the Creative Commons Attribution 4.0 License.
the Creative Commons Attribution 4.0 License.
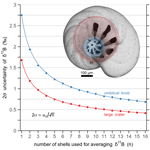
Technical note: Single-shell δ11B analysis of Cibicidoides wuellerstorfi using femtosecond laser ablation MC-ICPMS and secondary ion mass spectrometry
Claire Rollion-Bard
Ingo Horn
Grit Steinhoefel
Albert Benthien
Klaus-Uwe Richter
Matthieu Buisson
Pascale Louvat
Jelle Bijma
The boron isotopic composition (δ11B) of benthic foraminifera provides a valuable tool to reconstruct past deep-water pH. As the abundance of monospecific species might be limited in sediments, microanalytical techniques can help to overcome this problem, but such studies on benthic foraminiferal δ11B are sparse. In addition, microanalytics provide information on the distribution of δ11B at high spatial resolution to increase the knowledge of biomineralization processes, for example. For this study, we investigated the intra- and inter-shell δ11B variability of the epibenthic species Cibicidoides wuellerstorfi, which is widely used in paleoceanography, by secondary ion mass spectrometry (SIMS) and femtosecond laser ablation multicollector inductively coupled plasma mass spectrometry (LA-MC-ICPMS). While the average δ11B values obtained from these different techniques agree remarkably well with bulk solution values to within ±0.1 ‰, a relatively large intra-shell variability was observed. Based on multiple measurements within single shells, the SIMS and LA data suggest median variations of 4.8 ‰ and 1.3 ‰ (2σ), respectively, while the larger spread for SIMS is attributed to the smaller volume of calcite being analyzed in each run. When analytical uncertainties and volume-dependent differences in δ11B variations are taken into account for these methods, the intra-shell variability is estimated to be on the order of ∼3 ‰ and ∼0.4 ‰ (2σ) on a ∼20 and 100 µm scale, respectively. In comparison, the δ11B variability between shells exhibits a total range of ∼3 ‰ for both techniques, suggesting that several shells need to be analyzed for accurate mean δ11B values. Based on a simple resampling method, we conclude that ∼12 shells of C. wuellerstorfi must be analyzed using LA-MC-ICPMS to obtain an accurate average value within ±0.5 ‰ (2σ) to resolve pH variations of ∼0.1. Based on our findings, we suggest preferring the conventional bulk solution MC-ICPMS over the in situ methods for paleo-pH studies, for example. However, SIMS and LA provide powerful tools for high-resolution paleoreconstructions, or for investigating ontogenetic trends in δ11B.
- Article
(2843 KB) - Full-text XML
-
Supplement
(124 KB) - BibTeX
- EndNote
The boron isotopic composition (δ11B) of benthic foraminifera has been used to reconstruct deep-water pH (Hönisch et al., 2008; Rae et al., 2011; Raitzsch et al., 2020; Yu et al., 2010) and to estimate the Cenozoic evolution of seawater δ11B (Raitzsch and Hönisch, 2013). The underlying mechanism behind the boron isotope method lies in the constant equilibrium fractionation of 27.2 ‰±0.6 ‰ between the pH-dependent speciation of trigonal boric acid and the tetrahedral borate in seawater (Klochko et al., 2006), where only the borate ion is incorporated into the foraminifera test (Branson et al., 2015; Hemming and Hanson, 1992).
However, while the number of studies on planktonic foraminiferal δ11B to estimate surface-ocean pH has rapidly increased within the last decade, deep-sea pH reconstructions based on benthic foraminifera are relatively rare (Hönisch et al., 2008; Rae et al., 2011; Raitzsch et al., 2020; Yu et al., 2010). Possible reasons for this might be the lower abundance of benthic foraminifera, compared to planktonic species, and a limited selection of species that truly record bottom-water rather than pore-water conditions (Rae et al., 2011). Fortunately, there are two suitable candidates, Cibicidoides wuellerstorfi and Cibicidoides mundulus, that cover a relatively large oceanographic and stratigraphic range, and which have a high boron content of ∼12–27 ppm (Raitzsch et al., 2011; Yu and Elderfield, 2007). Although their high [B] may partly compensate for the low abundance in the sediments, in many cases the availability of enough specimens for δ11B analysis remains limiting.
Here, microanalytical techniques such as laser ablation multicollector inductively coupled plasma mass spectrometry (LA-MC-ICPMS) and secondary ion mass spectrometry (SIMS) can help to overcome the problem of sample limitation. These techniques have already been successfully used for a variety of biogenic carbonates to gain information on biomineralization processes or seasonal pH variations (e.g., Blamart et al., 2007; Fietzke et al., 2015; Howes et al., 2017; Kaczmarek et al., 2015a; Mayk et al., 2020; Rollion-Bard and Erez, 2010; Sadekov et al., 2019). However, microanalytical analysis of δ11B is usually afflicted with larger uncertainties in terms of repeatability and reproducibility, as well as of natural δ11B heterogeneity within single shells and within a population. In addition, some recent studies using LA-MC-ICPMS suggest correction modes for measured δ11B values because detected interferences on the 10B peak, possibly due to scattered Ca ions from the carbonate sample, can result in large offsets from the expected value (Thil et al., 2016; Sadekov et al., 2019; Standish et al., 2019), whereas in other studies this matrix-induced effect was not observed (Fietzke et al., 2010; Kaczmarek et al., 2015b; Mayk et al., 2020).
Also, the reported analytical reproducibility for δ11B in biogenic carbonate using LA-MC-ICPMS differs considerably among different studies, ranging between ±0.22 ‰ and 1.60 ‰ (2σ=2 standard deviations), determined from repeated measurements of either a carbonate or glass standard (Fietzke et al., 2010; Kaczmarek et al., 2015b; Mayk et al., 2020; Sadekov et al., 2019; Standish et al., 2019; Thil et al., 2016). As there is no standardized protocol nor a commercially available homogenized δ11B carbonate standard for determining the analytical uncertainty of LA-MC-ICPMS, this issue remains the most challenging task to compare the different labs and instruments. The most commonly used carbonate standards with well-constrained boron isotopic compositions are samples from a coral (JCp-1) and a giant clam (JCt-1), provided by the Geological Survey of Japan (e.g., Inoue et al., 2004; Okai et al., 2004). However, for microanalytical analysis the standard is usually powdered in a mortar and finally pressed to a pellet, which is produced individually in each laboratory, thus potentially resulting in different heterogeneities (e.g., through different grain sizes or applied pressures) in each pellet. This issue is also true for SIMS analyses, and the reported reproducibility is strongly linked to the in-house reference material used (e.g., Kaseman et al., 2009; Rollion-Bard and Blamart, 2014).
In this study, we investigate a population of 23 specimens of C. wuellerstorfi, which is a widely used benthic foraminifer species in paleoceanographic studies, to extend our knowledge of δ11B variability within and between individuals. The aim of our study is to demonstrate the capabilities and limitations of δ11B analyses in C. wuellerstorfi on a microscale. For this purpose, we used the femtosecond LA-MC-ICPMS and SIMS techniques and compared the results with bulk-solution MC-ICPMS. Finally, we examine the size of population required for targeted δ11B uncertainty levels in paleoceanographic studies using LA-MC-ICPMS.
2.1 Foraminifer samples
For this study, we used sediment samples from GeoB core 1032-3, taken in the Angola Basin on the Walvis Ridge at a water depth of 2505 m. From a Holocene interval (6–8 cm, 5.6 ka), 23 pristine (glassy) shells of the benthic foraminifer species C. wuellerstorfi from the size fraction >350 µm were picked and prepared for subsequent microanalytical analysis. Five large specimens (>400 µm) were embedded in epoxy and polished down to a planar surface for SIMS analyses, while the remaining 18 specimens were mounted on carbon tape for LA measurements. From these 18 individuals, two large tests were analyzed for detailed chamber-to-chamber variability, while the remaining 16 tests were used to measure quasi-bulk δ11B by ablating large shell areas, preceded by measurements of the smaller umbilical knob area.
2.2 Secondary ion mass spectrometry
For the ion microprobe analyses, we used the same technique as described in Rollion-Bard et al. (2003) and Blamart et al. (2007). Boron isotopic compositions were measured with the Cameca ims 1270 ion microprobe at CRPG-CNRS, Nancy, France. A primary beam of 16O− ions generated using a radio frequency plasma source (Malherbe et al., 2016) with an intensity of 50 nA was focused to a spot of about 20 µm. A mass resolution of 3000 was used for B isotope analyses, allowing the elimination of all isobaric interferences. Boron isotopes were analyzed in mono-collection mode using the central electron multiplier. The dead time of the electron multiplier was determined before the analytical session and set to 65 ns. A pre-sputtering of 120 s was applied before the analysis itself. The typical intensities of 11B+ in foraminifer tests were between 2000 and 4500 counts per second (cps), depending on the boron concentration. The analysis consists of 60 cycles of 10 s for 10B+ and 6 s for 11B+, respectively. The reference material was a calcium carbonate with a B concentration of 22 ppm and a δ11B of 16.76 ‰±0.11 ‰, relative to the standard reference material (SRM) NIST 951 (WP22, value determined at IPGP using the method of Louvat et al., 2014). The reproducibility, as estimated by multiple measurements of the reference material, was 2.48 ‰ (2σ, n=8) and is very close to the predicted 2σ uncertainty derived from counting statistics.
2.3 Femtosecond laser ablation MC-ICPMS
Boron isotope measurements were performed using a customized UV-femtosecond laser ablation system coupled to a Plasma II MC-ICPMS (Nu Instruments) at the AWI, Bremerhaven. The laser ablation system is based on a Ti:sapphire regenerative amplifier system (Solstice, Spectra-Physics, USA) operating at the fundamental wavelength of 775 nm with a pulse width of 100 fs and pulse energy of 3.5 mJ per pulse. Consecutive frequency conversion results in an output beam with a wavelength in the UV spectra (193 nm) and a pulse energy of 0.08 mJ. The short femtosecond pulses were shown to have major advantages over nanosecond pulses for a wide range of element and isotope ratios with respect to laser-induced and particle-size-related fractionation, thus enabling non-matrix-matched calibrations (e.g., Horn and von Blanckenburg, 2007; Steinhoefel et al., 2009).
The sample and standard materials were mounted in an ablation chamber with an active volume of ca. 45 cm3 and ablated in a helix-mode scan at a speed of 2 mm s−1 by using a laser spot size of ∼40 µm. This technique allows ablation craters of almost any diameter to be produced, in this study ranging from ∼80 µm for analysis of single chambers to ∼400 µm to cover whole shells. The aerosol was transported via a He gas flow (∼0.5 L min−1) and admixed with Ar gas (∼0.5 L min−1) before entering the MC-ICP-MS. Torch position, ion optics and gas flows were optimized to gain maximum signal intensity and stability on 10B and 11B peaks. The mass spectrometer was equipped with standard Ni sample and skimmer cones for dry plasma conditions. The radio frequency power was set to 1300 W. Boron isotopes were determined on Daly detectors, where high-mass D5 was used for 11B and D0 for 10B. Each measured sample 11B∕10B was normalized to 11B∕10B measurements of the glass standard NIST SRM 610 (δ11B=0 ‰ NBS 951), using the standard-sample-bracketing technique. The analyses were performed at low mass resolution (, 5 ‰), which was sufficient to resolve all interferences.
We performed mass scans on the peaks of 10B and 11B for both gas blanks (laser off) and measurements on carbonate (laser on) (Fig. 1) to investigate possible effects by scattered ions of matrix elements as observed in some recent studies (Sadekov et al., 2019; Standish et al., 2019). For our set-up, we can exclude such matrix-induced effects, which is in line with Fietzke et al. (2010) and Mayk et al. (2020). Hence, there was no need to correct the raw LA data as done in the recent studies by Sadekov et al. (2019) and Standish et al. (2019). Before analysis, sample and standard materials were pre-ablated to remove potential surface contamination. Laser repetition rates ranged between 12 and 60 Hz to match the signal intensity between carbonate samples and standard material NIST SRM 610 (∼300 000 cps on 11B). As ablation efficiency and hence signal intensity may vary with progressively increasing surface roughness and crater depth, we adjusted the repetition rate, if required, to target intensity matching between sample and standard. Whereas this approach could result in bulgy time-resolved isotope signals, as shown in Fig. 2, clean calcium carbonate was identified from a plateau-like 11B ∕ 10B signal. Conversely, any contaminated phase from partial ablation of clay infillings, indicated by dropping 11B ∕ 10B ratios accompanied by rising [B], were excluded from further data treatment (Fig. 2).
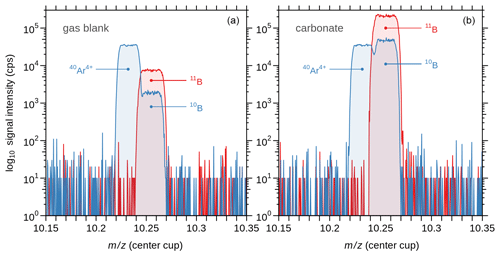
Figure 1Mass scans over atomic masses 10 (blue) and 11 (red) using Daly detectors, where peak center coincidence appears at ∼10.25 amu in the center cup. (a) Gas blank (laser off), showing the typical double peak of 40Ar4+ and 10B, and the 11B peak. (b) Signal of ablated calcium carbonate (laser on). The baseline exhibits only electronic noise from the Daly detectors, but no sign of unresolved interferences on 10B as matrix-induced scattered Ca ion. Note that the signal intensity is on a logarithmic scale.
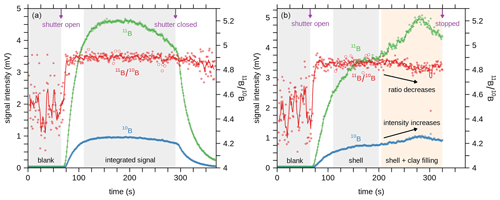
Figure 2(a) Example of time-resolved laser ablation analysis for 10B and 11B of a C. wuellerstorfi shell using Daly detectors, preceded by a ∼60 s blank measurement. Dots represent 1 s cycles, and lines 5-point running averages. Open symbols are data that are excluded by the 2σ outlier test. (b) Example of a shell that was penetrated by the laser beam, resulting in the ablation of clay infillings.
Each analysis was preceded by an on-peak gas blank measurement of 60 s on 10B and 11B, which was subtracted from the LA signal. The LA analysis itself was assessed by calculating the mean of the blank-corrected 11B∕10B signal within an interval of up to 370 cycles (1 s each), where all data exceeding two standard deviations were removed as outliers. After analysis, B was washed out for 120 to 180 s until reaching background levels before a new measurement was started. A typical blank had ∼7000 cps on 11B at the beginning of a session but decreased to less than 3000 cps during the course of a day. As signal intensity on 11B was aimed at ∼ 300 000 cps, the signal-to-noise ratio was on the order of ∼100.
Accuracy of boron isotope measurements was frequently checked by ablating an in-house carbonate standard that was also used for SIMS analysis (i.e. WP22, Rollion-Bard et al., 2003). The average δ11B of 16.49 ‰±1.26 ‰ (2σ, n=20) for WP22 is very close to the bulk solution values ( (2σ, n=6) measured at AWI, and measured at IPGP). As the measurement uncertainty is mainly dependent on the ablation time, we report measurement uncertainties (as 2σ) for each δ11B analysis as a function of analysis time, which was determined from multiple measurements of NIST glass standards and carbonate standards, and which is very close to the predicted uncertainty based on counting statistics (Fig. 3).
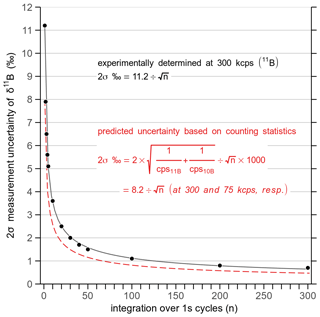
Figure 3Measurement uncertainty of 11B∕10B (2σ) at count rates of 300 000 cps (11B) as a function of the laser ablation time. The uncertainty of each boron isotope measurement is calculated based on this relationship (black solid line). A major portion (∼70 %) of the measurement uncertainty is related to Poisson-distributed counts (red dashed line).
2.4 Bulk solution MC-ICPMS
After LA analyses, the 18 shells were carefully removed from the carbon tape and cleaned following the procedure outlined by Raitzsch et al. (2018). Briefly, foraminifer shells were gently crushed under a binocular between two glass slides and transferred to Eppendorf vials. After the clay removal and oxidative cleaning steps, the samples were leached in 0.001 N HNO3 and finally dissolved in 60 µL of 1 N HNO3.
Prior to boron isotope analysis, we used the micro-distillation technique to separate B from the calcium carbonate matrix (Gaillardet et al., 2001; Misra et al., 2014; Raitzsch et al., 2018; Wang et al., 2010). The distillate was diluted with 400 µL of 0.3 N HNO3. The B concentration of a small aliquot was determined using a quick (20 s) on-peak measurement of 11B on Faraday cup H9 using a Nu Plasma II MC-ICPMS (AWI, Bremerhaven). The remainder of the sample was then diluted to yield a solution with a [B] of 3 ppb and concentration-matched with the SRM NBS 951 to within ±3 %.
For isotope ratio measurements, boron was collected on Daly detectors, where high-mass D5 was used for 11B and D0 for 10B. Boron isotope data were measured in triplicate using the standard-sample-bracketing technique and reported in delta notation normalized to SRM NBS 951:
When 2σ of the mean derived from the triplicate was smaller than the long-term reproducibility (0.30 ‰), we report the latter as the measurement uncertainty. In addition, a small fragment of an in-house carbonate reference material WP22, used for our SIMS and LA-MC-ICPMS study, was cleaned and measured exactly the same way as the foraminifera sample to obtain a bulk δ11B value for comparison (16.60 ‰±0.30 ‰). This value is almost identical to that measured at IPGP using the bulk solution ICP-MS (16.76 ‰±0.11 ‰).
3.1 Intra-shell δ11B variability
The results from SIMS measurements conducted on five large specimens reveal a high δ11B variability ranging between 4.6 and 6.8 (mean 5.2) ‰ (2σ, 2 standard deviation of n individual measurements) within single shells, based on 8 to 19 single-spot analyses on each shell. A similar variability of 4.4 ‰ (2σ) on average is observed for measurements within single chambers (Fig. 4). Since it is difficult to distinguish between the very small (i.e. the juvenile) chambers in the central part, we allocated these measurements to the umbilical “knob”, which is also equivalent to the thick central part of the spiral side used for LA measurements. If δ11B is averaged for each chamber (one to three analyses per chamber), the mean variability between chambers is 4.2 ‰ (2σ) (Fig. 4). The two specimens measured chamber by chamber with LA also show variable δ11B, but with a much lower variation of ∼1.1 ‰ (2σ), compared to the SIMS data (Fig. 4). The average δ11B variability from all 16 shells measured multiple times is ∼1.3 ‰ (2σ).
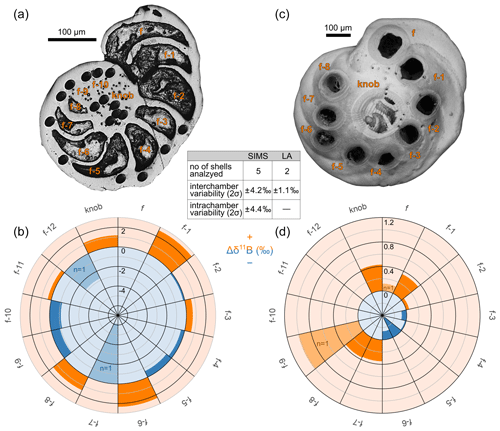
Figure 4Intra-shell variability of δ11B using SIMS (a) and LA-MC-ICPMS (b) on selected large individuals of C. wuellerstorfi. The residual Δδ11B (difference between single-spot and mean δ11B, Eq. 2) averaged from all analyzed specimens is shown for each chamber (f is the final chamber, f−1 the penultimate one, and so on). Orange color stands for higher-than-mean and blue for lower-than-mean values. Lighter colors indicate data that are based on only one measurement. The inset table summarizes the measured intra-shell variability derived from the two techniques.
Here the question arises of whether the difference in δ11B variability between the two methods is due to differences in analytical uncertainty or different scales of natural heterogeneity. If we consider an average uncertainty of ±0.9 ‰ for LA (Fig. 3), intra-shell variability is reduced from 1.3 ‰ to 0.4 ‰. As the 2σ measurement uncertainty for SIMS is roughly ±2.5 ‰, the remaining difference in variability between SIMS and LA methods of ∼2.3 ‰ is likely due to the different sampling volumes and hence related to heterogeneous boron isotopic distribution in the test. While the spot size for the SIMS method is ∼20 and ∼1 µm at depth, the laser-ablated volume ranges from 80 to 100 µm in diameter (Fig. 4) and approximately 10 µm at depth. Consequently, the ∼200 times larger volume analyzed by LA would reduce the δ11B variability detected by SIMS to . Hence we argue that the “true” δ11B heterogeneity is scale-dependent and assumedly on the order of ∼3 and ∼0.4 ‰ (2σ) on a ∼20 and 100 µm grid, respectively.
To examine potential systematic trends in δ11B among successive chambers, we calculated the residual boron isotopic composition Δδ11B for each site within each shell by comparing the B isotopic composition of a single-chamber with the mean value of the shell :
The SIMS data suggest that Δδ11B tends to decrease from the penultimate chamber (f−1) towards chamber f−5 by roughly 4 ‰ (Fig. 4), whereas no systematic change exists between chambers f−6 and the juvenile chambers. However, it is compelling that also the LA results suggest a decreasing trend in Δδ11B from the final chambers towards chamber f−5 by more than 0.5 ‰, while in the earlier chambers no systematic change can be observed (Fig. 4). For both methods, Wilcoxon–Mann–Whitney tests and Welch's t tests suggest that the Δδ11B change between the final chambers and f−5 is statistically insignificant at a 95 % significance level (p values ≥ 0.07). However, decreasing δ11B from the final chamber towards earlier chambers would be in line with the LA study by Sadekov et al. (2019) showing a ∼2 ‰ decrease along the last whorl of C. wuellerstorfi. A similar pattern was also observed for B∕Ca, with the highest value in the final chamber (Raitzsch et al., 2011; Sadekov et al., 2019), suggesting a strong biological influence or kinetic (i.e. growth rate) effect on boron incorporation. An in-depth discussion of biological and calcification processes is beyond the scope of this study, but the discovery of such high variability has implications for the use of δ11B-microanalytical techniques in paleoceanographic studies (e.g., Rollion-Bard and Erez, 2010).
Another notable feature derived from LA and SIMS is the elevated δ11B (by ∼0.5 ‰ on average) of the umbilical knob, compared to the whole-shell δ11B. This is confirmed by supplementary ablation of the knob of individuals, which were used for whole-shell analysis in Sect. 3.2. On average, umbilical knob δ11B was ∼0.4 ‰ higher than the value derived from the larger ablated area (see inset picture in Fig. 7), although this behavior is not systematic and was observed in only two thirds of the cases.
3.2 Inter-shell δ11B variability
Apart from the seven specimens used for inspecting the chamber-to-chamber variability, 16 individuals of C. wuellerstorfi were laser-ablated using a large area of at least 300 µm in diameter to cover a major part of the spiral side, and in 14 specimens subsequently analyzed for the composition of the thicker umbilical knob using a smaller crater (inset picture in Fig. 7). This way, we approached quasi-bulk δ11B values for single shells. Together with the δ11B medians from the two specimens described in the previous section, a total of 18 shells were used for determining the inter-shell δ11B variability using LA-MC-ICPMS (Fig. 5). It should be noted that we usually report the average as median, since it is less sensitive to outliers than the mean and also reflects the average of a non-uniform distribution. For SIMS analyses, the medians of single-spot analyses were calculated for each of the five shells.
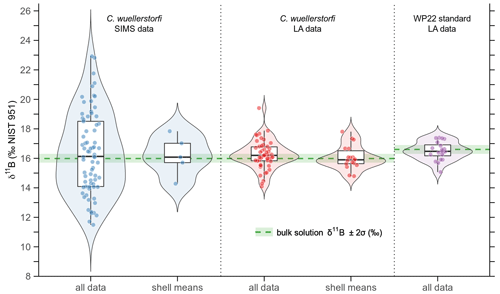
Figure 5Violin, box and jitter plots showing the distribution of all single-site δ11B values and single-shell means, both for the SIMS and laser ablation techniques. For comparison, the distribution of δ11B values measured on the in-house reference material WP22 is displayed as well. The green dashed lines and bars represent the bulk solution δ11B±2σ values.
The SIMS data reveal a huge spread of single-spot δ11B across the five specimens (Sect. 3.1), but the δ11B values averaged for each shell exhibit a narrower range between tests, with a median δ11B of 16.08 ‰±2.70 ‰ (2σ) (Fig. 5). In contrast, the single-site LA data across all 18 individuals show a smaller variation in δ11B than the SIMS data, where the values averaged for each shell yield a median of 15.90 ‰±1.62 ‰ (2σ). Both the average δ11B measurement uncertainty for LA of ±0.9 ‰ (2σ) and the variation difference between foraminiferal shells and WP22 of ∼0.4 ‰ suggest a residual inter-shell variability on the order of 0.4 ‰ to 0.7 ‰. Similarly, if an uncertainty of ±2.50 ‰ (2σ) for SIMS measurements is taken into account, the remaining inter-shell variability is only ∼0.2 ‰. Therefore, we estimate the “true” variability between shells of a population to be ∼0.4 ‰, which is the same as the variation estimated for the intra-shell variability (Sect. 3.1).
For shells where both large areas and knobs were measured (n=14), it is interesting to note that if only the large LA craters are considered, the mean δ11B is 15.87 ‰±1.78 ‰ (2σ), while it is 16.27 ‰±2.75 ‰ (2σ), if solely the small LA craters are taken into account (see Fig. 7, inset picture). As the volume of the large LA craters is ∼3 times larger than the smaller ones, the resulting variability among means of three resampled small-crater values is (2σ), which is quite close to the 1.78 ‰ derived from large craters, and confirms our conclusion that the δ11B variability is dependent on the scale at which it is measured.
3.3 Bulk solution δ11B
Both the SIMS and LA results reveal median values that match the bulk δ11B of 15.99 ‰±0.30 ‰ (2σ) measured in solution to within analytical uncertainties (Fig. 5). It should be noted again that the same specimens measured in solution had been measured before by LA, ensuring that we compare different techniques based on the same set of samples. Similarly, the average δ11B of 16.48 ‰±1.26 ‰ (2σ) in the reference material WP22 determined with LA-MC-ICPMS is not distinguishable from the bulk solution value of 16.60 ‰±0.30 ‰ (2σ), which confirms the robustness of the LA technique, and also the SIMS results, as the median foraminifera values are identical for LA and SIMS techniques.
The δ11B values obtained from all three methods fit in with the calibration data set for C. wuellerstorfi from the study by Rae et al. (2011) (Fig. 6) and confirm that the boron isotopic composition in this species closely matches that of borate of ambient seawater. Further, it proves that LA-MC-ICPMS and SIMS yield accurate results for δ11B, if the data set is large enough to overcome the issues of intra- and inter-shell variability (∼0.4 ‰), and analytical uncertainty of micro-analytical techniques ( ‰ and ±2.5 ‰ for LA and SIMS, respectively).
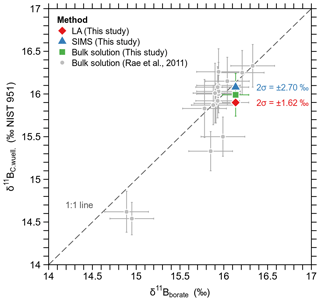
Figure 6Median δ11B of Holocene (5.6 ka) C. wuellerstorfi from GeoB core 1032 (Walvis Ridge, South Atlantic) measured with different techniques, shown along with the core-top calibration from (Rae et al., 2011). Note that the bulk solution analysis of this study was carried out on the same population measured before with laser ablation. Pooled δ11B uncertainties for SIMS (n=5 shells) and LA-MC-ICPMS (n=18 shells) are shown as numbers, as error bars exceed the y axis scale.
3.4 Implications for paleoreconstruction studies
The large intra- and inter-shell variations in δ11B described in Sects. 3.1 and 3.2 raise the question whether microanalytical techniques such as SIMS or LA-MC-ICPMS can be used for analyzing δ11B in C. wuellerstorfi to reconstruct past deep-water pH. The SIMS method requires careful embedding of foraminifer shells in epoxy and polishing down to a planar surface, which precludes further processing for bulk solution analyses, for example. However, because the size of the beam spot is small (20 µm or less), it is still possible to measure some other elemental and isotopic ratios at the same location on the sample; e.g., the same foraminifera specimens were used to measure δ18O (Rollion-Bard et al., 2008), δ11B (Rollion-Bard and Erez, 2010) and δ7Li (Vigier et al., 2015). The SIMS technique is very useful for biomineralization studies (e.g., Rollion-Bard and Erez, 2010), but for paleoreconstruction of deep-sea pH, where high precision is necessary, it may not be the most appropriate technique for routine downcore δ11B analysis. However, here we will inspect LA-MC-ICPMS as a potential tool for paleo-pH studies.
To attain information on the number of shells required for accurate LA analysis of δ11B to within a target uncertainty, we applied a Monte Carlo approach to generate two data sets with 10 000 δ11B data each, within a quoted uncertainty of ±1.68 ‰ and ±2.75 ‰ (2σ) for “large crater” and “knob” measurements, respectively, as given by the original data set (n=16 and n=14, resp.). The average δ11B values are considered identical between large craters and umbilical knobs, as in the original data they agree to within analytical uncertainty. Then we applied the “combn()” function of the R package “utils v3.4.4” (R Core Team, 2018) on each of the simulated data sets. With this function, we can calculate the uncertainty by generating all possible combinations of n shells taken from the simulated populations. For instance, if we would randomly pick five shells from this sediment sample, the analyzed δ11B would be accurate to within ±0.75 ‰ with a probability of 95 %, if large areas, and ±1.22 ‰, if only the knob areas were analyzed. If we targeted a standard uncertainty of ±0.50 ‰, which is equivalent to a pH uncertainty of roughly ±0.1, we would need to measure ∼12 specimens with LA, if large areas, and ∼14 specimens, if only the knob areas were analyzed (Fig. 7). The relationship between number of analyzed shells (n) and the estimated 2σ uncertainty is given by the quoted variability uq, i.e. the measured δ11B variation across a population (as 2σ), and n:
Given that the analysis uncertainty of the same amount measured in solution is about ±0.3 ‰, bulk solution analysis appears to be the more convenient technique for reconstructing paleo-pH. In contrast, the LA technique may be useful for generating high-resolution records, where sharp pH trends would partly compensate for the larger standard uncertainty or when only a few foraminifera specimens are available. Further, LA, like SIMS, has the potential to provide insight into ontogenetic δ11B variations, helping to better understand the biological uptake of boron during chamber formation.
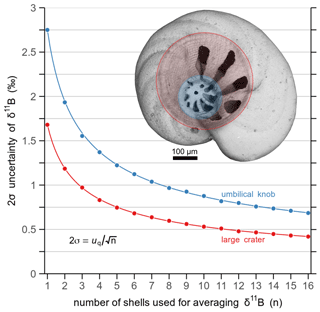
Figure 7Results from Monte Carlo simulations of 2σ uncertainty for δ11B using LA-MC-ICPMS in relation to the number of analyzed C. wuellerstorfi shells (n). In red is the estimated uncertainty based on “large crater”, and in blue on “umbilical knob” measurements (see inset scanning electron microscope picture for different areas). The estimated 2σ uncertainty can be described by a function of the quoted uncertainty (uq) and n (Eq. 3).
Microanalytical methods such as SIMS or LA-MC-ICPMS are potentially powerful tools for studying biomineralization processes or possible alternatives to conventional bulk solution analysis of δ11B in benthic foraminifera, if sample material is limited. For this study, we measured a population of 23 C. wuellerstorfi in total using SIMS and femtosecond LA-MC-ICPMS and compared the results with the bulk-solution δ11B, revealing consistent average values among the different techniques. While the medians agree to within ±0.1 ‰, a large intra-shell variability was observed, with up to 6.8 ‰ and 4.5 ‰ (2σ) derived from the SIMS and LA methods, respectively. We propose that the larger spread for SIMS, compared to LA, can be attributed to the much smaller volume () of calcite being analyzed in each run and hence supposedly reflects a larger heterogeneity of δ11B in the foraminiferal test on a smaller scale. When analytical uncertainties and scale-dependent differences in δ11B variations are taken into account, the intra-shell variability is likely on the order of ±0.4 ‰ and 3 ‰ (2σ) on a 100 and 20 µm scale, respectively.
The δ11B variability between shells exhibits total ranges of ∼3 ‰ for both techniques, suggesting that a number of shells needs to be analyzed for accurate mean δ11B values. We applied a simple resampling method and conclude that about 12 shells of C. wuellerstorfi must be analyzed using LA-MC-ICPMS to obtain an accurate average value to within ±0.5 ‰ (2σ). Hence, we suggest that, based on this high number of required individuals, the bulk solution MC-ICPMS method remains the first choice for analysis of δ11B in routine paleo-pH studies.
The boron isotope data collected for this study are available from Table S1 in the Supplement.
The supplement related to this article is available online at: https://doi.org/10.5194/bg-17-5365-2020-supplement.
MR, CRB, IH, and JB conceived the study. MR, CRB, and PL carried out measurements, analyzed the data, and performed data statistics. AB, KUR, and GS maintained and provided access to analytical instruments at AWI. JB, CRB, and IH raised funding for the French-German project “B2SeaCarb”. MR produced the figures for the paper. MR and CRB wrote the first draft of the paper, and all authors interpreted, edited, and reviewed the paper.
The authors declare that they have no conflict of interest.
This research was carried out in the framework of the joint French/German project “B2SeaCarb” and was supported by the Deutsche Forschungsgemeinschaft (DFG) grant number BI 432/10-1 to Jelle Bijma and DFG grant number HO 3257/5-1 to Ingo Horn. On the French side, the project was supported by the French National Research Agency (ANR) grant number ANR-16-CE92-0010 to Claire Rollion-Bard. Claire Rollion-Bard thanks Nordine Bouden (CRPG) for his technical help, and the MARUM GeoB core repository is acknowledged for providing sediment samples. Kaoru Kubota as well as the referees Dennis Mayk and Lubos Polerecky are thanked for their help in improving the paper.
This research has been supported by the Deutsche Forschungsgemeinschaft (grant nos. BI 432/10-1 and HO 3257/5-1) and the Agence Nationale de la Recherche (grant no. ANR-16-CE92-0010).
The article processing charges for this open-access publication were covered by the University of Bremen.
This paper was edited by Jack Middelburg and reviewed by Dennis Mayk and Lubos Polerecky.
Blamart, D., Rollion-Bard, C., Meibom, A., Cuif, J.-P., Juillet-Leclerc, A., and Dauphin, Y.: Correlation of boron isotopic composition with ultrastructure in the deep-sea coral Lophelia pertusa: Implications for biomineralization and paleo-pH, Geochem. Geophy. Geosy., 8, Q12001, https://doi.org/10.1029/2007GC001686, 2007.
Branson, O., Kaczmarek, K., Redfern, S. A. T., Misra, S., Langer, G., Tyliszczak, T., Bijma, J., and Elderfield, H.: The coordination and distribution of B in foraminiferal calcite, Earth Planet. Sc. Lett., 416, 67–72, https://doi.org/10.1016/j.epsl.2015.02.006, 2015.
Fietzke, J., Heinemann, A., Taubner, I., Böhm, F., Erez, J., and Eisenhauer, A.: Boron isotope ratio determination in carbonates via LA-MC-ICP-MS using soda-lime glass standards as reference material, J. Anal. At. Spectrom., 25, 1953, https://doi.org/10.1039/c0ja00036a, 2010.
Fietzke, J., Ragazzola, F., Halfar, J., Dietze, H., Foster, L. C., Hansteen, T. H., Eisenhauer, A., and Steneck, R. S.: Century-scale trends and seasonality in pH and temperature for shallow zones of the Bering Sea, P. Natl. Acad. Sci. USA, 112, 2960–2965, https://doi.org/10.1073/pnas.1419216112, 2015.
Gaillardet, J., Lemarchand, D., Göpel, C., and Manhès, G.: Evaporation and Sublimation of Boric Acid: Application for Boron Purification from Organic Rich Solutions, Geostand. Newsl., 25, 67–75, https://doi.org/10.1111/j.1751-908X.2001.tb00788.x, 2001.
Hemming, N. G. and Hanson, G. N.: Boron isotopic composition and concentration in modern marine carbonates, Geochim. Cosmochim. Ac., 56, 537–543, https://doi.org/10.1016/0016-7037(92)90151-8, 1992.
Hönisch, B., Bickert, T., and Hemming, N. G.: Modern and Pleistocene boron isotope composition of the benthic foraminifer Cibicidoides wuellerstorfi, Earth Planet. Sci. Lett., 272, 309–318, https://doi.org/10.1016/j.epsl.2008.04.047, 2008.
Horn, I. and von Blanckenburg, F.: Investigation on elemental and isotopic fractionation during 196 nm femtosecond laser ablation multiple collector inductively coupled plasma mass spectrometry, Spectrochim. Acta B-At. Spectrosc., 62, 410–422, https://doi.org/10.1016/j.sab.2007.03.034, 2007.
Howes, E. L., Kaczmarek, K., Raitzsch, M., Mewes, A., Bijma, N., Horn, I., Misra, S., Gattuso, J.-P., and Bijma, J.: Decoupled carbonate chemistry controls on the incorporation of boron into Orbulina universa, Biogeosciences, 14, 415–430, https://doi.org/10.5194/bg-14-415-2017, 2017.
Inoue, M., Nohara, M., Okai, T., Suzuki, A., and Kawahata, H.: Concentrations of Trace Elements in Carbonate Reference Materials Coral JCp-1 and Giant Clam JCt-1 by Inductively Plasma-Mass Spectrometry, Geostand. Geoanal. Res., 28, 411–416, 2004.
Kaczmarek, K., Horn, I., Nehrke, G., and Bijma, J.: Simultaneous determination of δ11B and B ∕ Ca ratio in marine biogenic carbonates at nanogram level, Chem. Geol., 392, 32–42, https://doi.org/10.1016/j.chemgeo.2014.11.011, 2015a.
Kaczmarek, K., Langer, G., Nehrke, G., Horn, I., Misra, S., Janse, M., and Bijma, J.: Boron incorporation in the foraminifer Amphistegina lessonii under a decoupled carbonate chemistry, Biogeosciences, 12, 1753–1763, https://doi.org/10.5194/bg-12-1753-2015, 2015b.
Kasemann, S. A., Schmidt, D. N., Bijma, J., and Foster, G. L.: In situ boron isotope analysis in marine carbonates and its application for foraminifera and palaeo-pH, Chem. Geol., 260, 138–147, https://doi.org/10.1016/j.chemgeo.2008.12.015, 2009.
Klochko, K., Kaufman, A. J., Yao, W., Byrne, R. H., and Tossell, J. A.: Experimental measurement of boron isotope fractionation in seawater, Earth Planet. Sci. Lett., 248, 276–285, https://doi.org/10.1016/j.epsl.2006.05.034, 2006.
Louvat, P., Moureau, J., Paris, G., Bouchez, J., Noireaux, J., and Gaillardet, J.: A fully automated direct injection nebulizer (d-DIHEN) for MC-ICP-MS isotope analysis: application to boron isotope ratio measurements, J. Anal. At. Spectrom., 29, 1698–1707, https://doi.org/10.1039/C4JA00098F, 2014.
Malherbe, J., Penen, F., Isaure, M.-P., Frank, J., Hause, G., Dobritzsch, D., Gontier, E., Horréard, F., Hillion, F., and Schaumlöffel, D.: A New Radio Frequency Plasma Oxygen Primary Ion Source on Nano Secondary Ion Mass Spectrometry for Improved Lateral Resolution and Detection of Electropositive Elements at Single Cell Level, Anal. Chem., 88, 7130–7136, https://doi.org/10.1021/acs.analchem.6b01153, 2016.
Mayk, D., Fietzke, J., Anagnostou, E., and Paytan, A.: LA-MC-ICP-MS study of boron isotopes in individual planktonic foraminifera: A novel approach to obtain seasonal variability patterns, Chem. Geol., 531, 119351, https://doi.org/10.1016/j.chemgeo.2019.119351, 2020.
Misra, S., Owen, R., Kerr, J., Greaves, M., and Elderfield, H.: Determination of δ11B by HR-ICP-MS from mass limited samples: Application to natural carbonates and water samples, Geochim. Cosmochim. Ac., 140, 531–552, https://doi.org/10.1016/j.gca.2014.05.047, 2014.
Okai, T., Suzuki, A., Terashima, S., Inoue, M., Nohara, M., Kawahata, H., and Imai, N.: Collaborative Analysis of GSJ/AIST Geochemical Reference Materials JCp-1 (Coral) and JCt-1 (Giant Clam), Chikyu Kagaku, 38, 281–286, 2004.
Rae, J. W. B., Foster, G. L., Schmidt, D. N., and Elliott, T.: Boron isotopes and B ∕ Ca in benthic foraminifera: Proxies for the deep ocean carbonate system, Earth Planet. Sc. Lett., 302, 403–413, https://doi.org/10.1016/j.epsl.2010.12.034, 2011.
Raitzsch, M. and Hönisch, B.: Cenozoic boron isotope variations in benthic foraminifers, Geology, 41, 591–594, https://doi.org/10.1130/G34031.1, 2013.
Raitzsch, M., Hathorne, E. C., Kuhnert, H., Groeneveld, J., and Bickert, T.: Modern and late Pleistocene B ∕ Ca ratios of the benthic foraminifer Planulina wuellerstorfi determined with laser ablation ICP-MS, Geology, 39, 1039–1042, https://doi.org/10.1130/G32009.1, 2011.
Raitzsch, M., Bijma, J., Benthien, A., Richter, K.-U., Steinhoefel, G., and Kučera, M.: Boron isotope-based seasonal paleo-pH reconstruction for the Southeast Atlantic – A multispecies approach using habitat preference of planktonic foraminifera, Earth Planet. Sc. Lett., 487, 138–150, https://doi.org/10.1016/j.epsl.2018.02.002, 2018.
Raitzsch, M., Bijma, J., Bickert, T., Schulz, M., Holbourn, A., and Kučera, M.: Eccentricity-paced atmospheric carbon-dioxide variations across the middle Miocene climate transition, Clim. Past Discuss., https://doi.org/10.5194/cp-2020-96, in review, 2020.
R Core Team: A Language and Environment for Statistical Computing, R Foundation for Statistical Computing, Vienna, available at: https://www.R-project.org (last access: 29 February 2020), 2018.
Rollion-Bard, C. and Blamart, D.: In: Biomineralization Sourcebook: Characterization of Biominerals and Biomimetic Materials, pp. 249–261, CRC Press, Taylor & Francis Group, Boca Raton, FL, 2014.
Rollion-Bard, C., Erez, J., and Zilberman, T.: Intra-shell oxygen isotope ratios in the benthic foraminifera genus Amphistegina and the influence of seawater carbonate chemistry and temperature on this ratio, Geochim. Cosmochim. Ac., 72, 6006–6014, https://doi.org/10.1016/j.gca.2008.09.013, 2008.
Rollion-Bard, C. and Erez, J.: Intra-shell boron isotope ratios in the symbiont-bearing benthic foraminiferan Amphistegina lobifera: Implications for δ11B vital effects and paleo-pH reconstructions, Geochim. Cosmochim. Ac., 74, 1530–1536, https://doi.org/10.1016/j.gca.2009.11.017, 2010.
Rollion-Bard, C., Chaussidon, M., and France-Lanord, C.: pH control on oxygen isotopic composition of symbiotic corals, Earth Planet. Sc. Lett., 215, 275–288, https://doi.org/10.1016/S0012-821X(03)00391-1, 2003.
Sadekov, A., Lloyd, N. S., Misra, S., Trotter, J., D'Olivo, J., and McCulloch, M.: Accurate and precise microscale measurements of boron isotope ratios in calcium carbonates using laser ablation multicollector-ICPMS, J. Anal. At. Spectrom., 34, 550–560, https://doi.org/10.1039/C8JA00444G, 2019.
Standish, C. D., Chalk, T. B., Babila, T. L., Milton, J. A., Palmer, M. R. and Foster, G. L.: The effect of matrix interferences on in situ boron isotope analysis by laser ablation multi-collector inductively coupled plasma mass spectrometry, Rapid Commun. Mass Sp., 33, 959–968, https://doi.org/10.1002/rcm.8432, 2019.
Steinhoefel, G., Horn, I., and von Blanckenburg, F.: Matrix-independent Fe isotope ratio determination in silicates using UV femtosecond laser ablation, Chem. Geol., 268, 67–73, https://doi.org/10.1016/j.chemgeo.2009.07.010, 2009.
Thil, F., Blamart, D., Assailly, C., Lazareth, C. E., Leblanc, T., Butsher, J., and Douville, E.: Development of laser ablation multi-collector inductively coupled plasma mass spectrometry for boron isotopic measurement in marine biocarbonates: new improvements and application to a modern Porites coral, Rapid Commun. Mass Sp., 30, 359–371, https://doi.org/10.1002/rcm.7448, 2016.
Vigier, N., Rollion-Bard, C., Levenson, Y., and Erez, J.: Lithium isotopes in foraminifera shells as a novel proxy for the ocean dissolved inorganic carbon (DIC), C.R. Geosci., 347, 43–51, https://doi.org/10.1016/j.crte.2014.12.001, 2015.
Wang, B.-S., You, C.-F., Huang, K.-F., Wu, S.-F., Aggarwal, S. K., Chung, C.-H., and Lin, P.-Y.: Direct separation of boron from Na- and Ca-rich matrices by sublimation for stable isotope measurement by MC-ICP-MS, Talanta, 82, 1378–1384, https://doi.org/10.1016/j.talanta.2010.07.010, 2010.
Yu, J. and Elderfield, H.: Benthic foraminiferal B ∕ Ca ratios reflect deep water carbonate saturation state, Earth Planet. Sc. Lett., 258, 73–86, https://doi.org/10.1016/j.epsl.2007.03.025, 2007.
Yu, J., Foster, G. L., Elderfield, H., Broecker, W. S., and Clark, E.: An evaluation of benthic foraminiferal B ∕ Ca and δ11B for deep ocean carbonate ion and pH reconstructions, Earth Planet. Sc. Lett., 293, 114–120, https://doi.org/10.1016/j.epsl.2010.02.029, 2010.