the Creative Commons Attribution 4.0 License.
the Creative Commons Attribution 4.0 License.
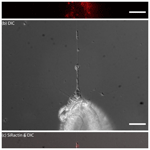
SiR-actin-labelled granules in foraminifera: patterns, dynamics, and hypotheses
Jarosław Tyszka
Ulf Bickmeyer
Jelle Bijma
Recent advances in fluorescence imaging facilitate actualistic studies of organisms used for palaeoceanographic reconstructions. Observations of cytoskeleton organisation and dynamics in living foraminifera foster understanding of morphogenetic and biomineralisation principles. This paper describes the organisation of a foraminiferal actin cytoskeleton using in vivo staining based on fluorescent SiR-actin. Surprisingly, the most distinctive pattern of SiR-actin staining in foraminifera is the prevalence of SiR-actin-labelled granules (ALGs) within pseudopodial structures. Fluorescent signals obtained from granules dominate over dispersed signals from the actin meshwork. SiR-actin-labelled granules are small (around 1 µm in diameter) actin-rich structures, demonstrating a wide range of motility behaviours, from almost stationarily oscillating around certain points to exhibiting rapid motion. These labelled microstructures are present both in Globothalamea (Amphistegina, Ammonia) and Tubothalamea (Quinqueloculina). They are found to be active in all kinds of pseudopodial ectoplasmic structures, including granuloreticulopodia, globopodia, and lamellipodia, as well as within the endoplasm. Several hypotheses are set up to explain either specific or non-specific actin staining. Two hypotheses regarding their function are proposed if specific actin labelling is taken into account: (1) granules are involved in endocytosis and intracellular transport of different kinds of cargo, or (2) they transport prefabricated and/or recycled actin fibres to the sites where they are needed. These hypotheses are not mutually exclusive. The first hypothesis is based on the presence of similar actin structures in fungi, fungi-like protists, and some plant cells. The later hypothesis is based on the assumption that actin granules are analogous to tubulin paracrystals responsible for efficient transport of tubulin. Actin patches transported in that manner are most likely involved in maintaining shape, rapid reorganisation, and elasticity of pseudopodial structures, as well as in adhesion to the substrate. Finally, our comparative studies suggest that a large proportion of SiR-actin-labelled granules probably represent fibrillar vesicles and elliptical fuzzy-coated vesicles often identified in transmission electron microscope images.
- Article
(4331 KB) - Full-text XML
-
Supplement
(1923 KB) - BibTeX
- EndNote
Since foraminifera were firstly recognised by science in the beginning of the 19th century, thanks to the work of d'Orbigny (Lipps et al., 2011), they have been the subject of extensive study. Most foraminifera species create shells (tests) that have great potential for preservation in the fossil record and are primarily important in Earth science disciplines. Application of foraminiferal research include, among others, biostratigraphy, palaeoclimatology, palaeo-environmental studies, and oil and gas exploration. As a consequence, morphology, geochemical composition, and evolution of their tests are much better understood than their biology. However, to properly understand fossils, it is essential to take into account the physiology of the living organisms. Recognition of this problem, together with advances in research methods, has led to an increasing number of studies concerning ultrastructure of foraminiferal cytoplasm and its role in biomineralisation (e.g. Spero, 1988; de Nooijer et al., 2009; Tyszka et al., 2019).
Cytoplasm in foraminifera can be divided into two parts: ectoplasm (outside the test) and endoplasm (inside the test) (e.g. Boltovskoy and Wright, 1976). They differ not only in location relative to the test but also in composition and appearance under the light microscope: endoplasm is much thicker and is usually coloured even in the non-symbiotic species, and ectoplasm is less dense and transparent. In addition, many organelles, such as nuclei, ribosomes, and Golgi apparatus are reported to occur only in the endoplasm (Travis and Bowser, 1991). The most prominent ectoplasmic structures in foraminifera are pseudopods, which have a characteristic granular appearance, distinguishing foraminifera from amoeba such as Gromia (Cavalier-Smith et al., 2018). This versatile network of branching pseudopods is involved in motility (Kitazato, 1988), feeding, construction of the test, and responding to environmental stimuli (Goldstein, 1999). As granuloreticulopodia are typically the outermost part of foraminiferal cell, these structures must fulfil a crucial role in that process. The presence of granuloreticulopodia is the most fundamental morphological feature of foraminifera and must have appeared very early in the evolutionary history of this group (Pawlowski et al., 2003). Foraminifera probably owe much of their evolutionary success to this versatile structure.
Despite numerous studies concerning structure and function of granuloreticulopodia, many aspects of their organisation and physiology are still unclear. The most striking reticulopodial features are fine granules that exhibit various behaviours. Granules move rapidly along threads of pseudopods, and even along a single thread they exhibit movement in both directions (Jahn and Rinaldi, 1959; Kitazato, 1988). There are numerous different categories of granules, including food particles (phagosomes), defecation vacuoles, mitochondria, dense bodies, clathrin-coated vesicles, and elliptical vesicles (Travis and Bowser, 1991). Granuloreticulopodia are not the only forms of exoplasmic (pseudopodial) structures present in foraminifera. Pseudopodial structures are also represented by lamellipodia (see Travis et al., 1983; Tyszka et al., 2019), globopodia, and frothy pseudopodia (see Tyszka et al., 2019). All of these pseudopodial structures are highly functional and well expressed by their different morphologies and temporal organisation linked to life strategies and behaviour.
Previous studies have shown that pseudopodial structures in foraminifera depend on cytoskeleton organisation, which includes microtubules (built from tubulin proteins) and actin filaments (Travis et al., 1983; Koonce et al., 1986b; Tyszka et al., 2019). The latest investigations of morphogenesis of foraminiferal shells revealed that chamber formation and biomineralisation are directly supported by actin meshworks and closely associated with microtubular networks (Tyszka et al., 2019). The same study also reported granularity of actin detected under fluorescent light of live actin-stained foraminifera. This active, bidirectional granular organisation of actin was observed in all types of pseudopodial structures, including reticulopodia, as well as globopodia and lamellipodia, during chamber formation of A. lessonii d'Orbigny. Motile granules followed relatively straight and often anastomosing tracks (Tyszka et al., 2019, their Movies S1–S6). However, the authors focused neither on this aspect of actin organisation nor on its dynamics. Structural and functional relationships between actin meshworks and their association with actin granularity have never been described or interpreted (see Frontalini et al., 2019).
This paper is an attempt to fill the gap in our knowledge on actin organisation and dynamics in foraminifera. Therefore, the main objectives of this study are as follows:
- a.
Live fluorescent labelling of actin within ectoplasmic (pseudopodial) structures during various behavioural and/or physiological activities;
- b.
Live fluorescent co-labelling of mitochondria to identify a relative localisation and dynamics of granules represented by mitochondria and SiR-actin-labelled structures;
- c.
Identification and detailed description of the actin cytoskeleton organisation in foraminifera, with particular focus on its granularity and dynamics by means of live fluorescence imaging;
- d.
Assessment of unspecific labelling risk in order to evaluate reliability of staining results;
- e.
Comparative analysis of published images of cytoplasmic foraminiferal ultrastructure observed in transmission electron microscope (TEM) images to identify granular structures on TEM images that may correspond to SiR-actin-labelled granules;
- f.
Interpretation and discussion of working hypotheses regarding the functionality of actin granularity and its evolutionary consequences, taking into account the physiological role of similar actin structures identified and described so far in other organisms.
2.1 Foraminiferal culture
Experiments were performed on three species of foraminifera: Amphistegina lessonii d'Orbigny, Ammonia sp., and Quinqueloculina sp. They belong to both main classes of multilocular foraminifera, i.e. the first two species belong to Globothalamea and the third species belongs to Tubothalamea. Specimens of A. lessonii were collected from the coral aquarium of Burgers' Zoo in Arnhem (the Netherlands). This aquarium contains a diverse assemblage of corals and other organisms from the Indo-Pacific, among them there are around fifty species of benthic foraminifera, including A. lessonii (Ernst et al., 2011). Samples of sediment with living foraminifera were transferred to the Alfred Wegener Institute (AWI) in Bremerhaven (Germany) and the Institute of Geological Sciences of the Polish Academy of Sciences (ING PAN) in Kraków (Poland), where cultures were established in 10 L aquaria immediately after delivery. Samples containing Quinqueloculina sp. were collected in the oceanarium as a part of the Africarium in the Zoo Wrocław (Poland) and transported to the ING PAN in Kraków, where they were cultured in 50 L aquaria. Cultures of A. lessonii were kept in 12 : 12 light : dark cycles and natural seawater (salinity of 34). Samples of mud with Ammonia sp. were collected from tidal flats in Dorum (Lower Saxony, Germany), transported to ING PAN (Kraków), and stored in 0.25–0.5 L bottles with natural seawater (salinity of 34) in a thermostatic cabinet (12 : 12 light : dark cycle; 8 ∘C).
We employed two different methods of sample preparation for observation during experiments. (1) Searching for asexual reproduction events, we monitored large individuals of A. lessonii climbing the glass walls of the aquaria. The juvenile individuals (2–5 d old) were picked up using a fine paintbrush and transferred into a sterile imaging Petri dish (ibidi® polymer coverslip bottom) containing 2 mL of clean culture medium (up to 10 individuals per dish). After one dark phase of the light : dark cycle, when individuals attached to the coverslip bottom, they were examined under a stereomicroscope looking for pseudopodial activity and chamber formation. This method was applied to run chamber formation experiments (for more details, see the Supplement to Tyszka et al., 2019).
(2) In the second method, adult individuals of A. lessonii, Ammonia sp., and Quinqueloculina sp. were picked from the culture aquaria or bottles and cleaned with fine paintbrushes under the stereomicroscope to remove algae and grains of sediment covering the specimens. Following this, they were transferred to glass-bottomed Petri dishes, which had been previously treated with hydrochloric acid over 16 h, containing 2 mL low-calcium artificial seawater, prepared as described in Bowser and Travis (2000). The reason for using low-calcium artificial seawater was to avoid the so-called beading response (Bowser and Travis, 2000). This response causes transition of pseudopodia into droplets and may be provoked by different chemical and physical factors (Travis et al., 1983). After acclimation to the low-calcium seawater, when reticulopodia were extended and adhered to the glass bottom, specimens were stained and observed. Toyofuku et al. (2008) reported that incubation of foraminifera in low-calcium seawater for 3 d significantly decreases the level of intracellular calcium. As a result, the presence of intracellular calcium cannot be deducted with fluorescence probes. However, we always started our experiments immediately after reticulopodia were extended (usually within 0.5 h after the transfer to low-calcium seawater), and within this period the signal did not decrease below the detectable level. The second method was employed for observations of reticulopodia.
2.2 Fluorescent probes and staining procedure
We focused on staining F-actin with SiR-actin but also used MitoTracker™ Green to stain mitochondria and Celltrace™ calcein red–orange AM for staining cytoplasm. For experiments focusing on actin organisation during chamber formation (Figs. 3–4 and S1–S2 in the Supplement and Movie S2; for further information, please see the section “Video Supplements” at the end of the paper and the Supplement for an explanatory text), we added a stock solution of probes prepared according to the manufacturers' instructions directly onto the imaging Petri dish containing living specimens of A. lessonii up to a final concentration of 1 µM. For experiments regarding reticulopodia (Figs. 1–2, 5–6, S3–S5, and Movie S1), the concentration of SiR-actin was 0.5 µM and the concentration of MitoTracker™ Green was 1 µM. SiR-actin is a cell-permeable, fluorogenic probe-labelling F-actin, thus it is suitable for live-staining (Lukinavičius et al., 2014).
After 15–20 min the signal was sufficient to perform observations. Calcein red–orange AM is a cell-permeable dye that stains the cytoplasm of living cells and is often used to indicate the viability of cells (Frontalini et al., 2019). Calcein AM is hydrolysed in the cytosol and fluoresce in the presence of calcium ions. It differs in chemical structure and fluorescence from the calcein AM used for staining cytoplasm by Ohno et al. (2016). In our experiments the main purpose of using Calcein red–orange AM was to indicate the limits of the cytoplasm and highlight 3-D structure of pseudopodia (e.g. globopodium). Live cell structures are stained with this dye even if they are surrounded by calcium-free artificial seawater, as calcium ions are always present within living cells. Table S1 (in the Supplement) summarises information about fluorescent probes used in the presented research.
Absorption and emission parameters of the SiR-actin probe overlap with the autofluorescence of chlorophyll from endosymbionts, thus distinguishing between SiR-actin signal and autofluorescence emitted from the endoplasm of A. lessonii or other species hosting endosymbionts is difficult. To minimise the problems caused by autofluorescence, we focused primarily on pseudopods where endosymbiotic algae are not present. Chlorophyll and other substances present in food particles captured by pseudopods may also give some fluorescent signal. To ensure that such food particles are absent within observed pseudopods, all specimens were starved for 24 h prior to staining and observation.
2.3 Fluorescence and transmitted light microscopy
Images were obtained with a Leica SP5 inverted confocal microscope at the AWI and with a Zeiss Axio Observer Z1. equipped with ApoTome.2 at the ING PAN in Kraków. ApoTome.2 is a device that enables the removal of scattered light in fluorescence imaging. It takes between 3 and 15 images with different positions of a grid placed in the light path between the fluorescent lamp and the sample. On the basis of those images, the dedicated ApoTome software calculates optical sections of the sample using a structured illumination principle to enhance the signal to noise ratio of the image (Weigel et al., 2009). In cases where living samples containing moving structures it may result in the multiplication of some rapidly moving objects. Because foraminiferal ectoplasmic structures are highly dynamic, we choose to set up ApoTome.2 to take only three pictures per frame and use maximum light intensity to decrease exposure time. Despite this, the most rapidly moving objects may appear in triplicate in some images. Hardware settings used for obtaining images are summarised in Table S2 (exposure time, binning mode, objectives, and Apotome mode in experiments conducted on a Zeiss Axio Observer Z1), Table S3 (hardware settings in experiments conducted on a Leica SP5 inverted confocal microscope), and Table S4 (filter sets used for different fluorescent dyes for experiments performed on a Zeiss Axio Observer Z1). We optimised these settings using a trial and error method. To provide additional information over all structures of observed individuals, we captured bright field images for experiments with the Leica SP5 and Nomarski contrast imaging for the experiments with the Zeiss Axio Observer Z1. Nomarski contrast or differential interference contrast (DIC) is a microscopy technique utilising the interferometry principle for improving contrast in transparent objects (Lang, 1968).
2.4 Measurement of velocity of the actin-labelled granules
For measuring the velocities of granules in pseudopodia we used time-lapse records of pseudopodia labelled with SiR-actin using the Zeiss Axio Observer Z1. A time-lapse movie consisting of 50 frames was recorded with 0.419 s time interval between timeframes (Movie S4). SiR-actin-labelled granules (ALGs) can display very rapid movement, therefore tracking requires dense time-lapses. To minimise the time necessary for capturing a single frame, we used only one fluorescent channel (without ApoTome).
The TrackMate plug-in (Tinevez et al., 2017), applied to the Fiji software (Schindelin et al., 2012), was used for calculating the velocities of the ALGs. Calculating velocities required two main steps: (1) annotation of spots representing ALGs in each of the frames of the time-lapse and (2) creating links between particular spots in subsequent frames in the time-lapse (tracks of movement of spots). The software allows for choosing several options for both of the main steps. This can be done either manually or automatically (with several different options in the latter case). We used the LoG (Laplacian of Gaussian) detector for automatic annotation. As the approximate size of the ALGs is 1 µm, we used 1 µm as a “blob” size parameter in the LoG detector. The threshold value was set to up to 100. Blob is a technical term used in the tracking of any objects during time-lapse measurements. These values were optimised by trial and error to minimise two types of errors: (1) lack of annotation of some objects that can be clearly identified on the images and (2) annotation as spots areas with no apparent ALGs. It is not always easy to track which blobs in frame n+1 match with those in frame n. ALGs may temporally get so close to each other that it limits their separation in subsequent frames. To recognise this we allowed for merging and splitting the tracks. After testing automatic and manual options for the second step (creating links between spots), we decided to perform it manually, as automatic methods seem to give random results for the ALGs. When spots are annotated and links between spots in the subsequent frames are created, velocities of spots are measured automatically.
3.1 Identification of SiR-actin-labelled structures by fluorescence microscopy
Fluorescent SiR-actin labelling has revealed three considerably different patterns of staining in Ammonia sp., i.e. (1) weak but non-uniform staining following all membranous surfaces of pseudopodial structures, (2) linear or ring-like structures showing intense fluorescence, and (3) small but strongly labelled granular structures that often exhibit very rapid dynamics (Fig. 1; Movie S1). The term SiR-actin-labelled granules (ALGs) is introduced here for these small oval objects. Their size has been estimated to be approximately 1 µm. This is consistent with measurement of the size of objects corresponding to ALGs seen in Nomarski contrast (DIC) images (Figs. 1, 2).
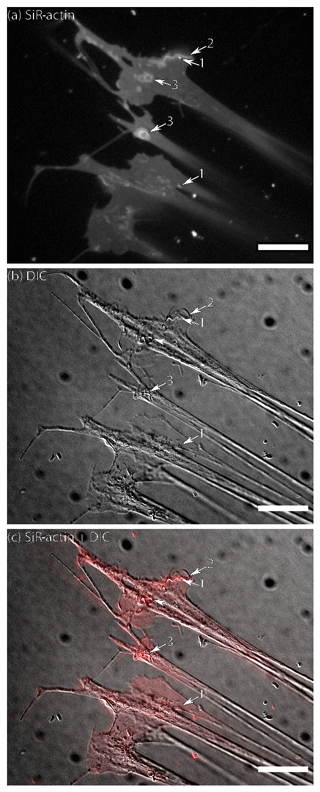
Figure 1Flattened lamellipodia of living Ammonia sp. attached to glass: (a) fluorescence of SiR-actin-labelled structures, (b) DIC image of the same area, and (c) merged image of fluorescence and DIC channels (since the reticulopodia were moving, the DIC image is slightly shifted in relation to fluorescent one). The numbers indicate the following objects: 1 – actin-labelled granules (ALGs); 2 – linear SiR-actin-labelled structures; 3 – SiR-actin-labelled rings. Note the weak but non-uniform SiR-actin labelling following all membranous surfaces of pseudopodial structures. The linear structure (2) was subsequently transformed into a ring structure (see Movie S1). Structures corresponding to ALGs, SiR-actin-labelled rings, and linear structures can be seen in DIC image. Conventional fluorescence images were obtained with a Zeiss Axio Observer Z1 (scale bar 20 µm).
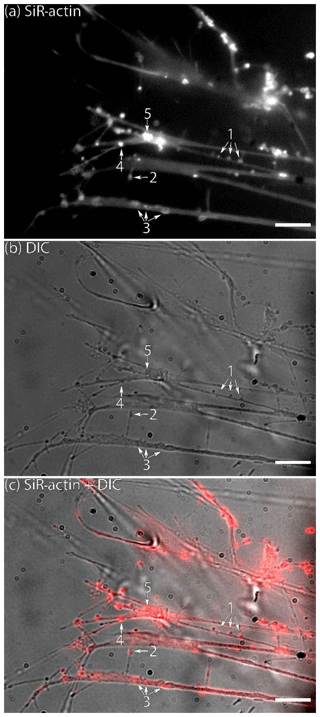
Figure 2Pseudopodia of living Ammonia sp. attached to glass: (a) conventional fluorescence of SiR-actin-labelled structures, (b) DIC image of the same area, and (c) merged image of fluorescence and DIC channels (since the reticulopodia were moving, the DIC image is slightly shifted in relation to fluorescent one). Weak but non-uniform actin labelling following all membranes can be seen in pseudopodia. The number indicate the following objects: 1 – group of three SiR-actin-labelled granules (ALGs) transported along one thread of pseudopodia; 2 – actin in the tip of thin filopodium; 3 – larger SiR-actin-labelled areas showing smudgy fluorescence weaker than in most ALGs; 4 – single ALG in bifurcation of reticulopodia; 5 – a group of very bright densely packed ALGs in the thick reticulopodium. Conventional fluorescence images were obtained with a Zeiss Axio Observer Z1 (scale bar 10 µm).
ALGs are present within lamellipodia covering the foraminiferal tests in A. lessonii (Figs. 3 and S1–S2 in the Supplement) or any other structure they are attached to; finger-like rhizopodial structures, constructing outer protective envelopes of chamber formation sites (Fig. 4); and reticulopodia during feeding and locomotion (Fig. S3 in the Supplement). They can be identified in endoplasmic structures within the chambers of non-symbiont-bearing species such as Quinqueloculina sp., close to surfaces of internal walls of the test (Fig. 5). At first glance ALGs seem to show fast and random movements but actually they can display different “behaviours”.
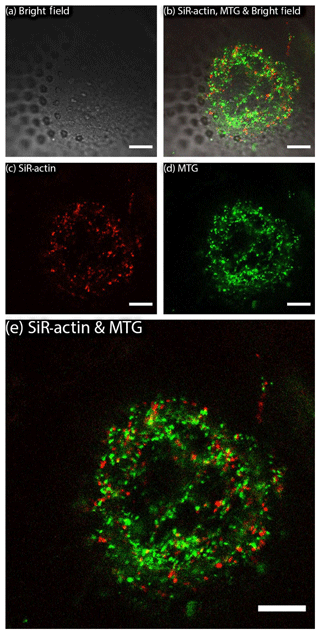
Figure 3SiR-actin-labelled granules (ALGs) and mitochondria in cross section of a newly forming chamber in Amphistegina lessonii during biomineralisation (pores are already visible in transmitted light). ALGs and mitochondria do not show co-localisation. Images were obtained with Leica SP5 inverted confocal microscope (scale bar 10 µm). For the entire time-lapse, see Movie S2.
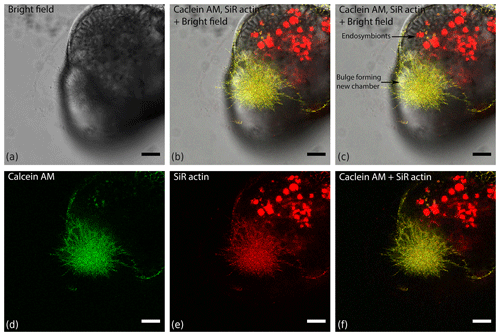
Figure 4Organisation of actin within a finger-like structure preceding globopodium during chamber formation in Amphistegina lessonii, compared with localisation of cytoplasm stained with calcein red–orange AM. Images was obtained with a Leica SP5 inverted confocal microscope (scale bar 20 µm).
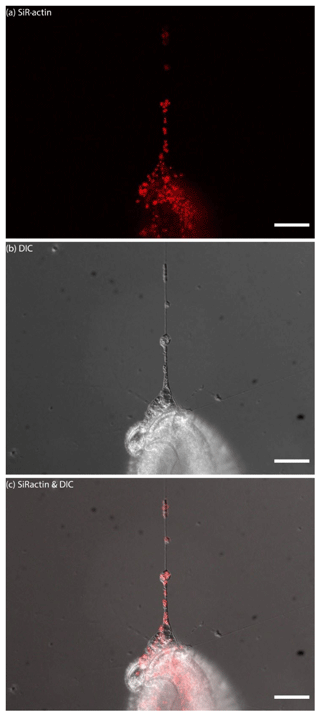
Figure 5SiR-actin-labelled granules (ALGs) in pseudopodia and endoplasm of Quinqueloculina sp. Panel (a) presents actin stained with SiR-actin, panel (b) presents images obtained with DIC optics (inverted LUT), and panel (c) presents merged fluorescent and DIC channels. Conventional fluorescence images were obtained with a Zeiss Axio Observer Z1 (scale bar 50 µm).
Structures labelled with SiR-actin can be matched with pseudopodial structures and granular microstructures identified in Nomarski contrast (DIC) or in a bright field images. Figures 1–2 present a lamellipodial structure attached to the glass surface with a weak, dispersed fluorescent signal of SiR-actin staining the F-actin meshwork. Very fine bright spots represent ALGs that match with granules observed with DIC optics.
3.2 Testing the selectivity of labelling of granules: SiR-actin-labelled granules vs. mitochondria
Direct comparative analysis of fluorescence vs DIC images of A. lessonii indicate that SiR-actin-labelled granules do not overlap with all granules observed in DIC (Fig. S3 in the Supplement). This means that SiR-actin does not stain all the granules observed. Therefore, labelling of ectoplasmic granules is selective. In order to test ALGs' relationships with selected, well-defined granules, mitochondria were chosen for double-labelling experiments. Mitochondria were the best candidates because they had frequently been recognised within the cytoplasm, including reticulopodia (e.g. Travis and Bowser, 1986; Hottinger, 2006; Nomaki et al., 2016; LeKieffre et al., 2018a). Mitochondria usually appear oval or kidney shaped in cross section, with a length in the range of 0.5 to 1 µm, although they are sometimes larger and take various, even tubular shapes (LeKieffre et al., 2018a).
MitoTracker™ Green has been applied to living specimens of A. lessonii following the procedure described above (Sect. 2). This probe selectively accumulates in the mitochondrial matrix by covalent binding to mitochondrial proteins (Presley et al., 2003). Results of replicated live experiments do not show co-localisation of ALGs and mitochondria stained by MitoTracker™ Green (Figs. 3 and S3 and Movie S2). Therefore, they indicate that mitochondria and SiR-actin-labelled granules are two non-overlapping categories.
3.3 Dynamics of SiR-actin-labelled granules
The dynamics of the ALGs (velocity and overall pattern of movement) are described separately in granuloreticulopodia and in a globopodium during chamber formation. The dynamics may vary for different locations in the cell. Not all of the ALGs have the same pattern of movement. At first glance their movement may appear chaotic, but closer analysis reveals some general patterns.
For the sake of simplicity, particular threads of granuloreticulopodia may be considered one-dimensional structures that constrain the possible directions of movement: they can move along the thread of reticulopodia either inward or outward. Indeed bidirectional movement along a single thread is commonly observed in A. lessonii (Figs. S4–S5 in the Supplement), however, in cases of thick pseudopodial threads there may by a spatial separation: in the core of pseudopodium, ALGs move towards the cell body, while in the cortex they travel in the opposite direction (Movie S3). Usually one direction is dominant: when reticulopodia are formed, outward (centrifugal) transport is more common, but during retraction of reticulopodia inward (centripetal) movement is prevalent. During extension of a newly formed very fine thread of pseudopodium, there is usually a single ALG at the tip of this thread (Fig. 6). Sometimes clusters of granules moving together with the same speed along a pseudopodium may be identified. As the granuloreticulopodia themselves are very dynamic structures, it is not always possible to measure displacement of ALGs due to the absence of a stationary reference frame. Another problem is that ALGs can be so abundant in reticulopodia that they may be extremely difficult to track. To overcome this problem intervals between subsequent frames in time-lapse movies were minimised. Using this strategy we recorded time-lapse movies (Movie S4) showing a wide range of velocities of ALGs in reticulopodia of A. lessonii up to 15.4 µm s−1 (Fig. S8 and Tables S5–S6 in the Supplement).
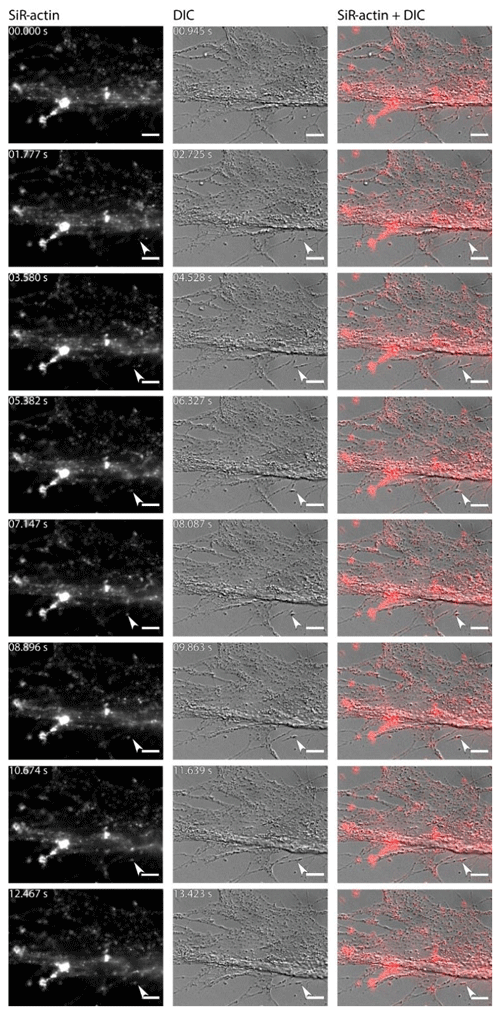
Figure 6Dynamics of SiR-actin-labelled granules (ALGs) in reticulopodia of Amphistegina lessonii. Eight frames of the time-lapse. Right column: actin stained with SiR actin; middle column: DIC; right column: overlay of fluorescent and DIC channels. Arrows indicate a granule in the tip of one very fine thread of forming pseudopodium. The numbers in top-right corner of each image of SiR actin and DIC channels indicate the time of acquisition. Conventional fluorescence images were obtained with a Zeiss Axio Observer Z1 (scale bar 10 µm).
Lamellipodia covering the test form two-dimensional sheets, resulting in a more complex pattern of displacement of ALGs than the one observed in granuloreticulopodia. There are areas dominated by directional protoplasm streaming and areas showing less organised behaviour. Accordingly, actin granules can be divided into several categories based on dominant movement patterns: (1) stationary or almost stationary ALGs that oscillate within a very narrow space, (2) ALGs showing saltatory movements as described in Travis and Bowser (1991), and (3) ALGs exhibiting extremely rapid movement that can be observed for up to a few seconds. Moreover, in some areas actin granules may move along a single line but with different velocities and in different directions. They may pass some stationary granules with no significant interaction observed.
4.1 Assessment of unspecific fluorescent labelling risk
All microscopy techniques are associated with a risk of capturing artefacts instead of imaging target structures. In the case of fluorescence microscopy, the greatest danger is unspecific labelling or autofluorescence. Comparison of stained individuals of A. lessonii to an unstained control shows that the SiR-actin fluorescent probe indeed stains endoplasmic structures in foraminifera (Fig. S6 in the Supplement). This may be caused when the concentration of the probe is too high or when the excitation intensities (or emission measurement sensitivity) are too high. Another problem might be that the probe is not specific enough and binds to other chemical compounds in the cell, the structure of which mimics the target structure. Most fluorescent probes were developed and tested to study mammalian cells; therefore, the risk of unspecific fluorescent labelling should be addressed to avoid confusion and over-interpretation of the results. Foraminifera are placed in the actin phylogenetic tree with Bikonta (Flakowski et al., 2005), thus the amino acid sequence of actin in foraminifera is significantly distinct from the actin sequence in Metazoa or fungi (belonging to opisthokonts) that are subject of most intensive research of actin physiology. Fluorescent probes may therefore interact differently with actin in foraminifera. Moreover, foraminifera may contain other organic compounds that mimic actin nanostructures and therefore interact with fluorescence probes, such as SiR-actin. It should be noted, however, that our results are reproducible.
As the granular pattern of SiR-actin staining is unusual compared to other eukaryotes, it requires an extensive discussion of all possible scenarios (see Fig. S7). There are three possible scenarios in which ALGs may represent real F-actin-containing structures that are labelled by the SiR-actin probe (Fig. S6a–c in the Supplement) and three additional possibilities that would reveal the observed patterns as artefacts (Fig. S6d–f in the Supplement). The first and most likely scenario (Fig. S6a in the Supplement) assumes that foraminifera possess granular structures filled with densely packed actin filaments that are specifically stained with SiR-actin. These structures possibly correspond to fibrillar vesicles known from TEM ultrastructure studies (see below in Sect. 4.5.1). According to the second scenario, labelled actin filaments surround some membranous vesicles (Fig. S7b in the Supplement). These vesicles are possibly involved in transport and endocytosis, and F-actin probably plays role in those processes. Alternatively, they may correspond to elliptical fuzzy-coated vesicles described by Koonce et al. (1986a) regulating motility of reticulopodia (see below Sect. 4.5.2 Elliptical fuzzy-coated vesicle). The third scenario assumes that actin filaments are located both inside and outside of some membrane-bound vesicles (Fig. S6c). Alternatively, the observed staining pattern may be explained as an artefact if SiR-actin binds to another, unidentified, organic molecule that is different from (and thus not associated with) F-actin either inside (Fig. S6d in the Supplement) or outside (Fig. S6e in the Supplement) of membranous vesicles. Lastly, SiR-actin may induce assemblage of actin filaments in the areas rich in G-actin (Fig. S6f in the Supplement), as suggested by Melak et al. (2017).
The first argument supporting the reliability of SiR-actin live staining is the fact that attachment sites of pseudopodia to the substratum often demonstrate a strong fluorescence signal (Fig. 1), as predicted from the essential role of actin for adhesion (Bowser et al., 1988). Secondly, as mentioned above, granular actin structures are visible on images of fixed reticulopodia stained with phalloidin (see Koonce et al., 1986a, their Fig. 3c; Koonce et al., 1986b, their Fig. 1f). It cannot be excluded, however, that ALGs are associated with a defence strategy to remove and dispose of toxic compounds introduced into the cell. If this were true, we would expect vesicles containing those probes to be transported outward. As ALGs are often moving bidirectionally (both inwards and outwards) (Figs. S4, S5 in the Supplement), this hypothesis is not very convincing. In fact, ALGs' inward movement is observed when a pseudopodial structure is being withdrawn and seems to indicate relocation of labelled actin into the endoplasm. Such observations support the notion that live staining using SiR-actin is specifically labelling actin and that the inward movement of ALGs is a functional response during withdrawal of pseudopodial structures.
Another issue that needs to be considered is the risk of interference of a probe with the physiology of actin itself, as it may, for instance, cause an artificial polymerisation of F-actin (Melak et al., 2017). In that case, we would expect negative interference of SiR-actin on morphogenesis and biomineralisation of new chambers. Nevertheless, such staining artefacts have never been observed (Tyszka et al., 2019). Moreover, if SiR-actin causes polymerisation of F-actin, live actin staining should have a visible impact on organisation and motility of pseudopodia. In our observations we did not recognise any apparent long-term differences either in morphology or in dynamics of reticulopodia after staining. Occasionally, we observed a temporary retraction of pseudopods immediately after adding the staining solution to the petri dish. However, after 10–15 min incubation with SiR-actin, this effect was not visible any more, and reticulopodia were spread out again, closely resembling the pre-staining structure and dynamics. A. lessonii is less prone to retraction of pseudopods and recovers faster than Ammonia sp. or Quinqueloculina sp. after applying the staining solution.
4.2 Previous studies of actin in foraminifera using fluorescent labelling
The most commonly used method of fluorescent labelling of the actin cytoskeleton is phalloidin staining (Melak et al., 2017). Its utility is limited mostly to staining fixed cells. Phalloidin staining was previously employed to study the actin cytoskeleton in reticulopodia of a few species of foraminifera, i.e. mainly Reticulomyxa filosa (Koonce et al., 1986a, b) and Allogromia sp. (Bowser et al., 1988). Actin staining of R. filosa showed cable-like structures concentrated in the cortex of the reticulopodia as a dominant pattern of actin organisation in reticulopodia. Along those structures there are visible granular actin structures in the figures of the cited publications that are not discussed or mentioned by the authors (Koonce et al., 1986a, their Fig. 3c; Koonce et al., 1986b, their Fig. 1f). In Allogromia sp. the actin cytoskeleton has a different organisation depending on the location in the reticulopodium: in proximal parts of pseudopodia it is a thick linear fibre; in more distal regions flattened on the glass it is visible only in a few locations, resembling the SiR-actin-labelled granules in our study; and in the most peripheral areas actin staining is absent (Bowser et al., 1988, their Figs. 1c, 2c, 3c). We suspect that the structure in the distal regions flattened on the glass corresponds to the ALGs described in our paper.
Although Fig. 1 demonstrates an SiR-actin-labelled linear structure and Fig. 4d presents indistinct SiR-actin-labelled fibres, clear cable-like structures are absent in our study in comparison to previous publications which may be a result of different staining procedures. This is due to the fact that every probe may have affinity to different epitopes of F-actin, therefore, may not label all different F-actin-containing structures equally. The effectiveness of staining F-actin using different probes was compared by Lemieux et al. (2014). They reported that different probes did not stain all subsets of F-actin equally. Apparently, the staining effectiveness of F-actin depends on the location of actin filaments within the cell. Even though this analysis does not include SiR-actin, the same issue may apply to this probe. The interaction between probes and F-actin may also lead to stabilisation or enhanced polymerisation of F-actin due to its structural similarities to Jasplakinolide (Melak et al., 2017). In addition, cell fixation procedures may stabilise dynamic structures or create some artefacts.
Previous studies of the dynamics of granules in foraminifera were conducted mostly on Allogromia and Astramina. The maximum speed of granules within reticulopodia was reported to be approximately 25 µm s−1, but most of them have velocities below 10 µm s−1 (Travis and Bowser, 1991). Velocities of ALGs fall within this range. The average speed of granules in foraminiferal pseudopodia reported by Kitazato (1988) is 13 µm s−1, which is roughly comparable to the maximum velocity recorded by us (15.5 µm s−1).
4.3 Main hypothesis regarding the functionality of actin-labelled granules
Actin-labelled granules described in this paper appear to be one of the main forms of actin cytoskeleton organisation in external cytoplasm (ectoplasm) of foraminifera. As they are ubiquitous in pseudopodia during feeding behaviour and in globopodia during chamber formation, they probably serve an important physiological role. At present, it is difficult to determine their function, however, there are a few hypotheses that could be proposed based on two sources of data.
As mentioned above, there are two possible explanations of the role of ALGs: (1) either ALGs mediate transportation of various types of cargo or (2) they are involved in transport of prefabricated or recycled actin fibres. The following paragraphs are dedicated to the discussion of these hypotheses. Firstly, we will discuss the relation of actin granules in foraminifera to similar structures described in other organisms. There are actin patches known from some fungi and fungi-like protists. Secondly, we compare actin granules to different ultrastructures known mostly from TEM images of foraminifera. We will focus on organelles or structures whose functions are questionable, e.g. fibrillar vesicles (LeKieffre et al., 2018a; Goldstein and Richardson, 2018) or elliptical fuzzy-coated vesicles, which are also known as motility-organising vesicles (Travis and Bowser, 1991).
4.3.1 Comparison of actin structures in other organisms
Structures similar to SiR-actin-labelled granules described in foraminifera have been found in other organisms. They are present in water moulds, e.g. Saprolegnia ferax (Geitmann and Emons, 2000), Phytophthora infestans (Meijer et al., 2014), as well as in yeast, Saccharomyces cerevisiae (Moseley and Goode, 2006; Rodal et al., 2005; Waddle et al., 1996; Winter et al., 1997), where they are abundant in buds. They are referred to as cortical actin patches in budding yeast and S. ferax (Geitmann and Emons, 2000) or actin plaques in P. infestans (Meijer et al., 2014). In these organisms they occur alongside different actin structures such as actin cables or rings.
Fluorescent images of Saprolegnia ferax (Geitmann and Emons, 2000) indicate that actin patches have a globular shape and diameters of approx. 0.5 µm. In yeast they appear to have a similar size. Therefore, their size is comparable to SiR-actin-labelled granules in foraminifera. The maximum velocity of actin patches observed in yeast is 1.9 µm s−1 (Waddle et al., 1996), thus it is significantly lower than the velocity of actin granules in foraminifera. Cortical actin patches are most likely involved in endocytosis (Moseley and Goode, 2006) and cell growth (Geitmann and Emons, 2000). For instance in budding yeast actin patches are present during budding within the daughter cell.
In foraminifera, ALGs appear in large numbers in the course of chamber formation, as well as within reticulopodia, which are known for their ability for rapid extension and retraction. Formation of a globopodium and reticulopodia in foraminifera and budding in yeasts require quick expansion of the cytoplasm and may share similar mechanisms facilitating those processes. Assembling actin filaments may generate a physical force that can be used to provide the pressure required for expansion of new protoplasm (Mogilner and Oster, 2003).
4.3.2 Comparison of SiR-actin-labelled granules to organelles identified in TEM images of foraminifera
Transmission electron microscopy (TEM) is a principal method for investigating cell ultrastructures. However, TEM images alone do not provide information about the chemical composition of certain structures. In contrast to TEM, fluorescent labelling sometimes gives detailed insight into the chemical composition of certain areas of the cell but in much lower resolution. Thus, combining the two approaches is essential to unravel the ultrastructure and chemical make-up and thus provide clues about the function of cell components. Hence, for a better understanding of the role of actin granules in foraminiferal cells, it is important to find the corresponding structures on TEM images.
Fibrillar vesicles (FVs) are the best candidates for the corresponding structures that represent ALGs under TEM. They are present in many different species of benthic foraminifera that are relatively abundant in various parts of their cytoplasm (Angell, 1967; Hottinger, 2006; LeKieffre et al., 2018a; Jauffrais et al., 2018; Koho et al., 2018). Their size (up to ∼1 µm in diameter) and vesicular, globular shape (LeKieffre et al., 2018a; Goldstein and Richardson, 2018) both correspond to ALGs (Figs. 2–3, 5–6, S3, and S4–S5 in the Supplement). Fibrillar vesicles appear to be separated from the cytosol by a lipid membrane (Figs. 7a, 8). Membranes enveloping the fibrillar vesicles may not cover the entire vesicle. It may form characteristic open vase-shaped structures (Goldstein and Richardson, 2018).
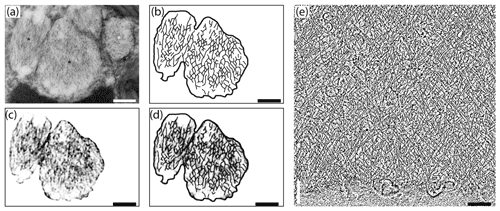
Figure 7Comparison of internal nanostructure of fibrillar vesicles (a–d) to actin meshwork (e) (scale bar 200 nm). (a) TEM image of a fibrillar vesicle, reprinted from LeKieffre et al. (2018a), with permission from Elsevier (their Fig. 14). (b) Model of geometry of fibrillary structures inside fibrillar vesicle based on panel (a). (c) The first step in drawing the model shown in (b). A fragment of panel (a) with the background removed and processed in FIJI software in order to make the geometry more apparent. (d) Overlay of panel (c) with a sketch of the internal structure of FB drawn in CorelDraw. (e) The structure of actin meshwork in lamellipodia based on a nano-tomograph reprinted from Mueller et al. (2017), with permission from Elsevier (their Fig. 4b, modified).
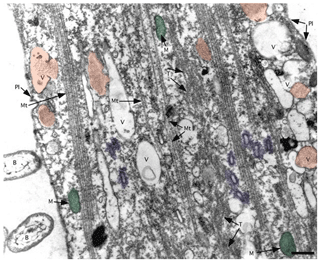
Figure 8TEM image of ectoplasm of Assilina ammonoides (Gronovius) (modified from Hottinger, 2006, their Fig. 67, based on a Creative Commons Attribution 2.5 License) Areas marked in red indicate vesicles that we interpret as fibrillar vesicles. Areas marked in violet indicate what we interpret as fuzzy-coated vesicles, also known as motility-organising vesicles (MOVs). Green areas correspond to mitochondria. B: bacteria; M: mitochondria; Mt: microtubule; Pl: plasmalemma; T: tubulin paracrystals; V: vacuoles with or without fibrillar content (scale bar 500 nm).
Although the chemical composition of FVs is uncertain we can assume from their ability to accumulate nitrogen (LeKieffre et al., 2018b) that they likely contain proteins. Internal material within FVs appears to have a specific 3-D net-like nanostructure. Most fibres are oriented along the long axis of the FVs, but they are not perfectly parallel. They form a network of cross-linked and branching fibres, spreading in two dominant directions and forming recurrent angles. This organisation pattern resembles the actin meshwork observed by cryoelectron tomography in Dictyostelium (Medalia et al., 2002) or in nano-tomography of lamellipodium in keratocyte of zebrafish (Mueller et al., 2017). The similarity in the spatial pattern of fibres inside FVs to the actin meshwork leads to the conclusion that FVs contain a network of actin filaments (Fig. 7). Similar but less organised structures of cross-linked fibres form an actin meshwork in the pseudopods of Allogromia (Bowser et al., 1988; Koury et al., 1985).
It is not clear how FVs are formed. LeKieffre et al. (2018a) proposed that they are produced similar to the model of forming of Golgi vesicles published by Anderson and Lee (1991). This model assumes that they originate in the trans-surface of Golgi apparatus, thus translation of the protein inside those vesicles must occur in the endoplasmic reticulum. This seems to be inconsistent with our hypothesis that fibrillar material consists of prefabricated actin filaments, as actin is a cytoplasmic protein, thus its translation takes place on ribosomes in the cytosol and not in the endoplasmic reticulum (ER). However, assuming that FVs are formed by enclosing fibrillar material produced in the cytosol by the cisternae of Golgi apparatus may resolve this issue. This assumption agrees with findings by Goldstein and Richardson (2018) that the membrane may not cover the entire vesicle.
It is worthwhile to mention that Anderson and Bé (1976) described, in planktic foraminifera, another subcellular structure called the fibrillar system or the fibrillary bodies (according to Hemleben et al., 1989; Schiebel and Hemleben, 2017). Spero (1988) presented this system, which contained proteins involved in construction of a protective envelope during chamber formation in Orbulina universa. However, it is not clear whether these structures represent structurally and functionally analogous organelles to FVs. Spero (1988; their Figs. 4e, f, 5d) documented vesicles using TEM that resemble FVs and are associated with the “primary organic membrane” during chamber formation. In fact, “fibrillar” as a descriptive term seems to describe different filamentous structures at different spatial scales. Fibrillar vesicles show a fibrillar internal ultrastructure, in contrast to the fibrillar system that represents “massive fibrous deposits” constructed from individual tubular structures called fibrillar units (see Spero, 1988). Therefore, the fibrillar system is often tubular, which contrasts with the granular (vesicular) appearance of FVs and ALGs. Nevertheless, Hemleben et al. (1989) note that fibrillar bodies originate in the cytoplasm inside the test as small vacuoles filled with densely packed fibrous material and that they typically enlarge and expand as they are transferred outside the test. However, the rhizopoda of Orbulina universa may contain small vacuoles resembling FVs, e.g. objects described as a vesicle containing an adhesive substance in Fig. 3.5(6) in Schiebel and Hemleben (2017). More comparative studies are needed to reveal whether FVs in benthic species are homologous to the fibrillar system in planktic ones.
Finally, elliptical fuzzy-coated vesicles are additional ultrastructural cellular components that may correspond to ALGs. These vesicles are structures unique to foraminifera. They include elongated structures that are typically approximately 300 nm in length identified in TEM images of reticulopodia (Koury et al., 1985; Travis and Bowser, 1991). Elliptical fuzzy-coated vesicles consist of a membrane coated with an unknown material that has a characteristic fibrillar appearance. They are reported to be involved in regulation of motility, thus the term motility-organising vesicles was coined to describe those structures (Travis and Bowser, 1991). The material coating these organelles has a characteristic fuzzy appearance that might resemble actin mesh.
4.4 Functional implications, evolutionary consequences, and future research prospects
SiR-actin-labelled granules (ALGs) are highly dynamic structures that are abundant in foraminiferal ectoplasm (Figs. 1–3, 5–6). They are small organelles involved in the physiology of granuloreticulopodia and other types of pseudopods, some of them directly involved in morphogenesis of new chambers and biomineralisation of the wall (see Tyszka et al., 2019). As they are ubiquitous in the cells of many species of both globothalamean and tubothalamean foraminifera (sensu Pawlowski et al., 2013), they have most likely evolved very early during evolution of foraminifera. It seems very likely that they correspond to fibrillar vesicles or fuzzy-coated vesicles observed in much higher resolution using TEM (Fig. 8). More studies are needed to corroborate or refute this hypothesis, particularly applying correlative light and electron microscopy as a crucial method to solve this puzzle.
The second question that should to be addressed relates to the presence of analogue structures in other eukaryotic organisms. Indeed, in some fungi or fungi-like protists similar actin structures have been identified in several previous studies (Geitmann and Emons, 2000; Meijer et al., 2014; Moseley and Goode, 2006; Rodal et al., 2005; Waddle et al., 1996; Winter et al., 1997). It is too early to state whether all these structures serve the same physiological function and share the same evolutionary origin. However, there are some facts suggesting that this actually may be the case. Firstly, all of them have a similar size and tend to be concentrated in a cortical layer of protoplasm just under the plasma membrane. Moreover, all the cells containing them have the ability to rapidly expand the volume of protoplasm and actin networks and patches, which may be involved in generating the force needed in this process. Investigation of the molecular basis of actin cytoskeleton regulation in broad phylogenetic context is required to address this issue.
Our working hypothesis is that ALGs most likely play a crucial role in intracellular transport that may be two-fold: (1) they may be involved in transport of various cargo inward (endocytosis) or outward (exocytosis), and/or (2) they may facilitate transfer of prefabricated actin filaments from endoplasm to the external parts of the foraminiferal cell. If the second hypothesis is correct, ALGs are fundamental for extension and adhesion of reticulopodia, as well as the formation and shaping of the globopodium during chamber formation.
Our hypothesis may solve the puzzle of efficient transport of proteins within extensive pseudopodial networks. In foraminifera, ribosomes are absent in the pseudopodial cytoplasm (Travis and Bowser, 1991), and as a consequence protein synthesis is restricted to the endoplasm. Therefore, foraminifera must have mechanisms to efficiently transport proteins needed for the formation of extensive pseudopodial networks. This issue applies primarily to the transportation of the cytoskeletal proteins that are in high demand within reticulopodia, due to their critical role in morphogenesis and movement of this network. Simple diffusion of monomers of tubulin and assembly of microtubules on site may not be sufficient (Bowser and Travis, 2002). Hence, it was proposed that foraminiferal cells use tubulin paracrystals as a storage of prefabricated MT (Travis and Bowser, 1991). Here, we suggest an analogous mechanism for efficient actin transport in form of microfilaments. This mode of transport facilitates a rapid formation, restructuring, and retraction of actin meshwork.
Such functional mechanisms employed for optimisation of intracellular motility of building blocks, pseudopodial dynamics and their overall morphogenesis may be one of the main evolutionary adaptations specific to foraminifera and possibly to related phylogenetic taxa included in the phylum Retaria (see Cavalier-Smith et al., 2018). Similar granuloreticulopodial organisation of pseudopods is known from Radiolaria (Anderson, 1976, 2012). Radiolaria, also called Radiozoa, are very likely a sister group of foraminifera (Burki et al., 2010; Cavalier-Smith et al., 2018; Ruggiero et al., 2015). It is not clear if all types of granules in ectoplasm of Radiozoa and foraminifera are the same. It has been reported that granules in radiolarian pseudopodia include mitochondria, digestive and defecation vacuoles, and osmophilic granules (Anderson, 2012).
Molecular phylogeny based on conservative actin gene sequences suggests that actin in foraminifera evolved at higher rates than in most other eukaryotes (Keeling, 2001). Moreover, duplication of gene-encoding actin occurred early in the evolution of a lineage containing foraminifera, resulting in the presence of two paralogs of that gene in many species (Flakowski et al., 2005). There is some evidence that this duplication is shared by the group Acantharea belonging to Radiolaria (Burki et al., 2010). However, in at least some foraminifera, actin genes have been duplicated many times, forming extraordinarily diverse gene families as is the case for Reticulomyxa filosa. It has been suggested that the diversification of actin genes was a key step in evolution of mechanisms of rapid transport between reticulopodia and the cell body (Glöckner et al., 2014). This suggests that physiology, dynamics, organisation and function of the actin cytoskeleton in foraminifera may differ significantly from most other organisms. More studies are essential for the understanding of the physiological functions of the actin cytoskeleton, including the following examples:
-
research regarding the expression of actin,
-
identification of actin-binding proteins in foraminifera,
-
experiments on inhibition of actin formation during different behaviour (feeding, chamber formation, locomotion, etc.),
-
imaging of actin structures with more refining methods including correlative light- and electron microscopy or super-resolution confocal microscopy.
This paper presents results of live fluorescent labelling of actin in foraminifera with a focus on ectoplasmic (pseudopodial) structures during various behavioural and physiological activities. Fluorescence labelling has revealed three considerably different SiR-actin-labelled patterns, which include (1) weak but non-uniform staining following all membranous surfaces of pseudopodial structures (Figs. 1, 2); (2) linear or ring-like structures showing intense fluorescence (Fig. 1); and (3) small, strongly labelled granular structures that often exhibit very rapid dynamics (Figs. 2–3, 5–6, S4–S5, and Movies S2–S3).
A granular appearance is the principal characteristic of actin cytoskeleton in all studied foraminiferal taxa. SiR-actin-labelled granules (ALGs) have been described as small (ca. 1 µm in diameter), oval, and dynamic objects that are numerous in pseudopodia but present in endoplasm as well. Besides ALGs, the actin cytoskeleton in foraminiferal pseudopodia may form linear and ring-like structures (Fig. 1).
Co-labelling of mitochondria with MitoTracker™ Green and actin cytoskeleton with SiR-actin has been performed in order to verify whether ALGs overlap with mitochondria as a test for the selectivity of granule labelling. As the presented images (Figs. 3, S2–S3 in the Supplement) indicate, there is very little co-localisation between those two types of organelles, however, ALGs and mitochondria probably constitute the majority of granules present in pseudopodia.
A detailed interpretation of the images is given, including the risk that ALGs may be a result of unspecific labelling. The presented arguments allow us to exclude this possibility. Furthermore, the relation of ALGs to similar structures found in other eukaryotes (mostly some fungi and fungi-like protists) has been discussed. It has been proposed that a main function of ALGs in physiology of foraminifera is facilitating transportation of different types of cargo, most likely including transport of prefabricated and/or recycled actin filaments themselves. Finally, the question regarding the correspondence of ALGs to objects known from published TEM images has been addressed. According to our presented hypothesis, most of ALGs correspond to fibrillar vesicles (see LeKieffre et al., 2018a; Goldstein and Richardson, 2018) and/or elliptical fuzzy-coated vesicles (Travis and Bowser, 1991). This is still a working hypothesis that should be verified by correlative TEM-fluorescence methods.
All data supporting the results of this article are included within the article an its Supplement and Video Supplement.
Video data can be accessed via the TIB AV-portal at https://doi.org/10.5446/45179 (Goleń et al., 2020a; Movie S1), https://doi.org/10.5446/45180 (Goleń et al., 2020b; Movie S2), https://doi.org/10.5446/45181 (Goleń et al., 2020c; Movie S3), and https://doi.org/10.5446/45182 (Goleń et al., 2020d; Movie S4).
The Supplement contains eight additional figures showing different actin structures in foraminifera, as well as six additional tables. The supplement related to this article is available online at: https://doi.org/10.5194/bg-17-995-2020-supplement.
JG designed and performed the research, analysed the data, cultured the foraminifera, wrote the paper, and prepared the graphics. JT proposed and supervised the research. UB and JB provided access and infrastructure at AWI. JT consulted interpretations. JT, UB, and JB corrected the text.
The authors declare that they have no conflict of interest.
The authors thank Joan M. Bernhard (WHOI) and Jeffrey Travis (SUNY) for their valuable comments on methodological aspects of fluorescent labelling and Karina Kaczmarek, Karolina Kobos, Anna Spadło, and Anna Wójtowicz for their help with culturing. We are also grateful to Max Janse from Burgers' Zoo in Arnhem and Jakub Kordas form Zoo Wrocław for providing us with samples of living foraminifera. The authors gratefully acknowledge Samuel Bowser, Takashi Toyofuku, and the anonymous referee for their constructive reviews that helped us improve our paper. Jan Goleń and Jarosław Tyszka received support from the Polish National Science Centre (UMO-2015/19/B/ST10/01944).
This research has been supported by the Polish National Science Centre (grant no. UMO-2015/19/B/ST10/01944).
This paper was edited by Hiroshi Kitazato and reviewed by Samuel Bowser, Takashi Toyofuku, and one anonymous referee.
Anderson, O. R.: Ultrastructure of a colonial radiolarian Collozoum inerme and a cytochemical determination of the role of its zooxanthellae, Tissue Cell, 8, 195–208, https://doi.org/10.1016/0040-8166(76)90046-X, 1976.
Anderson, O. R.: Radiolaria, Springer Science & Business Media, New York, Berlin, Heidelberg, Tokyo, 2012.
Anderson, O. R. and Bé, A. W.: The ultrastructure of a planktonic foraminifer, Globigerinoides sacculifer (Brady), and its symbiotic dinoflagellates, J. Foramin. Res., 6, 1–21, https://doi.org/10.2113/gsjfr.6.1.1, 1976.
Anderson, O. R. and Lee, J. J. (Eds.): Cytology and fine structure, in: Biology of Foraminifera, edited by: Lee, J. J. and Andreson, R., Academic Press (Harcourt Brace Jovanvich), London, San Diego, New York, Boston, Sydney, Tokyo, 7–40, 1991.
Angell, R. W.: The test structure and composition of the foraminifer Rosalina floridana, J. Protozool., 14, 299–307, https://doi.org/10.1111/j.1550-7408.1967.tb02001.x, 1967.
Boltovskoy, E. and Wright, R.: The systematic position and importance of the Foraminifera, in: Recent Foraminifera, edited by: Boltovskoy, E. and Wright, R., Springer, Dordrecht, the Netherlands, 5–21, 1976.
Bowser, S. S. and Travis, J. L.: Methods for structural studies of reticulopodia, the vital foraminiferal “soft part”, Micropaleontology, 46, 47–56, 2000.
Bowser, S. S. and Travis, J. L.: Reticulopodia: structural and behavioral basis for the suprageneric placement of granuloreticulosan protists, J. Foramin. Res., 32, 440–447, https://doi.org/10.2113/0320440, 2002.
Bowser, S. S., Travis, J. L., and Rieder, C. L.: Microtubules associate with actin-containing filaments at discrete sites along the ventral surface of Allogromia reticulopods, J. Cell Sci., 89, 297–307, 1988.
Burki, F., Kudryavtsev, A., Matz, M. V., Aglyamova, G. V., Bulman, S., Fiers, M., Keeling, P. J., and Pawlowski, J.: Evolution of Rhizaria: new insights from phylogenomic analysis of uncultivated protists, BMC Evol. Biol., 10, 377, https://doi.org/10.1186/1471-2148-10-377, 2010.
Cavalier-Smith, T., Chao, E. E., and Lewis, R.: Multigene phylogeny and cell evolution of chromist infrakingdom Rhizaria: contrasting cell organisation of sister phyla Cercozoa and Retaria, Protoplasma, 255, 1517–1574, https://doi.org/10.1007/s00709-018-1241-1, 2018.
de Nooijer, L. J., Langer, G., Nehrke, G., and Bijma, J.: Physiological controls on seawater uptake and calcification in the benthic foraminifer Ammonia tepida, Biogeosciences, 6, 2669–2675, https://doi.org/10.5194/bg-6-2669-2009, 2009.
Ernst, S., Janse, M., Renema, W., Kouwenhoven, T., Goudeau, M. L., and Reichart, G. J.: Benthic foraminifera in a large Indo-Pacific coral reef aquarium, J. Foramin. Res., 41, 101–113, https://doi.org/10.2113/gsjfr.41.2.101, 2011.
Flakowski, J., Bolivar, I., Fahrni, J., and Pawlowski, J.: Actin phylogeny of foraminifera, J. Foramin. Res., 35, 93–102, https://doi.org/10.2113/35.2.93, 2005.
Frontalini, F., Losada, M. T., Toyofuku, T., Tyszka, J., Goleń, J., de Nooijer, L., Canonico B., Cesarini, E., Nagai, Y., Bickmeyer, U., Ikuta, T., Tsubaki, R., Besteiro Rodriguez C., Al-Enazi, E., Papa, S., Coccioni, R., Bijma, J., and Bernhard, J. M.: Foraminiferal ultrastructure: a perspective from fluorescent and fluorogenic probes, J. Geophys. Res.-Biogeo., 124, 2823–2850, https://doi.org/10.1029/2019JG005113, 2019.
Geitmann, A. and Emons, A. M. C.: The cytoskeleton in plant and fungal cell tip growth, J. Microsc., 198, 218–245, https://doi.org/10.1046/j.1365-2818.2000.00702.x, 2000.
Glöckner, G., Hülsmann, N., Schleicher, M., Noegel, A. A., Echinger, L., Gallinger, C., Pawlowski, J., Sierra, R., Euteneuer, U., Pillet, L., Moustafa, A., Platzer, M., Groth, M., Szafanski, K., and Schliwa, M.: The genome of the foraminiferan Reticulomyxa filose, Curr. Biol., 24, 11–18, https://doi.org/10.1016/j.cub.2013.11.027, 2014.
Goleń, J., Tyszka, J., Bickmeyer, U., and Bijma, J.: Actin cytoskeleton in lamellipodia of living Ammonia sp. (Foraminifera), TIB, https://doi.org/10.5446/45179, 2020a.
Goleń, J., Tyszka, J., Bickmeyer, U., and Bijma, J.: Actin cytoskeleton and mitochondria in a newly formed chamber in Amphistegina lessonii, TIB, https://doi.org/10.5446/45180, 2020b.
Goleń, J., Tyszka, J., Bickmeyer, U., and Bijma, J.: Actin cytoskeleton in a thick pseudopodial thread of living Amphistegina lessonii (Foraminifera), TIB, https://doi.org/10.5446/45181, 2020c.
Goleń, J., Tyszka, J., Bickmeyer, U., and Bijma, J.: Measurement of velocity of SiR-actin-labelled granules (ALGs) in pseudopodia of Amphistegina lessonii (Foraminifera), TIB, https://doi.org/10.5446/45182, 2020d.
Goldstein, S. T.: Foraminifera: a biological overview, in: Modern foraminifera, edited by: Gupta, B. K. S., Springer, Dordrecht, the Netherlands, 37–55, https://doi.org/10.1007/0-306-48104-9_3, 1999.
Goldstein, S. T. and Richardson, E. A.: Fine structure of the foraminifer Haynesina germanica (Ehrenberg) and its sequestered chloroplasts, Mar. Micropaleontol., 138, 63–71, https://doi.org/10.1016/j.marmicro.2017.10.010, 2018.
Hemleben, C., Spindler, M., and Anderson, O. R.: Modern planktonic foraminifera, Springer-Verlag, New York, Berlin, Heidelberg, London, Paris, Tokyo, 1989.
Hottinger, L.: Illustrated glossary of terms used in foraminiferal research, Carnets Geol., 2006, 1–126, https://doi.org/10.4267/2042/5832, 2006.
Jahn, T. L. and Rinaldi, R. A.: Protoplasmic movement in the foraminiferan, Allogromia laticollaris; and a theory of its mechanism, Biol. Bull., 117, 100–118, 1959.
Jauffrais, T., LeKieffre, C., Koho, K. A., Tsuchiya, M., Schweizer, M., Bernhard, J. M., Meibom, A., and Geslin, E.: Ultrastructure and distribution of kleptoplasts in benthic foraminifera from shallow-water (photic) habitats, Mar. Micropaleontol., 138, 46–62, https://doi.org/10.1016/j.marmicro.2017.10.003, 2018.
Keeling, P. J.: Foraminifera and Cercozoa are related in actin phylogeny: Two Orphans find a home?, Mol. Biol. Evol., 18, 1551–1557, https://doi.org/10.1093/oxfordjournals.molbev.a003941, 2001.
Kitazato, H.: Locomotion of some benthic foraminifera in and on sediments, J. Foramin. Res., 18, 344–349, https://doi.org/10.2113/gsjfr.18.4.344, 1988.
Koho, K. A., LeKieffre, C., Nomaki, H., Salonen, I., Geslin, E., Mabilleau, G., Søgaard Jensen, L. H., and Reichart, G.-J.: Changes in ultrastructural features of the foraminifera Ammonia spp. In response to anoxic conditions: Field and laboratory observations, Mar. Micropaleontol., 138, 72–82, https://doi.org/10.1016/j.marmicro.2017.10.011, 2018.
Koonce, M. P., Euteneuer, U., McDonald, K. L., Menzel, D., and Schliwa, M.: Cytoskeletal architecture and motility in a giant freshwater amoeba, Reticulomyxa, Cell Motil. Cytoskel., 6, 521–533, https://doi.org/10.1002/cm.970060511, 1986a.
Koonce, M. P., Euteneuer, U., and Schliwa, M.: Reticulomyxa: a new model system of intracellular transport, J. Cell Sci., 5, 145–159, 1986b.
Koury, S. T., Bowser S. S., and McGee-Russell, S. M.: Ultrastructural changes during reticulopod withdrawal in the foraminiferan protozoan Allogromia sp., strain NF, Protoplasma, 129, 149–156, 1985.
Lang, W.: Nomarski differential interference-contrast microscopy, Zeiss Inf., 70, 114–120, 1968.
LeKieffre, C., Bernhard, J. M., Mabilleau, G., Filipsson, H. L., Meibom, A., and Geslin, E.: An overview of cellular ultrastructure in benthic foraminifera: New observations of rotalid species in the context of existing literature, Mar. Micropaleontol., 138, 12–32, https://doi.org/10.1016/j.marmicro.2017.10.005, 2018a.
LeKieffre, C., Jauffrais, T., Geslin, E., Jesus, B., Bernhard, J. M., Giovani, M. E., and Meibom, A.: Inorganic carbon and nitrogen assimilation in cellular compartments of a benthic kleptoplastic foraminifer, Sci. Rep.-UK, 8, 10140, https://doi.org/10.1038/s41598-018-28455-1, 2018b.
Lemieux, M. G., Janzen, D., Hwang, R., Roldan, J., Jarchum, I., and Knecht, D. A.: Visualization of the actin cytoskeleton: different F-actin-binding probes tell different stories, Cytoskeleton, 71, 157–169, https://doi.org/10.1002/cm.21160, 2014.
Lipps, J. H., Finger, K. L., and Walker, S. E.: What should we call the Foraminifera?, J. Foramin. Res., 41, 309–313, https://doi.org/10.2113/gsjfr.41.4.309, 2011.
Lukinavičius, G., Reymond, L., D'Este, E., Masharina, A., Göttfert, F., Ta, H., Güther, A., Fournier, M., Rizzo, S., Waldmann, H., Blaukopf, C., Sommer, C., Gerlich, D. W., Arndt, H.-D., Hell, S. W., and Johnsson, K.: Fluorogenic probes for live-cell imaging of the cytoskeleton, Nat. Methods, 11, 731–733, https://doi.org/10.1038/nmeth.2972, 2014.
Meijer, H. J. G., Hua, C., Kots, K., Ketelaar, T., and Govers, F.: Actin dynamics in Phytophthora infestans; rapidly reorganizing cables and immobile, long-lived plaques, Cell. Microbiol., 16, 948–961, https://doi.org/10.1111/cmi.12254, 2014.
Medalia, O., Weber, I., Frangakis, A. S., Nicastro, D., Gerisch, G., and Baumeister, W.: Macromolecular architecture in eukaryotic cells visualized by cryoelectron tomography, Science, 298, 1209–1213, https://doi.org/10.1126/science.1076184, 2002.
Melak, M., Matthias, P., and Robert, G.: Actin visualization at a glance, J. Cell Sci., 130, 525–530, https://doi.org/10.1242/jcs.189068, 2017.
Mogilner, A. and Oster, G.: Force generation by actin polymerization II: the elastic ratchet and tethered filaments, Biophys. J., 84, 1591–1605, https://doi.org/10.1016/S0006-3495(03)74969-8, 2003.
Moseley, J. B. and Goode, B. L.: The yeast actin cytoskeleton: from cellular function to biochemical mechanism, Microbiol. Mol. Biol. R., 70, 605–645, https://doi.org/10.1128/MMBR.00013-06, 2006.
Mueller, J., Szep, G., Nemethova, M., de Vries, I., Lieber, A. D., Winkler, C., Kruse, K., Small, J. V., Schmeiser, C., Keren, K., Hauschild, R., and Sixt, M.: Load adaptation of lamellipodial actin networks, Cell, 171, 188–200, https://doi.org/10.1016/j.cell.2017.07.051, 2017.
Nomaki, H., Bernhard, J. M., Ishida, A., Tsuchiya, M., Uematsu, K., Tame, A., Kitahashi, T., Takahata, N., Sano, Y., and Toyofuku, T.: Intracellular isotope localization in Ammonia sp. (Foraminifera) of oxygen-depleted environments: Results of nitrate and sulfate labeling experiments, Aquat. Microbiol., 163, https://doi.org/10.3389/fmicb.2016.00163, 2016.
Ohno, Y., Fujita, K., Toyofuku, T., and Nakamaura, T.: Cytological observations of the large symbiotic foraminifer Amphisorus kudakajimensis using calcein acetoxymethyl ester, PloS one, 11, e0165844, https://doi.org/10.1371/journal.pone.0165844, 2016.
Pawlowski, J., Holzmann, M., Berney, C., Fahrni, J., Gooday, A. J., Cedhagen, T., Habura, A., and Bowser, S. S.: The evolution of early Foraminifera, P. Natl. Acad. Sci. USA, 100, 11494–11498, https://doi.org/10.1073/pnas.2035132100, 2003.
Pawlowski, J., Holzmann, M., and Tyszka, J.: New supraordinal classification of Foraminifera: Molecules meet morphology, Mar. Micropaleontol., 100, 1–10, https://doi.org/10.1016/j.marmicro.2013.04.002, 2013.
Presley, A. D., Fuller, K. M., and Arriaga, E. A.: MitoTracker Green labeling of mitochondrial proteins and their subsequent analysis by capillary electrophoresis with laser-induced fluorescence detection, J. Chromatogr. B, 793, 141–150, https://doi.org/10.1016/S1570-0232(03)00371-4, 2003.
Rodal, A. A., Kozubowski, L., Goode, B. L., Drubin, D. G., and Hartwig, J. H.: Actin and septin ultrastructures at the budding yeast cell cortex, Mol. Biol. Cell, 16, 372–384, https://doi.org/10.1091/mbc.E04-08-0734, 2005.
Ruggiero, M. A., Gordon, D. P., Orrell, T. M., Bailly, N., Bourgoin, T., Brusca, R. C., Cavalier-Smith, T., Guiry, M. D., and Kirk, P. M.: A higher level classification of all living organisms, PloS one, 10, e0119248, https://doi.org/10.1371/journal.pone.0119248, 2015.
Schiebel, R. and Hemleben, C.: Planktic foraminifers in the modern ocean, Springer, Berlin, 2017.
Schindelin, J., Arganda-Carreras, I., Frise, E., Kaynig, V., Longair, M., Pietzsch, T., Preibisch, S., Rueden, C., Saalfeld, S., Schmid, B., Tinevez, J. Y., White, D. J., Hartenstein, V., Eliceiri, K., and Tomancak, P.: Fiji: an open-source platform for biological-image analysis, Nat. Methods, 9, 676–682, https://doi.org/10.1038/nmeth.2019, 2012.
Spero, H. J.: Ultrastructural examination of chamber morphogenesis and biomineralization in the planktonic foraminifer Orbulina universa, Mar. Biol., 99, 9–20, https://doi.org/10.1007/BF00644972, 1988.
Tinevez, J.-Y., Perry, N., Schindelin, J., Hoopes, G. M., Reynolds, G. D., Laplantine, E., Bednarek, S. Y., Shorte, S. L., and Eliceiri, K. W.: TrackMate: An open and extensible platform for single-particle tracking, Methods, 115, 80–90, https://doi.org/10.1016/j.ymeth.2016.09.016, 2017.
Toyofuku, T., Jan de Nooijer, L., Yamamoto, H., and Kitazato, H.: Real-time visualization of calcium ion activity in shallow benthic foraminiferal cells using the fluorescent indicator Fluo-3 AM, Geochem. Geophy. Geosy., 9, 1525–2027, https://doi.org/10.1029/2007GC001772, 2008.
Travis, J. L. and Bowser, S. S.: A new model of reticulopodial motility and shape: Evidence for a microtubule-based motor and an actin skeleton, Cytoskeleton, 6, 2–14, https://doi.org/10.1002/cm.970060103, 1986.
Travis, J. L. and Bowser, S. S.: The motility of foraminifera, in: Biology of Foraminifera, edited by: Lee, J. J. and Anderson, O. R., Academic Press (Harcourt Brace Jovanvich), London, San Diego, New York, Boston, Sydney, Tokyo, Toronto, 90–155, 1991.
Travis, J. L., Kenealy, J. F., and Allen, R. D.: Studies on the motility of the Foraminifera, J. Cell Biol., 97, 1668–1676, https://doi.org/10.1083/jcb.97.6.1668, 1983.
Tyszka, J., Bickmeyer, U., Raitzsch, M., Bijma, J., Kaczmarek, K., Mewes, A., Topa, P., and Janse, M.: Form and function of F-actin during biomineralization: Live experiments on Foraminifera, P. Natl. Acad. Sci. USA, 116, 4111–4116, https://doi.org/10.1073/pnas.1810394116, 2019.
Waddle, J. A., Karpova, T. S., Waterston, R. H., and Cooper, J. A.: Movement of cortical actin patches in yeast, J. Cell Biol., 132, 861–870, 1996.
Weigel, A., Schild, D., and Zeug, A.: Resolution in the ApoTome and the confocal laser scanning microscope: comparison, J. Biomed. Opt., 14, 014022, https://doi.org/10.1117/1.3083439, 2009.
Winter, D., Podtelejnikov, A. V., Mann, M., and Li, R.: The complex containing actin-related proteins Arp2 and Arp3 is required for the motility and integrity of yeast actin patches, Curr. Biol., 7, 519–529, 1997.