared across organisms from different latitudes (i.e., tropical, temperate, and polar), habitats (i.e., pelagic, demersal, benthic), and collection depths (i.e., mesopelagic, bathypelagic, meso-bathypelagic, abyssopelagic, bathyal, bathyal-abyssal, abyssal, and hadal) to detect any significant differences. When the normality assumption was violated, Mann–Whitney rank sum test, Kruskal–Wallis one-way ANOVA on ranks, and Dunn's method pairwise comparisons were performed instead. In addition, multivariate statistics, i.e., principal coordinate analysis (PCO) and permutational MANOVA (PERMANOVA), were used to study the variability in the isotopic and FA composition of deep-water organisms across different latitudes, habitats, collection depths, and phyla. In addition, a distance-based linear model (DistLM) was run to assess which of these four factors contributed the most to such a variability. PCO, PERMANOVA, and DistLM were run on resemblance matrices, based on Euclidean distance for the isotopic data, and Bray–Curtis for the FA data. Data were not normalized or transformed prior to analysis. Univariate statistics were conducted using Sigmaplot 12.5, while PCO, PERMANOVA, and DistLM were run through Primer 7.0 with the add-on package PERMANOVA+ (Clarke and Gorley, 2006).
2.3 Results
Analyses revealed both latitudinal and depth-related trends for isotopic and essential FA composition. In particular, mean values (± SD) of δ15N and δ13C were significantly lower in deep-sea fauna sampled at high latitudes than in that collected at low latitudes (δ15N, ANOVA on ranks, H = 35.6, p ≤ 0.001; δ13C, ANOVA on ranks, H = 277.9, p ≤ 0.001; Fig. 2). Conversely, no difference was detected across latitudes in terms of ARA, but mean proportions (± SD) of EPA and DHA were significantly greater at polar latitudes than at temperate and tropical areas (EPA, ANOVA on ranks, H = 11.4, p=0.003; DHA, ANOVA on ranks, H = 63.6, p ≤ 0.001; Fig. 3). Similarly, PERMANOVA detected significant differences across latitudes in terms of both stable isotopes (pseudo-F = 81.4, p(perm) = 0.0001) and essential FAs (pseudo-F = 11.0, p(perm) = 0.0001).
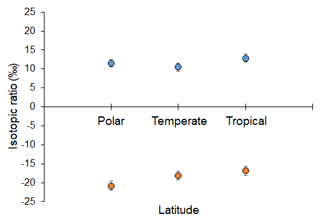
Figure 2Stable N and C isotopic composition of deep-sea animals across latitudes. Mean values of δ15N (blue circles above) and δ13C (orange circles below) (‰) measured in deep-sea organisms across polar, temperate, and tropical latitudes. Bars represent standard deviation (polar, n = 235; temperate, n = 1469; tropical, n = 41).
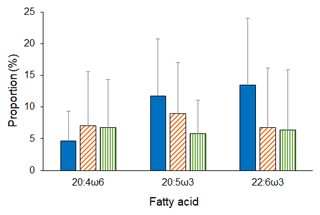
Figure 3Essential FA composition of deep-sea animals across latitudes. Mean proportions of essential FA measured in the tissues of deep-sea animals from polar (blue bars), temperate (orange diagonal striped bars), and tropical (green vertical striped bars) latitudes. Bars represent standard deviation (polar, n = 176; temperate, n = 227; tropical, n = 11).
When deep-water species were analyzed separately according to their habitat, the same latitudinal trend in the isotopic composition was shown for deep-water benthic species (δ15N, ANOVA on ranks, H = 40.5, p ≤ 0.001; δ13C, ANOVA on ranks, H = 171.2, p ≤ 0.001), whereas, for demersal and pelagic species, only the δ13C ratios were significantly lower at higher latitudes (ANOVA on ranks, H = 105.7, p ≤ 0.001, for demersal species; ANOVA on ranks, H = 11.5, p=0.003, for pelagic species). PERMANOVA showed that the isotopic composition of deep-sea animals was indeed statistically different across the three habitats (pseudo-F = 112.6, p(perm) = 0.0001), and benthic and demersal species had higher stable N and C isotope ratios than the pelagic counterparts (p<0.05). Conversely, only benthic and pelagic species revealed a latitudinal gradient in their essential FA composition (EPA, ANOVA on ranks, H = 12.1, p=0.002; DHA, ANOVA on ranks, H = 43.6, p ≤ 0.001, for benthic species; EPA, ANOVA, H = 6.4, p=0.011, for pelagic taxa). In this regard, pelagic, demersal, and benthic taxa had a different essential FA composition (ARA, ANOVA on ranks, H = 39.7, p ≤ 0.001; EPA, ANOVA on ranks, H = 12.5, p=0.002; DHA, ANOVA on ranks, H = 76.9, p ≤ 0.001; pseudo-F = 19.7, p(perm) = 0.0001). Benthic species had the highest proportions of ARA and EPA (p<0.05), while demersal species had the highest levels of DHA, although similar to those of pelagic species.
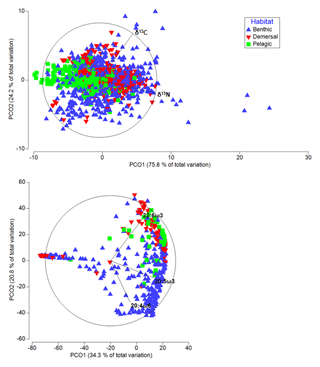
Figure 4Differences in terms of biochemical compositions among deep-sea animals from various habitats. Principal coordinate analysis plots representing differences in terms of isotopic (above) and essential FA composition (below) of deep-water species. In both cases, the variable “habitat” was one of the most important factors, contributing 12 % and 8 %, respectively, to the variability in the biochemical composition of the deep-sea species.
While mean values of both stable N and C isotope ratios significantly increased with depth for benthic and demersal species (δ15N, ANOVA on ranks, H = 63.9, p ≤ 0.001; δ13C, ANOVA on ranks, H = 126.2, p ≤ 0.001), only δ13C ratios showed the same trend in pelagic taxa (ANOVA on ranks, H = 125.5, p ≤ 0.001). Proportions of EPA significantly decreased along the bathymetric gradient for pelagic taxa (ANOVA on ranks, H = 12.3, p=0.002), and levels of ARA were significantly higher at abyssal depths for benthic and demersal species (ANOVA on ranks, H = 39.7, p ≤ 0.001). In addition, levels of δ15N, δ13C, and ARA increased for benthic and demersal organisms with increasing depth (δ15N, ANOVA on ranks, H = 84.7, p ≤ 0.001; δ13C, ANOVA on ranks, H = 105.0, p ≤ 0.001; ARA, ANOVA on ranks, H = 22.8, p ≤ 0.001). PERMANOVA revealed significant differences in the isotopic (pseudo-F = 74.6, p(perm) = 0.0001) and essential FA composition (pseudo-F = 8.6, p(perm) = 0.0001) across collection depths.
Among the four variables considered (i.e., latitude, habitat, collection depth, and phylum), analyses revealed that “habitat” and “phylum” were the most important factors influencing the variability of the stable isotope (respectively 12 % and 9 %; DistLM, adjusted R2=0.4) and FA (respectively 8 % and 11 %; DistLM, adjusted R2=0.3) composition of deep-water organisms (Fig. 4).
2.4 Discussion
The present analysis shows, for the first time, the existence of (a) latitudinal trends in both stable isotope and essential FA composition of deep-sea organisms, with decreasing δ13C ratios and increasing ω3 LC-PUFAs towards the poles, and (b) global bathymetric trends in the isotopic composition of deep-water fauna for which mean levels of δ15N, δ13C, and ARA increased with increasing depth. In addition, it provides further evidence of the link, across latitudes and depth, between surface primary production of the surface waters and the deep-water consumers. The present findings generally align with reports of decreasing values of δ13C in surface-water plankton and POM towards the polar regions, in both the Southern Hemisphere and Northern Hemisphere (Sackett et al., 1965; Rau et al., 1982; Francois et al., 1993), as well as of increasing POM isotopic ratios along a bathymetric gradient (Altabet et al., 1999). They also agree with Colombo et al. (2016), who noticed that proportions of ω3 LC-PUFAs were higher in marine organisms from polar and temperate regions in comparison to tropical regions, and with Parzanini et al. (2018a), who detected increasing proportions of ARA along a slope area in the deep northwest Atlantic.
Water temperature, in combination with other abiotic (e.g., oceanographic and biogeochemical processes, nutrient supply) and biological factors (e.g., species metabolism, taxonomic composition of deep-water communities, microbial remineralization processes), seems to play a role in these trends (Rau et al., 1982; Francois et al., 1993; Altabet et al., 1999; Colombo et al., 2016). In particular, water temperature influences isotopic fractionation processes and, typically, higher fractionation is associated with lower temperatures (Sackett et al., 1965). High fractionation rates are also linked to the pronounced denitrification activities characterizing oligotrophic areas, such as observed in some areas of the tropics (Hetherington et al., 2017). This may explain the higher δ15N ratios of the deep-sea organisms from the tropical latitudes analyzed in this study. Furthermore, water temperature affects membrane fluidity, and lower temperatures decrease the fluidity of cell membrane (Parrish, 2013; Colombo et al., 2016). Thus, in order to maintain normal membrane function and condition, i.e., health, ectotherms may counteract variations in water temperature by readjusting their FA composition (Cossins and Lee, 1985; Parrish, 2013). For example, larger proportions of long-chain unsaturated FAs (e.g., ARA, EPA) within the lipid bilayer help increase membrane fluidity (Parrish 2013), as these molecules are characterized by a higher flexibility (DeLong and Yayanos, 1985; Colombo et al., 2016).
Trends in the isotopic and FA composition of deep-sea organisms were also seen along a depth gradient. As a proxy for water temperature as well as nutrient supply, depth may influence biochemical composition of marine consumers (Parzanini et al., 2018a, b). POM becomes more isotopically enriched while sinking to deeper depth due to microbial degradation (Altabet et al., 1999). Thus, the isotopic composition of deep-water organisms which feed on POM may vary accordingly (Mintenbeck et al., 2007). In the present analysis, levels of ARA were globally higher at deeper depths, similar to the study by Parzanini et al. (2018a), which may be due to (i) a higher reliance of deeper-dwelling organisms on the benthic-detrital trophic pathway, and/or (ii) the need to maintain membrane fluidity at low temperatures via increasing the unsaturation levels of membrane phospholipids.
Finding latitudinal trends in the biochemical composition of deep-water organisms that mirror results from shallow depths provides further evidence of the link between the two systems, in that deep-sea benthic communities rely on POM sinking from the surface water as a primary food source (Gage, 2003; Hudson et al., 2004). Close dependence of deep-sea food webs on near-surface processes raises important concerns. According to the latest climate estimates, both air and water temperatures have been rising, and continue to increase, and seawater pH has already dropped by 0.1 units due to large CO2 emissions and is expected to decrease further (IPCC, 2014). Furthermore, models predict that increasing surface water temperature will favor stratification, while reducing vertical mixing as well as enhancing variability in the transport of primary production and energy (i.e., carbon) transport to the deep sea (Smith et al., 2009; Jones et al., 2014; Sweetman et al., 2017). At the same time, deep-water benthic biomass is expected to decrease due to the increasing variability in the food supply, which may in turn affect health and functioning of benthic ecosystems, as well as global biogeochemical cycles (Jones et al., 2014). Hixson and Arts (2016) showed that the FA composition of the six most common fresh- and saltwater phytoplankton species responded to temperature and, specifically, that their ω3 PUFA levels decreased with increasing temperature. Not only do ω3 PUFAs, such as EPA and DHA, play an important role in the response to temperature variations in aquatic systems, but they are also essential nutrients and are highly required by aquatic organisms for optimal growth and health (Parrish, 2009). A case in point, Rossoll et al. (2012) showed experimentally that growth and reproduction of the copepod Acartia tonsa were severely compromised by the alteration of FA content and composition of its primary food source, the diatom Thalassiosira pseudonana, exposed to high CO2 levels. The present investigation, therefore, suggests that changes in amounts and composition of surface production could also result in changes in essential nutrients and biomarkers in deep-sea benthic organisms that feed on it, with possible cascading effects throughout deep-water food webs. Such variations may alter nutrient intake of deep-sea benthic organisms, as well as trophodynamics; and they may also influence species' abilities to cope with deep cold waters.
This investigation provides a first summary of the information available on deep-sea food webs inferred by bulk stable isotope and FA analyses, providing guidance for future studies and a glimpse at global-scale patterns in the biochemical composition of deep-water organisms from heterotrophic ecosystems. Food-web tracers represent a powerful tool that can help elucidate the structure and dynamics of food webs from shallow to deeper waters, and support management initiatives. However, this tool is even more effective when combined with other techniques (e.g., gut content analysis), as each method provides uniquely valuable data. When comparing studies, it emerges that there are multiple sources of variations, whether biological, environmental, and/or analytical. Depending on the scale of the investigation, these differences are more or less susceptible to biases, suggesting that they have to be considered and acknowledged when attempting cross-comparisons even though they may be contextually acceptable. The preliminary analysis conducted here detected latitudinal and bathymetric trends in the isotopic and FA composition of deep-sea species. In light of global climate change and the link between surface production and deep-sea communities, changes in amounts and composition of surface production may influence the essential nutrient intake (e.g., ω3 PUFA) of deep-water organisms. Because ω3 PUFAs are involved in the response to temperature variations in ectotherms, climate change may also affect the ability of these species to cope with potential temperature shifts. However, more studies are required to help detect global trends, especially in those areas that are still poorly understood (most deep-sea areas) or not yet investigated (e.g., in the Southern Hemisphere). In addition, it is necessary to standardize analytical methods to limit their influence and help compensate for natural variability.
All data used for analysis can be found as supplementary material, in Tables S1 and S2.
The supplement related to this article is available online at: https://doi.org/10.5194/bg-16-2837-2019-supplement.
All the authors contributed to the paper conceptualization and methodology. CP was responsible for data curation, formal analysis, investigation, and writing the original draft of the paper. CCP, JH, and AM reviewed and edited the draft. Lastly, CCP and AM provided supervision as well as funds for this project.
The authors declare that they have no conflict of interest.
The authors acknowledge the Natural Science and Engineering Research Council of Canada (NSERC) Discovery Grant and Canada Foundation for Innovation (CFI) Leaders Opportunity Fund for funding. The authors also want to thank Jeff Drazen, Paul Snelgrove, Patrick Gagnon, Emaline Montgomery, and Kristin Bøe for providing ideas in the development and improvement of this paper and the two anonymous reviewers for insightful comments.
This research has been supported by the Natural Sciences and Engineering Research Council of Canada (grant no. 311406), the Natural Sciences and Engineering Research Council of Canada (grant no. 105379), and the Canada Foundation for Innovation (grant no. 11231).
This paper was edited by Jack Middelburg and reviewed by two anonymous referees.
Altabet, M. A., Pilskaln, C., Thunell, R., Pride, C., Sigman, D., Chavez, F., and Francois, R.: The nitrogen isotope biogeochemistry of sinking particles from the margin of the Eastern North Pacific, Deep-Sea Res. Pt. I, 46, 655–679, https://doi.org/10.1016/S0967-0637(98)00084-3, 1999.
Arrington, D. A. and Winemiller, K. O.: Preservation effects on stable isotope analysis of fish muscle, Trans. Am. Fish Soc., 131, 337–342, https://doi.org/10.1577/1548-8659(2002)131<0337:PEOSIA>2.0.CO;2, 2002.
Badalamenti, F., D'Anna, G., Pinnegar, J., and Polunin, N.: Size-related trophodynamic changes in three target fish species recovering from intensive trawling, Mar. Biol., 141, 561–570, https://doi.org/10.1007/s00227-002-0844-3, 2002.
Bergmann, M., Dannheim, J., Bauerfeind, E., and Klages, M.: Trophic relationships along a bathymetric gradient at the deep-sea observatory HAUSGARTEN, Deep-Sea Res. Pt. I., 56, 408–424, https://doi.org/10.1016/j.dsr.2008.10.004, 2009.
Boyle, M., Ebert, D., and Cailliet, G.: Stable-isotope analysis of a deep-sea benthic-fish assemblage: evidence of an enriched benthic food web, J. Fish Biol., 80, 1485–1507, https://doi.org/10.1111/j.1095-8649.2012.03243.x, 2012.
Bradley, C. J., Wallsgrove, N. J., Choy, C. A., Drazen, J. C., Hetherington, E. D., Hoen, D. K., and Popp, B. N.: Trophic position estimates of marine teleosts using amino acid compound specific isotopic analysis, Limnol. Oceanogr-Meth., 13, 476–493, https://doi.org/10.1002/lom3.10041, 2015.
Bunn, S., Loneragan, N., and Kempster, M.: Effects of acid washing on stable isotope ratios of C and N in penaeid shrimp and seagrass: implications for food-web studies using multiple stable isotopes, Limnol. Oceanogr., 40, 622–625, https://doi.org/10.4319/lo.1995.40.3.0622, 1995.
Carlier, A., Le Guilloux, E., Olu, K., Sarrazin, J., Mastrototaro, F., Taviani, M., and Clavier, J.: Trophic relationships in a deep Mediterranean cold-water coral bank (Santa Maria di Leuca, Ionian Sea), Mar. Ecol. Prog. Ser., 397, 125–137, https://doi.org/10.3354/meps08361, 2009.
Cherel, Y., Fontaine, C., Jackson, G. D., Jackson, C. H., and Richard, P.: Tissue, ontogenic and sex-related differences in δ13C and δ15N values of the oceanic squid Todarodes filippovae (Cephalopoda: Ommastrephidae), Mar. Biol., 156, 699–708, https://doi.org/10.1007/s00227-008-1121-x, 2009.
Choy, C. A., Popp, B. N., Hannides, C. C., and Drazen, J. C.: Trophic structure and food resources of epipelagic and mesopelagic fishes in the North Pacific Subtropical Gyre ecosystem inferred from nitrogen isotopic compositions, Limnol. Oceanogr., 60, 1156–1171, https://doi.org/10.1002/lno.10085, 2015.
Churchill, D. A., Heithaus, M. R., Vaudo, J. J., Grubbs, R. D., Gastrich, K., and Castro, J. I.: Trophic interactions of common elasmobranchs in deep-sea communities of the Gulf of Mexico revealed through stable isotope and stomach content analysis, Deep-Sea Res. Pt. II., 115, 92–102, https://doi.org/10.1016/j.dsr2.2014.10.011,2015.
Clarke, K. and Gorley, R.: PRIMER Plymouth, UK, PRIMERE Ltd, 2006.
Collins, M., Bailey, D., Ruxton, G., and Priede, I.: Trends in body size across an environmental gradient: a differential response in scavenging and non-scavenging demersal deep-sea fish, P. Roy. Soc. B-Biol. Sci., 272, 2051–2057, https://doi.org/10.1098/rspb.2005.3189, 2005.
Colombo, S. M., Wacker, A., Parrish, C. C., Kainz, M. J., and Arts, M. T.: A fundamental dichotomy in long-chain polyunsaturated fatty acid abundance between and within marine and terrestrial ecosystems, Environm. Rev., 25, 163–174, https://doi.org/10.1139/er-2016-0062, 2016.
Cossins, A. and Lee, J.: The adaptation of membrane structure and lipid composition to cold, in: Circulation, respiration, and metabolism, edited by: Gylles, R., Springer, Berlin, Heidelberg, Germany, 543–552, 1985.
DeLong, E. G. and Yayanos, A. A.: Adaptation of the membrane lipids of a deep-sea bacterium to changes in hydrostatic pressure, Science, 228, 1101–1104, 1985.
DeNiro, M. J. and Epstein, S.: Mechanism of carbon isotope fractionation associated with lipid synthesis, Science, 197, 261–263, 1977.
Drazen, J. C., Phleger, C. F., Guest, M. A., and Nichols, P. D.: Lipid, sterols and fatty acid composition of abyssal holoturians and ophiuroids from the North-East Pacific Ocean: food web implications, Comp. Biochem. Phys. B., 151, 79–87, https://doi.org/10.1016/j.cbpb.2008.05.013, 2008a.
Drazen, J. C., Phleger, C. F., Guest, M. A., and Nichols, P. D.: Lipid, sterols and fatty acids of abyssal polychaetes, crustaceans, and a cnidarian from the northeast Pacific Ocean: food web implications, Mar. Ecol. Prog. Ser., 372, 157–167, https://doi.org/10.3354/meps07707, 2008b.
Drazen, J. C., Popp, B. N., Choy, C. A., Clemente, T., Forest, L. D., and Smith, K. L.: Bypassing the abyssal benthic food web: Macrourid diet in the eastern North Pacific inferred from stomach content and stable isotopes analyses, Limnol. Oceanogr., 53, 2644–2654, https://doi.org/10.4319/lo.2008.53.6.2644, 2008c.
Drazen, J. C., Phleger, C. F., Guest, M. A., and Nichols, P. D.: Lipid composition and diet inferences in abyssal macrourids of the eastern North Pacific, Mar. Ecol. Prog. Ser., 387, 1–14, https://doi.org/10.3354/meps08106, 2009.
Eliassen, J. E. and Jobling, M.: Food of the roughhead grenadier, Macrourus berglax, Lacepede in North Norwegian waters, J. Fish Biol., 26, 367–376, https://doi.org/10.1111/j.1095-8649.1985.tb04276.x, 1985.
Fanelli, E., Cartes, J. E., Rumolo, P., and Sprovieri, M.: Food-web structure and trophodynamics of mesopelagic–suprabenthic bathyal macrofauna of the Algerian Basin based on stable isotopes of carbon and nitrogen, Deep-Sea Res. Pt. I., 56, 1504–1520, https://doi.org/10.1016/j.dsr.2009.04.004, 2009.
Fanelli, E., Cartes, J. E., and Papiol, V.: Food web structure of deep-sea macrozooplankton and micronekton off the Catalan slope: insight from stable isotopes, J. Marine Syst., 87, 79–89, https://doi.org/10.1016/j.jmarsys.2011.03.003, 2011a.
Fanelli, E., Papiol, V., Cartes, J. E., Rumolo, P., Brunet, C., and Sprovieri, M.: Food web structure of the epibenthic and infaunal invertebrates on the Catalan slope (NW Mediterranean): evidence from δ13C and δ15N analysis, Deep-Sea Res. Pt. I, 58, 98–109, https://doi.org/10.1016/j.dsr.2010.12.005, 2011b.
Fanelli, E., Papiol, V., Cartes, J. E., Rumolo, P., and López-Pérez, C.: Trophic webs of deep-sea megafauna on mainland and insular slopes of the NW Mediterranean: a comparison by stable isotope analysis, Mar. Ecol. Prog. Ser., 490, 199–221, https://doi.org/10.3354/meps10430, 2013.
FishBase: http://www.fishbase.org, last access: February 2019.
Francois, R., Altabet, M. A., Goericke, R., McCorkle, D. C., Brunet, C., and Poisson, A.: Changes in the δ13C of surface water particulate organic matter across the subtropical convergence in the SW Indian Ocean, Global Biogeochem. Cy., 7, 627–644, https://doi.org/10.1029/93GB01277, 1993.
Gage, J. D.: Food inputs, utilization, carbon flow and energetics, in: Ecosystems of the deep oceans, edited by: Tyler, P. A., Elsevier Science B.V., Amsterdam, tThe Netherlands, 313–382, 2003.
Gale, K. S., Hamel, J.-F., and Mercier, A.: Trophic ecology of deep-sea Asteroidea (Echinodermata) from eastern Canada, Deep-Sea Res. Pt. I, 80, 25–36, https://doi.org/10.1016/j.dsr.2013.05.016, 2013.
Galván, D., Sweeting, C., and Reid, W.: Power of stable isotope techniques to detect size-based feeding in marine fishes, Mar. Ecol. Prog. Ser., 407, 271–278, https://doi.org/10.3354/meps08528, 2010.
Gartner, J. V., Crabtree, R. E., and Sulak, K. J.: Feeding at depth, Fish physiology, 16, 115–193, https://doi.org/10.1016/S1546-5098(08)60229-0, 1997.
Hamoutene, D., Puestow, T., Miller-Banoub, J., and Wareham, V.: Main lipid classes in some species of deep-sea corals in the Newfoundland and Labrador region (Northwest Atlantic Ocean), Coral Reefs, 27, 237–246, https://doi.org/10.1007/s00338-007-0318-7, 2008.
Hetherington, E. D., Olson, R. J., Drazen, J. C., Lennert-Cody, C. E., Balance, L. T., Kaufmann, R. S., and Popp, B. N.: Spatial food-web structure in the eastern tropical Pacific Ocean based on compound-specific nitrogen isotope analysis of amino acids, Limnol. Oceanogr., 62, 541–560, https://doi.org/10.1002/lno.10443, 2017.
Hixson, S. M. and Arts, M. T.: Climate warming is predicted to reduce omega-3, long-chain, polyunsaturated fatty acid production in phytoplankton, Global Change Biol., 22, 2744–2755, https://doi.org/10.1111/gcb.13295, 2016.
Hoffman, J. C. and Sutton, T. T.: Lipid correction for carbon stable isotope analysis of deep-sea fishes, Deep-Sea Res. Pt. I, 57, 956–964, https://doi.org/10.1016/j.dsr.2010.05.003, 2010.
Howell, K. L., Pond, D. W., Billett, D. S., and Tyler, P. A.: Feeding ecology of deep-sea seastars (Echinodermata: Asteroidea): a fatty-acid biomarker approach, Mar. Ecol. Prog. Ser., 255, 193–206, 2003.
Hudson, I. R., Pond, D. W., Billett, D. S., Tyler, P. A., Lampitt, R. S., and Wolff, G. A.: Temporal variations in fatty acid composition of deep-sea holothurians: evidence of bentho-pelagic coupling, Mar. Ecol. Prog. Ser., 281, 109–120, https://doi.org/10.3354/meps281109, 2004.
Hussey, N., MacNeil, M., Olin, J., McMeans, B., Kinney, M., Chapman, D., and Fisk, A.: Stable isotopes and elasmobranchs: tissue types, methods, applications and assumptions, J. Fish Biol., 80, 1449–1484, https://doi.org/10.1111/j.1095-8649.2012.03251.x, 2012.
Iken, K., Brey, T., Wand, U., Voigt, J., and Junghans, P.: Food web structure of the benthic community at the Porcupine Abyssal Plain (NE Atlantic): a stable isotope analysis, Prog. Oceanogr., 50, 383–405, https://doi.org/10.1016/S0079-6611(01)00062-3, 2001.
Iken, K., Bluhm, B., and Gradinger, R.: Food web structure in the high Arctic Canada Basin: evidence from δ13C and δ15N analysis, Polar Biol., 28, 238–249, https://doi.org/10.1007/s00300-004-0669-2, 2005.
IPCC: Fifth assessment report, available at: https://www.ipcc.ch/site/assets/uploads/2018/02/SYR_AR5_FINAL_full.pdf (last access: February 2019), 2014.
Iverson, S. J.: Tracing aquatic food webs using fatty acids: from qualitative indicators to quantitative determination, in: Lipids in aquatic ecosystems, edited by: Arts, M. T., Brett, M. T., and Kainz, M. J., Springer, New York, NY, 281–308, https://doi.org/10.1007/978-0-387-89366-2_12, 2009.
Jeffreys, R. M., Wolff, G. A., and Murty, S. J.: The trophic ecology of key megafaunal species at the Pakistan Margin: evidence from stable isotopes and lipid biomarkers, Deep-Sea Res. Pt. I, 56, 1816–1833, https://doi.org/10.1016/j.dsr.2009.05.001, 2009.
Jones, D. O., Yool, A., Wei, C. L., Henson, S. A., Ruhl, H. A., Watson, R. A., and Gehlen, M.: Global reductions in seafloor biomass in response to climate change, Global Change Biol., 20, 1861–1872, https://doi.org/10.1111/gcb.12480, 2014.
Kharlamenko, V. I., Brandt, A., Kiyashko, S. I., and Würzberg, L.: Trophic relationship of benthic invertebrate fauna from the continental slope of the Sea of Japan, Deep-Sea Res. Pt. II, 86, 34–42, https://doi.org/10.1016/j.dsr2.2012.08.007, 2013.
Kharlamenko, V. I., Maiorova, A. S., and Ermolenko, E. V.: Fatty acid composition as an indicator of the trophic position of abyssal megabenthic deposit feeders in the Kuril Basin of the Sea of Okhotsk, Deep-Sea Res. Pt. II, 154, 374–382, https://doi.org/10.1016/j.dsr2.2018.03.005, 2018.
Kim, S. L. and Koch, P. L.: Methods to collect, preserve, and prepare elasmobranch tissues for stable isotope analysis, Environ. Biol. Fish, 95, 53–63, https://doi.org/10.1007/s10641-011-9860-9, 2012.
Kiyashko, S. I., Kharlamenko, V. I., Sanamyan, K., Alalykina, I. L., and Würzberg, L.: Trophic structure of the abyssal benthic community in the Sea of Japan inferred from stable isotope and fatty acid analyses, Mar. Ecol. Prog. Ser., 500, 121–137, https://doi.org/10.3354/meps10663, 2014.
Kopp, D., Robert, M., and Pawlowski, L.: Characterization of food web structure of the upper continental slope of the Celtic Sea highlighting the trophic ecology of five deep-sea fishes, J. Appl. Ichthyol., 34, 73–80, https://doi.org/10.1111/jai.13544, 2018.
Lampitt, R.: Evidence for the seasonal deposition of detritus to the deep-sea floor and its subsequent resuspension. Deep-Sea Res., 32, 885–897, https://doi.org/10.1016/0198-0149(85)90034-2, 1985.
Larsen, T., Taylor, D. L., Leigh, M. B., and O'Brien, D. M.: Stable isotope fingerprinting: a novel method for identifying plant, fungal, or bacterial origins of amino acids, Ecology, 90, 3526–3535, https://doi.org/10.1890/08-1695.1, 2009.
Lee, R., Nevenzel, J., and Paffenhöfer, G.-A.: Importance of wax esters and other lipids in the marine food chain: phytoplankton and copepods, Mar. Biol., 9, 99–108, https://doi.org/10.1007/BF00348249, 1971.
Lewis, R. W.: Fatty acid composition of some marine animals from various depths, J. Fish Res. Board. Can., 24, 1101–1115, 1967.
Madurell, T., Fanelli, E., and Cartes, J. E.: Isotopic composition of carbon and nitrogen of suprabenthic fauna in the NW Balearic Islands (western Mediterranean), J. Marine Syst., 71, 336–345, https://doi.org/10.1016/j.jmarsys.2007.03.006, 2008.
Martin-Creuzburg, D. and Von Elert, E.: Ecological significance of sterols in aquatic food webs, in: Lipids in aquatic ecosystems, edited by: Kainz, M., Brett, M. T., and Arts, M. T., Springer, New York, NY, 43–64, https://doi.org/10.1007/978-0-387-89366-2_3, 2009.
Mauchline, J. and Gordon, J.: Diets and bathymetric distributions of the macrourid fish of the Rockall Trough, northeastern Atlantic Ocean, Mar. Biol., 81, 107–121, https://doi.org/10.1007/BF00393109, 1984.
McClelland, J. W. and Montoya, J. P.: Trophic relationships and the nitrogen isotopic composition of amino acids in plankton, Ecology, 83, 2173–2180, 2002.
McConnaughey, T. and McRoy, C.: Food-web structure and the fractionation of carbon isotopes in the Bering Sea, Mar. Biol., 53, 257–262, https://doi.org/10.1007/BF00952434, 1979.
Michener, R. H., and Kaufman, L.: Stable isotope ratios as tracers in marine food webs: an update, in: Stable isotopes in ecology and environmental science, edited by: Michener, R. and Lajtha, K., Blackwell Publishing Ltd, Oxford, UK, 238–282, 2007.
Minagawa, M. and Wada, E. Stepwise enrichment of 15N along food chains: further evidence and the relation between δ15N and animal age, Geochim. Cosmochim. Ac., 48, 1135–1140, https://doi.org/10.1016/0016-7037(84)90204-7, 1984.
Mincks, S. L., Smith, C. R., Jeffreys, R. M., and Sumida, P. Y.: Trophic structure on the West Antarctic Peninsula shelf: detritivory and benthic inertia revealed by δ13C and δ15N analysis, Deep-Sea Res. Pt. II, 55, 2502–2514, https://doi.org/10.1016/j.dsr2.2008.06.009, 2008.
Mindel, B. L., Neat, F. C., Trueman, C. N., Webb, T. J., and Blanchard, J. L.: Functional, size and taxonomic diversity of fish along a depth gradient in the deep sea, PeerJ, 4, e2387, https://doi.org/10.7717/peerj.2387, 2016a.
Mindel, B. L., Webb, T. J., Neat, F. C., and Blanchard, J. L.: A trait-based metric sheds new light on the nature of the body size–depth relationship in the deep sea, J. Anim. Ecol., 85, 427–436, https://doi.org/10.1111/1365-2656.12471, 2016b.
Mintenbeck, K., Jacob, U., Knust, R., Arntz, W., and Brey, T.: Depth-dependence in stable isotope ratio δ15N of benthic POM consumers: the role of particle dynamics and organism trophic guild, Deep-Sea Res. Pt. I, 54, 1015–1023, https://doi.org/10.1016/j.dsr.2007.03.005, 2007.
Mordukhovich, V. V., Kiyashko, S. I., Kharlamenko, V. I., and Fadeeva, N. P.: Determination of food sources for nematodes in the Kuril Basin and eastern slope of the Kuril Islands by stable isotope and fatty acid analyses, Deep-Sea Res. Pt. II, 154, 365–373, https://doi.org/10.1016/j.dsr2.2018.01.003, 2018.
Økland, H. M., Stoknes, I. S., Remme, J. F., Kjerstad, M., and Synnes, M.: Proximate composition, fatty acid and lipid class composition of the muscle from deep-sea teleosts and elasmobranchs, Comp. Biochem. Phys. B, 140, 437–443, https://doi.org/10.1016/j.cbpc.2004.11.008, 2005.
Papiol, V., Cartes, J. E., Fanelli, E., and Rumolo, P.: Food web structure and seasonality of slope megafauna in the NW Mediterranean elucidated by stable isotopes: relationship with available food sources, J. Sea Res., 77, 53–69, https://doi.org/10.1016/j.seares.2012.10.002, 2013.
Parrish, C. C.: Essential fatty acids in aquatic food webs, in: Lipids in aquatic ecosystems, edited by: Arts, M. T., Brett, M. T., and Kainz, M. J., Springer New York, New York, NY, 309–326, 2009.
Parrish, C. C.: Lipids in marine ecosystems, ISRN Oceanography, 2013, 604045, https://doi.org/10.5402/2013/604045, 2013.
Parrish, C. C., Abrajano, T., Budge, S., Helleur, R., Hudson, E., Pulchan, K., and Ramos, C.: Lipid and phenolic biomarkers in marine ecosystems: analysis and applications, in: Marine chemistry, edited by: Wangersky, P., Springer-Verlag, Berlin, Heidelberg, 193–223, 2000.
Parzanini, C., Parrish, C. C., Hamel, J.-F., and Mercier, A.: Trophic ecology of a deep-sea fish assemblage in the Northwest Atlantic, Mar. Biol., 164, 206, https://doi.org/10.1007/s00227-017-3236-4, 2017.
Parzanini, C., Parrish, C. C., Hamel, J.-F., and Mercier, A.: Trophic relationships of deep-sea benthic invertebrates on a continental margin in the NW Atlantic inferred by stable isotope, elemental, and fatty acid composition, Prog. Oceanogr., 168, 279–295, https://doi.org/10.1016/j.pocean.2018.10.007, 2018a.
Parzanini, C., Parrish, C. C., Hamel, J.-F., and Mercier, A.: Functional diversity and nutritional content in a deep-sea faunal assemblage through total lipid, lipid class, and fatty acid analyses, PLoS One, 13, e0207395, https://doi.org/10.1371/journal.pone.0207395, 2018b.
Pétursdóttir, H., Gislason, A., and Falk-Petersen, S.: Lipid classes and fatty acid compositions of muscle, liver and skull oil in deep-sea redfish Sebastes mentella over the Reykjanes Ridge, J. Fish Biol., 73, 2485–2496, https://doi.org/10.1111/j.1095-8649.2008.02100.x, 2008a.
Pétursdóttir, H., Gislason, A., Falk-Petersen, S., Hop, H., and Svavarsson, J.: Trophic interactions of the pelagic ecosystem over the Reykjanes Ridge as evaluated by fatty acid and stable isotope analyses, Deep-Sea Res. Pt. II, 55, 83–93, https://doi.org/10.1016/j.dsr2.2007.09.003, 2008b.
Pfannkuche, O.: Allochthonous deep-sea benthic communities: functioning and forcing, in: Interactions between macro-and microorganisms in marine sediments, edited by: Kristensen, E., Ralf, R., and Kostka, E. J., American Geophysical Union, Washington DC, 251–266, 2005.
Pinnegar, J. and Polunin, N. Differential fractionation of δ13C and δ15N among fish tissues: implications for the study of trophic interactions, Funct. Ecol., 13, 225–231, https://doi.org/10.1046/j.1365-2435.1999.00301.x, 1999.
Polunin, N., Morales-Nin, B., Pawsey, W., Cartes, J., Pinnegar, J., and Moranta, J.: Feeding relationships in Mediterranean bathyal assemblages elucidated by stable nitrogen and carbon isotope data, Mar. Ecol. Prog. Ser., 220, 13–23, https://doi.org/10.3354/meps220013, 2001.
Post, D. M., Layman, C. A., Arrington, D. A., Takimoto, G., Quattrochi, J., and Montana, C. G.: Getting to the fat of the matter: models, methods and assumptions for dealing with lipids in stable isotope analyses, Oecologia, 152, 179–189, https://doi.org/10.1007/s00442-006-0630-x, 2007.
Preciado, I., Cartes, J. E., Punzón, A., Frutos, I., López-López, L., and Serrano, A.: Food web functioning of the benthopelagic community in a deep-sea seamount based on diet and stable isotope analyses, Deep-Sea Res. Pt. II, 137, 56–68, https://doi.org/10.1016/j.dsr2.2016.07.013, 2017.
Quiroga, E., Gerdes, D., Montiel, A., Knust, R., and Jacob, U.: Normalized biomass size spectra in high Antarctic macrobenthic communities: linking trophic position and body size, Mar. Ecol. Prog. Ser., 506, 99–113, https://doi.org/10.3354/meps10807, 2014.
Ramirez-Llodra, E., Brandt, A., Danovaro, R., De Mol, B., Escobar, E., German, C. R., Levin, L. A., Martinez Arbizu, P., Menot, L., Buhl-Mortensen, P., Narayanaswamy, B. E., Smith, C. R., Tittensor, D. P., Tyler, P. A., Vanreusel, A., and Vecchione, M.: Deep, diverse and definitely different: unique attributes of the world's largest ecosystem, Biogeosciences, 7, 2851–2899, https://doi.org/10.5194/bg-7-2851-2010, 2010.
Rau, G. H. and Hedges, J. I.: Carbon-13 depletion in a hydrothermal vent mussel: suggestion of a chemosynthetic food source, Science, 203, 648–649, https://doi.org/10.1126/science.203.4381.648, 1979.
Rau, G. H., Sweeney, R., and Kaplan, I.: Plankton 13C : 12C ratio changes with latitude: differences between northern and southern oceans, Deep-Sea Res., 29, 1035–1039, https://doi.org/10.1016/0198-0149(82)90026-7, 1982.
Reid, W. D., Wigham, B. D., McGill, R. A., and Polunin, N. V.: Elucidating trophic pathways in benthic deep-sea assemblages of the Mid-Atlantic Ridge north and south of the Charlie-Gibbs Fracture Zone, Mar. Ecol. Prog. Ser., 463, 89–103, https://doi.org/10.3354/meps09863, 2012.
Reid, W. D., Sweeting, C. J., Wigham, B. D., McGill, R. A., and Polunin, N. V.: High variability in spatial and temporal size-based trophodynamics of deep-sea fishes from the Mid-Atlantic Ridge elucidated by stable isotopes, Deep-Sea Res. Pt. II, 98, 412–420, https://doi.org/10.1016/j.dsr2.2013.01.020, 2013.
Rex, M. A., Stuart, C. T., Hessler, R. R., Allen, J. A., Sanders, H. L., and Wilson, G. D.: Global-scale latitudinal patterns of species diversity in the deep-sea benthos, Nature, 365, 636–639, https://doi.org/10.1038/365636a0, 1993.
Richards, T. M., Gipson, E. E., Cook, A., Sutton, T. T., and Wells, R. D.: Trophic ecology of meso-and bathypelagic predatory fishes in the Gulf of Mexico, ICES J. Mar. Sci, 76, 662–672, https://doi.org/10.1093/icesjms/fsy074, 2019.
Rieger, R. M.: The biphasic life cycle – a central theme of metazoan evolution, Am. Zool., 34, 484–491, https://doi.org/10.1093/icb/34.4.484, 1994.
Rossoll, D., Bermúdez, R., Hauss, H., Schulz, K. G., Riebesell, U., Sommer, U., and Winder, M.: Ocean acidification-induced food quality deterioration constrains trophic transfer, PLoS One, 7, e34737, https://doi.org/10.1371/journal.pone.0034737, 2012.
Sackett, W. M., Eckelmann, W. R., Bender, M. L., and Bé, A. W.: Temperature dependence of carbon isotope composition in marine plankton and sediments, Science, 148, 235–237, https://doi.org/10.1126/science.148.3667.235, 1965.
Saito, H. and Osako, K.: Confirmation of a new food chain utilizing geothermal energy: unusual fatty acids of a deep-sea bivalve, Calyptogena phaseoliformis, Limnol. Oceanogr., 52, 1910–1918, https://doi.org/10.4319/lo.2007.52.5.1910, 2007.
Salvo, F., Hamoutene, D., Hayes, V. E. W., Edinger, E. N., and Parrish, C. C.: Investigation of trophic ecology in Newfoundland cold-water deep-sea corals using lipid class and fatty acid analyses, Coral Reefs, 37, 157–171, https://doi.org/10.1007/s00338-017-1644-z, 2018.
Sherwood, O. A., Jamieson, R. E., Edinger, E. N., and Wareham, V. E.: Stable C and N isotopic composition of cold-water corals from the Newfoundland and Labrador continental slope: examination of trophic, depth and spatial effects, Deep-Sea Res. Pt. I, 55, 1392–1402, https://doi.org/10.1016/j.dsr.2008.05.013, 2008.
Shi, L., Xiao, W., Liu, Z., Pan, B., and Xu, Y.: Diet change of hadal amphipods revealed by fatty acid profile: a close relationship with surface ocean, Mar. Environ. Res., 142, 250-256, https://doi.org/10.1016/j.marenvres.2018.10.012, 2018.
Shine, R.: Ecological causes for the evolution of sexual dimorphism: a review of the evidence, Q. Rev. Biol., 64, 419–461, 1989.
Shipley, O. N., Brooks, E. J., Madigan, D. J., Sweeting, C. J., and Grubbs, R. D.: Stable isotope analysis in deep-sea chondrichthyans: recent challenges, ecological insights, and future directions, Rev. Fish Biol. Fisher., 27, 481–497, https://doi.org/10.1007/s11160-017-9466-1, 2017.
Smith, C. R. and Baco, A. R.: Ecology of whale falls at the deep-sea floor, Oceanogr. Mar. Biol. Ann. Rev., 41, 311–354, 2003.
Smith, K. L., Ruhl, H. A., Bett, B. J., Billett, D. S., Lampitt, R. S., and Kaufmann, R. S.: Climate, carbon cycling, and deep-ocean ecosystems, P. Natl. Acad. Sci. USA, 106, 19211–19218, https://doi.org/10.1073/pnas.0908322106, 2009.
Stowasser, G., McAllen, R., Pierce, G., Collins, M., Moffat, C., Priede, I., and Pond, D. W.: Trophic position of deep-sea fish – assessment through fatty acid and stable isotope analyses, Deep-Sea Res. Pt. I, 56, 812–826, https://doi.org/10.1016/j.dsr.2008.12.016, 2009.
Strebe: The world on Winkel tripel projection, 15∘ graticule, Imagery is a derivative of NASA's Blue Marble summer month composite with oceans lightened to enhance legibility and contrast, Image created with the Geocart map projection software, Wikimedia Commons (CC-BY-SA 3.0), available at: https://en.wikipedia.org/wiki/File:Winkel_triple_projection_SW.jpg (last access: 5 July 2019), 2011.
Stuart, C. T., Rex, M., and Etter, R. J.: Large-scale spatial and temporal patterns of deep-sea benthic species diversity, in: Ecosystems of the deep oceans, edited by: Tyler, P. A., Elsevier, the Netherlands, 295–312, 2003.
Sulzman, E. W.: Stable isotope chemistry and measurement: a primer, in: Stable isotopes in ecology and environmental science, edited by: Michener, R., and Lajtha, K., Blackwell Publishing Ltd., Oxford, UK, 1–21, 2007.
Sweetman, A. K., Thurber, A. R., Smith, C. R., Levin, L. A., Mora, C., Wei, C. L., Gooday, A. J., Jones, D. O., Rex, M., Yasuhara, M., and Ingels, J.: Major impacts of climate change on deep-sea benthic ecosystem, Elementa-Sci. Anthrop., 5, 4, https://doi.org/10.1525/elementa.203, 2017.
Syväranta, J., Martino, A., Kopp, D., Céréghino, R., and Santoul, F.: Freezing and chemical preservatives alter the stable isotope values of carbon and nitrogen of the Asiatic clam (Corbicula fluminea), Hydrobiologia, 658, 383–388, https://doi.org/10.1007/s10750-010-0512-4, 2011.
Tecchio, S., van Oevelen, D., Soetaert, K., Navarro, J., and Ramírez-Llodra, E.: Trophic dynamics of deep-sea megabenthos are mediated by surface productivity, PloS One, 8, e63796, https://doi.org/10.1371/journal.pone.0063796, 2013.
Trueman, C., Johnston, G., O'Hea, B., and MacKenzie, K.: Trophic interactions of fish communities at midwater depths enhance long-term carbon storage and benthic production on continental slopes, Proc. R. Soc. B, 281, 20140669, https://doi.org/10.1098/rspb.2014.0669, 2014.
Valls, M., Olivar, M. P., de Puelles, M. F., Molí, B., Bernal, A., and Sweeting, C. J.: Trophic structure of mesopelagic fishes in the western Mediterranean based on stable isotopes of carbon and nitrogen, J. Marine Syst., 138, 160–170, https://doi.org/10.1016/j.jmarsys.2014.04.007, 2014a.
Valls, M., Sweeting, C., Olivar, M., de Puelles, M. F., Pasqual, C., Polunin, N., and Quetglas, A.: Structure and dynamics of food webs in the water column on shelf and slope grounds of the western Mediterranean, J. Marine Syst., 138, 171–181, https://doi.org/10.1016/j.jmarsys.2014.04.002, 2014b.
van Oevelen, D., Duineveld, G. C., Lavaleye, M. S., Kutti, T., and Soetaert, K.: Trophic structure of cold-water coral communities revealed from the analysis of tissue isotopes and fatty acid composition, Mar. Biol. Res., 14, 287–306, https://doi.org/10.1080/17451000.2017.1398404, 2018.
WoRMS Editorial Board: World Register of Marine Species, available at: http://www.marinespecies.org/, last access: February 2019.
Würzberg, L., Peters, J., and Brandt, A.: Fatty acid patterns of Southern Ocean shelf and deep sea peracarid crustaceans and a possible food source, foraminiferans, Deep-Sea Res. Pt. II, 58, 2027–2035, https://doi.org/10.1016/j.dsr2.2011.05.013, 2011a.
Würzberg, L., Peters, J., Flores, H., and Brandt, A.: Demersal fishes from the Antarctic shelf and deep sea: A diet study based on fatty acid patterns and gut content analyses, Deep-Sea Res. Pt. II, 58, 2036–2042, https://doi.org/10.1016/j.dsr2.2011.05.012, 2011b.
Würzberg, L., Peters, J., Schüller, M., and Brandt, A.: Diet insights of deep-sea polychaetes derived from fatty acid analyses, Deep-Sea Res. Pt. II, 58, 153–162, https://doi.org/10.1016/j.dsr2.2010.10.014, 2011c.
Xu, J., Yang, Q., Zhang, M., Zhang, M., Xie, P., and Hansson, L-A.: Preservation effects on stable isotope ratios and consequences for the reconstruction of energetic pathways, Aquat. Ecol., 45, 483–492, https://doi.org/10.1007/s10452-011-9369-5, 2011.
- Article
(1269 KB) - Full-text XML