the Creative Commons Attribution 4.0 License.
the Creative Commons Attribution 4.0 License.
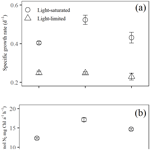
Light availability modulates the effects of warming in a marine N2 fixer
Xiangqi Yi
Fei-Xue Fu
David A. Hutchins
Trichodesmium species, as a group of photosynthetic N2 fixers (diazotrophs), play an important role in the marine biogeochemical cycles of nitrogen and carbon, especially in oligotrophic waters. How ongoing ocean warming may interact with light availability to affect Trichodesmium is not yet clear. We grew Trichodesmium erythraeum IMS 101 at three temperature levels of 23, 27, and 31∘C under growth-limiting and growth-saturating light levels of 50 and 160 µmol quanta m−2 s−1, respectively, for at least 10 generations and then measured physiological performance, including the specific growth rate, N2 fixation rate, and photosynthesis. Light availability significantly modulated the growth response of Trichodesmium to temperature, with the specific growth rate peaking at ∼27∘C under the light-saturating conditions, while growth of light-limited cultures was non-responsive across the tested temperatures (23, 27, and 31∘C). Short-term thermal responses for N2 fixation indicated that both high growth temperature and light intensity increased the optimum temperature (Topt) for N2 fixation and decreased its susceptibility to supra-optimal temperatures (deactivation energy – Eh). Simultaneously, all light-limited cultures with low Topt and high Eh were unable to sustain N2 fixation during short-term exposure to high temperatures (33–34∘C) that are not lethal for the cells grown under light-saturating conditions. Our results imply that Trichodesmium spp. growing under low light levels while distributed deep in the euphotic zone or under cloudy weather conditions might be less sensitive to long-term temperature changes that occur on the timescale of multiple generations but are more susceptible to abrupt (less than one generation time span) temperature changes, such as those induced by cyclones and heat waves.
- Article
(508 KB) - Full-text XML
-
Supplement
(434 KB) - BibTeX
- EndNote
In vast areas of the ocean, primary production is usually limited by availability of nitrogen (Moore et al., 2013). In addition to recycling within the euphotic zone, biologically available nitrogen sources can be supplied to phytoplankton from upwelling, aerosol deposition, and N2 fixation by diazotrophic prokaryotes, supporting new primary production (Dugdale and Goering, 1967). Trichodesmium is one of the major diazotrophic organisms occurring in the pelagic oceans (Zehr, 2011). Trichodesmium is a genus of filamentous cyanobacteria that exists as both single filaments and colonies consisting of tens to hundreds of trichomes and that is broadly distributed in oligotrophic tropical and subtropical oceans (Capone et al., 1997). Its contribution to local new production can even be more important than that of nitrate diffusion in some regions (Capone et al., 2005; LaRoche and Breitbarth, 2005; Mahaffey et al., 2005), and it thus plays a significant role in marine ecosystems and biogeochemical cycles of nitrogen and carbon (Sohm et al., 2011; Zehr, 2011).
Trichodesmium has attracted tremendous research interest since its discovery as a diazotroph in the early 1960s (Dugdale et al., 1961, 1964). Recently, considerable research attention has been focused on evaluating effects of the ongoing ocean climate change, including sea surface warming associated with global warming, on this keystone organism (Fu et al., 2014; Hutchins and Fu, 2017; Jiang et al., 2018; Hutchins et al., 2007). The IPCC RCP8.5 scenario predicts that upper-ocean temperature will increase by about 3∘C on average by the end of the 21st century, and the strongest ocean warming will happen in tropical and subtropical regions (Collins et al., 2013). Because of its important role in marine biogeochemical cycles and marine ecosystems, understanding the responses of Trichodesmium to ocean warming and their underlying mechanisms will be critical to evaluating the potential implications of climate change on marine primary productivity, food web dynamics, and biogeochemical cycles.
Previous studies demonstrate that without resource limitation the growth-versus-temperature curve is unimodal in Trichodesmium, with lower and upper tolerance limits separately at 18–20 and 32–34∘C and optimum temperature at 26–28∘C (Breitbarth et al., 2007; Chappell and Webb, 2010; Fu et al., 2014). Based on these findings and the spatial heterogeneity of present temperatures and projected warming of Trichodesmium's habitat (Capone et al., 1997; Collins et al., 2013), the effects of ocean warming on Trichodesmium can be spatially diverse, generally benefiting those occurring in relatively high latitudes but being harmful to those occurring near to the Equator (Breitbarth et al., 2007; Fu et al., 2014; Thomas et al., 2012). However, this pattern can be distorted and complicated by resource limitations. For example, it is shown that iron limitation, which is commonly experienced by Trichodesmium in nature (Hutchins and Boyd, 2016; Sohm et al., 2011), substantially increases the optimum temperature in Trichodesmium (Jiang et al., 2018).
Similar to iron availability, light is also among the key environmental drivers for Trichodesmium (Breitbarth et al., 2008; Cai and Gao, 2015; Cai et al., 2015). Trichodesmium can be distributed from the sea surface down to 150 m depth, where light intensity at noon ranges from > 2000 to < 10 µmol quanta m−2 s−1 (Davis and McGillicuddy, 2006; Olson et al., 2015). Moreover, Trichodesmium is known to be able to partially regulate its vertical position in the water column by buoyancy adjustment (Villareal and Carpenter, 2003). Currently, ocean warming effects on Trichodesmium have been widely examined under single, light-saturating conditions, providing important knowledge on this diazotroph's physiological responses to temperature changes (Breitbarth et al., 2007; Fu et al., 2014; Jiang et al., 2018; Levitan et al., 2010). However, how it responds to warming under both light-limiting and light-saturating conditions is also of general significance but has been rarely studied (Boatman et al., 2017).
The growth of phytoplankton is a holistic result of many biochemical and physiological activities, so its responses to environmental changes are dependent on species-specific physiology. Normally, warming increases enzyme activities, accelerating biochemical reactions (Gillooly et al., 2001). For phytoplankton, reduced growth at high temperatures might be the result of less carbon availability due to the higher thermal sensitivity of respiration compared to that of photosynthesis (Padfield et al., 2015). This negative effect of high temperatures might be exacerbated by direct reduction of photosynthesis under light limitation. In the diazotroph Trichodesmium, besides photosynthesis and respiration, the N2 fixation process might also play a critical role in its growth response to environmental changes. Little has been documented on this aspect (Jiang et al., 2018).
In this study, we explored the combined effects of temperature and light in Trichodesmium erythraeum IMS 101. We measured the specific growth rate, photosystem functions, and N2 fixation rate in Trichodesmium cultures acclimated to two light levels and three temperatures. Moreover, we measured their short-term thermal responses for N2 fixation. In this paper, “acclimation” and “acclimated” indicate that cultures were given enough time (several weeks) to respond to the environmental changes so that balanced growth was achieved, and “short term” refers to acute processes and changes occurring within hours. Although adaptation (over several hundreds of generations) has been demonstrated to be critical in evaluating the responses in phytoplankton to environmental changes (Hutchins et al., 2015; Li et al., 2017; Padfield et al., 2015; Schaum et al., 2018; Tong et al., 2018), it is beyond the scope of this study.
2.1 Culture conditions
Triplicate cultures of Trichodesmium erythraeum (strain IMS101, originally isolated from the North Atlantic Ocean by Prufert-Bebout et al., 1993) were established under six different culture conditions. These included factorial combinations of three temperatures (23±1, 27±1, and 31±1∘C) and two light intensities (light saturating, 160±20 µmol quanta m−2 s−1, and light limiting, 50±6 µmol quanta m−2 s−1). These three growth temperatures are representatives of present and future temperatures of Trichodesmium habitats (Breitbarth et al., 2007; Fu et al., 2014). The light-limiting and light-saturating levels were established based on a pilot experiment (Fig. S1a in the Supplement) and previous studies (Breitbarth et al., 2008; Cai et al., 2015; Garcia et al., 2011). All the cultures were run semi-continuously by continual dilutions (every 2–4 d) in the artificial seawater medium YBCII without combined nitrogen (Chen et al., 1996) in 1 L glass flasks maintained in plant growth chambers (HP300G–C, Ruihua, China). Light was provided by LED tubes (FSL, China) with a 12:12 light : dark cycle. Different levels of light intensity were achieved using neutral density filters. Cultures were continuously bubbled with air (outdoor) so that the cyanobacteria floated as single filaments. The cells were allowed to acclimate to each condition for at least 10 generations. Acclimation was confirmed by balanced growth with a stable specific growth rate. Then, we started the sampling and data collection.
2.2 Chlorophyll a (Chl a) concentration and specific growth rate
Chl a concentration was spectrophotometrically quantified by gently filtering the cells onto glass-fiber filters (GF/F, Whatman), followed by extraction in pure methanol at 4∘C for 24 h and centrifugation at 6000 g for 10 min. The absorbance spectrum of supernatant was determined using a spectrophotometer (DU800, Beckman, USA). Chl a concentration was calculated as [Chl a] (microgram per milliliter) = 12.9447 × (A665−A750), where A665 and A750 were, respectively, the absorbances at 665 and 750 nm (Ritchie, 2006).
Because Chl a concentration is a good proxy for biomass in Trichodesmium (Breitbarth et al., 2007), Chl a concentrations measured on different days were analyzed using Eq. (1) to obtain the specific growth rate:
where Chl a(t) is the Chl a concentration at time t (d), µ is the specific growth rate (d−1), and b is interpreted as the natural logarithm of Chl a concentration at day 0. Because the cultures were semi-continuously maintained, Chl a concentrations at each time point were corrected by the dilution ratios with the assumption of no dilution.
2.3 Short-term thermal response for N2 fixation
N2 fixation rates were determined using the acetylene reduction assay assuming a ratio of 4:1 to convert ethylene production to N2 fixation (Capone, 1993). To examine the responses of N2 fixation in the cells grown at different temperatures and light levels to short-term temperature changes, we simultaneously measured N2 fixation at five temperatures, ranging from 19 to 35∘C. For each replicate, a 25 mL aliquot of the culture was taken and dispensed into five vials. Five vials, each containing a 5 mL culture, were separately placed in five different zones of two multi-zone culture chambers (HP100–2 and HP100–3, Ruihua, China) and allowed to equilibrate to different target temperatures for 30 min. Pilot experiments had showed that 30 min was enough for temperature equilibrium. After temperature equilibration, each vial was spiked with 1 mL (12.5 % of headspace volume) pure acetylene and incubated for another 30 min under the growth light level. The quantity of ethylene produced was determined using a gas chromatograph with a flame ionization detector (Clarus 580, PerkinElmer, USA).
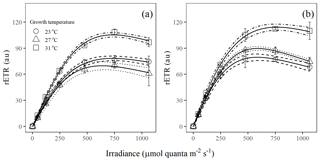
Figure 2Light response curves of rETR in Trichodesmium populations grown under (a) light-saturating and (b) light-limiting conditions (au – arbitrary units). Values represent the mean ± standard deviation of biological replicates (n=3). Solid lines illustrate the best fit to Eq. (4), with 95 % confidence intervals as dashed lines.
Typically, the short-term thermal response curves for N2 fixation were unimodal and negatively skewed, which could be accommodated to a modified version (Padfield et al., 2015; Schaum et al., 2018) of the Sharpe–Schoolfield model (Schoolfield et al., 1981; Sharpe and DeMichele, 1977):
where N(T) is the N2 fixation rate per unit of Chl a (µmol N2 mg Chl a−1 h−1) at temperature T (kelvin – K), Ea is the activation energy (electron volt – eV) for N2 fixation, being indicative of the steepness of the slope leading to a thermal optimum, Eh is the deactivation energy (electron volt – eV) characterizing high-temperature-induced inactivation above the deactivation temperature Th (K), k is Boltzmann's constant ( eV K−1), and N(Tc) is the N2 fixation rate at an arbitrary reference temperature Tc (here, Tc=25∘C) used for normalization. According to Eq. (2), the optimum temperature (Topt K) corresponding to the maximal N2 fixation rate (Nmax, µmol N2 mg Chl a−1 h−1) is
Additionally, we also obtained the N2 fixation rate at the growth temperature (Ngrowth) by inserting corresponding growth temperatures into Eq. (2).
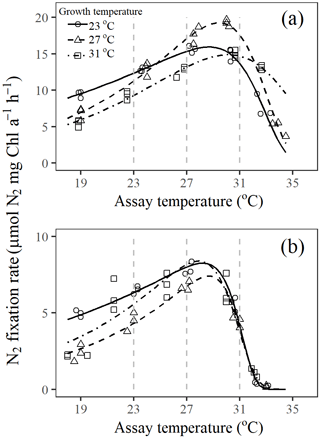
Figure 3Short-term thermal response curves for N2 fixation rate in Trichodesmium cultures grown under (a) light-saturating and (b) light-limiting conditions. Fitted lines are based on fixed-effect coefficients of the nonlinear mixed-effects model fit to Eq. (2). Vertical dotted lines mark the assay temperatures 23, 27, and 31∘C.
2.4 Chl a fluorometry
Photosystem II (PSII) effective quantum yield (ΦPSII) and the photosynthetic relative electron transport rate (rETR) were measured by using the Multiple Excitation Wavelength Chlorophyll Fluorescence Analyzer (MULTI-COLOR-PAM, Walz, Germany) equipped with the US-T temperature control unit (Walz, Germany). Aliquots of 1.5 mL of the culture were taken to determine effective quantum yield (ΦPSII) under actinic light levels that were the same as those of the growth conditions. Then, ΦPSII values were successively measured at seven levels of light intensity (E), ranging from 0 to 1064 µmol quanta m−2 s−1. Samples were allowed to acclimate to each light level for 3 min before ΦPSII measurements were taken (Suggett et a., 2007). The relative electron transport rate (rETR) at each light level was calculated as rETR = E×ΦPSII (Ralph and Gademann, 2005). The light response curve of rETR was analyzed according to the model of Eilers and Peeters (1988):
Photosynthetic parameters, including the photosynthetic light-harvesting efficiency (α), rETR maximum (rETRmax), and light saturation point (Ek), can be calculated as
During the measurements, the sample temperature was maintained at the corresponding growth temperatures using the US-T temperature control unit (Walz, Germany).
2.5 Statistical analyses
Statistical analyses were performed with the R language (version 3.5.3). [Chl a] versus time in each of the triplicate cultures for each treatment was fitted to Eq. (1) using function “lm” in the package “stats” to get the specific growth rate (μ). The significance of differences in specific growth rate (μ) between treatments was tested using the two-way analysis of variance (ANOVA; function “aov” in the package “stats”), followed by Tukey's test for pairwise comparison (function “TukeyHSD” in the package “stats”). The homogeneity of variance assumption and the residual normality assumption were separately checked by Levene's test (function “leveneTest” in the package “stats”) and the Shapiro–Wilk test (function “shapiro.test” in the package “stats”). The significance level was set to 0.05.
To test whether parameters N(Tc), Ea, Eh, Th, and Topt differ between different treatments, we fitted short-term thermal responses for N2 fixation to Eq. (2) using nonlinear mixed-effects models (“nlme” package). Models included random effects on each of the parameters of Eq. (2) by replicate cultures. The structure of the fixed effects of the nonlinear mixed effects model was determined by trying all possible models (625 models) and selecting the one with the lowest small sample-size corrected Akaike information criterion (AICc; see Supplement Table S3 for all tested models' AICc). The AICc was calculated using function “AICc” in the package “MuMIn”.
The light curve of rETR in each of the triplicate cultures for each treatment was fitted to Eq. (4) using function “nls” in the package “stats”. The significance of differences in the photosynthetic light-harvesting efficiency (α), rETR maximum (rETRmax), light saturation point (Ek), and effective quantum yield (ΦPSII) between treatments was tested using the same statistical methods as those for μ. The significance level was set to 0.05.
3.1 Specific growth rate and N2 fixation rate
Specific growth rates of Trichodesmium IMS 101 were significantly affected by growth light intensity (two-way ANOVA, , P< 0.001), growth temperature (two-way ANOVA, , P< 0.001), and the interaction between these two drivers (two-way ANOVA, , P< 0.001; Table 1). High growth light intensity increased specific growth rates of Trichodesmium IMS 101 by 63 % at 23∘C (Tukey's test comparing light-saturated and light-limited growth rates at 23 ∘C, P< 0.001), 111 % at 27∘C (Tukey's test comparing light-saturated and light-limited growth rates at 27∘C, P< 0.001), and 88 % at 31∘C (Tukey's test comparing light-saturated and light-limited growth rates at 31∘C, P< 0.001; Fig. 1a). The interaction between growth light intensity and temperature in the specific growth rate was indicated by the totally different temperature effects between light-saturated and light-limited cultures. Light-saturated growth rates of Trichodesmium IMS 101 were maximal at 27∘C, with a value of 0.52±0.02 d−1 (±SD), being higher by 29.5 % (Tukey's test comparing growth rates between light-saturated cultures grown at 27 and 23∘C, P< 0.001) and 21.3 % (Tukey's test comparing growth rates between light-saturated cultures grown at 27 and 31∘C, P< 0.001) than those at 23 and 31∘C, respectively. However, light-limited growth rates ranged from 0.23±0.02 d−1 (±SD) to 0.25±0.01 d−1 (±SD), with no significant differences between the tested temperatures (Tukey's test comparing growth rates among light-limited cultures grown at three temperatures, P>0.05 for all three comparisons).
Table 1Results of two-way ANOVA for specific growth rate, N2 fixation rate, effective quantum yield, and parameters derived from rETR light curve with interactions between temperature and light. See Table S2 for results of pairwise comparisons.
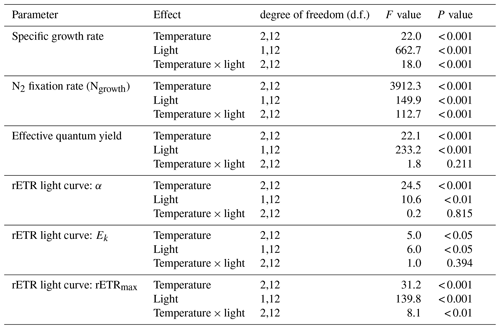
Overall, N2 fixation rates at the growth temperature (Ngrowth; Fig. 1b) were significantly higher in cultures grown under high light intensity compared to those grown under low light intensity (two-way ANOVA, , P< 0.001). Also, the growth temperature significantly affected Ngrowth (two-way ANOVA, , P< 0.001). Different thermal effects between light-saturated and light-limited cultures indicated a significant interaction between light and temperature in Ngrowth (two-way ANOVA, , P< 0.001). For light-saturated cultures, the Ngrowth peaked at 27∘C, with a value of 17.1±0.5 µmol N2 mg Chl a−1 h−1 (±SD), which was higher by 39 % and 17 % than those at 23∘C (Tukey's test comparing Ngrowth between light-saturated cultures grown at 27 and 23∘C, P< 0.001) and 31∘C (Tukey's test comparing Ngrowth between light-saturated cultures grown at 27 and 23∘C, P< 0.001), respectively. However, for light-limited cultures, the value of Ngrowth at 27∘C (6.8±0.2 µmol N2 mg Chl a−1 h−1, ±SD) was similar to that at 23∘C (6.3±0.1 µmol N2 mg Chl a−1 h−1, ±SD; Tukey's test comparing Ngrowth between light-limited cultures grown at 27 and 23∘C, P=0.54) but significantly higher than that at 31∘C (3.8±0.4 µmol N2 mg Chl a−1 h−1, ±SD; Tukey's test comparing Ngrowth between light-limited cultures grown at 27 and 31∘C, P< 0.001).
3.2 PSII effective quantum yield (ΦPSII) and rETR light response curves
Compared to light-saturated cells, light-limited cells had higher values of ΦPSII (Fig. 1c; two-way ANOVA, , P< 0.001). Meanwhile, under both light regimes, ΦPSII in Trichodesmium cultures grown at 31∘C was significantly higher than that in cultures grown at 23 and 27∘C (two-way ANOVA, , P< 0.001). No interaction between growth light intensity and temperature in ΦPSII was found (two-way ANOVA, , P=0.211).
The rETR light response curve of Trichodesmium IMS 101 was influenced by growth temperature in both light-saturated (Fig. 2a) and light-limited (Fig. 2b) treatments. This thermal impact was mainly reflected in the rETRmax (two-way ANOVA, , P< 0.001), which tended to be higher in cultures acclimated to 31∘C than those in cultures acclimated to 23∘C or 27∘C (Table 2; Fig. 2). Additionally, high growth light intensity tended to decreased the rETRmax (two-way ANOVA, , P< 0.001), especially for cultures grown at 27∘C (Tukey's test comparing rETRmax between light-saturated and light-limited cultures grown at 27∘C, P< 0.001). Both high light intensity (two-way ANOVA, , P< 0.05) and high temperatures (two-way ANOVA, , P< 0.05) significantly increased the Ek (Table 2).
Table 2The light-harvesting efficiency (α), relative election transport rate maximum (rETRmax), and light saturation point (Ek), derived from the light curves (Fig. 2), for Trichodesmium grown at different temperature and light intensity levels. Values represent the mean ± standard deviation of biological replicates (n=3). Superscripts with different letters represent significant difference (Turkey's test; more details in Table S2; p< 0.05) among the treatments. The units of Ek and rETRmax are micromoles of quanta per square meter per second and arbitrary unit, respectively.
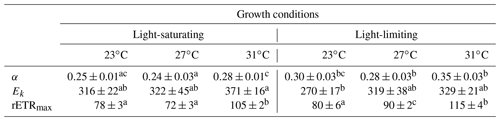
3.3 Short-term thermal response for N2 fixation
Optimum temperature (Topt) for N2 fixation in Trichodesmium IMS 101 was affected by both growth temperature and light intensity (Table 3). Generally, Topt for N2 fixation in light-saturated cultures was higher than that in light-limited cultures, whereas warming effects on N2 fixation Topt differed between light-saturated and light-limited cultures. For light-saturated cultures, elevations of the growth temperature raised the Topt for N2 fixation. A 4∘C warming was accompanied by a 0.5–0.8∘C increase in Topt, which was 28.7±0.2∘C (±SEM, standard error of the mean), 29.5±0.2∘C (±SEM), and 30.0±0.3∘C (±SEM) for the cells grown at 23, 27, and 31∘C, respectively. Under light-limiting levels, Topt in cultures grown at 27∘C (∘C, ±SEM) was higher than that in cultures grown at 23∘C (∘C, ±SEM), but Topt in cultures grown at 31∘C (∘C, ±SEM) was the lowest among all treatments. As expected, the maximal N2 fixation rate (Nmax) in light-saturated cultures was higher than that in light-limited cultures (Table 3). The temperature effect on Nmax was also dependent on the light availability. Light-saturated Nmax was highest in cultures grown at 27∘C (N µmol N2 mg Chl a−1 h−1, ±SEM), being higher by 21 % and 32 % than those grown at 23∘C (N µmol N2 mg Chl a−1 h−1, ±SEM) and 31∘C (N µmol N2 mg Chl a−1 h−1, ±SEM), respectively. However, Nmax for light-limited cultures was similar among different temperature treatments (Table 3).
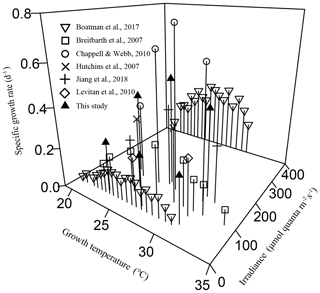
Figure 4The combined effects of temperature and light intensity on the specific growth rate in Trichodesmium IMS 101, with data from the literature involving at least two growth temperatures and this study.
The value of deactivation energy (Eh) for N2 fixation, reflecting the thermal susceptibility to supra-optimal temperatures, was affected by both light availability and growth temperature but not by their interaction (Table 3). Eh tended to be lower in Trichodesmium cultures grown under high temperatures and high light intensity. With the highest Topt (30.0±0.3∘C, ±SEM) and the lowest Eh (1.47±0.14 eV, ±SEM) among all treatments, light-saturated cultures acclimated to 31∘C were the only cultures that could maintain considerable N2 fixation rates at assay temperatures as high as 34∘C (Fig. 3). In addition, both light availability and growth temperature affected the deactivation temperature (Th) for N2 fixation in Trichodesmium, and no interaction between these two drivers was found on Th (Table 3). Th in light-saturated cultures tended to be higher than that in light-limited cultures regardless of the growth temperature. Th was lower in cultures grown at 31∘C compared to that in cultures grown at 23 or 27∘C under both light levels. The activation energy (Ea) for N2 fixation was affected by growth temperature but not by growth light intensity (Table 3). The values of Ea for N2 fixation increased from 0.49±0.04 eV (±SEM) to 0.91±0.05 (±SEM) and 1.07±0.04 eV (±SEM) as growth temperatures increased from 23 to 27 and 31 ∘C.
In this study, light availability not only affected growth rate and N2 fixation directly but also modulated their responses to temperature changes in Trichodesmium IMS 101. Reduced energy supply due to light limitation leads to lowered nitrogen fixation and thus reduced growth in the diazotroph. The specific growth rate was maximal at 27∘C for cells grown under saturating light but was virtually insensitive to temperature changes across the tested temperature (23, 27, and 31∘C) for light-limited cultures.
The interactions between temperature and light on Trichodesmium demonstrated in this work are relevant to natural light and temperature variations and to Trichodesmium global change physiology and biogeography. Light supplies energy for photosynthesis, growth, and other key activities, such as N2 fixation in cyanobacterial diazotrophs. The observed phenomenon that the growth rate becomes less sensitive to temperature changes (Figs. 1a and 4) in Trichodesmium IMS 101 under light-limiting levels can be attributed to insufficient energy supply for the cells to respond to temperature changes. While thermal biological responses are mainly based on enzymatic performance, light limitation suppresses syntheses of enzymes (Raven and Geider, 1988) and thus subsequently limits thermal responses. Although light-limited phytoplankton cells typically allocate more resources to light-harvesting systems to compensate for light shortages, at very low irradiances this compensation cannot prevent light-harvesting capacity from being a limiting factor for enzyme synthesis and growth (Raven and Geider, 1988). Field investigations show that vertical distributions of Trichodesmium can reach depths greater than 100 m, where light is absolutely limiting and temperature is lower compared to surface temperature (Olson et al., 2015; Rouco et al., 2016). According to the typical values of surface solar irradiances and the vertical extinction coefficient in tropical and subtropical oceans (Olson et al., 2015), the daily light dose received by the light-limited cultures in our study corresponds to that received by Trichodesmium at a depth of 50–60 m. The contribution of biomass and N2 fixation by Trichodesmium at depths greater than 50 m might range from 7 % to 28 % (Davis and McGillicuddy, 2006; Olson et al., 2015). Therefore, the evaluation of potential warming effects on Trichodesmium should not be constrained to the populations inhabiting light-saturated environments (upper tens of meters; Breitbarth et al., 2007; Jiang et al., 2018), making 3-D models indispensable. In existing 3-D model studies involving Trichodesmium (Boyd and Doney, 2002; Moore et al., 2002), the combined effects of temperature and light on Trichodesmium biological activities are simply assumed to be additive, which is proven to be inappropriate in this work. While the absolute values of the N2 fixation rate under light-limiting and light-saturating levels cannot be directly compared on the basis of Chl a content, since lower light levels resulted in more cellular Chl a content (Fig. S1b), comparison of the thermal response patterns can generate useful information for improving model predictions of diazotrophic responses to ocean climate change.
Thermal responses for organisms are known to be useful in evaluating thermal acclimation potential and probing low and high temperature tolerances (Gunderson et al, 2010; Somero, 2010; Way and Yamori, 2014). In this work, the shape of the short-term thermal response curves for N2 fixation is normalization-independent because cells were exposed to different assay temperatures for only 1 h, hardly changing the elemental stoichiometry or cellular pigment component. When exposed to abrupt temperature gradients, the light-saturated cells acclimated to higher temperatures exhibited higher Topt values (Table 3) and lower thermal susceptibility to supra-optimal temperatures (Eh; Table 3). This indicates an increased capability for the diazotroph to tolerate short-term warming impacts. However, light limitation made the cells more susceptible to warming due to decreased Topt and increased Eh for N2 fixation (Table 3). Moreover, with light limitation, acclimation to high temperatures did not help Trichodesmium cells tolerate short-term supra-optimal temperatures. On the other hand, Chl a fluorescence data show that the PSII in light-limited cultures was as healthy as that in cells grown under saturating light (Fig. 1c, 2), and it has been shown that damage to PSII usually occurs at temperatures above 45∘C (Yamori et al., 2014). Therefore, the collapse of N2 fixation at high temperatures was not likely caused by the dysfunction of the photosystems but might be caused by the uncoupling of adenosine triphosphate (ATP) synthesis to electron transport, since proton leakiness of the thylakoid membrane has been frequently proposed as a problem at high temperatures (Yamori et al., 2014). This is consistent with the observation that supra-optimal temperature inhibition of N2 fixation was aggravated by light limitation (Fig. 3). In addition, damage to nitrogenase at high temperatures might also be one of the reasons responsible for the faster drop of N2 fixation at high temperatures in light-limited cultures (Gallon et a., 1993). This is because the extra investment of resources to repair damaged nitrogenase could not be supported under light-limiting conditions (Fig. 3b). Therefore, light availability exerts critical control on the acclimation potential of N2 fixation in Trichodesmium to warming.
Acclimation to different temperatures also affected the activation energy (Ea) for N2 fixation in Trichodesmium IMS 101 (Table 3). For Trichodesmium species, N2 fixation can be controlled by supply of ATP and reducing equivalents, mainly coming from photosynthesis, and the inherent catalytic capacity of the nitrogenase. These two processes may exhibit different temperature dependence, i.e., different Ea. The Ea of the controlling process determines the N2 fixation Ea (Hikosaka et al., 2006; Staal et al., 2003). Therefore, the differences in N2 fixation Ea between cultures grown at different temperatures may reflect that N2 fixation was primarily controlled by different processes in cultures acclimated to different temperatures. Preliminary evidence supporting this hypothesis came from the various effects of assay light intensity on the values of Ea for N2 fixation between light-limited cultures grown at 23 and 27∘C (Table S1; Fig. S2). For Trichodesmium grown under light-limiting levels, the lower Ea values in cultures acclimated to 23∘C were significantly elevated by the increased assay light intensity which can provide more ATP and reducing equivalents (Table S1; Fig. S2a). This suggests that the constraint should be the supply of ATP and reducing equivalents. The higher Ea values in cultures acclimated to 27∘C were insensitive to the assay light intensity changes, suggesting that N2 fixation should not be controlled by the supply of ATP and reducing equivalents at this optimal temperature but may possibly be controlled by inherent catalytic capacity of the nitrogenase (Table S1; Fig. S2b).
The short-term thermal responses for N2 fixation mirror thermal shock responses. If cells are exposed to the thermal changes for longer time, acclimation will definitely change the thermal responses for N2 fixation in Trichodesmium (Breitbarth et al., 2007; Fu et al., 2014; Staal et al., 2003). To compare the short-term and acclimated thermal responses for N2 fixation, we calculated the corresponding values of Ea, Eh, and Topt, which are, respectively, 0.93±0.64 eV (±SEM), 1.86±1.19 eV (±SEM), and 27.1±1.0∘C (±SEM) for fully acclimated N2 fixation within the range of 20–34∘C growth temperatures in Trichodesmium IMS 101 (Breitbarth et al., 2007). These values of Ea and Eh are comparable to those derived from short-term thermal response for N2 fixation in the same strain grown under light-saturating conditions and 31∘C in our study (Table 3), but the Topt value is lower than that from short-term thermal response. On the other hand, we tried to derive values of Ea, Eh, and Topt for acclimated N2 fixation rates in another three Trichodesmium erythraeum strains (strains RLI, KO4-20, and 21-75; Fu et al., 2014), but the model fitting failed to converge. Instead of been negatively skewed, the thermal response curves of acclimated N2 fixation in these three Trichodesmium strains are nearly symmetrical. These comparisons show that thermal response for N2 fixation in Trichodesmium is strain-specific and is affected on the timescale of acclimation process.
In the oceans, Trichodesmium and other pelagic phytoplankton are often exposed to abrupt temperature changes due to strongly disturbed weather conditions, such as tropical cyclones and marine heat waves. Global warming has been predicted to increase both tropical cyclone intensities and the frequency of the most intense tropical cyclones (Elsner et al., 2008; Knutson et al., 2010; Wehner et al., 2018). Upper-ocean temperature declines prior to and during a cyclone event and then increases abruptly afterwards (Li et al., 2009), accompanied by strong variations in surface solar radiation and stratification (Sriver and Huber, 2007). These abrupt temperature changes occurring in nature are not as acute as those in our experiment. For example, temperature changes caused by cyclones and heat waves are on the order of 0.5–1∘C per day (Babin et al., 2004; Beca-Carretero et al., 2018). Nonetheless, these temperature changes occur within one generation of Trichodesmium because of its low growth rate, not leaving enough time for full acclimation. Therefore, the values of Ea, Eh, Th, and Topt provided in this study can likely serve as proxies for some types of abrupt natural temperature increases.
The data are available upon request to the corresponding author (Kunshan Gao).
The supplement related to this article is available online at: https://doi.org/10.5194/bg-17-1169-2020-supplement.
KG and XY designed the experiment. XY carried out the experiment. XY, FXF, DAH, and KG analyzed the data and wrote the paper.
The authors declare that they have no conflict of interest.
This research has been supported by the National Key R&D Program of China (grant no. 2016YFA0601400), the National Natural Science Foundation of China (grant nos. 41720104005 and 41721005), the Joint Project of the National Natural Science Foundation of China and Shandong Province (grant no. U1606404), and the US National Science Foundation (grant nos. OCE 1538525, OCE 1657757, and OCE 1638804).
This paper was edited by Carol Robinson and reviewed by three anonymous referees.
Babin, S. M., Carton, J. A., Dickey, T. D., and Wiggert, J. D.: Satellite evidence of hurricane-induced phytoplankton blooms in an oceanic desert, J. Geophys. Res., 109, C03043, https://doi.org/10.1029/2003jc001938, 2004.
Beca-Carretero, P., Guihéneuf, F., Marín-Guirao, L., Bernardeau-Esteller, J., García-Muñoz, R., Stengel, D. B., and Ruiz, J. M.: Effects of an experimental heat wave on fatty acid composition in two Mediterranean seagrass species, Mar. Pollut. Bull., 134, 27–37, https://doi.org/10.1016/j.marpolbul.2017.12.057, 2018.
Boatman, T. G., Lawson, T., and Geider, R. J.: A key marine diazotroph in a changing ocean: The interacting effects of temperature, CO2 and light on the growth of Trichodesmium Erythraeum ims101, PLOS ONE, 12, e0168796, https://doi.org/10.1371/journal.pone.0168796, 2017.
Boyd, P. W., and Doney, S. C.: Modelling regional responses by marine pelagic ecosystems to global climate change, Geophys. Res. Lett., 29, 53-1–53-4, https://doi.org/10.1029/2001GL014130, 2002.
Breitbarth, E., Oschlies, A., and LaRoche, J.: Physiological constraints on the global distribution of Trichodesmium – effect of temperature on diazotrophy, Biogeosciences, 4, 53–61, https://doi.org/10.5194/bg-4-53-2007, 2007.
Breitbarth, E., Wohlers, J., Kläs, J., LaRoche, J., and Peeken, I.: Nitrogen fixation and growth rates of Trichodesmium IMS-101 as a function of light intensity, Mar. Ecol. Prog. Ser., 359, 25–36, https://doi.org/10.3354/meps07241, 2008.
Cai, X. and Gao, K.: Levels of daily light doses under changed day-night cycles regulate temporal segregation of photosynthesis and N2 fixation in the cyanobacterium Trichodesmium erythraeum IMS101, PLOS ONE, 10, e0135401, https://doi.org/10.1371/journal.pone.0135401, 2015.
Cai, X., Gao, K., Fu, F., Campbell, D., Beardall, J., and Hutchins, D.: Electron transport kinetics in the diazotrophic cyanobacterium Trichodesmium spp. grown across a range of light levels, Photosynth. Res., 124, 45–56, https://doi.org/10.1007/s11120-015-0081-5, 2015.
Capone, D. G.: Determination of nitrogenase activity in aquatic samples using the acetylene reduction procedure, in: Current Methods in Aquatic Microbiology, edited by: Kemp, P. F., Sherr, B. F., Sherr, E. B., and Cole, J. J., Lewis Publishers, New York, 621–631, 1993.
Capone, D. G., Zehr, J. P., Paerl, H. W., Bergman, B., and Carpenter, E. J.: Trichodesmium, a globally significant marine cyanobacterium, Science, 276, 1221–1229, https://doi.org/10.1126/science.276.5316.1221, 1997.
Capone, D. G., Burns, J. A., Montoya, J. P., Subramaniam, A., Mahaffey, C., Gunderson, T., Michaels, A. F., and Carpenter, E. J.: Nitrogen fixation by Trichodesmium spp.: An important source of new nitrogen to the tropical and subtropical North Atlantic Ocean, Global Biogeochem. Cy., 19, GB2024, https://doi.org/10.1029/2004GB002331, 2005.
Chappell, P. D. and Webb, E. A.: A molecular assessment of the iron stress response in the two phylogenetic clades of Trichodesmium, Environ. Microbiol., 12, 13–27, https://doi.org/10.1111/j.1462-2920.2009.02026.x, 2010.
Chen, Y.-B., Zehr, J. P., and Mellon, M.: Growth and nitrogen fixation of the diazotrophic filamentous nonheterocystous cyanobacterium Trichodesmium sp. IMS 101 in defined media: Evidence for a circadian rhythm, J. Phycol., 32, 916–923, https://doi.org/10.1111/j.0022-3646.1996.00916.x, 1996.
Collins, M., R. Knutti, , J. Arblaster, J.-L. Dufresne, T. Fichefet, P. Friedlingstein, X. Gao, W. J. Gutowski, T. Johns, G. Krinner, M. Shongwe, C. Tebaldi, Weaver, A. J., and Wehner, M.: Long-term climate change: Projections, commitments and irreversibility, Climate Change 2013: The Physical Science Basis, Contribution Of Working Group I To The Fifth Assessment Report Of The Intergovernmental Panel On Climate Change, edited by: Stocker, T. F., D. Qin, G.-K. Plattner, M. Tignor, S. K. Allen, J. Boschung, A. Nauels, Y. Xia, Bex, V., and Midgley, P. M., Cambridge University Press, Cambridge, United Kingdom and New York, NY, USA, 1029–1136, 2013.
Davis, C. S. and McGillicuddy, D. J.: Transatlantic abundance of the N2-fixing colonial cyanobacterium Trichodesmium, Science, 312, 1517–1520, https://doi.org/10.1126/science.1123570, 2006.
Dugdale, R. C. and Goering, J. J.: Uptake of new and regenerated forms of nitrogen in primary productivity, Limnol. Oceanogr., 12, 196–206, https://doi.org/10.4319/lo.1967.12.2.0196, 1967.
Dugdale, R. C., Menzel, D. W., and Ryther, J. H.: Nitrogen fixation in the Sargasso Sea, Deep-Sea Res., 7, 297–300, https://doi.org/10.1016/0146-6313(61)90051-X, 1961.
Dugdale, R. C., Goering, J. J., and Ryther, J. H.: High nitrogen fixation rates in the Sargasso Sea and the Arabian Sea, Limnol. Oceanogr., 9, 507–510, https://doi.org/10.4319/lo.1964.9.4.0507, 1964.
Eilers, P. H. C. and Peeters, J. C. H.: A model for the relationship between light intensity and the rate of photosynthesis in phytoplankton, Ecol. Model., 42, 199–215, https://doi.org/10.1016/0304-3800(88)90057-9, 1988.
Elsner, J. B., Kossin, J. P., and Jagger, T. H.: The increasing intensity of the strongest tropical cyclones, Nature, 455, 92–95, https://doi.org/10.1038/nature07234, 2008.
Fu, F. X., Yu, E., Garcia, N. S., Gale, J., Luo, Y., Webb, E. A., and Hutchins, D. A.: Differing responses of marine N2 fixers to warming and consequences for future diazotroph community structure, Aquat. Microb. Ecol., 72, 33–46, https://doi.org/10.3354/ame01683, 2014.
Gallon, J. R., Pederson, D. M., and Smith, G. D.: The effect of temperature on the sensitivity of nitrogenase to oxygen in the cyanobacteria Anabaena cylindrica (Lemmermann) and Gloeothece (Nägeli), New Phytol., 124, 251–257, https://doi.org/10.1111/j.1469-8137.1993.tb03814.x, 1993.
Garcia, N. S., Fu, F. X., Breene, C. L., Bernhardt, P. W., Mulholland, M. R., Sohm, J. A., and Hutchins, D. A.: Interactive effects of irradiance and CO2 on CO2 fixation and N2 fixation in the diazotroph Trichodesmium erythraeum (cyanobacteria) J. Phycol., 47, 1292–1303, 2011.
Gillooly, J. F., Brown, J. H., West, G. B., Savage, V. M., and Charnov, E. L.: Effects of size and temperature on metabolic rate, Science, 293, 2248–2251, https://doi.org/10.1126/science.1061967, 2001.
Gunderson, C. A., O'hara, K. H., Campion, C. M., Walker, A. V., and Edwards, N. T.: Thermal plasticity of photosynthesis: the role of acclimation in forest responses to a warming climate, Glob. Change Biol., 16, 2272–2286, https://doi.org/10.1111/j.1365-2486.2009.02090.x, 2010.
Hikosaka, K., Ishikawa, K., Borjigidai, A., Muller, O., and Onoda, Y.: Temperature acclimation of photosynthesis: mechanisms involved in the changes in temperature dependence of photosynthetic rate, J. Exp. Bot., 57, 291–302, https://doi.org/10.1093/jxb/erj049, 2006.
Hutchins, D. A. and Boyd, P. W.: Marine phytoplankton and the changing ocean iron cycle, Nat. Clim. Change, 6, 1072–1079, https://doi.org/10.1038/nclimate3147, 2016.
Hutchins, D. A. and Fu, F.: Microorganisms and ocean global change, Nat. Microbiol., 2, 17058, https://doi.org/10.1038/nmicrobiol.2017.58, 2017.
Hutchins, D. A., Fu, F. X., Zhang, Y., Warner, M. E., Feng, Y., Portune, K., Bernhardt, P. W., and Mulholland, M. R.: CO2 control of Trichodesmium N2 fixation, photosynthesis, growth rates, and elemental ratios: Implications for past, present, and future ocean biogeochemistry, Limnol. Oceanogr., 52, 1293–1304, https://doi.org/10.4319/lo.2007.52.4.1293, 2007.
Hutchins, D. A., Walworth, N. G., Webb, E. A., Saito, M. A., Moran, D., McIlvin, M. R., Gale, J., and Fu, F.-X.: Irreversibly increased nitrogen fixation in Trichodesmium experimentally adapted to elevated carbon dioxide, Nat. Commun., 6, 8155, https://doi.org/10.1038/ncomms9155, 2015.
Jiang, H.-B., Fu, F.-X., Rivero-Calle, S., Levine, N. M., Sañudo-Wilhelmy, S. A., Qu, P.-P., Wang, X.-W., Pinedo-Gonzalez, P., Zhu, Z., and Hutchins, D. A.: Ocean warming alleviates iron limitation of marine nitrogen fixation, Nat. Clim. Change, 8, 709–712, https://doi.org/10.1038/s41558-018-0216-8, 2018.
Knutson, T. R., McBride, J. L., Chan, J., Emanuel, K., Holland, G., Landsea, C., Held, I., Kossin, J. P., Srivastava, A. K., and Sugi, M.: Tropical cyclones and climate change, Nat. Geosci., 3, 157–163, https://doi.org/10.1038/ngeo779, 2010.
LaRoche, J. and Breitbarth, E.: Importance of the diazotrophs as a source of new nitrogen in the ocean, J. Sea Res., 53, 67–91, https://doi.org/10.1016/j.seares.2004.05.005, 2005.
Levitan, O., Brown, C. M., Sudhaus, S., Campbell, D., LaRoche, J., and Berman-Frank, I.: Regulation of nitrogen metabolism in the marine diazotroph Trichodesmium IMS101 under varying temperatures and atmospheric CO2 concentrations, Environ. Microbiol., 12, 1899–1912, https://doi.org/10.1111/j.1462-2920.2010.02195.x, 2010.
Li, F., Beardall, J., Collins, S., and Gao, K.: Decreased photosynthesis and growth with reduced respiration in the model diatom Phaeodactylum tricornutum grown under elevated CO2 over 1800 generations, Glob. Change Biol., 23, 127–137, https://doi.org/10.1111/gcb.13501, 2017.
Li, G., Wu, Y., and Gao, K.: Effects of Typhoon Kaemi on coastal phytoplankton assemblages in the South China Sea, with special reference to the effects of solar UV radiation, J. Geophys. Res., 114, G04029, https://doi.org/10.1029/2008jg000896, 2009.
Mahaffey, C., Michaels, A. F., and Capone, D. G.: The conundrum of marine N2 fixation, Am. J. Sci., 305, 546–595, https://doi.org/10.2475/ajs.305.6-8.546, 2005.
Moore, C. M., Mills, M. M., Arrigo, K. R., Berman-Frank, I., Bopp, L., Boyd, P. W., Galbraith, E. D., Geider, R. J., Guieu, C., Jaccard, S. L., Jickells, T. D., La Roche, J., Lenton, T. M., Mahowald, N. M., Marañón, E., Marinov, I., Moore, J. K., Nakatsuka, T., Oschlies, A., Saito, M. A., Thingstad, T. F., Tsuda, A., and Ulloa, O.: Processes and patterns of oceanic nutrient limitation, Nat. Geosci., 6, 701–710, https://doi.org/10.1038/ngeo1765, 2013.
Moore, J. K., Doney, S. C., Kleypas, J. A., Glover, D. M., and Fung, I. Y.: An intermediate complexity marine ecosystem model for the global domain, Deep-Sea Res. Pt. II, 49, 403–462, https://doi.org/10.1016/S0967-0645(01)00108-4, 2002.
Olson, E. M., McGillicuddy Jr., D. J., Dyhrman, S. T., Waterbury, J. B., Davis, C. S., and Solow, A. R.: The depth-distribution of nitrogen fixation by Trichodesmium spp. colonies in the tropical-subtropical North Atlantic, Deep-Sea Res. PT. I., 104, 72–91, https://doi.org/10.1016/j.dsr.2015.06.012, 2015.
Padfield, D., Yvon-Durocher, G., Buckling, A., Jennings, S., and Yvon-Durocher, G.: Rapid evolution of metabolic traits explains thermal adaptation in phytoplankton, Ecol. Lett., 19, 133–142, https://doi.org/10.1111/ele.12545, 2015.
Prufert-Bebout, L., Paerl, H. W., and Lassen, C.: Growth, nitrogen fixation, and spectral attenuation in cultivated Trichodesmium species, Appl. Environ. Microbiol., 59, 1367–1375, 1993.
Ralph, P. J. and Gademann, R.: Rapid light curves: A powerful tool to assess photosynthetic activity, Aquat. Bot., 82, 222–237, https://doi.org/10.1016/j.aquabot.2005.02.006, 2005.
Raven, J. A. and Geider, R. J.: Temperature and algal growth, New Phytol., 110, 441–461, https://doi.org/10.1111/j.1469-8137.1988.tb00282.x, 1988.
Ritchie, R. J.: Consistent Sets of Spectrophotometric Chlorophyll Equations for Acetone, Methanol and Ethanol Solvents, Photosynth. Res., 89, 27–41, https://doi.org/10.1007/s11120-006-9065-9, 2006.
Rouco, M., Haley, S. T., Alexander, H., Wilson, S. T., Karl, D. M., and Dyhrman, S. T.: Variable depth distribution of Trichodesmium clades in the North Pacific Ocean, Env. Microbiol. Rep., 8, 1058–1066, https://doi.org/10.1111/1758-2229.12488, 2016.
Schaum, C. E., Buckling, A., Smirnoff, N., Studholme, D. J., and Yvon-Durocher, G.: Environmental fluctuations accelerate molecular evolution of thermal tolerance in a marine diatom, Nat. Commun., 9, 1719, https://doi.org/10.1038/s41467-018-03906-5, 2018.
Schoolfield, R. M., Sharpe, P. J. H., and Magnuson, C. E.: Non-linear regression of biological temperature-dependent rate models based on absolute reaction-rate theory, J. Theor. Biol., 88, 719–731, https://doi.org/10.1016/0022-5193(81)90246-0, 1981.
Sharpe, P. J. H. and DeMichele, D. W.: Reaction kinetics of poikilotherm development, J. Theor. Biol., 64, 649–670, https://doi.org/10.1016/0022-5193(77)90265-X, 1977.
Sohm, J. A., Webb, E. A., and Capone, D. G.: Emerging patterns of marine nitrogen fixation, Nat. Rev. Microbiol., 9, 499–508, https://doi.org/10.1038/nrmicro2594, 2011.
Somero, G. N.: The physiology of climate change: how potentials for acclimatization and genetic adaptation will determine “winners” and “losers”, J. Exp. Biol., 213, 912–920, https://doi.org/10.1242/jeb.037473, 2010.
Sriver, R. L. and Huber, M.: Observational evidence for an ocean heat pump induced by tropical cyclones, Nature, 447, 577–580, https://doi.org/10.1038/nature05785, 2007.
Staal, M., Meysman, F. J. R., and Stal, L. J.: Temperature excludes N2-fixing heterocystous cyanobacteria in the tropical oceans, Nature, 425, 504–507, https://doi.org/10.1038/nature01999, 2003.
Suggett, D. J., Le Floc'H, E., Harris, G. N., Leonardos, N., and Geider, R. J.: Different strategies of photoacclimation by two strains of Emiliania huxleyi (Haptophyta), J. Phycol., 43, 1209–1222, https://doi.org/10.1111/j.1529-8817.2007.00406.x, 2007.
Thomas, M. K., Kremer, C. T., Klausmeier, C. A., and Litchman, E.: A global pattern of thermal adaptation in marine phytoplankton, Science, 338, 1085–1088, https://doi.org/10.1126/science.1224836, 2012.
Tong, S., Gao, K., and Hutchins, D. A.: Adaptive evolution in the coccolithophore Gephyrocapsa oceanica following 1,000 generations of selection under elevated CO2, Global Change Biol., 24, 3055–3064, https://doi.org/10.1111/gcb.14065, 2018.
Villareal, T. and Carpenter, E.: Buoyancy regulation and the potential for vertical migration in the oceanic cyanobacterium Trichodesmium, Microb. Ecol., 45, 1–10, 2003.
Way, D. A. and Yamori, W.: Thermal acclimation of photosynthesis: on the importance of adjusting our definitions and accounting for thermal acclimation of respiration, Photosynth. Res., 119, 89–100, https://doi.org/10.1007/s11120-013-9873-7, 2014.
Wehner, M. F., Reed, K. A., Loring, B., Stone, D., and Krishnan, H.: Changes in tropical cyclones under stabilized 1.5 and 2.0 ∘C global warming scenarios as simulated by the Community Atmospheric Model under the HAPPI protocols, Earth Syst. Dynam., 9, 187–195, https://doi.org/10.5194/esd-9-187-2018, 2018.
Yamori, W., Hikosaka, K., and Way, D. A.: Temperature response of photosynthesis in C3, C4, and CAM plants: temperature acclimation and temperature adaptation, Photosynth. Res., 119, 101–117, https://doi.org/10.1007/s11120-013-9874-6, 2014.
Zehr, J. P.: Nitrogen fixation by marine cyanobacteria, Trends Microbiol., 19, 162–173, https://doi.org/10.1016/j.tim.2010.12.004, 2011.