the Creative Commons Attribution 4.0 License.
the Creative Commons Attribution 4.0 License.
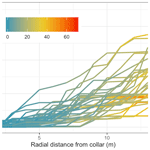
Localized basal area affects soil respiration temperature sensitivity in a coastal deciduous forest
Stephanie C. Pennington
Nate G. McDowell
J. Patrick Megonigal
James C. Stegen
Ben Bond-Lamberty
Soil respiration (Rs), the flow of CO2 from the soil surface to the atmosphere, is one of the largest carbon fluxes in the terrestrial biosphere. The spatial variability of Rs is both large and poorly understood, limiting our ability to robustly scale it in space. One factor in Rs spatial variability is the autotrophic contribution from plant roots, but it is uncertain how the presence of plants affects the magnitude and temperature sensitivity of Rs. This study used 1 year of Rs measurements to examine the effect of localized basal area on Rs in the growing and dormant seasons, as well as during moisture-limited times, in a temperate, coastal, deciduous forest in eastern Maryland, USA. In a linear mixed-effects model, tree basal area within a 5 m radius (BA5) exerted a significant positive effect on the temperature sensitivity of soil respiration. Soil moisture was the dominant control on Rs during the dry portions of the year, while soil moisture, temperature, and BA5 all exerted significant effects on Rs in wetter periods. Our results suggest that autotrophic respiration is more sensitive to temperature than heterotrophic respiration at these sites, although we did not measure these source fluxes directly, and that soil respiration is highly moisture sensitive, even in a record-rainfall year. The Rs flux magnitudes (0.46–15.0 µmol m−2 s−1) and variability (coefficient of variability 10 %–23 % across plots) observed in this study were comparable to values observed in similar forests. Six Rs observations would be required in order to estimate the mean across all study sites to within 50 %, and 518 would be required in order to estimate it to within 5 %, with 95 % confidence. A better understanding of the spatial interactions between plants and microbes, as well as the strength and speed of above- and belowground coupling, is necessary to link these processes with large-scale soil-to-atmosphere C fluxes.
- Article
(1640 KB) - Full-text XML
-
Supplement
(250 KB) - BibTeX
- EndNote
Soil respiration (Rs), the flow of CO2 from the soil to the atmosphere, is among the largest C fluxes in the terrestrial biosphere (Granier et al., 2000; Bond-Lamberty, 2018; Le Quéré et al., 2018) but remains poorly constrained both temporally and spatially at all scales. Unlike other large C fluxes such as net primary production, net ecosystem exchange, and gross primary production, Rs cannot be measured, even indirectly, at scales larger than a few square meters (Bond-Lamberty et al., 2016). Though global-scale Rs varies between vegetation types and biomes (Raich et al., 2002; Raich and Schlesinger, 1992), and responds to disturbances such as land use and climate changes (Hursh et al., 2017; Schlesinger and Andrews, 2000), it is uncertain how these patterns arise from local-scale variability, limiting our ability to robustly scale the process.
One obstacle to robust measurements of Rs is that the spatial and temporal variability is both large and poorly understood. This high variability has consequences for the sampling strategy required to accurately measure Rs at the stand scale (Rodeghiero and Cescatti, 2008; Saiz et al., 2006) and limits our ability to upscale Rs measurements to eddy covariance tower scales (Barba et al., 2018). Controls on the spatiotemporal variability of Rs differ among sites and ecosystems and include plant species, ecosystem productivity (Reichstein et al., 2003), soil temperature (Fang et al., 1998), moisture, spatial variability of vegetation, management, and soil compaction (Epron et al., 2004). The collective responses of plants and microbes to these factors determine the sensitivity of ecosystems to changes in temperature, precipitation, and other global change factors.
Plant root respiration constitutes ∼50 % of Rs in many ecosystems (Subke et al., 2006). At ecosystem scales, a number of studies have examined how the spatial distribution of Rs is affected by vegetation. Rs is typically higher closer to tree stems (Epron et al., 2004; Tang and Baldocchi, 2005) and with higher nearby stem density (Schwendenmann and Macinnis-Ng, 2016; Stegen et al., 2017). Photosynthesis is also a driver of the rhizospheric component of soil respiration (Hopkins et al., 2013) and influences seasonal trends in root contribution to total soil respiration (Brændholt et al., 2018; Högberg et al., 2001). Any spatial influences of plants on the magnitude and environmental sensitivities of Rs might thus be expected to be strong in temperate deciduous forests, as such forests tend to be highly productive (Gillman et al., 2015; Luyssaert et al., 2007).
This study examines the effect of tree proximity on measured Rs in a mid-Atlantic, deciduous forest in the Chesapeake Bay, USA, region. We hypothesized that
- i.
the amount of basal area close to Rs measurement locations would exert a significant and positive effect on measured Rs after taking into account the effects of abiotic drivers;
- ii.
this effect would occur in the growing (leaf on) season but not in the dormant (leaf off) season, because root respiration is much higher during the growing season; and
- iii.
this effect would be stronger during drier times of year, because trees might maintain access to deep soil moisture (Burgess et al., 1998) and thus continue respiring even when the surface soil is dry.
To test these hypotheses, we performed a spatially explicit analysis of 1 year of frequent Rs measurements in a temperate coastal deciduous forest in eastern Maryland, USA. Our study was conducted in the Chesapeake Bay watershed, an area subject to rapid rates of sea level rise (Ezer and Corlett, 2012; Sallenger et al., 2012) that may exert significant effects on the carbon cycling of coastal ecosystems (Rogers et al., 2019).
2.1 Site characteristics
This study was conducted in a mid-Atlantic, temperate, deciduous forest at the Smithsonian Environmental Research Center (SERC) in Edgewater, MD, USA. Three sites were chosen along Muddy Creek, a stream draining into an arm of the Chesapeake Bay. Each site was separated by ∼1 km (Fig. 1b). These sites were comprised of both lowland and upland forest with a mean annual precipitation of 1001 mm and mean annual temperature of 12.9 ∘C (Pitz and Megonigal, 2017). Dominant tree species include Liriodendron tulipifera, Fagus grandifolia, and Quercus spp.; soil types vary between Collington, Wist, and Annapolis soil (Table 1). At each site, three 20 m × 40 m plots were installed, separated by ∼25 m, and oriented perpendicular to the creek. The total elevation change between plots at each site was ∼2 m. Within each plot, we installed four 20 cm diameter PVC collars, randomly separated from each other by 2–15 m, for a total of 36 measurement collars. Collars were installed ∼1 week prior to the first sampling and left in place for the duration of the study.
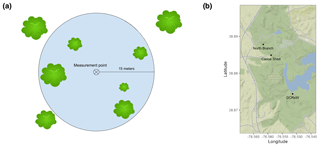
Figure 1(a) Tree proximity measurement schematic. Distance to each tree was recorded within a 15 m radius of each soil respiration measurement point, along with diameter at breast height (DBH) and species. (b) Map of the Smithsonian Environmental Research Center with the three sites labeled in black. (Map tiles by Stamen Design, under CC BY 3.0. Data by OpenStreetMap, under ODbL. © OpenStreetMap contributors 2019. Distributed under a Creative Commons BY-SA License.)
2.2 Soil respiration and ancillary measurements
Soil respiration measurements were taken using an infrared gas analyzer (IRGA; LI-8100A, LI-COR Inc., Lincoln, NE) with a 20 cm diameter opaque soil chamber attached. Measurements were taken every 10–14 d from April 2018 to April 2019. The IRGA measures concentrations every second over a one-minute period and calculates the CO2 flux as the exponential regression of CO2 accumulation in the closed chamber system over unit area and time; two successive measurements were taken at each collar and averaged. Vegetation was removed from inside the collar, and new vegetation was reclipped as necessary, to remove any aboveground autotrophic flux, so that the IRGA was measuring only soil-to-atmosphere CO2. Soil moisture and temperature (T5) were recorded at 5 cm depth, using auxiliary sensors attached to the LI-8100A, at the same time as soil respiration measurements. Temperature at 20 cm depth (T20) was also recorded using a handheld thermometer at the time of measurement.
2.3 Local basal area measurements
We recorded distance from the soil collar, diameter at breast height (1.37 m), and species of each tree within a 15 m radius of each soil respiration measurement point (Fig. 1a). Dead trees were included in the dataset but only account for <1 % of total forest basal area. Cumulative basal area was calculated at each 1 m radial distance from the collar, summing the cross-sectional areas of all trees within each distance. Tree root extent can be highly variable, but generally roots extend at least to the edge of the tree canopy (Stone and Kalisz, 1991). Mature tree canopies at SERC are ∼5 m in radius (Stephanie C. Pennington, personal observation, 2018), and we adopted this distance as an a priori assumption to test for the effect of basal area at 5 m (BA5) on Rs.
2.4 Statistical analysis
Respiration data were checked visually for artifacts or unusual outliers, but we did not exclude any data a priori. Data were then combined with the proximity measurements described above based on collar number. We used a linear mixed-effects model to test for the influence of BA5 on Rs, treating temperature, soil moisture (SM), and BA5 as fixed effects and site as a random effect (Eq. 1). Rs frequently follows a nonlinear response in relation to SM, so a quadratic SM term (Sierra et al., 2015) was included in the model. The dependent variable Rs was transformed by taking its natural logarithm to minimize heteroscedasticity, and thus the full linear model was specified as
We used restricted maximum likelihood estimation using the lme4 package (Bates et al., 2015) in R version 3.5.3 (R Development Core Team, 2019). All models were examined for influential outliers and deviations from normality. Nonsignificant terms were then eliminated using a forward-and-back stepwise algorithm (using the R package MASS version 7.3-47) based on the Akaike information criterion (AIC). Residuals from all fitted models were plotted and checked for trends or heteroscedasticity.
Our secondary hypotheses, that the effect of BA5 varies with growing season and soil moisture, were tested by subsetting the Rs data. We treated 15 April–14 October as the growing season, based on 2018 leaf-out and senescence, and 15 October–14 April as the dormant season. Soil moisture data were split up into equal thirds (low, <0.188 m3 m−3; medium, 0.188–0.368 m3 m−3; and high, >0.368 m3 m−3; all values volumetric). We then applied the statistical model described above to each subset of the data to test for BA5 significance in the model. The relaimpo package version 2.2-3 was used to calculate relative importance metrics for all terms in each model, in particular its lmg metric that averages sequential sums of squares over all orders of regressors (Lindeman et al., 1980), providing a robust decomposition of model R2.
We used the spatial variability between collars within individual plots to estimate the number of samples required for a robust estimate of the Rs population mean, i.e., a spatially representative mean. Specifically, we used a Student t test to calculate this based on the standard deviation of hourly Rs, the desired power of the test, and the allowable delta (difference from the true mean value), following Davidson et al. (2002).
We measured Rs, soil temperature, and soil moisture on 31 different days across the 1-year period (Fig. 2). Soil temperatures ranged from 0.1 to 27.5 ∘C (at 5 cm) and 1.7 to 24.4 ∘C (at 20 cm); volumetric soil moisture values were 0.01–0.56. Rs fluxes ranged from 0.35 µmol m−2 s−1 (in January 2019) to 15.3 µmol m−2 s−1 (in July 2018). The coefficient of variability (CV) between collars within plots, a measure of spatial variability, was 16.7 % ± 4.0. This implied that a large number of samples was required to estimate soil respiration accurately (Table 2).
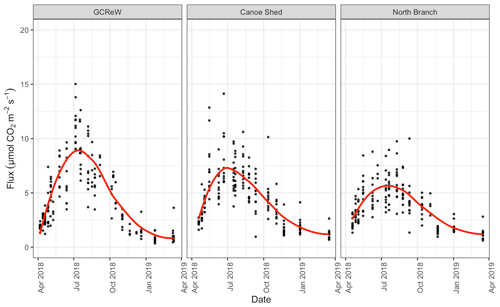
Figure 2CO2 flux over time from April 2018 to April 2019 for 36 measurement points across three sites; red line shows the seasonal trend using a loess smoother.
Table 2Sample size required to estimate soil respiration with a particular error (delta, left column, fraction of mean flux) for different statistical power values. Values are the mean (standard deviation) between plots. Power is the probability that the test rejects the null hypothesis when a specific alternative hypothesis is true and informally connotes the degree of confidence that the measurement is within some delta value of the true mean.
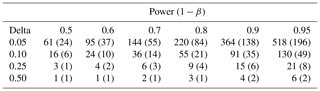
There was large variability in the basal area and number of trees close to the measurement collars (Fig. 3). The mean number of trees within 1, 5, and 10 m distance were 1, 6, and 21 trees (with respective nearby basal areas of 0.0002, 0.24, and 0.88 m2). Within our maximum radius of measurement, 15 m, there were on average 43 trees and 1.64 m2 of cumulative basal area, ranging from a minimum of 0.43 m2 to a maximum of 3.55 m2. The forest was thus highly spatially variable in its distribution of trees relative to the Rs measurement collars.
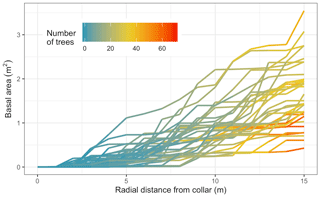
Figure 3Cumulative basal area for each soil collar (N=36) up to 15 m. Color indicates the number of trees at each distance, and each line is an individual collar.
3.1 Effect of BA on Rs
The linear mixed-effects model using temperature, soil moisture, and basal area within 5 m (BA5) predicted 37 % of the Rs variability (conditional R2=0.37). BA5 was not significant by itself in a Type III ANOVA using this model (χ2=0.081, P=0.776) but exhibited strong and significant interactions with T5 and T20 in the linear model (Table 3). In addition, the residuals of a model fit without BA5 had a significant trend with BA5 (Fig. 4). Separating the data into growing- and dormant-season subsets provided contrasting results. In the growing season, model outputs were similar to those of the full-year model, with BA5 having significant interactions with T5 and T20 (data not shown). The dormant season model, however, was quite different: only T20 (P≤0.000) and soil moisture (P=0.0377) were significant terms. In addition, the dormant season model explained more of the Rs variability (AIC = 119.80, marginal R2=0.48). In summary, collars with higher basal area within 5 m had a significantly higher temperature sensitivity of soil respiration, while basal area within 5 m of sampling points was not correlated with Rs during the dormant season.
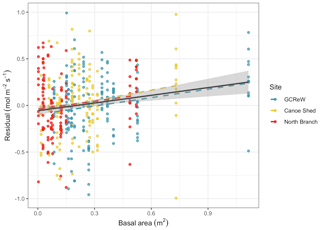
Figure 4Residuals of a soil respiration model, incorporating temperature and soil moisture as independent variables, versus cumulative tree basal area within 5 m, by site. Each point is an individual observation (see Fig. 2). Regression lines are shown for each site; the black line is the overall trend. Note that five extreme points are out of the plot but are accounted for in the regression lines.
There were strong differences between the driest and wettest thirds of the data, but our hypothesis that any basal area effect would be strongest in the driest time of year was not supported. In the driest third of the data, neither BA5 nor its interaction with T5 was significant (P=0.096 and 0.054 respectively); T20 was never significant; and the dominant control was instead soil moisture (χ2=15.23, P<0.001). In contrast, the wettest-third model resembled the full-year model, with BA5 interacting with temperature and soil moisture also significant.
3.2 Sensitivity test
Our a priori choice of 5 m for the basal area test was one of many possible choices and could potentially bias the results, as the actual extent of tree roots at these sites is unknown. Refitting the main statistical model and calculating variable importance metrics across a wide range of distances, however, showed that basal area and its interactions with T5 and T20 were almost always statistically significant (Fig. 5). Generally, the BA effects were not significant at short (<3 m) distances; this is expected, given that few collars were that close to trees. Interestingly, the BA effects remained significant all the way to our maximum measured distance of 15 m. In summary, our a priori analytical choice of a 5 m radius did not appear to bias our results.
Table 3Summary of the linear mixed-effects model testing the main hypothesis of the effect of nearby tree basal area on soil respiration (the dependent variable). Terms tested include soil temperature at 5 and 20 cm (T5 and T20 respectively), basal area (BA), and soil moisture (SM). Model AIC = 381.6, marginal R2=0.36.
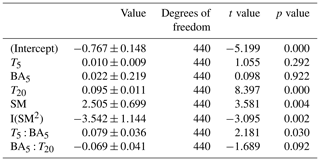
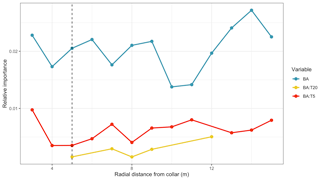
Figure 5Test of robustness of results, run at various distances from soil respiration measurement collars (x axis). Lines show the variable importance (calculated as R2 partitioned by averaging over orders; see Methods) of basal area (BA), as well as the interaction of BA and temperatures at 5 and 20 cm (T5 and T20 respectively). Vertical dashed line shows the 5 m radius used in Table 3 and Fig. 4 results. Note that the missing BA : T20 (in yellow) dots at distances <5 and >12 m mean that the terms were dropped from the model and are thus not significant.
The Rs fluxes observed in this study, 0.35–15.3 µmol m−2 s−1, were comparable to values in similar forests (Giasson et al., 2013) as well as those from the Soil Respiration Database (SRDB; Bond-Lamberty and Thomson, 2010), a synthesis of annual Rs studies (0 to 14.7 µmol m−2 s−1, n=1281 temperate deciduous studies). We observed a Rs CV of 10 %–22 % between plots, a value also comparable to previous studies. In a study of Rs in conifer forests and grasslands, Rodeghiero and Cescatti (2008) reported 28.9 %–41.5 % variability, Davidson et al. (2000) about 30 % in forest ecosystems, and a much broader range (0.11 %–84.5 %) for temperate, deciduous forests from the SRDB.
Sample size requirements to estimate annual Rs were high at SERC compared to previous studies. For example, to be within 10 % of the mean Rs flux at 95 % confidence required from 41 sample points (Davidson et al., 2002) in Harvard Forest, to 72 (Adachi et al., 2005) in a secondary forest, to 130 in this study. Within forest biomes, topography and stand structure (Søe and Buchmann, 2005) can be dominant controls. Significant spatial variation in stand structure and topography across the study domain may have resulted in the high variability seen in this study. In particular, the measurement points at our study sites ranged from 3 to 15 m in elevation (Table 1), as at all sites the land rises quickly away from Muddy Creek. These elevation gradients mean that some measurement points drain more quickly than others, creating strong differences in soil water content (CV 16.7 % ± 4.0 within plots) and thus Rs. This is consistent with the idea that topographic complexity can be an important and complex factor in Rs variation across sites (Riveros-Iregui et al., 2011).
4.1 Interactions between basal area and temperature sensitivity on Rs
Many studies have examined whether autotrophic respiration (Ra) or heterotrophic respiration (Rh) is more temperature sensitive and reached varying conclusions (Aguilos et al., 2011; Boone et al., 1998; Wei et al., 2010). In this study, the Type III SS interaction between BA5 and temperature was highly significant, meaning that collars with higher basal area within 5 m exhibited significantly higher temperature sensitivity of soil respiration. This suggests that Ra might be more sensitive to temperature than Rh at these sites. However, it is important to note that we did not directly measure the autotrophic and heterotrophic source fluxes contributing to the overall Rs flux. Instead, we assume that collars closer to trees have a larger fraction of Rs contributed by Ra, an assumption also made in previous studies such as Tang and Baldocchi (2005).
Mechanistically, these findings could be explained by a number of processes. When substrate supply from root exudates is higher during the growing season, Rs tends to be more sensitive to temperature (Luo and Zhou, 2006), presumably because under these conditions Rs is tightly coupled with photosynthesis (Ekblad and Högberg, 2001), as roots access photosynthate before microbes, and thus can respond more strongly to temperature changes. Leaf phenology likely also plays a role in a deciduous forest such as the one studied here, where the growth of photosynthetically active foliage in the spring can promote carbon allocation belowground and hence Ra. Input of leaf material in the fall may also stimulate Rh (Curiel Yuste et al., 2004; Epron et al., 2001; Ruehr et al., 2010) and is dependent on tree size and distribution (Bréchet et al., 2011).
There is also abundant evidence that soil moisture influences temperature sensitivity: Suseela et al. (2012), for example, found that Rs is less sensitive to temperature during water-limited times. If trees' roots have access to water consistently, their respiratory flux Ra measured at the soil surface as part of Rs will be more temperature sensitive on average, because Ra will be limited by soil moisture less frequently (Misson et al., 2006). It is important to note that these various mechanisms are not mutually exclusive.
4.2 Soil moisture controls on BA significance
We hypothesized that any BA5 effect would be particularly strong during the driest third of the year but instead found that only soil moisture controlled Rs during these periods, while neither temperature nor tree proximity (BA5) was significant. This demonstrates that Rs is highly moisture sensitive at these sites but does not support our idea that trees might have access to deeper or different water sources than surface soil microbes. Soil moisture is considered to be a primary Rs control in Mediterranean and desert ecosystems (Cable et al., 2010), but interestingly even this deciduous forest, in a year with record rainfall (National Weather Service, 2019), experienced significant moisture restrictions on Rs.
4.3 Dormant season Rs controls
Tree basal area within 5 m of our Rs sampling points was not significant in the dormant season model, supporting our hypothesis that total Ra contribution is often lower during the dormant reason than the growing season (Hanson et al., 2000), which suggests that Ra contributes less to Rs during the dormant season. This is expected, given the physiological link between photosynthesis and root respiration (Sprugel et al., 1995). Interestingly, T5 was not significant in the dormant season model, but rather T20 was the dominant control. The study site is in a mid-Atlantic, temperate location with cold air temperatures during the winter. Deeper soils are more insulated from cold air temperatures, allowing more favorable conditions for respiration and thus making T20 a dominant control on Rs during these times.
4.4 Limitations of this study
A number of limitations should be noted in our study design and execution. First, this was not a fully spatially explicit analysis; we did not map the collars relative to each other or construct a full spatial map of the forest stands (Atkins et al., 2018). Such mapping can be useful to examine the Rs spatial structure in more detail, as for example in Stegen et al. (2017), but our approach to mapping relative distances to trees provides an alternative spatial study construct. In a similar vein, Tang and Baldocchi (2005) measured Rs within a transect of two oak trees to draw inferences on the spatially variable contribution of Rh and Ra. Our study design still provides useful spatial information, however: the 15 m max distance in Fig. 5 implies that the range of a semivariogram, i.e., the distance of maximum autocorrelation, would be at least this far. This means that BA remained significant all the way to our maximum measured distance of 15 m, implying that the spatial influence of large trees persisted at least this far (Högberg et al., 2001).
Second, this study tested the effect of basal area on Rs, based on the assumption that BA is proportional to fine root biomass, the respiration of which is driven (with some time lag) by photosynthesis, and this in turn drives root respiration dynamics (Vose and Ryan, 2002). Stems with a diameter below 2 cm and understory were not inventoried or, as a result, included in the hypothesis-testing statistical models. If root respiration is instead correlated with number of stems, which are disproportionately small due to forest demographics, this would bias our results. There are not many understory/saplings at these sites (Table 1), however.
We found that measurement collars with higher tree basal area within 5 m had a significantly higher temperature sensitivity of Rs. Rs was also highly moisture sensitive at all of our study sites, with large differences among Rs in low- versus high-moisture times. These findings, in conjunction with large sample size requirements, suggests that the highly dynamic and variable nature of soil respiration at this site lends itself to localized basal area effects on Rs. This could have implications for measurement requirements in sites with particular stand structures. A better understanding of the spatial interactions between plants and microbes through Rh and Ra partitioning, as well as the speed and coupling between above- and belowground processes, is necessary to link these processes with collar- and ecosystem-scale soil-to-atmosphere C fluxes.
All code and data necessary to reproduce our results are available in our online GitHub repository (https://github.com/PNNL-PREMIS/PREMIS-ghg/releases/tag/v0.3-bgs-final) and permanently archived at Zenodo (https://doi.org/10.5281/zenodo.3613839, Pennington and Bond-Lamberty, 2020).
The supplement related to this article is available online at: https://doi.org/10.5194/bg-17-771-2020-supplement.
This study was designed by BBL and SCP. All fieldwork and data analysis was performed by SCP, except for the statistical analysis, which was written by BBL. NGM, JPM, and JCS provided feedback on the study design, analysis, and interpretation of results. SCP wrote the paper in close collaboration with all authors.
The authors declare that they have no conflict of interest.
This research was supported by the Smithsonian Environmental Research Center. We thank Alexey Shiklomanov for pointing out a crucial mistake in our statistical code.
This research has been supported by the Pacific Northwest National Laboratory (Laboratory Directed Research and Development, grant no. DE-AC05-76RL01830) as part of the PREMIS initiative.
This paper was edited by Michael Weintraub and reviewed by Daniel Epron and two anonymous referees.
Adachi, M., Bekku, Y. S., Konuma, A., Kadir, W. R., Okuda, T., and Koizumi, H.: Required sample size for estimating soil respiration rates in large areas of two tropical forests and of two types of plantation in Malaysia, Forest Ecol. Manag., 210, 455–459, 2005.
Aguilos, M., Takagi, K., Liang, N., Watanabe, Y., Goto, S., Takahashi, Y., Mukai, H., and Sasa, K.: Soil warming in a cool-temperate mixed forest with peat soil enhanced heterotrophic and basal respiration rates but Q10 remained unchanged, Biogeosciences Discuss., 8, 6415–6445, https://doi.org/10.5194/bgd-8-6415-2011, 2011.
Atkins, J. W., Bohrer, G., Fahey, R. T., Hardiman, B. S., Morin, T. H., Stovall, A. E. L., Zimmerman, N., and Gough, C. M.: Quantifying vegetation and canopy structural complexity from terrestrial LiDAR data using the forestr r package, edited by S. Goslee, Methods Ecol. Evol., 9, 2057–2066, 2018.
Barba, J., Cueva, A., Bahn, M., Barron-Gafford, G. A., Bond-Lamberty, B., Hanson, P. J., Jaimes, A., Kulmala, L., Pumpanen, J., Scott, R. L., Wohlfahrt, G., and Vargas, R.: Comparing ecosystem and soil respiration: Review and key challenges of tower-based and soil measurements, Agr. Forest Meteorol., 249, 434–443, 2018.
Bates, D., Mächler, M., Bolker, B., and Walker, S.: Fitting Linear Mixed-Effects Models Using lme4, J. Stat. Softw., 67, 1–48, 2015.
Bond-Lamberty, B.: New techniques and data for understanding the global soil respiration flux, Earth's Future, 6, 1176–1180, https://doi.org/10.1029/2018EF000866, 2018.
Bond-Lamberty, B. and Thomson, A.: A global database of soil respiration data, Biogeosciences, 7, 1915–1926, https://doi.org/10.5194/bg-7-1915-2010, 2010.
Bond-Lamberty, B., Epron, D., Harden, J. W., Harmon, M. E., Hoffman, F. M., Kumar, J., McGuire, A. D., and Vargas, R.: Estimating heterotrophic respiration at large scales: challenges, approaches, and next steps, Ecosphere, 7, d01380, https://doi.org/10.1002/ecs2.1380, 2016.
Boone, R. D., Nadelhoffer, K. J., Canary, J. D., and Kaye, J. P.: Roots exert a strong influence on the temperature sensitivity of soil respiration, Nature, 396, 570–572, 1998.
Brændholt, A., Ibrom, A., Larsen, K. S., and Pilegaard, K.: Partitioning of ecosystem respiration in a beech forest, Agr. Forest Meteorol., 252, 88–98, 2018.
Bréchet, L., Ponton, S., Alméras, T., Bonal, D., and Epron, D.: Does spatial distribution of tree size account for spatial variation in soil respiration in a tropical forest?, Plant Soil, 347, 293, https://doi.org/10.1007/s11104-011-0848-1, 2011.
Burgess, S. S. O., Adams, M. A., Turner, N. C., and Ong, C. K.: The redistribution of soil water by tree root systems, Oecologia, 115, 306–311, 1998.
Cable, J. M., Ogle, K., Lucas, R. W., Huxman, T. E., Loik, M. E., Smith, S. D., Tissue, D. T., Ewers, B. E., Pendall, E. G., Welker, J. M., Charlet, T. N., Cleary, M., Griffith, A., Nowak, R. S., Rogers, M., Steltzer, H., Sullivan, P. F., and van Gestel, N. C.: The temperature responses of soil respiration in deserts: a seven desert synthesis, Biogeochemistry, 103, 71–90, 2010.
Curiel Yuste, J., Janssens, I. A., Carrara, A., and Ceulemans, R.: Annual Q10 of soil respiration reflects plant phenological patterns as well as temperature sensitivity, Glob. Change Biol., 10, 161–169, 2004.
Davidson, E. A., Verchot, L. V., Cattânio, J. H., Ackerman, I. L., and Carvalho, J. E. M.: Effects of soil water content on soil respiration in forests and cattle pastures of eastern Amazonia, Biogeochemistry, 48, 53–69, 2000.
Davidson, E. A., Savage, K. E., Verchot, L. V., and Navarro, R.: Minimizing artifacts and biases in chamber-based measurements of soil respiration, Agr. Forest Meteorol., 113, 21–37, 2002.
Ekblad, A. and Högberg, P.: Natural abundance of 13C in CO2 respired from forest soils reveals speed of link between tree photosynthesis and root respiration, Oecologia, 127, 305–308, 2001.
Epron, D., Le Dantec, V., Dufrene, E., and Granier, A.: Seasonal dynamics of soil carbon dioxide efflux and simulated rhizosphere respiration in a beech forest, Tree Physiol., 21, 145–152, 2001.
Epron, D., Nouvellon, Y., Roupsard, O., Mouvondy, W., Mabiala, A., Saint-André, L., Joffre, R., Jourdan, C., Bonnefond, J.-M., Berbigier, P., and Hamel, O.: Spatial and temporal variations of soil respiration in a Eucalyptus plantation in Congo, Forest Ecol. Manag., 202, 149–160, 2004.
Ezer, T. and Corlett, W. B.: Is sea level rise accelerating in the Chesapeake Bay? A demonstration of a novel new approach for analyzing sea level data, Geophys. Res. Lett., 39, L19605, https://doi.org/10.1029/2012GL053435, 2012.
Fang, C., Moncrieff, J. B., Gholz, H. L., and Clark, K. L.: Soil CO2 efflux and its spatial variation in a Florida slash pine plantation, Plant Soil, 205, 135–146, https://doi.org/10.1023/A:1004304309827, 1998.
Giasson, M.-A., Ellison, A. M., Bowden, R. D., Crill, P. M., Davidson, E. A., Drake, J. E., Frey, S. D., Hadley, J. L., Lavine, M., Melillo, J. M., Munger, J. W., Nadelhoffer, K. J., Nicoll, L., Ollinger, S. V., Savage, K. E., Steudler, P. A., Tang, J., Varner, R. K., Wofsy, S. C., Foster, D. R., and Finzi, A. C.: Soil respiration in a northeastern US temperate forest: a 22-year synthesis, Ecosphere, 4, 140, https://doi.org/10.1890/ES13.00183.1, 2013.
Gillman, L. N., Wright, S. D., Cusens, J., McBride, P. D., Malhi, Y., and Whittaker, R. J.: Latitude, productivity and species richness: Latitude and productivity, Global Ecol. Biogeogr., 24, 107–117, 2015.
Granier, A., Biron, P., and Lemoine, D.: Water balance, transpiration and canopy conductance in two beech stands, Agr. Forest Meteorol., 100, 291–308, 2000.
Hanson, P. J., Edwards, N. T., Garten, C. T., and Andrews, J. A.: Separating root and soil microbial contributions to soil respiration: A review of methods and observations, Biogeochemistry, 48, 115–146, 2000.
Högberg, P., Nordgren, A., Buchmann, N., Taylor, A. F. S., Ekblad, A., Högberg, M. N., Nyberg, G., Ottosson-Löfvenius, M., and Read, D. J.: Large-scale forest girdling shows that current photosynthesis drives soil respiration, Nature, 411, 789–792, 2001.
Hopkins, F. M., Gonzalez-Meler, M. A., Flower, C. E., Lynch, D. J., Czimczik, C. I., Tang, J., and Subke, J.-A.: Ecosystem-level controls on root-rhizosphere respiration, New Phytol., 199, 339–351, 2013.
Hursh, A., Ballantyne, A., Cooper, L., Maneta, M., Kimball, J., and Watts, J.: The sensitivity of soil respiration to soil temperature, moisture, and carbon supply at the global scale, Glob. Change Biol., 23, 2090–2103, 2017.
Le Quéré, C., Andrew, R. M., Friedlingstein, P., Sitch, S., Pongratz, J., Manning, A. C., Korsbakken, J. I., Peters, G. P., Canadell, J. G., Jackson, R. B., Boden, T. A., Tans, P. P., Andrews, O. D., Arora, V. K., Bakker, D. C. E., Barbero, L., Becker, M., Betts, R. A., Bopp, L., Chevallier, F., Chini, L. P., Ciais, P., Cosca, C. E., Cross, J., Currie, K., Gasser, T., Harris, I., Hauck, J., Haverd, V., Houghton, R. A., Hunt, C. W., Hurtt, G., Ilyina, T., Jain, A. K., Kato, E., Kautz, M., Keeling, R. F., Klein Goldewijk, K., Körtzinger, A., Landschützer, P., Lefèvre, N., Lenton, A., Lienert, S., Lima, I., Lombardozzi, D., Metzl, N., Millero, F., Monteiro, P. M. S., Munro, D. R., Nabel, J. E. M. S., Nakaoka, S., Nojiri, Y., Padin, X. A., Peregon, A., Pfeil, B., Pierrot, D., Poulter, B., Rehder, G., Reimer, J., Rödenbeck, C., Schwinger, J., Séférian, R., Skjelvan, I., Stocker, B. D., Tian, H., Tilbrook, B., Tubiello, F. N., van der Laan-Luijkx, I. T., van der Werf, G. R., van Heuven, S., Viovy, N., Vuichard, N., Walker, A. P., Watson, A. J., Wiltshire, A. J., Zaehle, S., and Zhu, D.: Global Carbon Budget 2017, Earth Syst. Sci. Data, 10, 405–448, https://doi.org/10.5194/essd-10-405-2018, 2018.
Lindeman, R. H., Merenda, P. F., and Gold, R. Z.: Introduction to bivariate and multivariate analysis, Foresman and company, Glenview, IL, Scott, 1980.
Luo, Y. and Zhou, X.: Soil Respiration and the Environment, Elsevier/Academic Press, Amsterdam, 2006.
Luyssaert, S., Inglima, I., Jung, M., Richardson, A. D., Reichstein, M., Papale, D., Piao, S., Schulze, E.-D., Wingate, L., Matteucci, G., Aragão, L. E. O. C., Aubinet, M., Beer, C., Bernhofer, C., Black, K. G., Bonal, D., Bonnefond, J.-M., Chambers, J. L., Ciais, P., Cook, B. D., Davis, K. J., Dolman, A. J., Gielen, B., Goulden, M. L., Grace, J., Granier, A., Grelle, A., Griffis, T. J., Grünwald, T., Guidolotti, G., Hanson, P. J., Harding, R. B., Hollinger, D. Y., Hutyra, L. R., Kolari, P., Kruijt, B., Kutsch, W. L., Lagergren, F., Laurila, T., Law, B. E., Le Maire, G., Lindroth, A., Loustau, D., Malhi, Y., Mateus, J., Migliavacca, M., Misson, L., Montagnani, L., Moncrieff, J. B., Moors, E. J., Munger, J. W., Nikinmaa, E., Ollinger, S. V., Pita, G., Rebmann, C., Roupsard, O., Saigusa, N., Sanz, M. J., Seufert, G., Sierra, C. A., Smith, M.-L., Tang, J., Valentini, R., Vesala, T., and Janssens, I. A.: CO2 balance of boreal, temperate, and tropical forests derived from a global database, Glob. Change Biol., 13, 2509–2537, 2007.
Misson, L., Gershenson, A., Tang, J., McKay, M., Cheng, W., and Goldstein, A. H.: Influences of canopy photosynthesis and summer rain pulses on root dynamics and soil respiration in a young ponderosa pine forest, Tree Physiol., 26, 833–844, 2006.
National Weather Service: Record Rain and Flooding of 2018, available at: https://www.weather.gov/lwx/2018floods, last access: 30 May 2019.
Pennington, S. C. and Bond-Lamberty, B.: Code and Data Supporting Manuscript, Zenodo, https://doi.org/10.5281/zenodo.3613839, 2020.
Pitz, S. and Megonigal, J. P.: Temperate forest methane sink diminished by tree emissions, New Phytol., 214, 1432–1439, 2017.
Raich, J. W. and Schlesinger, W. H.: The global carbon dioxide flux in soil respiration and its relationship to vegetation and climate, Tellus B, 44, 81–99, 1992.
Raich, J. W., Potter, C. S., and Bhagawati, D.: Interannual variability in global soil respiration, 1980–94, Glob. Change Biol., 8, 800–812, 2002.
R Development Core Team: R: A language and environment for statistical computing. Version 3.5.3, available at: http://www.R-project.org/ (last access: 10 January 2020), 2019.
Reichstein, M., Rey, A., Freibauer, A., Tenhunen, J. D., Valentini, R., Banza, J., Casals, P., Cheng, Y., Grünzweig, J. M., Irvine, J., Joffre, R., Law, B. E., Loustau, D., Miglietta, F., Oechel, W. C., Ourcival, J.-M., Pereira, J., Peressotti, A., Ponti, F., Qi, Y., Rambal, S., Rayment, M. B., Romanya, J., Rossi, F., Tedeschi, V., Tirone, G., Xu, M., and Yakir, D.: Modeling temporal and large-scale spatial variability of soil respiration from soil water availability, temperature and vegetation productivity indices, Global Biochem. Cy., 17, 1104, https://doi.org/10.1029/2003GB002035, 2003.
Riveros-Iregui, D. A., Mcglynn, B. L., Emanuel, R. E., and Epstein, H. E.: Complex Terrain Leads to Bidirectional Responses of Soil Respiration to Inter-Annual Water Availability, Glob. Change Biol., 18, 749–756, https://doi.org/10.1111/j.1365-2486.2011.02556.x, 2011.
Rodeghiero, M. and Cescatti, A.: Spatial variability and optimal sampling strategy of soil respiration, Forest Ecol. Manag., 255, 106–112, 2008.
Rogers, K., Kelleway, J. J., Saintilan, N., Megonigal, J. P., Adams, J. B., Holmquist, J. R., Lu, M., Schile-Beers, L., Zawadzki, A., Mazumder, D., and Woodroffe, C. D.: Wetland carbon storage controlled by millennial-scale variation in relative sea-level rise, Nature, 567, 91–95, 2019.
Ruehr, N. K., Knohl, A., and Buchmann, N.: Environmental variables controlling soil respiration on diurnal, seasonal and annual time-scales in a mixed mountain forest in Switzerland, Biogeochemistry, 98, 153–170, 2010.
Saiz, G., Green, C., Butterbach-Bahl, K., Kiese, R., Avitabile, V., and Farrell, E. P.: Seasonal and spatial variability of soil respiration in four Sitka spruce stands, Plant Soil, 287, 161–176, 2006.
Sallenger, A., Doran, K., and Howd, P.: Hotspot of accelerated sea-level rise on the Atlantic coast of North America, Nat. Clim. Change, 2, 884–888, 2012.
Schlesinger, W. H. and Andrews, J. A.: Soil respiration and the global carbon cycle, Biogeochemistry, 48, 7–20, 2000.
Schwendenmann, L. and Macinnis-Ng, C.: Soil CO2 efflux in an old-growth southern conifer forest (Agathis australis) – magnitude, components and controls, SOIL, 2, 403–419, https://doi.org/10.5194/soil-2-403-2016, 2016.
Sierra, C. A., Trumbore, S. E., Davidson, E. A., Vicca, S., and Janssens, I. A.: Sensitivity of decomposition rates of soil organic matter with respect to simultaneous changes in temperature and moisture, J. Adv. Model. Earth Sy., 7, 335–356, 2015.
Søe, A. R. B. and Buchmann, N.: Spatial and temporal variations in soil respiration in relation to stand structure and soil parameters in an unmanaged beech forest, Tree Physiol., 25, 1427–1436, 2005.
Sprugel, D. G., Ryan, M. G., Brooks, J. R., Vogt, K. A., and Martin, T. A.: Respiration from the organ level to the stand, in: Resource Physiology of Conifers, edited by: Smith, W. K. and Hinckley, T. M., 255–299, Academic Press, San Diego, 1995.
Stegen, J. C., Anderson, C. G., Bond-Lamberty, B., Crump, A. R., Chen, X., and Hess, N.: Soil respiration across a permafrost transition zone: spatial structure and environmental correlates, Biogeosciences, 14, 4341–4354, https://doi.org/10.5194/bg-14-4341-2017, 2017.
Stone, E. L. and Kalisz, P. J.: On the maximum extent of tree roots, Forest Ecol. Manag., 46, 59–102, 1991.
Subke, J.-A., Inglima, I., and Cotrufo, M. F.: Trends and methodological impacts in soil CO2 efflux partitioning: A metaanalytical review, Glob. Change Biol., 12, 921–943, 2006.
Suseela, V., Conant, R. T., Wallenstein, M. D., and Dukes, J. S.: Effects of soil moisture on the temperature sensitivity of heterotrophic respiration vary seasonally in an old-field climate change experiment, Glob. Change Biol., 18, 336–348, 2012.
Tang, J. and Baldocchi, D. D.: Spatial-temporal variation in soil respiration in an oak-grass savanna ecosystem in California and its partitioning into autotrophic and heterotrophic components, Biogeochemistry, 73, 183–207, 2005.
Vose, J. M. and Ryan, M. G.: Seasonal respiration of foliage, fine roots, and woody tissues in relation to growth, tissue N, and photosynthesis, Glob. Change Biol., 8, 182–193, 2002.
Wei, W., Weile, C., and Shaopeng, W.: Forest soil respiration and its heterotrophic and autotrophic components: Global patterns and responses to temperature and precipitation, Soil Biol. Biochem., 42, 1236–1244, 2010.