the Creative Commons Attribution 4.0 License.
the Creative Commons Attribution 4.0 License.
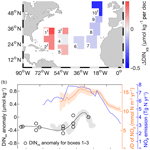
Contrasting decadal trends of subsurface excess nitrate in the western and eastern North Atlantic Ocean
Jin-Yu Terence Yang
Jia-Zhong Zhang
Ji-Young Moon
Joon-Soo Lee
In-Seong Han
Eunil Lee
Temporal variations in excess nitrate (DINxs) relative to dissolved inorganic phosphorus (DIP) were evaluated using datasets derived from repeated measurements along meridional and zonal transects in the upper (200–600 m) North Atlantic (NAtl) between the 1980s and 2010s. The analysis revealed that the DINxs trend in the western NAtl differed from that in the eastern NAtl. In the western NAtl, which has been subject to atmospheric nitrogen deposition (AND) from the USA, the subsurface DINxs concentrations have increased over the last 2 decades. This increase was associated with the increase in AND measured along the US East Coast, with a mean lag period of 15 years. This time lag was approximately equivalent to the time elapsed since the subsurface waters in the western NAtl were last in contact with the atmosphere (the ventilation age), suggesting a major role for a physical mechanism in transporting the AND signals to the subsurface. Our finding provides evidence that the DINxs dynamics in the western NAtl in recent years has been affected by anthropogenic nitrogen inputs, although this influence is weak relative to that in the western North Pacific. In contrast, a decreasing trend in subsurface DINxs was observed after the 2000s in the eastern NAtl, particularly in the high latitudes. This finding was not associated with the comparable decrease in AND from Europe. Other natural processes (a possible decline in tropical N2 fixation and weakening of the Atlantic meridional overturning circulation) may be responsible, but lack of time-resolved data on N2 fixation and meridional circulation is an impediment to assessment of these processes. Our results highlight the importance of both anthropogenic and natural forcing in impacting the nutrient dynamics in the upper NAtl.
- Article
(4295 KB) - Full-text XML
-
Supplement
(1857 KB) - BibTeX
- EndNote
The supply of bioavailable nitrogen (Nbio= NOy+ NHx) to the surface ocean is limited in most of the oligotrophic marine environments (Fanning, 1989; Moore et al., 2013; Moore, 2016). The addition of Nbio will lead to an increase in primary and export production and eventually an enhancement of carbon sequestration in the ocean interior (Okin et al., 2011; Jickells and Moore, 2015). Anthropogenic nitrogen deposition (AND) to the contemporary ocean is comparable in magnitude to marine biological N2 fixation (Duce et al., 2008), which has been thought to be the major external source of Nbio to the oligotrophic ocean (Duce et al., 2008; Fowler et al., 2013; Jickells et al., 2017). In particular, AND has been found to enhance phytoplankton productivity in N-depleted tropical and subtropical oceans located downwind of continents that are sources of Nbio (T.-W. Kim et al., 2014; St-Laurent et al., 2017). Any changes in this external source of Nbio induced largely by human activities could cause a wide range of ecological and biogeochemical consequences (e.g., Doney et al., 2007; Yang and Gruber, 2016).
The impact of AND on the dissolved inorganic nitrogen concentration (DIN) in seawater has recently been assessed in coastal and marginal seas (Kim et al., 2011; Moon et al., 2016), as well as in the remote open ocean (I.-N. Kim et al., 2014), using historical nutrient concentration datasets. The analysis of 30 years of data showed that the DIN has increased in marginal seas off the northeastern Asian continent, whereas the dissolved inorganic phosphorus concentration (DIP) has remained relatively unchanged over this time period (Kim et al., 2011). For open-ocean areas, the temporal change in excess DIN relative to DIP (DINxs= DIN − DIP, where RN:P is the average DIN : DIP ratio of 15:1 for deep waters) was estimated using the relationship between DINxs in a particular water parcel and the chlorofluorocarbon (CFC)-12-derived ventilation age of that parcel (I.-N. Kim et al., 2014). An underlying assumption in this analysis is that ocean biological processes (i.e., production and microbial oxidation of organic matter) operate at a DIN : DIP ratio of 15:1 and thus do not change DINxs. Changes in seawater DINxs only occur when either N input (i.e., AND and N2 fixation) or N loss (i.e., denitrification) occurs. The analysis using this method revealed that the DINxs has increased in the western North Pacific Ocean (NPO) since the 1970s (I.-N. Kim et al., 2014).
The addition of Nbio to the North Atlantic Ocean (NAtl), which is located downwind from North America, has more than doubled since 1986 (Galloway et al., 1996). The increasing addition of Nbio may lead to an increase in DINxs in the NAtl (Zamora et al., 2010). However, it has been argued that N2 fixation is a more likely cause of the higher subsurface DINxs in the NAtl (Gruber and Sarmiento, 1997; Bates and Hansell, 2004). Differentiating the contributions of N2 fixation and AND is not straightforward because both processes leave similar biogeochemical signals in seawater, including a high DIN : DIP ratio and low nitrogen isotope composition (Hastings et al., 2003; Knapp et al., 2010; Yang et al., 2014). In addition to these two processes, climate variations (commonly expressed as the North Atlantic Oscillation Index) can concurrently influence the nutrient dynamics in the NAtl (Bates and Hansell, 2004; Singh et al., 2013). As a result, the processes causing the change in the subsurface DINxs signal in the NAtl remain unresolved. This knowledge gap needs to be filled to improve understanding of the marine nitrogen cycle (Gruber and Deutsch, 2014).
The present study was designed to explore the occurrence and rate of decadal change in DINxs (ΔDINxs in µmol kg−1 per decade) in the subsurface NAtl, as well as the explanations for ΔDINxs, based on repeat measurements of nutrients and other oceanographic parameters made over the past 3 decades or longer.
2.1 Data
Historical data on temperature, salinity, and the concentrations of nitrate, nitrite, phosphate, and oxygen used in this study have been collected as parts of the Transient Tracers in the Ocean (TTO), the World Ocean Circulation Experiment (WOCE), the Climate Variability CO2 Repeat Hydrography (CLIVAR), and the Global Ocean Ship-based Hydrographic Investigations (GO-SHIP) programs. Analysis of nutrient data was based only on concentrations greater than 0.1 µmol kg−1 for DIN and 0.01 µmol kg−1 for DIP. These concentration levels approximate the detection limits of DIN and DIP for the analytical methods used in the field observations (Zhang et al., 2001; Hydes et al., 2010). Our analysis for estimation of DINxs focused exclusively on data collected from 200–600 m depth (see below), where nutrient concentrations were higher than 1.4 µmol kg−1 for DIN and 0.08 µmol kg−1 for DIP. More explicitly, the lower ends of DIN and DIP concentrations in these waters are several-fold higher than the detection limits of DIN and DIP. As low DIP concentration (<0.1 µmol kg−1) may result in uncertainties (Martiny et al., 2019), we did not use those DIP and accompanying DIN data (accounting for 1.4 % of the total 1955 data points) to eliminate any potential bias in the DINxs estimates. Removal of the low DIP data did not alter our findings (e.g., the trend of increasing DINxs in the western subtropical NAtl). The data used in our analysis are available at https://www.nodc.noaa.gov/ocads/oceans/ (last access: 20 February 2020; the Global Ocean Data Analysis Project version 2, GLODAPv2, product and CLIVAR database).
Data analysis was primarily focused on three meridional (A22, A20, and A16N) transects in the NAtl (Fig. 1). A zonal transect (A05) was also included for comparison. All four transects used in the study are located downwind of the North American continent, which was predicted by Dentener et al. (2006) to be a major source region for anthropogenic nitrogen (Fig. 1). Each of these transects was sampled three or four times during the past 30 years (Table S1 in the Supplement). To extend temporal data coverage in the analysis, historical data obtained from locations adjacent to the four study transects were included in the analysis. Moreover, the repeat measurements along transect A22 occurred on slightly different tracks, particularly in the Caribbean Sea and in the northern end of the transect. We therefore excluded data for areas south of Puerto Rico (∼18.5∘ N) and north of 36∘ N, where the distance between the location of repeat measurements exceeded 2∘ longitude. Data obtained south of 20∘ N along A16N and south of 15∘ N along A20 were also excluded from the analysis, because these regions are considerably influenced by the water masses originated from the equatorial upwelling region (Hansell et al., 2004), and any change in the intensity of upwelling could bias our analysis of changes in DINxs.
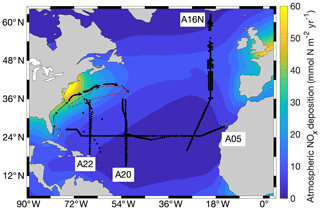
Figure 1Nutrient sampling locations (black dots) in the North Atlantic Ocean (NAtl). All datasets from the meridional (A22, A20, and A16N) and zonal (A05) cruises were collected in the GLODAPv2 product and CLIVAR database (see text). The red dashed line indicates the region of subtropical mode water (STMW) formation, and the solid arrows indicate streamlines of high transport in the western NAtl (i.e., the Gulf Stream, modified from Palter et al., 2005). The color scale indicates the model-derived atmospheric NOx deposition into the NAtl for 2000 (Dentener et al., 2006).
The GLODAPv2 product includes data obtained from >700 cruises during the period 1972–2013. These large datasets collected in different years and by different investigators may contain some systematic and analytical errors. To remove these systematic errors, quality control of the data was performed by Key et al. (2015) and by Olsen et al. (2016), largely based on comparison of repeated measurements made for waters deeper than 2000 m at the same locations. Any biases found were corrected by applying adjustment factors to the raw datasets, and the adjusted datasets were reported in the GLODAPv2 product (Key et al., 2015; Olsen et al., 2016).
More recent data, obtained during the 2010s, were not thoroughly compared with the data collected in the 2000s or earlier. To account for any small discrepancies that may exist among the various datasets collected on the four transects, we adjusted the DIN and DIP concentrations based on the assumption that the physical and chemical properties in deep waters of the tropical and subtropical NAtl (south of 50∘ N) did not change on decadal timescales (Figs. S1 and S2; see details in Text S1 in the Supplement). The mean corrections were found to be 0.04±0.03 µmol kg−1 for DIN and 0.006±0.004 µmol kg−1 for DIP, corresponding to their adjustment factors mostly less than 1.5 % (Table S2 and Fig. S3). These corrections fell within the detection limits for DIN and DIP and were an order of magnitude smaller than the subsurface ΔDINxs signals (see Sect. 3.1). The finding that the subsurface ΔDINxs signals were considerably greater than the detection limit of DIN is a strong indication that our data adjustments probably did not influence the temporal trend of DINxs. It also suggests that our method can extract the decadal trends of DINxs from fewer time-resolved datasets, as has successfully been used in previous studies (Zhang et al., 2000; Ríos et al., 2015; Woosley et al., 2016).
2.2 Relative abundance of DIN over DIP (DINxs) in water parcels
We calculated the DIN surplus relative to DIP in each seawater sample (i.e., the deviation of the DIN : DIP ratio from that in deep water) by calculating DINxs (Fig. 2). This calculation was performed in the upper 1500 m; and the ΔDINxs signals between GO-SHIP and WOCE time periods were also evaluated using data obtained from the subsurface layer (200–600 m) because the majority of DINxs signals derive from this layer, and hence any changes would be expected to be more marked (see the next section and the ΔDINxs signals at 1200–1500 m for reference in Fig. 2). The errors in DINxs estimates would be less than 0.04 µmol kg−1 for the target subsurface layer (200–600 m depth) if an overall uncertainty of 0.4 % for both DIN and DIP was used (Baringer et al., 2014); this is an order of magnitude smaller than the subsurface DINxs changes in the western subtropical NAtl (Fig. 3). In addition, the effect of seasonal variations on DINxs signals at the depth layer of 200–600 m is generally insignificant, because seasonal variations are largely confined to waters shallower than the climatological winter mixed layer (down to 200 m depth). Based on analysis of data obtained from the BATS site, seasonal variations in subsurface (the target water depth range) mean DINxs values were <0.1 µmol kg−1 (note that nutrient data at the BATS site are available at http://batsftp.bios.edu/BATS/bottle/bats_bottle.txt, last access: 20 February 2020).
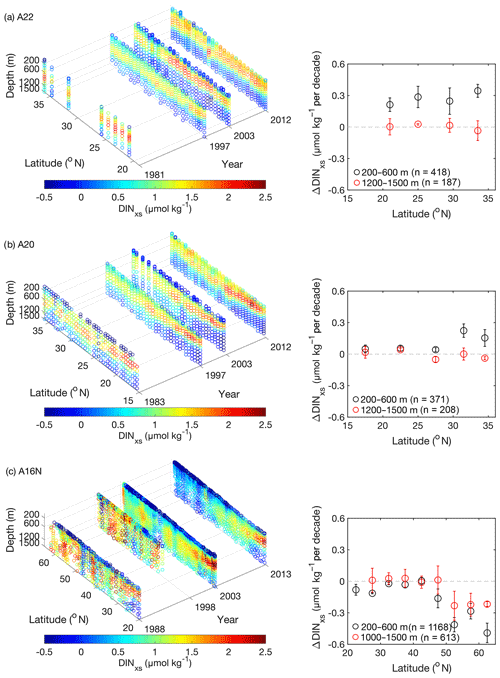
Figure 2The vertical distributions of DINxs in the upper 1500 m for different cruises along three meridional transects are shown in (a) A22, (b) A20, and (c) A16N, respectively. The insets in (a)–(c) show the average rates (with 95 % confidence limits) of ΔDINxs at 200–600 m and 1200–1500 m averaged for each 3–8∘ latitude interval between GO-SHIP (2010s) and WOCE (late 1980s to 1990s) time periods along each transect (see Table S1). Data from three cruises between the GO-SHIP and WOCE time periods were used to calculate the ΔDINxs along each transect (numbers in the insets indicate the number of data points for the ΔDINxs estimates at depth ranges of 200–600 and 1200–1500 m, respectively).
The values for ΔDINxs and nutrients in the water column could be biased because of mixing of water masses having different DINxs concentrations and due to different nitrogen-to-phosphorus ratios associated with organic matter oxidation during various observation periods. To minimize biases caused by these natural processes, we examined changes in potential temperature θ, salinity, and apparent oxygen utilization (AOU) along the potential density surfaces σθ (corresponding to 200–600 m, where the DINxs signals are the largest) at 5–15∘ latitude or longitude intervals representing average regional variations along each transect. We found that the θ and salinity of a water mass occupying any given density surface did not change between repeat occupations (p>0.05, Student's t test and ANOVA with Games–Howell test), except for slightly lower θ and salinity since 2000s in the subpolar region (north of 45∘ N) along A16N (Figs. S4 and S5). This finding is a strong indication that biases in ΔDINxs from the mixing of different water masses were negligible in the subtropical regions over the observation periods (approximately 30 years). In contrast, we found slight differences in ΔAOU among a few of selected reoccupations (not shown). To remove the contribution of DIN and DIP from oxidation of organic matter, adjustment of the nutrient concentrations was made by using the DIP : DIN : O2 remineralization ratio of (−160) derived from data along the σθ=27.0 horizon in the NAtl (Takahashi et al., 1985; Anderson and Sarmiento, 1994). The estimated DIN : DIP ratio for remineralization of organic matter was in the range 15–18 (Takahashi et al., 1985; Anderson and Sarmiento, 1994). The chosen value of the DIN : DIP ratio for remineralization did not significantly change the patterns of ΔDINxs. Therefore, the ΔDINxs signals within the layer of the DINxs maxima along all transects examined were free from biases of either mixing of water masses or changes in oxidation of organic matter.
3.1 Decadal variations of DINxs in the upper North Atlantic Ocean
High DINxs values were broadly distributed in the subsurface waters (<1000 m) in the NAtl. In particular, the maximum DINxs values were found between 200 and 600 m (Fig. 2) and were slightly higher in the western basin (an average value of 1.4±0.3 µmol kg−1 was calculated for A22 in 2012) than those in the eastern basin (an average value of 0.8±0.2 µmol kg−1 was calculated for A16N in 2013).
Based on multiple cruises along each transect, changes in DINxs were discernable over the decadal periods; these changes were most pronounced between 200 and 600 m (Fig. 2). The rate of ΔDINxs in the NAtl differed among locations of transects between GO-SHIP and WOCE periods. Specifically, the ΔDINxs values were mostly positive in the western NAtl (A22 and A20), where they varied from 0.02 to 0.33 µmol kg−1 per decade, with the highest rate found at 31∘ N–36∘ N along A22. In contrast, the ΔDINxs values became negative in the eastern NAtl (20–60∘ N along A16N), where they ranged from −0.07 to −0.40 µmol kg−1 per decade; the greatest rate of DINxs decrease was in the subpolar region (north of 45∘ N). Moreover, the ΔDINxs values remained close to zero in the intermediate waters (1200–1500 m) in the western and eastern subtropical NAtl (Fig. 2). This observation confirms that the marked changes in DINxs largely occurred in the subsurface waters. It is notable that the DINxs values were also found to be lower in the intermediate waters at high latitudes (north of 45∘ N) along the A16N transect, just as we observed for the subsurface waters. In this subpolar region, deep winter convection occurs and the North Atlantic Deep Water forms, both of which would be likely to lead to the propagation of subsurface DINxs signals to the intermediate waters.
The variations in DINxs in the NAtl showed geographically distinct patterns after removing the influence of remineralization of organic matter (Fig. 3). We found that the ΔDINxs within the layer of the DINxs maximum increased since 1997 (the year of a cruise carried out) along the transects near the source continent (i.e., the entire transect of A22, and 31–36∘ N along A20) (Fig. 3a and b), and its rates ranged from 0.19 to 0.33 µmol kg−1 per decade (Fig. 2). The trend in DINxs found at the BATS site is broadly comparable to that found between 31 and 36∘ N along A22 (Fig. 3a). The rate of increase of DINxs at the BATS site since the late 1990s (0.40 µmol kg−1 per decade) was also similar to that observed in the latitude band 31–36∘ N along A22. Such agreement with time-series data strengthens our finding derived from fewer time-resolved datasets.
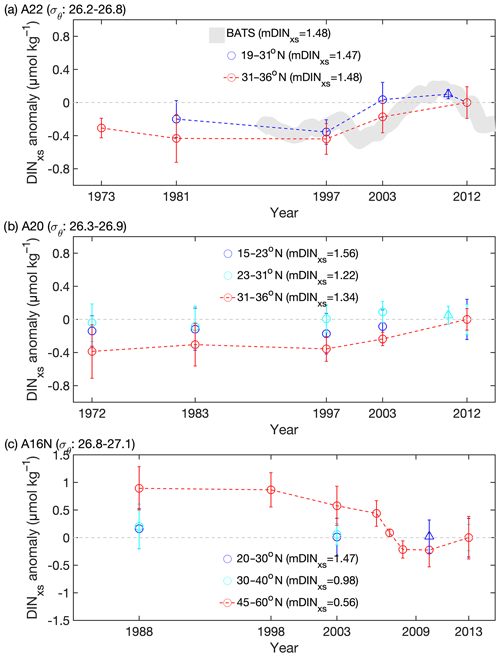
Figure 3Temporal trends of DINxs anomalies (dots) for the corresponding latitude interval for the subsurface potential density intervals σθ along the three meridional transects (a) A22, (b) A20, and (c) A16N in the NAtl. Data from A05 obtained in 2010 at three crossover sites are also shown (triangles). mDINxs values in parentheses indicate the mean DINxs in the GO-SHIP dataset. For each subregion, DINxs anomalies indicate individual DINxs values minus the mDINxs values from the GO-SHIP dataset. Note that the positive anomalies indicate higher values than the GO-SHIP data. The selected σθ intervals are typically located at the depth intervals of 200–600 m with DINxs maximum along each transect. Note that the selected σθ interval (= 27.2–27.6) in the subpolar region along A16N (45–60∘ N) is different from that in the subtropical region, as σθ for 200–600 m depth becomes larger in the high-latitude region. Besides repeat cruises of these transects, the datasets from other cruises with similar tracks (Fig. 1) in the subregions were included for comparison. The DINxs values were corrected by the changes in AOU to remove the contribution from remineralization of organic matter (see text). The data points connected by the dashed lines indicate that the ΔDINxs values were statistically significant in these regions (p<0.05, Student's t test and ANOVA with Games–Howell test). Otherwise, the data that were statistically unchanged are not connected by the dashed lines. The smoothed trend using the 5-year robust locally weighted scatterplot smoothing filter for DINxs anomalies of the STMW at the same depth ranges of the BATS site (near A22) is also shown (the gray shading in a). The gray dashed lines indicate that the DINxs anomaly equals to zero. The size of symbol for DINxs indicates the error of DINxs.
The discernable increase in DINxs rapidly diminished in the central gyre of the NAtl (15–31∘ N for A20 and 20–45∘ N for A16N and A05), where the variation in DINxs was statistically insignificant (p>0.05, Student's t test and ANOVA with Games–Howell test; Figs. 3 and S7). Furthermore, the level of DINxs appeared to decrease at high latitudes in the eastern NAtl (north of 45∘ N on A16N; Fig. 3c). The trend of decrease has been more pronounced since the 2000s in this region and occurred concurrently with decreases in temperature and salinity (p<0.05, Student's t test and ANOVA with Games–Howell test; Figs. S4c and S5c). Our observations indicate that the mechanisms responsible for the ΔDINxs in the subtropical and subpolar NAtl are likely to differ.
3.2 AND influence on the ΔDINxs in the western North Atlantic Ocean
More pronounced increase in the subsurface DINxshas been observed in recent decades in the western midlatitude NAtl (Fig. 3), which is subject to considerable AND input from the North American continent (Dentener et al., 2006). Model results show that the total AND over the NAtl basin in 2000 varied from 35 to 70 mmol N m−2 yr−1, reaching higher levels in the US coastal areas (Duce et al., 2008). Recent studies suggest that the reduced form of nitrogen (i.e., ammonium and dissolved organic nitrogen) entering the NAtl is primarily of marine autochthonous origin rather than of anthropogenic origin (i.e., atmospheric deposition) (Altieri et al., 2014, 2016). This marine-derived reduced nitrogen would not influence seawater DINxs values. We only included the effect of nitrogen deposition in the oxidized form (mostly in the forms of and HNO3; hereinafter NOx is used to represent these oxidized forms of nitrogen) on the DINxs values (Figs. 1 and 5b). From the 1970s to the 2010s the NOx emissions from the USA showed a three-phase temporal transition (EPA, 2000). The NOx emissions from the USA increased from 1970 to the mid-1980s, stayed at high levels for approximately 20 years, and then decreased gradually after the mid-2000s as a result of the regulation of air pollutant emissions throughout the North American continent (Fig. 5b). The anthropogenic nitrogen pollutants are mostly transported eastward and ultimately deposited in the western NAtl (Fig. 1).
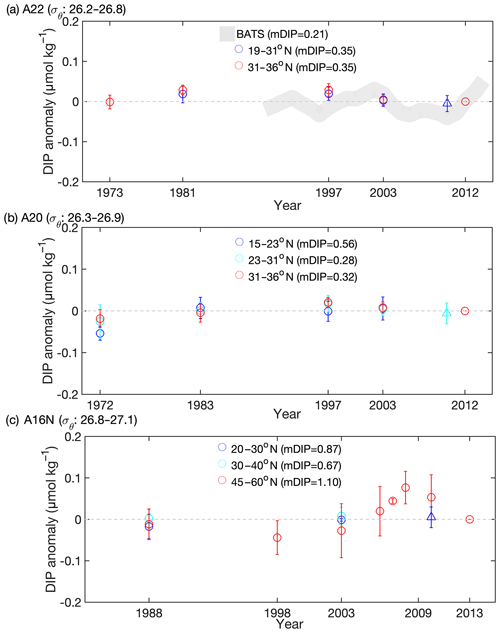
Figure 4As for Fig. 3 but for DIP anomalies. mDIP values in parentheses indicate the mean DIP in the GO-SHIP dataset. For each subregion, DIP anomalies indicate individual DIP values minus the mDIP values from the GO-SHIP dataset. The gray dashed lines indicate that the DIP anomaly equals to zero. Data from the BATS site are also included in (a).
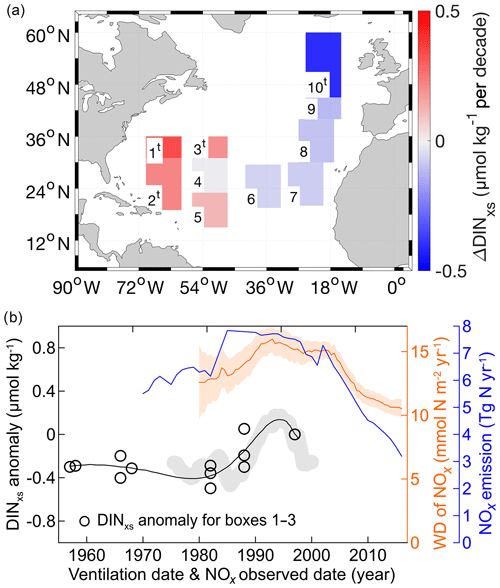
Figure 5(a) The rates of ΔDINxs in the subsurface waters (200−600 m) along the four transects between GO-SHIP and WOCE time periods (see Table S1). The study area is divided into 10 subregions of 10∘ longitude by 5–15∘ latitude along the transects A22 (boxes 1–2), A20 (boxes 3–5), part of A05 (box 6), and A16N (boxes 7–10). The statistically significant changes (Student's t test and ANOVA with Games–Howell test, p<0.05) are marked with the superscript “t” for the box numbers. (b) Temporal variations of DINxs anomalies (open dots and their fitting curve) in the western NAtl in which the subsurface DINxs increased significantly (boxes 1–3 in a). Trend in DINxs anomalies in the subsurface waters at the BATS site is shown in gray shading (the same as Fig. 3a). To ensure consistent comparisons between atmospheric N deposition rates and seawater DINxs anomalies, the seawater DINxs anomaly values were shifted backward by 15 years. The 15-year shift corresponded to the mean time period that had elapsed since a given subsurface water mass had last been in contact with the atmosphere prior to subduction. The year that the subsurface water mass in the NAtl last had contacted the atmosphere was calculated using the CFC contents in that subsurface water. The orange curve and its shading show the 5-year moving average values of atmospheric wet deposition (WD) of NOx from the US Atlantic Coast and the range of the 95 % confidence intervals, respectively (the monitoring sites are presented in Table S3). Blue curve indicates the NOx emission from the USA. The NOx emission strongly correlates with the WD of NOx (r=0.93, p<0.01).
Although there are limited data (time and space) on wet deposition of NOx, the temporal pattern based on measurements on the US Atlantic Coast is comparable to that for the NOx emissions. For example, based on data obtained from the National Atmospheric Deposition Program (NADP; http://nadp.slh.wisc.edu, last access: 15 May 2019), there was an increase from the 1980s to early 1990s, and the level remained high for approximately 15 years and then decreased (Fig. 5b). This trend of wet deposition of NOx was commonly found at AND monitoring sites located along the US Atlantic Coast (Table S3).
The AND signals can be transported to the subsurface waters of the mesopelagic western NAtl via two associated mechanisms. The first process involves production and bacterial oxidation of organic matter. In these biological processes, new anthropogenic nitrogen added by atmospheric deposition is removed from the surface via photosynthetic utilization by phytoplankton and gravitational sinking of the resulting organic matter with a N:P ratio higher than 15:1 (Singh et al., 2013). This N-rich organic matter is subsequently remineralized by bacteria at depth (Antia, 2005). This process involving well-known biological processes would facilitate the transfer of high surface N:P signals to the subsurface waters. The second process involves the physical transport of surface waters with greater N:P signals, which is a plausible mechanism for generating the subsurface AND signals observed in the western NAtl. High inputs of NOx by atmospheric deposition occur over the coastal areas of the NAtl and are mostly entrained in areas close to the northern edge of the western NAtl via the strong and persistent western boundary current (i.e., the Gulf Stream, Fig. 1). Both active winter mixing and the concurrent formation of mode water in this region would be expected to facilitate the transport of surface waters loaded with high DINxs (and anthropogenic CO2 and CFCs) to the subsurface layer and to spread these DINxs-loaded waters southward (Palter et al., 2005).
The substantial increase in subsurface DINxs after 1997 (approximately equal to the pCFC-12 ventilation year of 1982) at sites having greater inputs of AND (boxes 1–3 in Fig. 5a, and at the BATS site) appears to coincide with the increasing wet deposition of NOx from the US continent, with a lag period of approximately 15 years. The time lag observed is approximately equal to the ventilation age of the target subsurface waters in this region, which was estimated to be 6–25 years based on the CFC concentrations (Hansell et al., 2004, 2007). The time lag suggests that the physical mechanism is important in transporting the AND signals to the subsurface waters, although the mismatch between the observed time lag and the ventilation age of water masses may in part be because of biological processes, which could contribute to the elevation of N:P signals at depth through the oxidation of organic matter containing anthropogenic nitrogen.
The rates of DINxs increase (0.19–0.33 µmol kg−1 per decade; boxes 1–3 in Fig. 5a) measured in the western NAtl (reported in the preceding section) are equivalent to an increase of 78–135 mmol N m−2 per decade in the subsurface DIN inventory (200–600 m) of the western NAtl relative to the subsurface DIP inventory. This increase is slightly higher than the increase in wet NOx deposition (approximately 60 mmol N m−2 per decade) measured along the US East Coast from the 1980s to the 2000s (Fig. 5b) but is broadly consistent with the total NOx fluxes (approximately 90 mmol N m−2 per decade) if dry deposition is included in the modeled and observed results (Dentener et al., 2006; Baker et al., 2010). The atmospheric deposition possesses a considerably high N:P ratio (up to 1000; Baker et al., 2010), which would disproportionately contribute to the DIN inventory in the western NAtl. We thus suggest that anthropogenic nitrogen input is probably a main driver of DINxs increase in the western basin. A similar phenomenon, indicating an anthropogenic influence manifested in oceanic nutrient dynamics having a lag period of 20 years, has also been detected at 200–600 m in the Mediterranean Sea (Moon et al., 2016). They found a three-phase temporal transition (a trend of increase–stability–decline) in DIN concentration between 1985 and 2014; this was probably associated with corresponding changes in anthropogenic nitrogen input from the European continent.
The temporal trend of the nitrogen isotope record (CS-δ15N) measured on the Bermuda coral skeleton is comparable to the trends of NOx emission from the USA (Fig. S8), indicating that the AND signals have been embedded in the coral δ15N record. The CS-δ15N record on the Bermuda coral reflects the annual biological response to the local AND signals in the surface waters; hence, its trend may follow changes in anthropogenic NOx input without a time lag. For the western NAtl, the rates of DINxs increase we found are in agreement with those from the earlier studies using different datasets and methodologies (Hansell et al., 2007; Landolfi et al., 2008; Singh et al., 2013) but are lower than those observed in the NPO (0.30–1.20 µmol kg−1 per decade, I.-N. Kim et al., 2014). The different rates of seawater DINxs increase found between the western NAtl and NPO appear to be consistent with the CS-δ15N records in these two basins. During the 20th century, a small decline (−0.2 ‰) in CS-δ15N was observed in corals from Bermuda (Wang et al., 2018), whereas a greater decrease (−0.7 ‰) in CS-δ15N was detected from the South China Sea (Ren et al., 2017). The lower rates of seawater DINxs increase (or slower decline in CS-δ15N) in the NAtl were likely due to the lower rate of nitrogen emissions (also indicating nitrogen deposition) from the North American continent (0.15 Tg N yr−1 observed from 1970 to 2000; EPA, 2000) than from northeast Asia (0.40 Tg N yr−1 observed from 1980 to 2010; Liu et al., 2013). In this case, the recent trend of decreasing emission in anthropogenic nitrogen from North America, as well as the decrease in wet nitrogen deposition observed along the US East Coast, may reverse the pattern of the increase in subsurface DINxs in the western NAtl in the near future. Indeed, this reversed pattern appears to have emerged recently at the BATS site (Figs. 3a and 5b). Together, our findings suggest that the AND has affected the nutrient dynamics in the western NAtl, although the magnitude of this effect is relatively small, and its influence would be expected to become less significant under a scenario of increased control of pollutant emissions.
3.3 Biogeochemical processes that may affect the ΔDINxs in the western North Atlantic Ocean
Other biogeochemical processes may also affect the observed pattern of ΔDINxs in the western NAtl. Nitrogen fixation contributes considerably to the total export production (1.3–3.8 mol C m−2 yr−1; Lee, 2001) in oligotrophic gyres of the NAtl (Lee et al., 2002; Ko et al., 2018), which could therefore generate the positive signals of DINxs in subsurface waters (Hansell et al., 2004). The rate of N2 fixation and the abundance of diazotrophs have been reported to be highest (Luo et al., 2012; Benavides and Voss, 2015) in the subtropical gyre of the western NAtl (see boxes 4–6 in Fig. 5a); however, the subsurface DINxs did not change significantly among the repeat occupations of transects (Figs. 3 and S7a). No direct evidence for increasing activity of diazotrophs in the NAtl is available (Mahaffey et al., 2005; Benavides and Voss, 2015). Contrary to our expectation, the increase in subsurface DINxs was only found upstream of the subduction zone (north of the hot spots for N2 fixation; Figs. 3 and 5a). In this region (boxes 1–3 in Fig. 5a) the observed rate of N2 fixation was 4.2 mmol m−2 yr−1 (Luo et al., 2012), which is considerably lower than the modeled atmospheric NOx deposition (10–40 mmol m−2 yr−1; see Fig. 1). If N2 fixation mainly drives the increase in subsurface DINxs in this region, its rate would have been expected to increase by 2- to 3-fold during recent decades. Such an increase in N2 fixation activity is highly unlikely (Benavides and Voss, 2015). Moreover, if N2 fixation activity had increased during the study period we would have expected more DIP assimilated in the surface and subsequently remineralized in the thermocline, leading to an increase in DIP concentration in the thermocline (I.-N. Kim et al., 2014), but no thermocline DIP increase was observed (Fig. 4). Therefore, N2 fixation has probably not been a major factor leading to the increase in DINxs in the western NAtl over the study period.
Remineralization of particulate and dissolved organic matter (POM and DOM) is another potential source of subsurface DINxs in the NAtl, as a result of the high N:P ratios of organic matter and the preferential remineralization of P from POM and DOM (Landolfi et al., 2008; Lomas et al., 2010). The DON concentration in the subsurface waters in the western NAtl (near the BATS site), however, remained unchanged during the period 1998–2011 (http://bats.bios.edu/, last access: 20 February 2020). Moreover, the N:P ratios in DOM and suspended POM obtained at 0–100 m at the BATS site did not change between 2004 and 2012 (Singh et al., 2015). Likewise, we did not find any discernible interannual changes in the N:P ratio of sinking particles collected between 150 and 300 m at the BATS site (Fig. S9). Thus, the change in subsurface DINxs in the western NAtl was not primarily driven by variable N:P ratios of sinking POM. Taken together, these findings suggest that DOM and POM remineralization has not contributed to the ΔDINxs in the subsurface waters of the western NAtl during the period of analysis. Having excluded N2 fixation and remineralization of organic matter as key drivers, we hypothesize that the addition of AND has been the major contributor to the recent increases in subsurface DINxs in the western NAtl.
3.4 Influences of climate variability on the ΔDINxs in the western North Atlantic Ocean
As a prevailing climate mode over the NAtl, the North Atlantic Oscillation (NAO) strongly influences the formation of the subtropical mode water (STMW) in the western NAtl, which in turn affects subsurface nutrient and DINxs concentrations in the downstream region (Bates and Hansell, 2004; Palter et al., 2005). The STMW is known to form in areas south of the Gulf Stream extension and then primarily flows southward to the entire western basin; its intrusion to the eastern basin has been suggested to be minor (Palter et al., 2005, 2011). The formation of the STMW is generally enhanced when the NAO index becomes negative (Rodwell et al., 1999). During the negative phase of the NAO, an increased contribution of low-nutrient water to the STMW lowers the subsurface nutrient concentrations and DINxs in the subtropical gyre. In contrast, during the positive phase of the NAO, the STMW formation becomes weaker, and thus the subsurface nutrient concentrations and DINxs would increase downstream of the STMW formation region (Palter et al., 2005). The winter (December–March) NAO index has been mostly positive values since 1980, although its trend appeared to show an increase before the early 1990s and to decrease slightly thereafter (Fig. S10). Contrary to the trend in this atmospheric forcing, our nutrient data showed no evident changes in the subsurface DIP in the downstream region (e.g., A22 and A20; p>0.05, Student's t test and ANOVA with Games–Howell test; Figs. 4 and S7b) over the past 3 decades, irrespective of changes in the NAO index. These observations indicate that the basin-wide ΔDINxs signals are probably less likely controlled by a persistent positive phase of the NAO. Time-series data further strengthened the conclusion drawn from the basin-scale data. For example, the decline in the Bermuda CS-δ15N value was accompanied by several superimposed decadal oscillations induced by the NAO (Wang et al., 2018). Similarly, such oscillations appear to be imprinted in the time-series measurements of subsurface DINxs at the BATS site (Fig. 5b). Nonetheless, the basin-wide ΔDINxs trends induced by anthropogenic inputs of nitrogen are still visible.
3.5 Subsurface ΔDINxs trend in the eastern North Atlantic Ocean
There was an apparent decrease in subsurface DINxs in the eastern NAtl (e.g., A16N), which is the opposite trend to that found in the western NAtl (Fig. 5a). The decreasing trend (−0.40 µmol kg−1 per decade) in the subsurface DINxs in the eastern subpolar NAtl (45–60∘ N along A16N) has been more evident since the 2000s (Fig. 3c). A significant decrease in the subsurface (300–500 m) DIN between 1998 and 2013 was also found at a site (68.0∘ N, 12.7∘ W) in the northern Iceland Sea, but no concurrent decrease in DIP was observed (Fig. S12). As a result, the subsurface DINxs therein declined remarkedly after 2005.
A decrease in the rate of anthropogenic N input from Europe is a probable explanation for the decrease in subsurface DINxs in the eastern NAtl. From 1999 to 2009, NOx emission from Europe has decreased by 31 %, mainly owing to a change in energy consumption from fossil fuels to nuclear power (Vet et al., 2014). This decline in recent NOx emission from Europe (the blue solid line in Fig. S11) may cause the decrease rate of AND in the eastern subtropical and subpolar NAtl. However, much of the NOx derived from Europe is probably deposited to the European coasts, because the prevailing westerly winds carry it eastward to the eastern European continent (Fig. 1) (Baker et al., 2010). Moreover, the amounts of NOx deposited onto the eastern subpolar basin (<10 mmol N m−2 yr−1) were found to be small (Fig. 1). In the extreme scenario in which no such NOx deposition occurred during the period of analysis, the lack of this NOx deposition would only account for <20 % of the total decline in subsurface DINxs in the eastern subpolar NAtl (Fig. 2c). This suggests that the influence of European AND on seawater nutrient dynamics in the eastern subpolar NAtl is small. It is unclear what other processes are sufficiently large to account for the subsurface DINxs decrease in the eastern subpolar NAtl. Candidates include the potential decrease in N2 fixation caused by a reduction in the supply of iron and DIP from Africa to the eastern NAtl (Foltz and McPhaden, 2008; Ridley et al., 2014) and the recently observed weakening of the Atlantic meridional overturning circulation (AMOC) (Srokosz and Bryden, 2015; Robson et al., 2016). However, time-resolved data are needed to enable future assessment of these processes.
Our results support that AND has been a cause of the temporal variations in seawater DINxs in the subsurface waters of the western NAtl during the recent 2 decades. In the eastern NAtl, a decreasing trend in the subsurface DINxs in the high latitudes observed after the 2000s was not driven by the comparable decrease in AND from Europe. A possible decline in tropical N2 fixation and weakening of the AMOC are viable explanations. However, we do not have data to support our hypothesis. Our study shows that both human activities and natural variations together exert a discernable impact on the decadal variations of DINxs in the subsurface waters of the NAtl.
Human activities may have begun to influence the concentrations and stoichiometry of nutrients, at least in the western NAtl, and profound changes have been verified on the western NPO (I.-N. Kim et al., 2014) and Mediterranean Sea (Moon et al., 2016). These findings indicate global-scale changes in marine biogeochemistry, caused by human activities that are simultaneously influencing carbon sequestration and greenhouse gas emission (e.g., N2O) (Duce et al., 2008). Continuing observations of change in DINxs in the NAtl are needed to determine whether the levels have followed the recent decrease in AND, particularly from the USA. Such external perturbations could also alter the close homeostasis of the marine N cycle and its feedback to climate (Gruber and Deutsch, 2014).
All datasets used in our study can be downloaded from https://www.ncei.noaa.gov/data/oceans/ncei/ocads/data/0162565/data_product/ (GLODAPv2 group, 2020).
The supplement related to this article is available online at: https://doi.org/10.5194/bg-17-3631-2020-supplement.
JYTY and KL designed the present work and drafted the manuscript. JYTY and JYM performed the data analysis. JZZ, ISH, JSL, and EL contributed to discussion and interpretation of the data.
The authors declare that they have no conflict of interest.
This article is part of the special issue “Atmospheric deposition in the low-nutrient–low-chlorophyll (LNLC) ocean: effects on marine life today and in the future (ACP/BG inter-journal SI)”. It is not associated with a conference.
We wish to thank all of scientists who contributed to data used in this study. This work was supported by the National Institute of Fisheries Science (R2020044) funded by the Ministry of Ocean and Fisheries (MOF). Additional support for Jin-Yu Terence Yang was provided by the Principal's Fund of Xiamen University (ZK1114). Jia-Zhong Zhang was supported by NOAA Ocean and Atmospheric Research. The scientific results and conclusions, as well as any views or opinions expressed herein, are those of the authors and do not necessarily reflect the views of NOAA or the US Department of Commerce. This is the State Key Laboratory of Marine Environmental Science contribution no. melpublication2020387.
This research has been supported by the National Institute of Fisheries Science (grant no. R2020044) and the Xiamen University (grant no. ZK1114).
This paper was edited by Jan-Berend Stuut and reviewed by two anonymous referees.
Altieri, K. E., Hastings, M. G., Peters, A. J., Oleynik, S., and Sigman, D. M.: Isotopic evidence for a marine ammonium source in rainwater at Bermuda, Global Biogeochem. Cy., 28, 1066–1080, https://doi.org/10.1002/2014GB004809, 2014.
Altieri, K. E., Fawcett, S. E., Peters, A. J., Sigman, D. M., and Hastings, M. G.: Marine biogenic source of atmospheric organic nitrogen in the subtropical North Atlantic, P. Natl. Acad. Sci. USA, 113, 925–930, https://doi.org/10.1073/pnas.1516847113, 2016.
Anderson, L. A. and Sarmiento, J. L.: Redfield ratios of remineralization determined by nutrient data analysis, Global Biogeochem. Cy., 8, 65–80, https://doi.org/10.1029/93GB03318, 1994.
Antia, A. N.: Solublization of particles in sediment trap, revising the stoichiometry of mixed layer export, Biogeosciences, 2, 189–204, https://doi.org/10.5194/bg-2-189-2005, 2005.
Baker, A. R., Lesworth, T., Adams, C., Jickells, T. D., and Ganzeveld, L.: Estimation of atmospheric nutrient inputs to the Atlantic Ocean from 50∘ N to 50∘ S based on large-scale field sampling: Fixed nitrogen and dry deposition of phosphorus, Global Biogeochem. Cy., 24, GB3006, https://doi.org/10.1029/2009GB003634, 2010.
Baringer, M., Bullister, J., Feely, R., Wanninkhof, R., Millero, F., Hansell, D., Zhang, J.-Z., Mordy, C., Langdon, C., Schlosser, P., Jenkins, W., McNichol A., and Key, R.: Carbon Dioxide, Hydrographic, and Chemical Data Obtained During the R/V Ronald H. Brown Cruise in the Atlantic Ocean on GO-SHIP/CLIVAR Repeat Hydrography Section A16N (3 August–1 October 2013). Carbon Dioxide Information Analysis Center, Oak Ridge National Laboratory, US Department of Energy, Oak Ridge, Tennessee, https://doi.org/10.3334/CDIAC/OTG.GOSHIP_A16N_2013, 2014
Bates, N. R. and Hansell, D. A.: Temporal variability of excess nitrate in the subtropical mode water of the North Atlantic Ocean, Mar. Chem., 84, 225–241, https://doi.org/10.1016/j.marchem.2003.08.003, 2004.
Benavides, M. and Voss, M.: Five decades of N2 fixation research in the North Atlantic Ocean, Front. Mar. Sci., 2, 1–20, https://doi.org/10.3389/fmars.2015.00040, 2015.
Dentener, F., Drevet, J., Lamarque, J. F., Bey, I., Eickhout, B., Fiore, A. M., Hauglustaine, D., Horowitz, L. W., Krol, M., Kulshrestha, U. C., Lawrence, M., Galy-Lacaux, C., Rast, S., Shindell, D., Stevenson, D., Van Noije, T., Atherton, C., Bell, N., Bergman, D., Butler, T., Cofala, J., Collins, B., Doherty, R., Ellingsen, K., Galloway, J., Gauss, M., Montanaro, V., Müller, J. F., Pitari, G., Rodriguez, J., Sanderson, M., Solmon, F., Strahan, S., Schultz, M., Sudo, K., Szopa, S., and Wild, O.: Nitrogen and sulfur deposition on regional and global scales: A multimodel evaluation, Global Biogeochem. Cy., 20, GB4003, https://doi.org/10.1029/2005gb002672, 2006.
Doney, S. C., Mahowald, N., Lima, I., Feely, R. A., Mackenzie, F. T., Lamarque, J. -F., and Rasch, P. J.: Impact of anthropogenic atmopheric nitrogen and sulfur deposition on ocean acidification and the inorganic carbon system, P. Natl. Acad. Sci. USA, 104, 14580–14585, https://doi.org/10.1073/pnas.0702218104, 2007.
Duce, R. A., LaRoche, J., Altieri, K., Arrigo, K. R., Baker, A. R., Capone, D. G., Cornell, S., Dentener, F., Galloway, J., Ganeshram, R. S., Geider, R. J., Jickells, T., Kuypers, M. M., Langlois, R., Liss, P. S., Liu, S. M., Middelburg, J. J., Moore, C. M., Nickovic, S., Oschlies, A., Pedersen, T., Prospero, J., Schlitzer, R., Seitzinger, S., Sorensen, L. L., Uematsu, M., Ulloa, O., Voss, M., Ward, B., and Zamora, L.: Impacts of atmospheric anthropogenic nitrogen on the open ocean, Science, 320, 893–897, https://doi.org/10.1126/science.1150369, 2008
EPA: National Air Pollutant Emission Trends, 1900–1998, Office of Air Quality Planning and Standards, Research Triangle Park, A7–A10, 2000.
Fanning, K. A.: Influence of atmospheric pollution on nutrient limitation in the ocean, Nature, 339, 460–463, https://doi.org/10.1038/339460a0, 1989.
Foltz, G. R. and McPhaden, M. J.: Trends in Saharan dust and tropical Atlantic climate during 1980–2006, Geophys. Res. Lett., 35, L20706, https://doi.org/10.1029/2008GL035042, 2008.
Fowler, D., Pyle, J. A., Raven, J. A., and Sutton, M. A.: The golbal nitrogen cycle in the twenty-first century, Philos. T. R. Soc. Lond. B, 368, 1–13, https://doi.org/10.1098/rstb.2013.0164, 2013.
Galloway, J. N., Howarth, R. W., Michaels, A. F., Nixon, S. W., Prospero, J. M., and Dentener, F. J.: Nitrogen and phosphorus budgets of the North Atlantic Ocean and its watershed, Biogeochemistry, 35, 3–25, https://doi.org/10.1007/BF02179823, 1996.
GLODAPv2 group: GLODAPv2 Atlantic Ocean, available at: https://www.ncei.noaa.gov/data/oceans/ncei/ocads/data/0162565/data_product/, last access: 10 July 2020.
Gruber, N. and Deutsch, C. A.: Redfield's evolving legacy, Nat. Geosci., 7, 853–855, https://doi.org/10.1038/ngeo2308, 2014.
Gruber, N. and Sarmiento, J.: Global patterns of marine nitrogen fixation and denitrification, Global Biogeochem. Cy., 11, 235–266, https://doi.org/10.1029/97GB00077, 1997.
Hansell, D. A., Bates, N. R., and Olson, D. B.: Excess nitrate and nitrogen fixation in the North Atlantic Ocean, Mar. Chem., 84, 243–265, https://doi.org/10.1016/j.marchem.2003.08.004, 2004.
Hansell, D. A., Olson, D. B., Dentener, F., and Zamora, L. M.: Assessment of excess nitrate development in the subtropical North Atlantic, Mar. Chem., 106, 562–579, https://doi.org/10.1016/j.marchem.2007.06.005, 2007.
Hastings, M. G., Sigman, D. M., and Lipschultz, F.: Isotopic evidence for source changes of nitrate in rain at Bermuda, J. Geophys. Res.-Atmos., 108, 4790, https://doi.org/10.1029/2003JD003789, 2003.
Hydes, D., Aoyama, M., Aminot, A., Bakker, K., Becker, S., Coverly, S., Daniel, A., Dickson, A., Grosso, O., Kerouel, R., van Ooijen, J., Sato, K., Tanhua, T., Woodward, M., and Zhang, J.: Determination of dissolved nutrients (N, P, Si) in seawater with high precision and inter-comparability using gas-segmented continuous flow analysers, in: The GO-SHIP Repeat Hydrography Manual: A Collection of Expert Reports and guidelines. IOCCP Report No 14, ICPO Publication Series No. 134, version 1, UNESCO/IOC., 87, 2010.
Jickells, T. and Moore, C. M.: The importance of atmospheric deposition for ocean productivity, Annu. Rev. Ecol. Evol. S., 46, 481–501, https://doi.org/10.1146/annurev-ecolsys-112414-054118, 2015.
Jickells, T. D., Buitenhuis, E., Altieri, K., Baker, A. R., Capone, D., Duce, R. A., Dentener, F., Fennel, K., Kanakidou, M., LaRoche, J., Lee, K., Liss, P., Middelburg, J. J., Moore, J. K., Okin, G., Oschlies, A., Sarin, M., Seitzinger, S., Sharples, J., Singh, A., Suntharalingam, P., Uematsu, M., and Zamora, L. M.: A reevaluation of the magnitude and impacts of anthropogenic atmospheric nitrogen inputs on the ocean, Global Biogeochem. Cy., 31, 289–305, https://doi.org/10.1002/2016GB005586, 2017.
Key, R. M., Olsen, A., van Heuven, S., Lauvset, S. K., Velo, A., Lin, X., Schirnick, C., Kozyr, A., Tanhua, T., Hoppema, M., Jutterström, S., Steinfeldt, R., Jeansson, E., Ishii, M., Perez, F. F., and Suzuki, T.: Global Ocean Data Analysis Project, Version 2 (GLODAPv2), (ORNL/CDIAC-162, ND-P093), Carbon Dioxide Information Analysis Center, Oak Ridge National Laboratory, US Department of Energy, 1–9, 2015.
Kim, I.-N., Lee, K., Gruber, N., Karl, D. M., Bullister, J. L., Yang, S., and Kim, T.-W.: Increasing anthropogenic nitrogen in the North Pacific Ocean, Science, 346, 1102–1106, https://doi.org/10.1126/science.1258396, 2014.
Kim, T.-W., Lee, K., Najjar, R. G., Jeong, H. D., and Jeong, H. J.: Increasing N abundance in the northwestern Pacific Ocean due to atmospheric nitrogen deposition, Science, 334, 505–509, https://doi.org/10.1126/science.1206583, 2011.
Kim, T.-W., Lee, K. Duce, R., and Liss, P.: Impact of atmospheric nitrogen deposition on phytoplankton productivity in the South China Sea, Geophys. Res. Lett., 41, 3156–3162, https://doi.org/10.1002/2014GL059665, 2014.
Knapp, A. N., Hastings, M. G., Sigman, D. M., Lipschultz, F., and Galloway, J. N.: The flux and isotopic composition of reduced and total nitrogen in Bermuda rain, Mar. Chem., 120, 83–89, https://doi.org/10.1016/j.marchem.2008.08.007, 2010.
Ko, Y. H., Lee, K., Takahashi, T., Karl, D. M., Kang, S.-H., and Lee, E.: Carbon-based estimate of nitrogen-fixation-derived net community production in N-depleted ocean gyres, Global Biogeochem. Cy., 32, 1241–1252, https://doi.org/10.1029/2017GB005634, 2018.
Landolfi, A., Oschlies, A., and Sanders, R.: Organic nutrients and excess nitrogen in the North Atlantic subtropical gyre, Biogeosciences, 5, 1199–1213, https://doi.org/10.5194/bg-5-1199-2008, 2008.
Lee, K.: Global net community production estimated from the annual cycle of surface water total dissolved inorganic carbon, Limnol. Oceanogr., 46, 1287–1297, https://doi.org/10.4319/lo.2001.46.6.1287, 2001.
Lee, K., Karl, D. M., Wanninkhof, R., and Zhang, J.-Z.: Global estimates of net carbon production in the nitrate-depleted tropical and subtropical oceans, Geophys. Res. Lett., 29, 1907, https://doi.org/10.1029/2001GL014198, 2002.
Liu, X. Zhang, Y., Han, W., Tang, A., Shen, J., Cui, Z., Vitousek, P., Erisman, J. W., Goulding, K., Christie, P., Fangmeier, A., and Zhang, F.: Enhanced nitrogen deposition over China, Nature, 494, 459–462, https://doi.org/10.1038/nature11917, 2013.
Lomas, M. W., Burke, A. L., Lomas, D. A., Bell, D. W., Shen, C., Dyhrman, S. T., and Ammerman, J. W.: Sargasso Sea phosphorus biogeochemistry: an important role for dissolved organic phosphorus (DOP), Biogeosciences, 7, 695–710, https://doi.org/10.5194/bg-7-695-2010, 2010.
Luo, Y. W., Doney, S. C., Anderson, L. A., Benavides, M., Berman-Frank, I., Bode, A., Bonnet, S., Boström, K. H., Böttjer, D., Capone, D. G., Carpenter, E. J., Chen, Y. L., Church, M. J., Dore, J. E., Falcón, L. I., Fernández, A., Foster, R. A., Furuya, K., Gómez, F., Gundersen, K., Hynes, A. M., Karl, D. M., Kitajima, S., Langlois, R. J., LaRoche, J., Letelier, R. M., Marañón, E., McGillicuddy Jr., D. J., Moisander, P. H., Moore, C. M., Mouriño-Carballido, B., Mulholland, M. R., Needoba, J. A., Orcutt, K. M., Poulton, A. J., Rahav, E., Raimbault, P., Rees, A. P., Riemann, L., Shiozaki, T., Subramaniam, A., Tyrrell, T., Turk-Kubo, K. A., Varela, M., Villareal, T. A., Webb, E. A., White, A. E., Wu, J., and Zehr, J. P.: Database of diazotrophs in global ocean, abundance, biomass and nitrogen fixation rates, Earth Syst. Sci. Data, 4, 47–73, https://doi.org/10.5194/essd-4-47-2012, 2012.
Mahaffey, C., Michaels, A. F., and Capone, D. G.: The conundrum of marine N2 fixation, Am. J. Sci. 305, 546–595, https://doi.org/10.2475/ajs.305.6-8.546, 2005.
Martiny, A. C., Lomas, M. W., Fu, W., Boyd, P. W., Chen, Y.-l. L., Cutter, G. A., Ellwood, M. J., Furuya, K., Hashihama, F., Kanda, J., Karl, D. M., Kodama, T., Li, Q. P., Ma, J., Moutin, T., Woodward, E. M. S., and Moore, J. K.: Biogeochemical controls of surface ocean phosphate, Sci. Adv., 5, eaax0341, https://doi.org/10.1126/sciadv.aax0341, 2019.
Moon, J.-Y., Lee, K., Tanhua, T., Kress, N., and Kim, I.-N.: Temporal nutrient dynamics in the Mediterranean Sea in response to anthropogenic inputs, Geophys. Res. Lett., 43, 5243–5251, https://doi.org/10.1002/2016GL068788, 2016.
Moore, C. M.: Diagnosing oceanic nutrient deficiency, Philos. T. R. Soc. A, 374, 20150290, https://doi.org/10.1098/rsta.2015.0290, 2016.
Moore, C. M., Mills, M. M., Arrigo, K. R., Berman-Frank, I., Bopp, L., Boyd, P. W., Galbraith, E. D., Geider, R. J., Guieu, C., Jaccard, S. L., Jickells, T. D., La Roche, J., Lenton, T. M., Mahowald, N. M., Marañón, E., Marinov, I., Moore, J. K., Nakatsuka, T., Oschlies, A., Saito, M. A., Thingstad, T. F., Tsude, A., and Ulloa, O.: Processes and patterns of oceanic nutrient limitation, Nat. Geosci., 6, 701–710, https://doi.org/10.1038/ngeo1765, 2013.
Okin, G. S., Baker, A. R., Tegen, I., Mahowald, N. M., Dentener, F. J., Duce, R. A., Galloway, J. N., Hunter, K., Kanakidou, M., Kubilay, N., Prospero, J. M., Sarin, M., Surapipith, V., Uematsu, M., and Zhu, T.: Impacts of atmospheric nutrient deposition on marine productivity, Roles of nitrogen, phosphorus, and iron, Global Biogeochem. Cy., 25, GB2022, https://doi.org/10.1029/2010GB003858, 2011.
Olsen, A., Key, R. M., van Heuven, S., Lauvset, S. K., Velo, A., Lin, X., Schirnick, C., Kozyr, A., Tanhua, T., Hoppema, M., Jutterström, S., Steinfeldt, R., Jeansson, E., Ishii, M., Pérez, F. F., and Suzuki, T.: The Global Ocean Data Analysis Project version 2 (GLODAPv2) – an internally consistent data product for the world ocean, Earth Syst. Sci. Data, 8, 297–323, https://doi.org/10.5194/essd-8-297-2016, 2016.
Palter, J. B., Lozier, M. S., and Barber, R. T.: The effect of advection on the nutrient reservoir in the North Atlantic subtropical gyre, Nature, 437, 687-692, https://doi.org/10.1038/nature03969, 2005.
Palter, J. B., Lozier, M. S., Samiento, J. L., and Williams, R. G.: The supply of excess phosphate across the Gulf Stream and the maintenance of subtropical nitrogen fixation, Global Biogeochem. Cy., 25, GB4007, https://doi.org/10.1029/2010GB003955, 2011.
Ren, H., Chen, Y.-C., Wang, X. T., Wong, G. T. F., Cohen, A. L., DeCarlo, T. M., Weigand, M. A., Mii, H.-S., and Sigman, D. M.: 21st-century rise in anthropogenic nitrogen deposition on a remote coral reef, Science, 356, 749–752, https://doi.org/10.1126/science.aal3869, 2017.
Ridley, D. A., Heald, C. L., and Prospero, J. M.: What controls the recent changes in African mineral dust aerosol across the Atlantic?, Atoms. Chem. Phys., 14, 5735–5747, https://doi.org/10.5194/acp-14-5735-2014, 2014.
Ríos, A. F., Resplandy, L., García-Ibáñez, M. I., Fajar, N. M., Velo, A., Padin, X. A., Wanninkhof, R., Steinfeldt, R., Rosón, G., and Pérez, F. F.: Decadal acidification in the water masses of the Atlantic Ocean, P. Natl. Acad. Sci. USA, 112, 9950–9955, https://doi.org/10.1073/pnas.1504613112, 2015.
Rodwell, M. J., Rowell, D. P., and Folland, C. K.: Oceanic forcing of the wintertime North Atlantic Oscillation and European climate, Nature, 398, 320–323, https://doi.org/10.1038/18648, 1999.
Robson, J., Ortega, P., and Sutton, R.: A reversal of climatic trends in the North Atlantic since 2005, Nat. Geosci., 9, 513–517, https://doi.org/10.1038/ngeo2727, 2016.
Singh, A., Lomas, M. W., and Bates, N. R.: Revisiting N2 fixation in the North Atlantic Ocean: Significance of deviations from the Redfield Ratio, atmospheric deposition and climate variability, Deep-Sea Res. Pt. II, 93, 148–158, https://doi.org/10.1016/j.dsr2.2013.04.008, 2013.
Singh, A., Baer, S. E., Riebesell, U., Martiny, A. C., and Lomas, M. W.: C, N, P stoichiometry at the Bermuda Atlantic Time-series Study station in the North Atlantic Ocean, Biogeosciences, 12, 6389–6403, https://doi.org/10.5194/bg-12-6389-2015, 2015.
Srokosz, M. A. and Bryden, H. L.: Observing the Atlantic Meridional Overturning Circulation yields a decade of inevitable surprises, Science, 348, 1330, https://doi.org/10.1126/science.1255575, 2015.
St-Laurent, P., Friedrichs, M. A. M., Najjar, R. G., Martins, D. K., Herrmann, M., Miller, S. K., and Wilkin, J.: Impacts of atmospheric nitrogen deposition on surface waters of the western North Atlantic mitigated by multiple feedbacks, J. Geophys. Res.-Ocean., 122, 8406–8426, https://doi.org/10.1002/2017JC013072, 2017.
Takahashi, T., Broecker, W. S., and Langer, S.: Redfield ratio based on chemical data from isopycnal surfaces, J. Geophys. Res., 90, 6907–6924, https://doi.org/10.1029/JC090iC04p06907, 1985.
Vet, R., Artz, R. S., Carou, S., Shaw, M., Ro, C.-U., Aas, W., Baker, A., Bowersox, V. C., Dentener, F., Galy-Lacaux, C., Hou, A., Pienaar, J. J., Gillett, R., Forti, M. C., Gromov, S., Hara, H., Khodzher, T., Mahowald, N. M., Nickovic, S., Rao, P. S. P., and Reid, N. W.: A global assessment of precipitation chemistry and deposition of sulfur, nitrogen, sea salt, base cations, organic acids, acidity and pH, and phosphorus, Atmos. Environ., 93, 3–100, https://doi.org/10.1016/j.atmosenv.2013.10.060, 2014.
Wang, X. T., Cohen, A. L., Luu, V., Ren, H., Su, Z., Haug, G. H., and Sigman, D. M.: Natural forcing of the North Atlantic nitrogen cycle in the Anthropocene, P. Natl. Acad. Sci. USA, 115, 10606–10611, https://doi.org/10.1073/pnas.1801049115, 2018.
Woosley, R. J., Millero, F. J., and Wanninkhof, R.: Rapid anthropogenic changes in CO2 and pH in the Atlantic Ocean: 2003–2014, Global Biogeochem. Cy., 30, 70–90, https://doi.org/10.1002/2015GB005248, 2016.
Yang, J. Y. T., Hsu, S. C., Dai, M. H., Hsiao, S. S. Y., and Kao, S. J.: Isotopic composition of water-soluble nitrate in bulk atmospheric deposition at Dongsha Island, sources and implications of external N supply to the northern South China Sea, Biogeosciences, 11, 1833–1846, https://doi.org/10.5194/bg-11-1833-2014, 2014.
Yang, S. and Gruber, N.: The anthropogenic perturbation of the marine nitrogen cycle by atmospheric deposition, Nitrogen cycle feedbacks and the 15N Habor-Bosch effect, Global Biogeochem. Cy., 30, 1418–1440, https://doi.org/10.1002/2016GB005421, 2016.
Zamora, L. M., Landolfi, A., Oschlies, A., Hansell, D. A., Dietze, H., and Dentener F.: Atmospheric deposition of nutrients and excess N formation in the North Atlantic, Biogeosciences, 7, 777–793, https://doi.org/10.5194/bg-7-777-2010, 2010.
Zhang, J.-Z., Mordy, C. W., Gordon, L. I., Ross, A., and Garcia, H. E..: Temporal trends in deep ocean Redfield ratios, Science, 289, 1839–1839, https://doi.org/10.1126/science.289.5486.1839a, 2000.
Zhang, J.-Z., Wanninkhof, R., and Lee, K.: Enhanced new production observed from the diurnal cycle of nitrate in an oligotrophic anticyclonic eddy, Geophys. Res. Lett., 28, 1579–1582, https://doi.org/10.1029/2000GL012065, 2001.