the Creative Commons Attribution 4.0 License.
the Creative Commons Attribution 4.0 License.
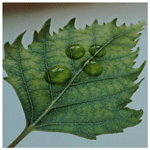
Surfaces of silver birch (Betula pendula) are sources of biological ice nuclei: in vivo and in situ investigations
Teresa M. Seifried
Paul Bieber
Laura Felgitsch
Julian Vlasich
Florian Reyzek
David G. Schmale III
Hinrich Grothe
Silver birch (Betula pendula) is known to contain ice-nucleating macromolecules (INMs) to survive in harsh environments. However, little is known about the release and transport of INMs from birch trees into the atmosphere. In this study, we conducted in situ and in vivo investigations on INMs from nine birches growing in an alpine valley (Ötztal, Austria). A detailed analysis of drill cores showed that INM concentration increases towards outer layers, reaching its maximum near the surface. Aqueous extracts from the surfaces of leaves, bark, primary wood and secondary wood contained INMs (34∕36) with concentrations ranging from 9.9×105 to 1.8×109 INMs cm−2. In a field study, we analysed the effect of precipitation on the release of these INMs attached to the surface of the trees. These experiments showed that INMs are splashed and aerosolized into the environment during rainfall events, at concentrations and freezing temperatures similar to in vivo samples. Our work sheds new light on the release and transport of INMs from birch surfaces into the troposphere. Birches growing in boreal and alpine forests should be considered an important terrestrial source of INMs.
- Article
(4950 KB) - Full-text XML
-
Supplement
(91 KB) - BibTeX
- EndNote
If temperatures at ambient pressure fall below 0 ∘C, ice is the thermodynamically favourable state of water (Cantrell and Heymsfield, 2005; Hegg and Baker, 2009; Murray et al., 2010). For the phase transition from liquid to solid state, water molecules need to arrange in an ice-like structure. Depending on the temperature, the formed ice embryo needs to overcome a critical cluster size to initiate freezing of the bulk, which typically takes place well below 0 ∘C (Cantrell and Heymsfield, 2005; Turnbull and Fisher, 1949). Homogeneous freezing temperatures for small droplets as present in clouds are typically below −35 ∘C (Pruppbacher and Klett, 1997). A broad spectrum of substances has been found to catalyse freezing at higher temperatures, in a process called heterogeneous ice nucleation (Dorsey, 1948; Murray et al., 2012). Particles that trigger higher freezing temperatures are referred to as ice-nucleating particles (INPs) (Vali et al., 2015). Among these are mineral dusts (Broadley et al., 2012; Zolles et al., 2015), soot (DeMott, 1990; Gorbunov et al., 2001) and biogenic particles (Pöschl et al., 2010), and when airborne they have the potential to affect cloud glaciation and consequently weather and climate (Lohmann, 2002; Mishchenko et al., 1996; Forster et al., 2007; Baker, 1997). The land surface is a major contributor to global aerosols (Fröhlich-Nowoisky et al., 2016; Pöschl, 2005; Després et al., 2012; Jaenicke, 2005). Atmospheric concentrations of primary biological aerosol particles (PBAPs) are highly dependent on sampling sites and meteorological factors (Jones and Harrison, 2004). PBAPs include a wide variety of substances with varying sizes from millimetres down to nanometres (e.g. fragments of insects, scurf from plants, pollen, fungal spores, bacteria and viruses), with cellular material carrying proteins being an important part of it (Jaenicke, 2005). However, our knowledge on the distribution and impact of PBAPs in the atmosphere is still rather limited.
Heterogeneous ice nucleation of biological organisms has been reported in plants (Diehl et al., 2001; Pummer et al., 2012), bacteria (Lindow et al., 1982; Wolber et al., 1986), fungi (Pouleur et al., 1992; Fröhlich-Nowoisky et al., 2015; Kunert et al., 2019; Iannone et al., 2011; Haga et al., 2013), moss spores (Weber, 2016) and lichen (Kieft, 1988). Plants growing in temperate environments use mechanisms such as extracellular freezing (Burke et al., 1976; Storey and Storey, 2005; Pearce, 2001) or extra-organ freezing (Quamme, 1978; Ishikawa and Sakai, 1981) to survive sub-zero temperatures. Consequently, ice nucleation is an important means of regulating freezing in plants. Extracellular freezing in frost-tolerant woody plants occurs mostly in bark tissues (Burke et al., 1976; Ashworth et al., 1988; Ashworth, 1996). INPs trigger ice crystal formation in extracellular spaces that allow the withdrawal of water from the cell. This leads to an increase in the supercooling capacity of the cell, preventing it from damage (Ishikawa and Sakai, 1981). Extra-organ freezing is common in leaf buds (Ishikawa et al., 2015), where bud scales serve as ice sinks, removing water from the flower primordia. The resulting supercooling of flower primordia prevents the tissues from freezing (Ishikawa et al., 2015).
Many frost-hardy plants contain INPs. Leaves of winter rye (Secale cereal) embody INPs active between −7 and −8 ∘C (Brush et al., 1994). The stems of high-bush blueberries (Vaccinium corymbosum) (Kishimoto et al., 2014b), wood of Prunus (Gross et al., 1988) and a variety of berries from perennial plants (Felgitsch et al., 2019) are also known to contain INPs, whereby the former show the highest INP concentrations in autumn, just before frost events occur (Kishimoto et al., 2014a). Additionally, decayed leaf litter was found to be a potent source for INPs (Conen et al., 2016, 2017; Schnell and Vali, 1973), some of which may be stable for almost 50 years (Vasebi et al., 2019). Conen et al. (2017) found that the emission of these INPs (active at temperatures above −8 ∘C) increases in autumn, and it is hypothesized that the vegetation could contribute to local climate. Moreover, INPs are found to be present in various pollen from different trees (e.g. birch, pine and juniper) (Pummer et al., 2012; Diehl et al., 2001). Past studies showed that INPs from biological systems are rather in a macromolecular size range (ice-nucleating macromolecules, INMs) (Pummer et al., 2012; Kunert et al., 2019). If those INMs can be easily washed off a plant's surface, they would have the potential to become aerosolized and serve as an important source for INPs in the atmosphere.
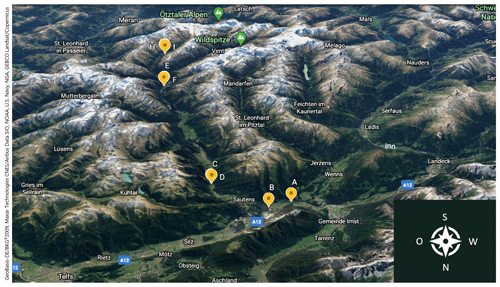
Figure 1Locations of the sampled birches (yellow labels: Tree A to I) along the Ötztal valley in Tyrol, Austria. Picture adapted from Google Earth ©, https://earth.google.com/, last access: 10 February 2020.
Heavy rain events and thunderstorms have been associated with high INP concentrations (Bigg and Miles, 1964; Isono and Tanaka, 1966). More recently, studies highlight that rainfall triggers the release of PBAPs into the atmosphere (Huffman et al., 2013; Rathnayake et al., 2017; Prenni et al., 2013; Hara et al., 2016; Tobo et al., 2013). Huffman and colleagues showed that the burst in PBAPs correlates with fluorescence activity and high INP concentrations (Huffman et al., 2013). During rainfall, splash-induced emissions of fungal spores can occur (Hirst, 1953; Allitt, 2000; Kim et al., 2019). Rain droplets impacting on leaf surfaces hosting ice-nucleation active microbes (Morris et al., 2004) or soluble INMs (Pummer et al., 2015) may produce aerosols containing INPs. High relative humidity may also result in an increase in INPs (Wright et al., 2014), priming fungi to eject spores (Jones and Harrison, 2004) and encouraging pollen grains to germinate or rupture due to enhanced osmotic pressure (Taylor et al., 2004). Both processes release particles with aerodynamic diameters smaller than 2 µm (Grote et al., 2003), with the ability to be transported into the atmosphere by wind (Pöschl, 2005). Pollen grains of birch are known to trigger ice nucleation at around −18 ∘C (Pummer et al., 2012; Diehl et al., 2001). Pummer et al. (2012) were able to show that these ice nuclei are in the macromolecular size range since INMs could pass through a 200 nm cut-off filter, whereas the pollen grains are retained (Pummer et al., 2012, 2015). The INMs are easily detached from the pollen and are released in the highest concentrations to the aqueous phase. INMs are not only present in the pollen grains of birches, but are spread throughout different parts of the tree, including leaves and branch wood (Felgitsch et al., 2018). However, only extracts of pulverized plant material were analysed so far (Felgitsch et al., 2018), and the availability of INMs from birches to the surrounding environment remains unclear. In this paper, we extend the work of Felgitsch et al. (2018) by analysing the distribution of INMs throughout different birch trees, with a focus on intact surfaces of silver birches as sources of biological ice nuclei. We hypothesize that INMs are located on the surface of birches and are transported into the troposphere. The specific objectives of this work were to study the distribution and concentrations of INMs in birch tissues and investigate the transport of INMs from birch trees into the environment. Drill cores from three selected trees were characterized for their ice nucleation activity. INMs were extracted from leaves, branch wood (primary and secondary wood) and bark. A field study was conducted using four birches at different locations to analyse the effect of precipitation on the release of INMs from the surface of the trees. Collectively, our work sheds new light on the release and transport of INMs from the terrestrial biosphere into the atmosphere.
2.1 Sample collection
Birch trees were selected in an alpine valley (Ötztal) in the western part of Austria (GPS: 48.756098, 15.891850) (Felgitsch et al., 2018). The valley climbs from 799 m a.s.l., where the environment is dominated by fields and forests, to 3400 m, where the valley ends in a glacier area. This area is remote and sparsely populated; anthropogenic aerosol emissions (e.g. traffic and industrial emission as well as biomass burning) are limited. We collected samples from nine different mature birches growing throughout the valley along an altitudinal gradient from 799 to 1925 m (timberline). The higher the altitude the more the environment is comparable to northern latitudes (boreal forests), where trees need to cope with cold winters. We numbered the sampled trees alphabetically (Tree A to I). Tree A and B are growing at the foot of the valley in a forest. Tree C, D, E, F, H and I are located next to a mountain river (riparian forest). Tree G is not surrounded by other trees and is located at the highest point, growing directly at the timberline (1925 m). GPS way points, altitudes and further information on the sampled trees are given in Table 1. Figure 1 shows the valley and locations of the sampled trees (map adapted from Google Earth ©).
Table 1Information for the nine sampled birches in Tyrol, Austria. Cores and samples for surface extraction experiments were taken in summer 2016. Rain samples were collected in October 2019 underneath Tree E, F, G and H.
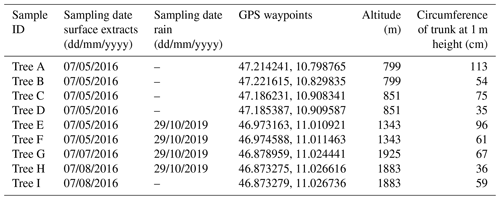
We took bark, branches (primary and secondary wood), leaves as well as drill core samples in summer 2016 (see Fig. 2). Ice nucleation activity of powder extracts from leaf and branch wood samples has been reported previously (Felgitsch et al., 2018). However, in this study we focused on the investigation of intact tissues. Primary wood is newly grown and still photosynthetically active. In contrast, secondary wood is older, appears brown and is not photosynthetically active anymore. We took approx. 50 mm sections of these branch wood samples, originating from the same branch per tree. From the trunk, we collected bark samples using tweezers and drill cores at 1 m height, which were obtained with an increment borer (5 mm diameter, Haglöf, Sweden). The drill cores consist of the bark (outermost layer), the bast and the inner wood (see Fig. 2e). A microscopic picture of each segment is given in Fig. 2f, g and h on the bottom. All tools used during sampling were disinfected with ethanol (approx. 90 vol %) after each usage. Samples were frozen within a few hours of sampling at −20 ∘C.
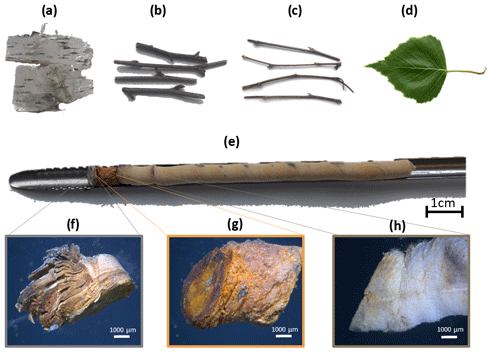
Figure 2Type of samples collected. (a) Bark, (b) secondary wood, (c) primary wood, (d) leaves and (e) drill core. Microscopic pictures of (f) bark, (g) bast and (h) inner wood from the drill core (Tree C).
In October 2019, a series of precipitation collectors were placed underneath the birches Tree E, F, G and H. These rain collectors consisted of sterile centrifuge tubes (polypropylene, 50 mL, Brand®, Germany) mounted on wooden sticks anchored to the ground with three guy lines (Fig. 3). Three to four collectors were placed under each tree and cardinal positions (north, N; east, E; south, S; west, W) were noted. All collectors were placed directly under the crown of the tree. One collector was set up next to each tree in an open terrain (>5 m away from the tree) collecting pure rainwater to serve as a control.
2.2 Sample preparation
The drill cores of Tree A, C and I were separated in bark, bast and approx. three 5 mm large sections of inner wood (see Fig. 2). To determine the surface availability of each part of the drill core, we approximated the segments as cylinders. Each segment was extracted with ultra-pure water for 6 h. Since we were interested in substances in the macromolecular size range, the coarse fraction of the extracts was removed, and the remaining aqueous phase was then centrifuged at 3500 rpm (1123 rcf, relative centrifugal force) for 10 min and filtered with a 0.2 µm syringe filter (cellulose acetate membrane, sterile, VWR, USA). The surface area of each drill core segment per volume of water used for the extraction is summarized in Table 2.
Table 2Surface area per millilitre of extracted water (ultra-pure) for drill core samples from Tree A, C and I. Bark and bast segments are the outermost layers, followed by three layers of the inner wood (1–3).
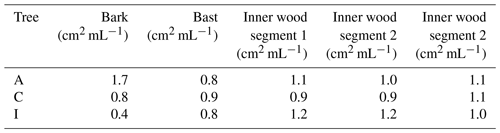
The branch wood samples were cut in approx. 10 mm sections, sealing the edges with paraffin wax (Sigma Aldrich, USA) to avoid leakage of components through the cut surface and extracting the samples with ultra-pure water (between 0.6 and 3 mL) for 6 h. The wax was tested for ice nucleation activity prior sample treatment and was found to be inactive. In the case of the leaves we used whole leaves, again covering the petiole with paraffin wax and extracting the samples in 5–10 mL water per sample for 6 h. The extraction volume varies due to the varying sizes of the samples. The bark was analysed using a 5 mm punch and extracting three punched pieces of bark per sample in 1 mL ultrapure water for 6 h. In line with drill core samples, the particular solid wood or leaf sample was removed, and the remaining sample solution was centrifuged (1123 rcf) and filtered (0.2 µm syringe filter). In order to calculate the extracted samples' surfaces, samples were roughly estimated as geometric figures: branch wood as cylinders, leaves as convex deltoids and bark punches as circles. The detailed calculations are described in Sect. 2.4. The surfaces per extracted millilitre are given in Table 3.
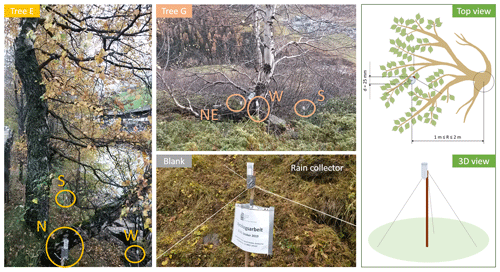
Figure 3Rain sampling setup underneath Tree E (left, yellow) and Tree G (top middle, light orange). Three to four rainwater collectors were placed underneath each of the sampled trees. Next to the trees of interest, we mounted a control collector (blank, centre middle grey), which was placed in an open terrain. Top view as well as a schematic 3D picture of the rain collector (right, green).
Rain samples were stored in a −20 ∘C freezer. The volume of each rain sample was determined after the sample was defrosted, immediately prior to analysis. One millilitre aliquots were taken from these thawed rain samples, filtrated with a 0.2 µm syringe filter, as described for the wood and leaf samples, and measured for ice nucleation activity.
2.3 Cryo-microscopy
All freezing experiments (immersion freezing mode) were performed using the cryo-microscopy setup VODCA (Vienna Optical Droplet Crystallization Analyser). A detailed description of the setup is given in Felgitsch et al. (2018). In short, the setup consists of two main components: an incident light microscope (BX51M, Olympus, Japan) with an attached camera (MDC320, Hengtech, Germany) linked to a computer and a cryo-cell. The cryo-cell is a polymer-based compartment that can be closed airtight. It contains a cooling unit consisting of a Peltier element (Quick-Cool QC-31-1.4-3.7M) with a thermocouple fixed on top and a heat exchanger, cooling the warm side of the Peltier element during freezing experiments. The samples are all measured as aqueous components of an emulsion created on a clean glass slide which is placed on top of the Peltier element during the cooling process. A LabVIEW-based software enables us to record videos during the freezing process. Prior to sample measurements, a reference sample (ultra-pure water produced with Millipore® SAS SIMSV001, Merck Millipore, USA) was analysed (see Fig. S8 in the Supplement). All freezing experiments were performed with a cooling rate of 10 ∘C min−1. Only droplets in the size range between 15 and 40 µm (droplet volume: 1.8–34 pL) were included in our evaluation of the freezing experiments. To observe at least one heterogeneous freezing event within 100 droplets (total amount of droplets in one measurement), the lower detection limit of VODCA is about 103 INMs µL−1 (105 INMs cm−2).
2.4 Data analysis
Considering ice nucleation to be a time-independent process, the number of INMs or INPs active above a certain temperature can be expressed by the cumulative nucleus spectrum (K(T)) (Murray et al., 2012; Vali, 1971) as stated in Eq. (1).
D is the dilution factor, Vdroplet is the analysed droplet volume (8.2 pL using VODCA) and fice(T) is the fraction of frozen droplets at a given temperature, whereas nfrozen is the number droplets which froze at a regarded temperature, and ntotal is the total number of droplets frozen during an experiment.
To refer the number of INMs extractable from the surface of the respective sample, we modified K(T) by multiplying with the extraction volume, Vextraction, divided by the surface of the sample, σsample (Eq. 2).
To estimate the area of the sample surfaces, we used approximations as indicated in Sect. 2.2. Drill core segments as well as primary and secondary wood samples were estimated as cylinders. We used the whole surface area for the core samples and the surface area without the base for the secondary and primary wood samples (since the top and bottom base were covered with paraffin wax). Leaves were approximated with the area of two triangles to best capture the kite-like shape (convex deltoid). In our surface estimations, we accounted the dorsal and ventral side for the area of the leaf. For the bark samples, we used two punches with a diameter of 5 mm; therefore, the surface was approximated as the circular area multiplied by two to capture the top and bottom of the punch. We did not account for surface roughness. Surface sites are given in Tables 2 and 3.
To calculate the INM concentrations extracted from the birches during rainfall events, K(T) from Eq. (1) was modified by multiplying with the rain volume, Vrain, divided by the area of the precipitation collectors' inlet, σinlet (circular) (Eq. 3).
Calculating K(−34 ∘C) and K(−25 ∘C) depending on the sampled rain volume per surface (cross-section area of sample collector) made it possible to compare the data of rain samples with samples extracted in the laboratory. In immersion freezing experiments, only INMs with the highest activity present in a droplet can be observed, and INMs in the same droplet with lower activity are not captured. Thus, INMs active at lower temperatures are easily underrepresented in samples with high concentrations of INMs. To avoid underestimation, a sample was diluted and remeasured when it froze purely heterogeneously. Thus, the full range of present INMs is captured within the experiments.
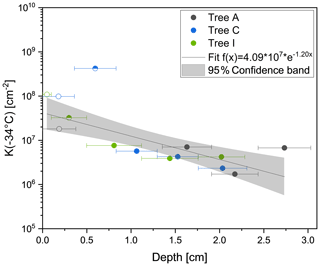
Figure 4Cumulative nucleus concentration at −34 ∘C (K(−34 ∘C)) of the analysed core samples of Tree A (grey), Tree C (blue) and Tree I (green). Bark samples are marked with a hollow symbol, and bast samples are marked with a half-filled symbol. Filled symbols correspond to inner wood samples. To assign the depth of the different core segments, the centre of the segments was used. The sizes of the segments are visualized with corresponding horizontal lines. INM concentration increases towards outer layers of the trunk. The bast sample from Tree A does not freeze heterogeneously.
3.1 Drill cores (in vivo)
The results of drill cores show that K(−34 ∘C) values are highest at the outermost parts of the tree, meaning the bark and the bast (Fig. 4). K(−34 ∘C) gives the number of INMs active at temperatures higher than −34 ∘C, attributed purely to heterogeneous freezing events (calculated by Eq. 2). The bark values varied between 1.8×107 cm−2 (Tree A) and 9.8×107 cm−2 (Tree C). Yet, the bast sample from Tree A did not freeze heterogeneously at all, likely due to a concentration of INMs under the limit of detection for the freezing assay. However, the Tree C bast sample showed the highest K(−34 ∘C) value (4.2×108 cm−2) of all analysed core samples. The inner wood segments of all three drill cores exhibited significantly lower INM concentrations compared to the rest, ranging between 1.7×106 cm−2 (Tree A, inner wood segment 2) and 6.7×107 cm−2 (Tree A, inner wood segment 3). To model the concentration gradient of INMs throughout the drill cores, the data were fitted with an exponential function (, where y represents K(−34 ∘C) (cm−2) and x the depth (cm)). We see that the INM concentration gradient within the trunk of the tree increases towards outer layers of the trunk, reaching the maximum near the surface.
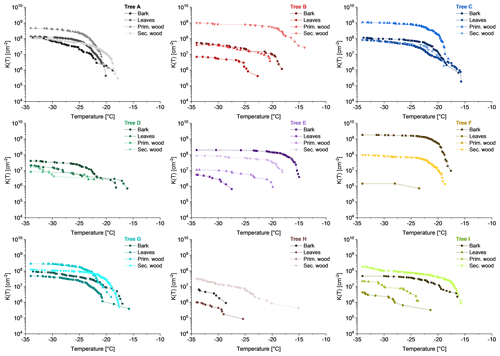
Figure 5Cumulative nucleus spectra K(T) of aqueous extracted bark, branch wood and leaf surfaces. Samples originate from nine different birches (named Tree A to I) in Tyrol, Austria. Rectangles show bark samples, dots represent leaves, triangles with the cone end up illustrate primary wood and triangles with the cone end down secondary wood. Nearly all samples freeze heterogeneously. Two samples are not active, both of which are primary wood (Tree F and H).
3.2 Barks, leaves and branch woods (in vivo)
Nearly all analysed surface extracts of bark, leaf and branch wood samples released INMs with K(−25 ∘C) and K(−34 ∘C) values in the order of magnitude between 105 and 109 cm−2. Figure 5 provides the cumulative nucleus spectrum as a function of temperature (K(T)), providing information on the course of heterogeneous freezing including the onset freezing temperatures (Ton). In general, we observed rather high concentrations for bark and branch wood samples compared to leaves. Two of all analysed samples had K(−34 ∘C) values below the limit of detection, both of which were primary wood, one from Tree F and another one from Tree H. Within the samples from the same tree K(−25 ∘C) as well as K(−34 ∘C) values are considerably similar from Tree A, D and G. Comparing K(−25 ∘C) to K(−34 ∘C) values from all leaf samples, the concentrations increase towards colder temperatures. Further, Ton values across all heterogeneously nucleated samples ranged from −14.0 ∘C (Tree B, secondary wood) to −28.6 ∘C (Tree H, bark). Bark, leaves, primary and secondary wood samples from Tree A, C, D and G showed similar Ton values. However, Ton varies strongly across samples from Tree B, E, F, H and I. Considering the whole data set, the type of sample that started to freeze at the highest temperature varied across the trees. Focusing on all leaf samples, a strong variation of Ton is observed ranging from −15.7 (Tree C) to −27.5 ∘C (Tree E). In contrast, secondary woods showed low variations not only for Ton but also for K(−34 ∘C) values. In addition, secondary wood tended to exhibit highest Ton values.
3.3 Rainfall event (in situ)
Ice nucleation data of rain collected underneath the birches reveal heterogeneous freezing in all samples (Fig. 6), whereas control samples (pure rainwater, collectors were set up next to the trees in an open terrain) did not show heterogeneous freezing at all. K(−25 ∘C) and K(−34 ∘C) values, calculated using Eq. (3), varied in the orders of magnitude from 105 to 109 and from 106 to 109 per square centimetre (cm−2) area of rain. Within the samples from the same tree the cumulative nucleus spectra are considerably similar. However, the concentration of sample W (west) from Tree G is approximately 2 orders of magnitude below the other two samples (S, south; NE, north-east). Furthermore, Ton values within the samples from each tree were comparably similar. Nonetheless, Ton of sample W from Tree G is an outlier. Moreover, two trees, namely Tree E and F, were covered with leaves (most of which were yellow), whereas Tree G and H were leafless. On the one hand, all samples from leaf-covered trees exhibit similar K(−25 ∘C) and K(−34 ∘C) values (higher than 107 INM cm−2). On the other hand, samples from trees without leaves show a higher variation. Furthermore, the cumulative nucleus spectra indicate steep increases at the beginning of the curves at temperatures between −16.0 and −23.0 ∘C. Ton of rain samples varied between −15.6 and −23.8 ∘C, which is rather similar to in vivo samples.
The influence of PBAPs acting as INPs in atmospheric processes as well as the transport of these particles from the terrestrial surface to the atmosphere remains elusive. It is well known that many plants growing in boreal and alpine forests contain INPs or INMs to survive in extreme conditions (Sakai and Larcher, 1987). However, less is known about the amount of INMs on the land surface and actual emission rates. This study shows that INMs from birches are concentrated near the surface of the tree, especially around the trunk. Within the outer layer of the trunk, the water transport and thus the provision of nutrients is taking place, whereas the inner wood is rather important for the static support. In the bark, extra-cellular freezing mechanisms, where INPs are important, are taking place (Ashworth, 1996) and thus explain the observation of INMs found to be present more intensively on the outer layer of the trunk.
INMs were extracted from all surfaces of leaf and branch wood samples, except for two, both of which were primary wood (originated from Tree H and F). The non-active samples indicate an absence of INMs in the observed droplets of the freezing experiments. This could be caused by the hydrophobic surfaces of primary wood, leading to an INM concentration too low to be captured with our freezing assay. Nonetheless, when larger water droplets are on the surface of a plant tissue, even a small concentration of INMs could be enough to induce one ice nucleation event which can affect the entire plant (Hacker and Neuner, 2008). Felgitsch et al. (2018) analysed the INM concentration of powder extracts from birch leaves and branch woods from the same trees analysed within this work. To compare the data from this study to the previous one, we converted the cumulative nucleus concentration from cm−2 (which relates to the surface of the sample) to mg−1 (see Supplement, Fig. S7). The comparison of the K(−34 ∘C) values shows that the INM concentration of secondary wood is about 2–3 orders of magnitudes lower for surface extracts than for powder extracts. The same trend can be observed for primary wood, however less pronounced (decrease between 1–2 orders of magnitude). In contrast to the branch wood samples, the INM concentrations of leaf powder and surface extracts were greatly similar, as the surface-to-mass ratio for powder leaf samples is quite similar to intact leaf samples. Concerning Ton of analysed samples, there is a strong agreement with results from birch pollen (Pummer et al., 2012) and pulverized extracts of birch tree tissues (Felgitsch et al., 2018). These results suggest that birch tissues are a source of a large proportion of INMs in the environment.
A further finding is the ability of INMs to be extracted by rain from the surface of birches. In all precipitation collectors underneath the studied trees (which were Tree E, F, G and H for the rain samples) INMs were found to be present. K(−25 ∘C) and K(−34 ∘C) values of sample W from Tree G are approximately 2 orders of magnitude below the other two samples from this tree (S, NE). This could be either due to the sample collector been situated too far from the tree, due to the interaction between rain and the tree's surface being insufficient, or that the western part of the tree exhibits fewer INMs. Two of the trees were covered with leaves (Tree E and F), whereas the other ones were leafless (Tree G and H). Rainwater samples from trees with leaves show low variability, whereas the concentration of samples from leafless trees varies between 1.3×107 and 2.6×109 INM cm−2 active above −34 ∘C. Thus, we assume the interaction of rain droplets with the birches surface to be distributed more randomly, when no leaves are present. However, we see for two out of six samples rather high INM concentrations, and therefore leaves are not indispensable for rainfall events to extract ice nucleation material from silver birches.
When comparing the ice nucleation data of in vivo prepared extracts to in situ sampled rainwater, INM concentrations (both K(−25 ∘C) and K(−34 ∘C) values) of all samples analysed are considerably similar. Furthermore, the Ton of the trees with leaves are comparable (Tree E: Ton, −15.0 ∘C (in vivo), −15.6 ∘C (in situ); Tree F: Ton, −17.6 ∘C (in vivo), −17.2 ∘C (in situ)). In contrast, Ton values of trees with no leaves vary more widely, with in vivo extracts starting at higher freezing temperatures. Moreover, the shape of the cumulative nucleus spectra of trees with leaves (Tree E and F) differ. The curves of in vivo samples from these trees initially ascend strongly and then flatten towards colder temperatures. In contrast, the spectra of the in situ sample from Tree E is convex towards the y axis in the beginning and changes then further into a linear increase. The curves of the in situ samples from Tree F look rather linear. On the contrary, the cumulative nucleus spectra of in situ and in vivo samples from leafless trees (Tree G and H) have very similar shapes. Both in vivo and in situ sample curves of Tree G increase sharply in the beginning and flatten towards colder temperatures. The in vivo and in situ curves from Tree H have rather linear slopes on the logarithmic scale.
In our data, we found the highest freezing onset temperature on the surface of intact leaves to be −15.7 ∘C, which instead excludes the presence of highly concentrated ice-nucleating bacteria. Furthermore, we observed that INMs can be released into the environment. In 1972, Schnell and Vali discovered leaf litter to contain INPs (Schnell and Vali, 1973), which was later associated with the presence of the bacteria Pseudomonas syringae (typically active above −10 ∘C). Recently, the leaf litter was reanalysed after almost 50 years, and a variety of species inducing ice nucleation were characterized. Furthermore, Conen et al. (2016) analysed leaf litter on the ground and found concentration to be 2×102 INPs µg−1 (active above −15 ∘C). In addition, they studied the transport of these INPs and claimed that the vegetation change in autumn due to decaying leaf litter leads to an increase in atmospheric INP concentrations (Conen et al., 2016). One theory on the transport mechanism could be that INMs situated for example on the wet surface of leaves are ejected mechanically by leaf movement caused by the rain, i.e. a mechanism similar to the bioaerosol generation described by Joung et al. (2017). A rain droplet hits the INM-containing surface, and small bubbles possibly containing INMs are ejected, which can be transported further (Joung et al., 2017). It is also possible that INMs are released from birch surfaces by microscopic tornadoes generated by raindrop impacts, similar to what has been observed for spores of a fungal plant pathogen (Kim et al., 2019).
If we assume that Betula pendula and Betula pubescens, which are the main birch species found in northern Europe (Beck et al., 2016), behave similarly concerning ice nucleation activity (INA) and that the INA throughout the crown of the tree stays comparable, we can estimate the potential role of birches in environmental INM concentrations in Europe. The leaf area index (LAI) describes the leaf-area-to-ground-area ratio in a forest and varies depending on the analysed forest and the method of determination. The LAI of selected studies for northern birch forests and birch tree stands varies between 0.66 and 4.09 m2 m−2 (Karlsson et al., 2005; Dahlberg et al., 2004; Heiskanen, 2006; Johansson, 2000; Uri et al., 2007). For our calculations we assumed the LAI to be 2 m2 m−2. Assuming that the birches in northern birch forests behave similarly to our measured trees and that leaves in the upper canopy are comparable to those in the lower canopy, the aqueously extractable INM fraction is 1.99×1010 to 2.68×1012 INMs per square metre (m2) of forest for the leaves alone. Estimating the general leaf area of a tree, however, is extremely inaccurate since the density of a crown is highly dependent on the stand density (Hynynen et al., 2009). To estimate the surface of the trunk, we took the data from investigations of Betula pendula stands containing trees between 7 and 60 years of age and field data from a boreal forest. The data showed a height between 3.2 and 28 m and diameters between 32 and 640 mm (Hynynen et al., 2009; Johansson, 2000; Ene et al., 2012). Taking these values and approximating the trunk of the tree as a cone, we can estimate the surface area of the stem, leading to a surface area between 0.162 and 28.5 m2. Estimating the surface of the trunk to be on average 14.3 m2, this adds another 5.7×1012 to 2.6×1014 INM for the bark on the stem per birch tree. Taking the data from two multiple birch stand investigations the density varied between 0.04 and 10 trees per square metre (Johansson, 2000; Hynynen et al., 2009; Uri et al., 2012), leading to 2.8×1013 to 1.3×1015 INMs per square metre of the birch stands. This calculation contains an overestimation of the maximum tree surface per square metre since the densest stands are typically young stands with rather thin stems. However, comparing these calculations with the INM concentration found to be released for one tree after a rain shower (Fig. 6), we find the concentrations to be comparable in the order of magnitude. Further, considering a 10 ha birch forest in northern Europe, 2.8×1018 to 1.3×1020 INMs could be released after a 6 h of rainfall.
Indeed, the findings of our study show that INMs are released during rain events. However, possible transport mechanisms of INMs between the land surface and the atmosphere remain unclear and need further investigations. In the future, we plan to analyse the release and transport of INMs from birches into the environment using remote-controlled vehicles. Very recently we reported first results of a drone-based sampling platform that will be used to analyse airborne INMs above emission sources (e.g. birch forest) (Bieber et al., 2020). INMs are in the submicron size range (<200 nm). Aerosolized, they could be transported over great distances and hence affect atmospheric processes, e.g. cloud glaciation, which further affects precipitation formation and thus influences the hydrological cycle.
Results from this study shed new light on possible pathways of INMs between the terrestrial biosphere and the atmosphere. We found high concentrations of INMs on the surface of birches. Nearly all biological surfaces (34 out of 36) contained extractable INMs smaller than 0.2 µm, active at about −20 ∘C. Drill cores of the trunk point out the INMs to be enriched on the surface. We calculated and measured INMs to be present in orders of magnitude of about 1013 to 1015 INM per square metre of birch forest. Indeed, we suggest that rain-induced aerosolization of INMs (i.e. splashing of rain droplets on the tree's surface; soil particles serving as atmospheric shuttles of impacted INMs) contributes as a large source of biogenic INPs beside pollination season and fungal spores. The exact pathways of INMs being transported from the trees into the atmosphere during rainfall is still not elucidated. One natural assumption would be that INMs are washed off the tree's surface and deposit on the ground surface (i.e. leaf litter or soil). Through strong winds at the ground level, INMs could be aerosolized through abrasive dislodgment and transported further. Another pathway would be that the aqueous INM extracts on the ground form a liquid film and get aerosolized during the mechanical impact of following rain droplets, similar to the bioaerosol generation mechanism suggested by Joung et al. (2017), Wang et al. (2016) and Kim et al. (2020). Thereafter, splash-induced aerosol can be transported further during turbulent wind events and convection. Quantitative determination of these emission fluxes could improve scientific knowledge on aerosol–cloud interactions. We found high similarities regarding the INM concentrations between in vivo prepared extracts and in situ sampled rainwater, confirming the hypothesis that rainfall washes off INMs from birch surfaces. However, chemical identification of INMs and airborne measurements are of high importance for further studies, both of which lead to vertical profiles of INMs above emission sources. Thus, weather and climate models can be adapted to the influence of bioaerosols from alpine or boreal forests.
All data are available from the corresponding author upon request.
The supplement related to this article is available online at: https://doi.org/10.5194/bg-17-5655-2020-supplement.
TMS, PB, LF, DGS III and HG designed the experiments. JV, FR, TMS and PB performed the experiments. TMS and PB conducted the field measurement. TMS, PB and HG discussed the results. TMS, LF and PB wrote the manuscript with contributions of all co-authors.
The authors declare that they have no conflict of interest.
The authors would like to thank the Austrian Science Fund (FWF), project no. P26040, for funding. Furthermore, we thank Leopold Puchinger for his help with recording the microscopy pictures and Romana Bieber for her support during the planning phase of the field campaign. We thank Cindy Morris and the anonymous referee for helpful comments during the review process.
This research has been supported by the FWF (grant no. P26040).
This paper was edited by Paul Stoy and reviewed by Cindy Morris and one anonymous referee.
Allitt, U.: Airborne fungal spores and the thunderstorm of 24 June 1994, Aerobiologia, 16, 397–406, https://doi.org/10.1023/A:1026503500730, 2000.
Ashworth, E. N., Echlin, P., Pearce, R. S., and Hayes, T. L.: Ice Formation and Tissue-Response in Apple Twigs, Plant Cell Environ, 11, 703–710, https://doi.org/10.1111/j.1365-3040.1988.tb01153.x, 1988.
Ashworth, E. N.: Response of bark and wood cells to freezing, in: Advances in Low-Temperature Biology, 65–106, 1996.
Baker, M. B.: Cloud Microphysics and Climate, Science, 276, 1072–1078, https://doi.org/10.1126/science.276.5315.1072, 1997.
Beck, P., Caudullo, G., de Rigo, D., and Tinner, W.: Betula pendula, Betula pubescens and other birches in Europe: distribution, habitat, usage and threats, in: European Atlas of Forest Tree Species, Publ. Off. EU, Luxembourg, 70–73, 2016.
Bieber, P., Seifried, T. M., Burkart, J., Gratzl, J., Kasper-Giebl, A., Schmale, D. G., and Grothe, H.: A Drone-Based Bioaerosol Sampling System to Monitor Ice Nucleation Particles in the Lower Atmosphere, Remote Sens., 12, 552, https://doi.org/10.3390/rs12030552, 2020.
Bigg, E. K. and Miles, G. T.: The Results of Large–Scale Measurements of Natural Ice Nuclei, J. Atmos. Sci., 21, 396–403, https://doi.org/10.1175/1520-0469, 1964.
Broadley, S. L., Murray, B. J., Herbert, R. J., Atkinson, J. D., Dobbie, S., Malkin, T. L., Condliffe, E., and Neve, L.: Immersion mode heterogeneous ice nucleation by an illite rich powder representative of atmospheric mineral dust, Atmos. Chem. Phys., 12, 287–307, https://doi.org/10.5194/acp-12-287-2012, 2012.
Brush, R. A., Griffith, M., and Mlynarz, A.: Characterization and Quantification of Intrinsic Ice Nucleators in Winter Rye (Secale cereale) Leaves, Plant Physiol., 104, 725–735, https://doi.org/10.1104/pp.104.2.725, 1994.
Burke, M., Gusta, L., Quamme, H., Weiser, C., and Li, P.: Freezing and injury in plants, Ann. Rev. Plant Physiol., 27, 507–528, 1976.
Cantrell, W. and Heymsfield, A.: Production of ice in tropospheric clouds: A review, B. Am. Meteorol. Soc., 86, 795–808, 2005.
Conen, F., Stopelli, E., and Zimmermann, L.: Clues that decaying leaves enrich Arctic air with ice nucleating particles, Atmos. Environ., 129, 91–94, https://doi.org/10.1016/j.atmosenv.2016.01.027, 2016.
Conen, F., Yakutin, M., Yttri, K., and Hüglin, C.: Ice Nucleating Particle Concentrations Increase When Leaves Fall in Autumn, Atmosphere, 8, 202, https://doi.org/10.3390/atmos8100202, 2017.
Dahlberg, U., Berge, T. W., Petersson, H., and Vencatasawmy, C. P.: Modelling biomass and leaf area index in a sub-arctic Scandinavian mountain area, Scand. J. Forest Res., 19, 60–71, https://doi.org/10.1080/02827580310019266, 2004.
DeMott, P. J.: An Exploratory Study of Ice Nucleation by Soot Aerosols, J. Appl. Meteorol., 29, 1072–1079, https://doi.org/10.1175/1520-0450, 1990.
Després, V., Huffman, J. A., Burrows, S. M., Hoose, C., Safatov, A., Buryak, G., Fröhlich-Nowoisky, J., Elbert, W., Andreae, M., Pöschl, U., and Jaenicke, R.: Primary biological aerosol particles in the atmosphere: a review, Tellus B, 64, 15598, https://doi.org/10.3402/tellusb.v64i0.15598, 2012.
Diehl, K., Quick, C., Matthias-Maser, S., Mitra, S. K., and Jaenicke, R.: The ice nucleating ability of pollen: Part I: Laboratory studies in deposition and condensation freezing modes, Atmos. Res., 58, 75–87, https://doi.org/10.1016/S0169-8095(01)00091-6, 2001.
Dorsey, N. E.: The Freezing of Supercooled Water, T. Am. Philos. Soc., 38, 247–328, https://doi.org/10.2307/1005602, 1948.
Ene, L., Næsset, E., and Gobakken, T.: Single tree detection in heterogeneous boreal forests using airborne laser scanning and area-based stem number estimates, Int. J. Remote Sens., 33, 5171–5193, https://doi.org/10.1080/01431161.2012.657363, 2012.
Felgitsch, L., Baloh, P., Burkart, J., Mayr, M., Momken, M. E., Seifried, T. M., Winkler, P., Schmale III, D. G., and Grothe, H.: Birch leaves and branches as a source of ice-nucleating macromolecules, Atmos. Chem. Phys., 18, 16063–16079, https://doi.org/10.5194/acp-18-16063-2018, 2018.
Felgitsch, L., Bichler, M., Burkart, J., Fiala, B., Häusler, T., Hitzenberger, R., and Grothe, H.: Heterogeneous Freezing of Liquid Suspensions Including Juices and Extracts from Berries and Leaves from Perennial Plants, Atmosphere, 10, 37, https://doi.org/10.3390/atmos10010037, 2019.
Forster, P., Ramaswamy, V., Artaxo, P., Berntsen, T., Betts, R., Fahey, D. W., Haywood, J., Lean, J., Lowe, D. C., and Myhre, G.: Changes in atmospheric constituents and in radiative forcing. Chapter 2, in: Climate Change 2007, The Physical Science Basis, 996 pp., 2007.
Fröhlich-Nowoisky, J., Hill, T. C. J., Pummer, B. G., Yordanova, P., Franc, G. D., and Pöschl, U.: Ice nucleation activity in the widespread soil fungus Mortierella alpina, Biogeosciences, 12, 1057–1071, https://doi.org/10.5194/bg-12-1057-2015, 2015.
Fröhlich-Nowoisky, J., Kampf, C. J., Weber, B., Huffman, J. A., Pöhlker, C., Andreae, M. O., Lang-Yona, N., Burrows, S. M., Gunthe, S. S., Elbert, W., Su, H., Hoor, P., Thines, E., Hoffmann, T., Després, V. R., and Pöschl, U.: Bioaerosols in the Earth system: Climate, health, and ecosystem interactions, Atmos. Res., 182, 346–376, https://doi.org/10.1016/j.atmosres.2016.07.018, 2016.
Gorbunov, B., Baklanov, A., Kakutkina, N., Windsor, H., and Toumi, R.: Ice nucleation on soot particles, J. Aerosol Sci., 32, 199–215, https://doi.org/10.1016/S0021-8502(00)00077-X, 2001.
Gross, D. C., Proebsting, E. L., and Maccrindle-Zimmerman, H.: Development, distribution, and characteristics of intrinsic, nonbacterial ice nuclei in Prunus wood, Plant Physiol., 88, 915–922, https://doi.org/10.1104/pp.88.3.915, 1988.
Grote, M., Valenta, R., and Reichelt, R.: Abortive pollen germination: A mechanism of allergen release in birch, alder, and hazel revealed by immunogold electron microscopy, J. Allergy Clin. Immun., 111, 1017–1023, https://doi.org/10.1067/mai.2003.1452, 2003.
Hacker, J. and Neuner, G.: Ice Propagation in Dehardened Alpine Plant Species Studied by Infrared Differential Thermal Analysis (IDTA), Arctic, Antarctic, and Alpine Research, 40, 660–670, 611, https://doi.org/10.1657/1523-0430(07-077), 2008.
Haga, D. I., Iannone, R., Wheeler, M. J., Mason, R., Polishchuk, E. A., Fetch Jr., T., Van der Kamp, B. J., McKendry, I. G., and Bertram, A. K.: Ice nucleation properties of rust and bunt fungal spores and their transport to high altitudes, where they can cause heterogeneous freezing, J. Geophys. Res.-Atmos., 118, 7260–7272, https://doi.org/10.1002/jgrd.50556, 2013.
Hara, K., Maki, T., Kobayashi, F., Kakikawa, M., Wada, M., and Matsuki, A.: Variations of ice nuclei concentration induced by rain and snowfall within a local forested site in Japan, Atmos. Environ., 127, 1–5, https://doi.org/10.1016/j.atmosenv.2015.12.009, 2016.
Hegg, D. and Baker, M.: Nucleation in the atmosphere, Reports on progress in Physics, 72, 056801, https://doi.org/10.1088/0034-4885/72/5/056801, 2009.
Heiskanen, J.: Estimating aboveground tree biomass and leaf area index in a mountain birch forest using ASTER satellite data, Int. J. Remote Sens., 27, 1135–1158, https://doi.org/10.1080/01431160500353858, 2006.
Hirst, J. M.: Changes in atmospheric spore content: Diurnal periodicity and the effects of weather, T. Brit. Mycol. Soc., 36, 375–393, https://doi.org/10.1016/S0007-1536(53)80034-3, 1953.
Huffman, J. A., Prenni, A. J., DeMott, P. J., Pöhlker, C., Mason, R. H., Robinson, N. H., Fröhlich-Nowoisky, J., Tobo, Y., Després, V. R., Garcia, E., Gochis, D. J., Harris, E., Müller-Germann, I., Ruzene, C., Schmer, B., Sinha, B., Day, D. A., Andreae, M. O., Jimenez, J. L., Gallagher, M., Kreidenweis, S. M., Bertram, A. K., and Pöschl, U.: High concentrations of biological aerosol particles and ice nuclei during and after rain, Atmos. Chem. Phys., 13, 6151–6164, https://doi.org/10.5194/acp-13-6151-2013, 2013.
Hynynen, J., Niemistö, P., Viherä-Aarnio, A., Brunner, A., Hein, S., and Velling, P.: Silviculture of birch (Betula pendula Roth and Betula pubescens Ehrh.) in northern Europe, Forestry: An International Journal of Forest Research, 83, 103–119, https://doi.org/10.1093/forestry/cpp035, 2009.
Iannone, R., Chernoff, D. I., Pringle, A., Martin, S. T., and Bertram, A. K.: The ice nucleation ability of one of the most abundant types of fungal spores found in the atmosphere, Atmos. Chem. Phys., 11, 1191–1201, https://doi.org/10.5194/acp-11-1191-2011, 2011.
Ishikawa, M. and Sakai, A.: Freezing avoidance mechanisms by supercooling in some Rhododendron flower buds with reference to water relations, Plant Cell Physiol., 22, 953–967, https://doi.org/10.1093/oxfordjournals.pcp.a076259, 1981.
Ishikawa, M., Ishikawa, M., Toyomasu, T., Aoki, T., and Price, W. S.: Ice nucleation activity in various tissues of Rhododendron flower buds: their relevance to extraorgan freezing, Front, Plant Sci., 6, 1–12, https://doi.org/10.3389/fpls.2015.00149, 2015.
Isono, K. and Tanaka, T.: Sudden Increase of Ice Nucleus Concentration Associated with Thunderstorm, J. Meteorol. Soc. Jpn., 44, 255–259, https://doi.org/10.2151/jmsj1965.44.5_255, 1966.
Jaenicke, R.: Abundance of Cellular Material and Proteins in the Atmosphere, Science, 308, 73–73, https://doi.org/10.1126/science.1106335, 2005.
Johansson, T.: Biomass equations for determining fractions of common and grey alders growing on abandoned farmland and some practical implications, Biomass and Bioenergy, 18, 147–159, https://doi.org/10.1016/S0961-9534(99)00078-1, 2000.
Jones, A. M. and Harrison, R. M.: The effects of meteorological factors on atmospheric bioaerosol concentrations – a review, Science of The Total Environment, 326, 151–180, https://doi.org/10.1016/j.scitotenv.2003.11.021, 2004.
Joung, Y. S., Ge, Z., and Buie, C. R.: Bioaerosol generation by raindrops on soil, Nat. Commun., 8, 14668, https://doi.org/10.1038/ncomms14668, 2017.
Karlsson, P. S., Weih, M., and Borg, C.: Mountain birch growth in relation to climate and herbivores, in: Plant ecology, herbivory, and human impact in Nordic mountain birch forests, Springer, 71–86, 2005.
Kieft, T. L.: Ice Nucleation Activity in Lichens, Appl. Environ. Microb., 54, 1678–1681, 1988.
Kim, S., Park, H., Gruszewski, H. A., Schmale III, D. G., and Jung, S.: Vortex-induced dispersal of a plant pathogen by raindrop impact, P. Natl. Acad. Sci. USA, 116, 4917–4922, https://doi.org/10.1073/pnas.1820318116, 2019.
Kim, S., Wu, Z., Esmaili, E., Dombroskie, J. J., and Jung, S.: How a raindrop gets shattered on biological surfaces, P. Natl. Acad. Sci. USA, 117, 13901–13907, https://doi.org/10.1073/pnas.2002924117 2020.
Kishimoto, T., Sekozawa, Y., Yamazaki, H., Murakawa, H., Kuchitsu, K., and Ishikawa, M.: Seasonal changes in ice nucleation activity in blueberry stems and effects of cold treatments in vitro, Environmental and Experimental Botany, 106, 13-23, https://doi.org/10.1016/j.envexpbot.2014.02.010, 2014a.
Kishimoto, T., Yamazaki, H., Saruwatari, A., Murakawa, H., Sekozawa, Y., Kuchitsu, K., Price, W. S., and Ishikawa, M.: High ice nucleation activity located in blueberry stem bark is linked to primary freeze initiation and adaptive freezing behaviour of the bark, AoB Plants, 6, 1–17, https://doi.org/10.1093/aobpla/plu044, 2014b.
Kunert, A. T., Pöhlker, M. L., Tang, K., Krevert, C. S., Wieder, C., Speth, K. R., Hanson, L. E., Morris, C. E., Schmale III, D. G., Pöschl, U., and Fröhlich-Nowoisky, J.: Macromolecular fungal ice nuclei in Fusarium: effects of physical and chemical processing, Biogeosciences, 16, 4647–4659, https://doi.org/10.5194/bg-16-4647-2019, 2019.
Lindow, S. E., Arny, D. C., and Upper, C. D.: Bacterial Ice Nucleation: A Factor in Frost Injury to Plants, Plant Physiol., 70, 1084–1089, https://doi.org/10.1104/pp.70.4.1084, 1982.
Lohmann, U.: A glaciation indirect aerosol effect caused by soot aerosols, Geophys. Res. Lett., 29, 11-1–11-4, https://doi.org/10.1029/2001GL014357, 2002.
Mishchenko, M. I., Rossow, W. B., Macke, A., and Lacis, A. A.: Sensitivity of cirrus cloud albedo, bidirectional reflectance and optical thickness retrieval accuracy to ice particle shape, J. Geophys. Res., 101, 16973–16985, https://doi.org/10.1029/96jd01155, 1996.
Morris, C. E., Georgakopoulos, D. G., and Sands, D. C.: Ice nucleation active bacteria and their potential role in precipitation, Journal de Physique IV (Proceedings), 121, 87–103, https://doi.org/10.1051/jp4:2004121004, 2004.
Murray, B. J., Broadley, S. L., Wilson, T. W., Bull, S. J., Wills, R. H., Christenson, H. K., and Murray, E. J.: Kinetics of the homogeneous freezing of water, Phys. Chem. Chem. Phys., 12, 10380–10387, https://doi.org/10.1039/c003297b, 2010.
Murray, B. J., O'Sullivan, D., Atkinson, J. D., and Webb, M. E.: Ice nucleation by particles immersed in supercooled cloud droplets, Chemical Society Reviews, 41, 6519–6554, https://doi.org/10.1039/C2CS35200A, 2012.
Pearce, R. S.: Plant Freezing and Damage, Ann. Bot., 87, 417–424, https://doi.org/10.1006/anbo.2000.1352, 2001.
Pöschl, U.: Atmospheric Aerosols: Composition, Transformation, Climate and Health Effects, Angewandte Chemie International Edition, 44, 7520–7540, https://doi.org/10.1002/anie.200501122, 2005.
Pöschl, U., Martin, S. T., Sinha, B., Chen, Q., Gunthe, S. S., Huffman, J. A., Borrmann, S., Farmer, D. K., Garland, R. M., Helas, G., Jimenez, J. L., King, S. M., Manzi, A., Mikhailov, E., Pauliquevis, T., Petters, M. D., Prenni, A. J., Roldin, P., Rose, D., Schneider, J., Su, H., Zorn, S. R., Artaxo, P., and Andreae, M. O.: Rainforest Aerosols as Biogenic Nuclei of Clouds and Precipitation in the Amazon, Science, 329, 1513–1516, https://doi.org/10.1126/science.1191056, 2010.
Pouleur, S., Richard, C., Martin, J.-G., and Antoun, H.: Ice Nucleation Activity in Fusarium acuminatum and Fusarium avenaceum, Applied and Environmental Microbiology, 58, 2960–2964, 1992.
Prenni, A. J., Tobo, Y., Garcia, E., DeMott, P. J., Huffman, J. A., McCluskey, C. S., Kreidenweis, S. M., Prenni, J. E., Pöhlker, C., and Pöschl, U.: The impact of rain on ice nuclei populations at a forested site in Colorado, Geophys. Res. Lett., 40, 227–231, https://doi.org/10.1029/2012gl053953, 2013.
Pruppbacher, H. R. and Klett, J. D.: Microphysics of Clouds and Precipitation, 2nd ed., Kluwer Academic Publishers, 954 pp., 1997.
Pummer, B. G., Bauer, H., Bernardi, J., Bleicher, S., and Grothe, H.: Suspendable macromolecules are responsible for ice nucleation activity of birch and conifer pollen, Atmos. Chem. Phys., 12, 2541–2550, https://doi.org/10.5194/acp-12-2541-2012, 2012.
Pummer, B. G., Budke, C., Augustin-Bauditz, S., Niedermeier, D., Felgitsch, L., Kampf, C. J., Huber, R. G., Liedl, K. R., Loerting, T., Moschen, T., Schauperl, M., Tollinger, M., Morris, C. E., Wex, H., Grothe, H., Pöschl, U., Koop, T., and Fröhlich-Nowoisky, J.: Ice nucleation by water-soluble macromolecules, Atmos. Chem. Phys., 15, 4077–4091, https://doi.org/10.5194/acp-15-4077-2015, 2015.
Quamme, H. A.: Mechanism of supercooling in overwintering peach flower buds, Journal of the American Society for Horticultural Science, 103, 57–61, 1978.
Rathnayake, C. M., Metwali, N., Jayarathne, T., Kettler, J., Huang, Y., Thorne, P. S., O'Shaughnessy, P. T., and Stone, E. A.: Influence of rain on the abundance of bioaerosols in fine and coarse particles, Atmos. Chem. Phys., 17, 2459–2475, https://doi.org/10.5194/acp-17-2459-2017, 2017.
Sakai, A. and Larcher, W.: Frost Survival of Plants: Responses and Adaptation to Freezing Stress, Ecological Studies, Springer Verlag, Berlin, 321 pp., 1987.
Schnell, R. C. and Vali, G.: World-wide Source of Leaf-derived Freezing Nuclei, Nature, 246, 212–213, https://doi.org/10.1038/246212a0, 1973.
Storey, J. M. and Storey, K. B.: Cold hardiness and freeze tolerance, Functional metabolism: regulation and adaptation, 473–503, 2005.
Taylor, P. E., Flagan, R. C., Miguel, A. G., Valenta, R., and Glovsky, M. M.: Birch pollen rupture and the release of aerosols of respirable allergens, Clinical & Experimental Allergy, 34, 1591–1596, https://doi.org/10.1111/j.1365-2222.2004.02078.x, 2004.
Tobo, Y., Prenni, A. J., DeMott, P. J., Huffman, J. A., McCluskey, C. S., Tian, G., Pöhlker, C., Pöschl, U., and Kreidenweis, S. M.: Biological aerosol particles as a key determinant of ice nuclei populations in a forest ecosystem, J. Geophys. Res.-Atmos., 118, 10, https://doi.org/10.1002/jgrd.50801, 2013.
Turnbull, D. and Fisher, J. C.: Rate of Nucleation in Condensed Systems, The Journal of Chemical Physics, 17, 71–73, https://doi.org/10.1063/1.1747055, 1949.
Uri, V., Lõhmus, K., Ostonen, I., Tullus, H., Lastik, R., and Vildo, M.: Biomass production, foliar and root characteristics and nutrient accumulation in young silver birch (Betula pendula Roth.) stand growing on abandoned agricultural land, European Journal of Forest Research, 126, 495–506, https://doi.org/10.1007/s10342-007-0171-9, 2007.
Uri, V., Varik, M., Aosaar, J., Kanal, A., Kukumägi, M., and Lõhmus, K.: Biomass production and carbon sequestration in a fertile silver birch (Betula pendula Roth) forest chronosequence, Forest Ecol. Manage., 267, 117–126, https://doi.org/10.1016/j.foreco.2011.11.033, 2012.
Vali, G.: Quantitative Evaluation of Experimental Results an the Heterogeneous Freezing Nucleation of Supercooled Liquids, J. Atmos. Sci., 28, 402–409, https://doi.org/10.1175/1520-0469, 1971.
Vali, G., DeMott, P. J., Möhler, O., and Whale, T. F.: Technical Note: A proposal for ice nucleation terminology, Atmos. Chem. Phys., 15, 10263–10270, https://doi.org/10.5194/acp-15-10263-2015, 2015.
Vasebi, Y., Mechan Llontop, M. E., Hanlon, R., Schmale III, D. G., Schnell, R., and Vinatzer, B. A.: Comprehensive characterization of an aspen (Populus tremuloides) leaf litter sample that maintained ice nucleation activity for 48 years, Biogeosciences, 16, 1675–1683, https://doi.org/10.5194/bg-16-1675-2019, 2019.
Wang, B., Harder, T. H., Kelly, S. T., Piens, D. S., China, S., Kovarik, L., Keiluweit, M., Arey, B. W., Gilles, M. K., and Laskin, A.: Airborne soil organic particles generated by precipitation, Nat. Geosci., 9, 433–437, https://10.1038/ngeo2705, 2016.
Weber, C. F.: Polytrichum commune spores nucleate ice and associated microorganisms increase the temperature of ice nucleation activity onset, Aerobiologia, 32, 353–361, https://doi.org/10.1007/s10453-015-9395-1, 2016.
Wolber, P. K., Deininger, C. A., Southworth, M. W., Vandekerckhove, J., van Montagu, M., and Warren, G. J.: Identification and purification of a bacterial ice-nucleation protein, P. Natl. Acad. Sci. USA, 83, 7256–7260, https://doi.org/10.1073/pnas.83.19.7256, 1986.
Wright, T. P., Hader, J. D., McMeeking, G. R., and Petters, M. D.: High Relative Humidity as a Trigger for Widespread Release of Ice Nuclei, Aerosol Science and Technology, 48, i–v, https://doi.org/10.1080/02786826.2014.968244, 2014.
Zolles, T., Burkart, J., Häusler, T., Pummer, B., Hitzenberger, R., and Grothe, H.: Identification of Ice Nucleation Active Sites on Feldspar Dust Particles, The Journal of Physical Chemistry A, 119, 2692–2700, https://doi.org/10.1021/jp509839x, 2015.