the Creative Commons Attribution 4.0 License.
the Creative Commons Attribution 4.0 License.
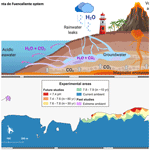
Chemical characterization of the Punta de Fuencaliente CO2-enriched system (La Palma, NE Atlantic Ocean): a new natural laboratory for ocean acidification studies
Sara González-Delgado
David González-Santana
Magdalena Santana-Casiano
Melchor González-Dávila
Celso A. Hernández
Carlos Sangil
José Carlos Hernández
We present a new natural carbon dioxide (CO2) system located off the southern coast of the island of La Palma (Canary Islands, Spain). Like CO2 seeps, these CO2 submarine groundwater discharges (SGDs) can be used as an analogue to study the effects of ocean acidification (OA) on the marine realm. With this aim, we present the chemical characterization of the area, describing the carbon system dynamics, by measuring pH, AT and CT and calculating Ω aragonite and calcite. Our explorations of the area have found several emission points with similar chemical features. Here, the CT varies from 2120.10 to 10 784.84 µmol kg−1, AT from 2415.20 to 10 817.12 µmol kg−1, pH from 7.12 to 8.07, Ω aragonite from 0.71 to 4.15 and Ω calcite from 1.09 to 6.49 units. Also, the CO2 emission flux varies between 2.8 and 28 kg CO2 d−1, becoming a significant source of carbon. These CO2 emissions, which are of volcanic origin, acidify the brackish groundwater that is discharged to the coast and alter the local seawater chemistry. Although this kind of acidified system is not a perfect image of future oceans, this area of La Palma is an exceptional spot to perform studies aimed at understanding the effect of different levels of OA on the functioning of marine ecosystems. These studies can then be used to comprehend how life has persisted through past eras, with higher atmospheric CO2, or to predict the consequences of present fossil fuel usage on the marine ecosystem of the future oceans.
Please read the corrigendum first before continuing.
-
Notice on corrigendum
The requested paper has a corresponding corrigendum published. Please read the corrigendum first before downloading the article.
-
Article
(5482 KB)
- Corrigendum
-
Supplement
(3093 KB)
-
The requested paper has a corresponding corrigendum published. Please read the corrigendum first before downloading the article.
- Article
(5482 KB) - Full-text XML
- Corrigendum
-
Supplement
(3093 KB) - BibTeX
- EndNote
For the last decade, marine systems with natural carbon dioxide (CO2) sources have been used as analogous of the acidified future oceans to understand CO2 effects on organisms and marine ecosystems functioning (IPCC, 2014; Hall-Spencer et al., 2008; Foo et al., 2018; González-Delgado and Hernández, 2018). These areas are characterized by an extra CO2 input from volcanic (normally called CO2 seeps), karstic or biological sources, or they originate from upwelling (González-Delgado and Hernández, 2018). Due to the origin of CO2, CO2 vent systems are very common and can be found all over the world from mid-oceanic ridges to oceanic islands and intra-plate magmatic areas (Dando et al., 1999; Tarasov et al., 2005). In general, the vent systems have emissions in the form of bubbles which are 90 %–99 % CO2. The most notable features of these acidified systems are the fluctuation in pH, the aragonite and calcite saturation states (Ω) (declining between 1 and 3), and dissolved inorganic carbon (DIC) which increases up to 3.2 mol C m−3 (González-Delgado and Hernández, 2018).
Moreover, there are marine shallow areas affected by CO2 gas diffusive emissions through submarine groundwater discharges (SGDs) that acidify the surrounding waters (Hall-Spencer et al., 2008).
Numerous advances in ocean acidification (OA) studies have been achieved using these systems, such as in understanding the acidification effect on ecology interaction (e.g. Nagelkerken et al., 2016), physiological adaptations (e.g. Migliaccio et al., 2019) and genetic adaptations (e.g. Olivé et al., 2017). Nowadays, it is possible to better understand the direct and indirect effects of OA in marine environments due to these acidified systems; for instance we now know that OA-related changes will be reflected in the services that ecosystems provide to us (Hall-Spencer and Harvey, 2019). Acidified systems can also be used to look back into the past of the Earth and to study how early life could have originated on the planet (Martin et al., 2008). Understanding how life has adapted in the past acidified eras can be extremely useful to understand how current life will change in the expected future (Gattuso et al., 1998).
The Canary Islands, located in the north-eastern Atlantic Ocean, are an oceanic volcanic archipelago formed by numerous hotspot island chains (Carracedo et al., 2001). The youngest islands are El Hierro at 1.1 million years and La Palma with an age of 2 million years (Carracedo et al., 2001). These islands are located to the west of the archipelago, and they are where the last historical eruptions took place. The last two were the Teneguía volcano on La Palma in 1971 and the Tagoro volcano on El Hierro in 2011 (Padrón et al., 2015; Santana-Casiano et al., 2016).
Currently, in the historic volcanic area on the south of La Palma (Cumbre Vieja volcano complex), there is a continuous degassing of CO2 (Carracedo et al., 2001; Padrón et al., 2015). Correspondingly, on the nearby shore, CO2 emissions have been detected recently in two different locations: the Las Cabras site (Hernandez et al., 2016) and Punta de Fuencaliente, which has already been used for OA ecological studies (Pérez, 2017; Viotti et al., 2019). However, in these works only the pH and pCO2 were measured, at localized points where certain samples were taken.
The local name “Fuencaliente”, which translates as hot springs, refers to the thermal fresh waters that emerge at the coast. Before the conquest of the islands in 1492, its waters were used by locals for their healing properties and after that by visitors from all over the world (Soler-Liceras, 2007). However, these thermal springs were buried by the eruption of the San Antonio volcano in the 17th century. These thermal waters have been so famous and important for Fuencaliente people that there was an engineering project to dig them up (Soler-Liceras, 2007). The brackish water features measured by Soler-Liceras (2007) showed high concentrations of bicarbonate (HCO), sulfate (SO) and chloride (Cl−) that together with high temperatures (almost 50 ∘C) confirmed the influence of internal magmatic activity. Nearby, there are brackish lagoons located in the innermost part of the Echentive beach, about 200 m from the coastline with diameters of 30 m and depths of up to 4 m (Fig. 1). Measures of oxygen isotopes (δ18O SMOW) (Calvet et al., 2003) suggest a slight dilution of the seawater in the lagoons by inland brackish groundwater flowing into them. This indicates that in the system there are groundwater discharges, which probably come from the thermal waters studied by Soler-Liceras (2007).
In the last 2 decades, an increasing number of studies have underlined the importance of SGD (Jeandel, 2016). SGD is an essential but poorly recognized pathway of material transport to the marine environment (Szymczycha et al., 2014). The term SGD includes the discharge of fresh groundwater to coastal seas to which recirculation of seawater often contributes (Burnett et al., 2006; Charette et al., 2016). For issues related to oceanography, the term is restricted to fluid circulation through continental shelf sediments with emphasis on the coastal zone (Burnett et al., 2006; Jeandel, 2016). One aspect that has yet not been considered is what occurs in areas where SGD is enriched by the emissions of recent volcanism or by hydrothermal activity. In these cases, these discharges can also act as sources of gases and hydrothermal emission compounds to the ocean and become points of emission of CO2 that contribute to the OA. However, shallow coastal beaches and intertidal lagoons are highly dynamic systems controlled by physical processes and subjected to marine and continental influences. Processes such as the tide or the submarine groundwater discharges produce higher ranges of variation in physical and chemical parameters than processes in the open ocean water and could provide a natural environment for laboratory studies.
Hence, and with the purpose of using the Punta de Fuencaliente area as a naturally acidified laboratory, an accurate physical and chemical characterization of this area is presented in this study. The main objectives were to (1) determine the area affected by the emissions and detect new emissions points for replication studies, (2) characterize the ocean chemistry of the area, and (3) confirm the volcanic origin of the acidification.
2.1 Study area
The physical–chemical parameters were sampled across the south of La Palma, located in the west of the Canary Islands (north-eastern Atlantic Ocean) (Fig. 1a, File S1 in the Supplement). The sampling took places between a 0 and 2 m depth, at three different times (March 2018, December 2018 and June 2019) and during low and high tide when it was necessary to assess the continuity of the natural emissions (Fig. 1b, Appendix A). Following the previous studies in the area (Hernández et al., 2016; Pérez, 2017; González-Delgado et al., 2018a, b; Hernández et al., 2018; Viotti et al., 2019), a sampling network was created for the first time. It is formed by the following sites: Playa del Faro, Los Porretos and surroundings (that together with the Las Cabras site are known as the Punta de Fuencaliente system or PFS), Playa Echentive and the two Echentive lagoons (Fig. 1c).
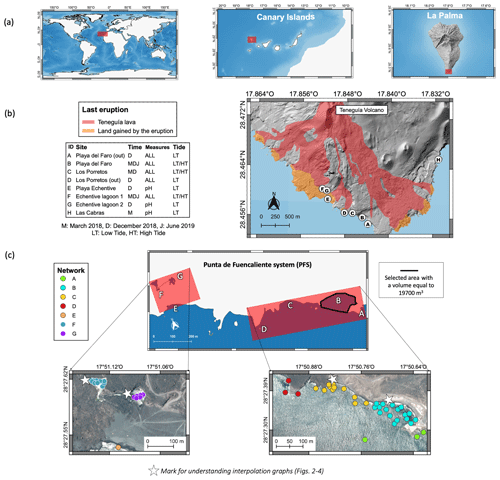
Figure 1(a) Location of study are in north-eastern Atlantic Ocean, in the west of the Canary Islands, on the south of La Palma. (b) Location of the seven sampling sites (A–G) on the south of the island of La Palma. The location of Punta Las Cabras (H) considered in Hernández et al. (2016) is also included. The area covered by the last volcanic eruption, Teneguía volcano, is also indicated. (c) Location of sampling network performed in this work around the Punta de Fuencaliente system (PFS) and the selected area where the volume was calculated for CO2 flux calculation. The stars are included as an aid to better interpret and locate the interpolation graphics from Figs. 2–4. The map and image base layers used are distributed in the public domain (https://www.grafcan.es/, last access: 20 September 2019).
Scuba diving was used for sampling all bottles except in the reference station off Playa del Faro (Fig. 1cA), where a CTD rosette was used. For the scuba sampling, the bottle was previously rinsed three times at the sampling location and then the bottle was immersed with the mouth down and turned at 1 m depth for sampling. Samples were poisoned with 100 µL of saturated HgCl2 solution, sealed, kept in darkness and analysed in the lab. In March 2018, this was performed on the same day, while in December it was performed 2 d later. For pH, 100 mL borosilicate glass bottles were filled with seawater.
2.2 Carbon dioxide system parameters
In March and December 2018, the total dissolved inorganic carbon concentration (CT), total alkalinity (AT), pH, salinity and temperature were measured, whilst in June 2019 only the pH and temperature were measured. Total alkalinity and CT were determined by potentiometric and coulometric methods, respectively, using a VINDTA 3C system (Mintrop et al., 2000). The calibrations were made using certified reference material batch no. 163 (González-Dávila et al., 2007). The pH was measured at a constant temperature of 25 ∘C within 1 h of sampling, using an Orion pH meter with a combined Orion glass electrode (pHT,is). The calibration was performed on the total seawater scale using a Tris artificial seawater buffer (salinity 35) according to the Guide to Best Practices for Ocean CO2 Measurements (Dickson et al., 2007, SOP 6a).
Salinity and temperature were measured in situ using a handheld conductivity meter (Hanna Instruments HI98192). Furthermore, 200 mL salinity bottles were measured in the laboratory within 2 d and using a high-precision Portasal salinometer, accurate to ±0.001. The pH under in situ conditions, the partial pressure of carbon dioxide (pCO2) and the saturation states of calcium carbonate forms (Ω aragonite and Ω calcite) were determined from AT and CT data using the CO2SYS program (Pierrot et al., 2006).
Atmospheric CO2 concentrations used for flux calculations were those measured at the Izaña station on the island of Tenerife (IZO site and available in the World Data Centre for Greenhouse Gases).
We used the linear interpolation method to represent the AT, CT, pHT,is, Ω aragonite and calcite parameter measurement when anomalies were found.
After extensive sampling throughout the south of La Palma, we detected four areas where natural enrichment of CO2 groundwater emissions occurs. These four areas, Las Cabras, Playa del Faro, Los Porretos and the two Echentive lagoons (Fig. 1b, c), correspond to areas that were not buried by the lava during the last eruption (Teneguía volcano, 1971; Padrón et al., 2015; Fig. 1b). The Las Cabras site was discarded in subsequent samplings due to difficult access, the poor sea conditions and the small size of the area affected by the emissions (Hernández et al., 2016). In all cases, the anomalies were the highest during low tide (Appendix B).
3.1 Temperature and salinity
Temperature and salinity on Playa del Faro and Los Porretos do not present major changes between the different time points (File S2). During March 2018, Playa del Faro had an average temperature of 19.00±0.20 ∘C with colder values of 18.70 ∘C near the shore; Los Porretos was not measured at this time. In December 2018, both Playa del Faro and Los Porretos presented an average temperature of 21.50±0.02 ∘C. However, salinity values present a minor diminution from 37.05 to 36.51 on Playa del Faro and from 37.05 to 36.07 on Los Porretos (File S2). Both sites presented colder and slightly less saline water near the coast. Regarding the Echentive lagoons, only the biggest lagoon was measured, where the salinity varied from 31.00 to 32.00 units (File S2). The same lagoons presented warmer temperatures than the coastal waters during June 2019, 26.40±0.70 and 22.00±0.10 ∘C, respectively.
3.2 Carbon dioxide system parameters
In both studied shore areas of the PFS (Playa del Faro and Los Porretos) the parameters of the carbon dioxide system, pHT,is (Fig. 2a, b), AT, CT, and Ω aragonite and calcite (Figs. 3, 4B), were strongly affected by the entrance of the SGD with less salinity.
3.2.1 Playa del Faro
In March 2018, the pH changed from 8.06 in offshore samples to 7.50 near the shore, reaching 7.16 and 7.13 during December 2018 and June 2019, respectively (Fig. 2a). Similarly, high AT and DIC were measured throughout Playa del Faro. In March 2018, the ocean data obtained in the furthest coast station of Playa del Faro reached typical values of 2132.13 and 2418.38 µmol kg−1 for CT and AT, respectively (File S2). As we approached the shore, both factors increased to values that exceeded 3100 µmol kg−1, following an inverse distribution observed with salinity, with an increase in the CT : AT ratio close to 1 : 1, indicating an important contribution of bicarbonate in the area (Fig. 3a, b). In December 2018, the anomaly increased to over 3500 µmol kg−1 for both parameters. As a direct consequence of the low pH values, although compensated for by the high CT, AT and dissolved calcium contents (determined by ICP-MS – inductively coupled plasma mass spectrometry, data not presented), the calcite and aragonite saturation states were also affected. It was observed that the area nearest to the shore presented saturation values of calcite and aragonite that were below 1.50 (Fig. 3c, d).
During high tide, the anomalies almost disappeared, which means that the tide acts as a pressure plug of the flow of this water to the coastal area. Nevertheless, we still found a mild increase in AT and CT (reaching 2692.13 and 2512.35 µmol kg−1, respectively) (Fig. 3a, b) and pH values of 7.75–7.85 in the sampling points closest to the coast (Fig. 2a).
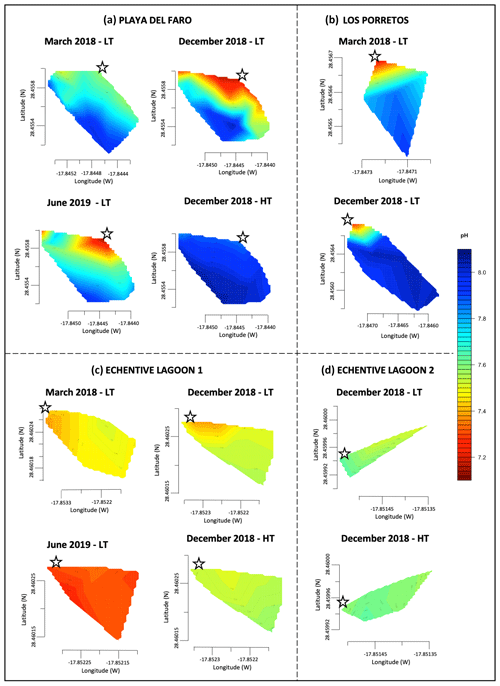
Figure 2Linear interpolation graphs of pH values that were collected in March 2018, December 2018 and June 2019 during low tide (LT) and high tide (HT) on Playa del Faro (a), on Los Porretos (b), in Echentive lagoon 1 (c) and in Echentive lagoon 2 (d). The star symbol is the reference mark on the map in Fig. 1c.
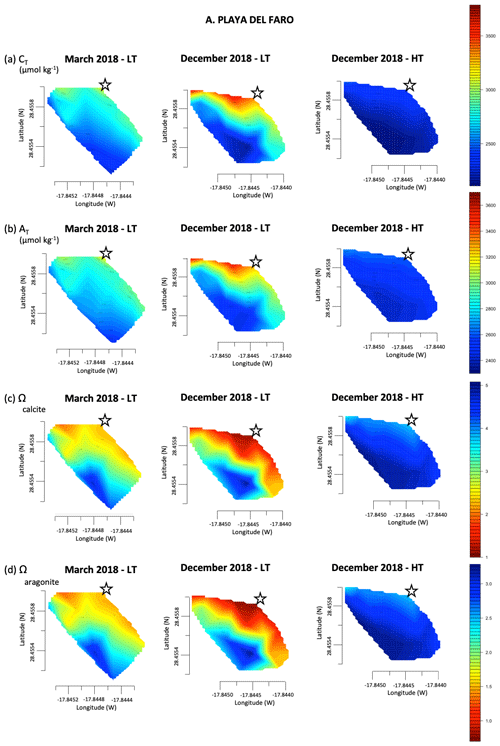
Figure 3Linear interpolation graphs of CT (a), AT (b), Ωcalcite (c) and Ωaragonite (d) values during March 2018 and December 2018 during low tide (LT) and high tide (HT) on Playa del Faro. The star symbol is the reference mark on the map in Fig. 1c.
3.2.2 Los Porretos
Los Porretos is a continuation of Playa del Faro that is also affected by the SGD with high CT and low pH. This discharge was first observed during March 2018. The measured CT exceeded 3400 µmol kg−1, and the pHT,is reached 7.25 at the emission station (Figs. 2b, 4B). In December, the sampling was repeated, observing that the most anomalous values occurred in the stations closest to the coast. The emission point presented CT concentrations of 3456.6 µmol kg−1 (corresponding to carbon dioxide pressure values of 5200 µatm), pH values of 7.27, and 1.45 and 0.95 values of Ω calcite and aragonite, respectively (Figs. 2b, 4B; File S2).
In both beaches, the emission is acting as an important source of CO2 into the atmosphere. On Playa del Faro, the partial pressures of CO2 in surface waters reached up to 5000 µatm at low tide (the values in the atmosphere were between 405 and 410 µatm) (File S2). This produced high concentration gradients that combined with high-intensity winds characteristic of the area and produced CO2 fluxes that can reach up to 1 mol m−2 d−1 (considering its main effects during low tide and Wanninkhof, 2014, for the gas transfer velocity coefficient) that amount to 150 t CO2 yr−1.
3.2.3 Echentive lagoons
The two lagoons at Playa Echentive (Fig. 1c) show the maximum anomalies on the south of La Palma. They presented low salinities and low pH, below 7.5 in all stations and reaching 7.39 in the north-west during March 2018 (data only from the big lagoon) (Fig. 2c, d). Similarly, the CT was above 9700 µmol kg−1, with comparable values for AT (Fig. 4Ca, Cb). These CT and AT concentrations together with the low pH values counteracted the saturation states of calcite and aragonite that were never below 4.35 and 2.79, respectively (Fig. 4Cc, Cd). Furthermore, when both lagoons were sampled during December 2018, similar concentrations were measured at low and high tide (Fig. 2c, d). The north-western part of the big lagoon presented the highest CT concentration (greater than 10 000 µmol kg−1), and the lowest pH reached 7.38 at low tide and 7.55 at high tide, which coincided with a decrease in salinity and a mild temperature increase (Fig. 2c, d; File S2). The rest of the big lagoon remained at pH 7.58, like the small lagoon with a maximum pH of 7.63. However, the small lagoon presented a lower pH range, with a minimum of 7.50 at low tide and a maximum of 7.64 at high tide in the northern part (Fig. 2c, d).
The water levels in both lagoons were tide dependent. The entry of salty marine water during high tide reduced the anomaly caused by the intrusion of lower-salinity water rich in CT and AT.
3.3 CO2 flux calculation
The CO2 flux was calculated for Playa del Faro. We assumed two endmembers, the open ocean endmember and the SGD endmember. Soler-Liceras (2007) discovered an aquifer near this area with brackish water (salinity of 30). Considering the bathymetry, the volume occupied by seawater was 19 700 m3. We also assumed that groundwater discharge only occurred at low tide. The average salinity changed from 36.93 (equivalent to 745.8 t of sea salt) at low tide to 37.02 at high tide (747.5 t of sea salt). The decrease in salinity at low tide could be accounted for by the emission of 57 m3 of brackish groundwater.
The brackish groundwater was also responsible for the AT and CT changes (Fig. 3a, b). Alkalinity increased by 219 µmol kg−1 from high tide (2465 µmol kg−1) to low tide (2684 µmol kg−1). Considering 57 m3 of brackish water, 4.40 kmol of alkalinity was required; therefore, the brackish groundwater had an AT concentration of 76 mmol kg−1. Similarly, the CT on the beach increased by 333 µmol kg−1, from high tide (2190 µmol kg−1) to low tide (2523 µmol kg−1). The brackish water caused the increase of 6.7 kmol of inorganic carbon on the beach and, therefore, had an endmember concentration of 116 mmol kg−1.
Considering the in situ temperature (20.67 ∘C), the pHT,is decreased by 0.25 from 8.01 at high tide to 7.76 at low tide. This meant that the acidity increased by 80 %. This pH reduction meant that the water discharged on the beach had a pH of 5.57. The medium partial pressure of carbon dioxide for the area increased from 459 µatm at high tide to a value of 988 µatm at low tide. Considering an average wind speed at the beach of 7 m s−1 (https://datosclima.es/Aemethistorico/Vientostad.php, last access: 5 March 2020), Playa del Faro acts as a strong source of CO2, emitting 5.70 mmol CO2 m−2 d−1 at high tide and increasing by an order of magnitude at low tide (57 mmol CO2 m−2 d−1; Wanninkhof, 2014). Consequently, Playa del Faro with its small area of only 0.01 km2 is responsible for an atmospheric CO2 emission flux varying between 2.80 and 28 kg CO2 d−1.
4.1 The origin of the CO2 submarine groundwater discharge
Although CO2 emissions on the Fuencaliente coast had already been detected (e.g. Hernández et al., 2016; Viotti et al., 2019), this is the first time that this naturally acidified system has been described chemically and physically. Previous works have focused on specific questions; Hernández et al. (2016) published for the first time the presence of CO2 SGD in Fuencaliente, specifically on Las Cabras beach. Later, in the thesis by Pérez (2017), as well as in the conference papers by González-Delgado et al. (2018a, b) and in the article by Viotti et al. (2019), new points of acidification were discovered on Playa del Faro and Los Porretos. However, in none of them was a chemical characterization of the whole area made as it was here. Our results reveal the continuous influence of brackish water discharge in the acidification process of the Punta de Fuencaliente system (PFS), which had been missed before (Fig. 5). Similarly to aerial remnant volcanic activity on La Palma that generates high CO2 diffusive atmospheric concentration (Padrón et al., 2015), submarine remnant volcanic activity causes the acidification process found here, as indicated by the chemical composition of the groundwater analysed, which is less than 200 m from the coast (Soler-Liceras, 2007). The activity of this SGD is comparable with other CO2 vent and seep systems worldwide (references within González-Delgado and Hernández, 2018). Moreover, the presence of the acidic water flow of La Palma also has a slight resemblance to the acidification phenomenon found in Mexico, originating from a karstic groundwater discharge (Crook et al., 2012). Furthermore, the highly alkalized and bicarbonate waters found in Echentive lagoons are an artefact of water discharge from the hydrothermally affected aquifers of the area (Soler-Liceras, 2007), as found in Las Cañadas del Teide, in Tenerife (another island of the same archipelago) (Marrero et al., 2008).
In the PFS there is a decrease in salinity due to brackish water discharges. Hence, there is a constant filtration of brackish acidified waters through highly permeable volcanic rocks (Carracedo et al., 2001; Marrero et al., 2008), with chemical features due to underground volcanic activity, such as a 5.57 pH and a concentration of 76 mmol kg−1 of AT and 116 mmol kg−1 of CT. However, the effect on the surrounding seawaters depends upon tidal pressure and, more likely, upon other oceanic forces such as wind and waves (Moore, 2010; Mulligan et al., 2019).
4.2 Alteration of the carbon chemistry system and implications for organism's assemblages
In the case of the PFS, the water with lower salinity (36.79–36.45) and high concentrations of CT and AT affect the surroundings, decreasing the seawater pH by up to 0.8 and reducing the carbonates' saturation state by up to 1.1 for calcite and 0.7 for aragonite. This situation generates a carbon imbalance affecting carbonated organisms, especially those that precipitate aragonite on their calcareous structures (Kroeker et al., 2010). When the saturation values are below 1, the formation of carbonates is not thermodynamically possible, although certain species require much higher saturation levels (Kroeker et al., 2010). The calcifying organisms that could live in these acidified areas may present weaker shells, skeletons and/or others solid structures, as we have recently observed in the mollusc Phorcus sauciatus (Viotti et al., 2019), as well as in other calcifying organisms (Pérez, 2017). This excess of CO2 has also modified the community composition and trophic structure, causing a loss of ecological and functional diversity in the benthic marine ecosystem (González-Delgado et al., 2021).
In the case of Echentive lagoons, the anomaly is amplified due to a lower tidal influence and insulation. These acidified lagoons, which are at around a 200 m distance from the coast (Fig. 1), have a salinity of 32 and CT and AT concentrations 5 times higher than normal ocean values. The CT and AT concentrations are so high that they compensate for the decrease in pH with the content of carbonates in the water. These singular characteristics create a unique marine ecosystem. The environment is dominated by a biofilm of microorganisms, predominantly microalgae, cyanobacteria and diatoms (Sangil et al., 2008) and probably other bacteria and fungi. Nonetheless, some marine invertebrates persist, such as the common errant polychaete Eurythoe complanata and the anemone Actinia sp. (Sangil et al., 2008). A more in-depth physiological study of these species could help us to better understand their adaptation process to these conditions and to give insights into what we might expect in future ocean acidification conditions, especially in the PFS area.
4.3 La Palma as a natural laboratory for marine research
The natural CO2 gradients south of La Palma have been characterized from shore to offshore, varying for CT from 2120.10 to 3794.00 µmol kg−1, for pH from 7.12 to 8.07, for Ω aragonite from 0.71 to 3.28 and for Ω calcite from 1.09 to 5.02. This high local variability is in line with other acidified natural systems. For example, the CO2 vent of Ischia (Italy) has pH levels from 6.07 to 8.17, Ω aragonite from 0.07 to 4.28 and Ω calcite from 0.11 to 6.40 (Hall-Spencer et al., 2008). The one from the island of Vulcano (Italy) has pH values between 6.80 and 8.20, Ω aragonite from 1.49 to 4.65, and Ω calcite from 2.28 to 7.00 (Boatta et al., 2013). Meanwhile the CO2 seeps from Papua New Guinea have pH levels between 7.29–7.98, Ω aragonite between 1.2–3.4 and Ω calcite between 1.36–5.12 (Fabricius et al., 2011). Those from Shikine-jima (Japan) have pH values between 6.80 and 8.10, Ω aragonite from 0.20 to 2.22, and Ω calcite from 0.30 to 3.45 (Agostini et al., 2015). Although these systems are far from being perfect predictors of the ocean future due to their chemical variability and physical limitations, they have proven to be important tools for the study of ocean acidification (Foo et al., 2018; González-Delgado and Hernández, 2018; Aiuppa et al., 2021). These naturally acidified systems, such as the Punta de Fuencaliente system (PFS), can be used as natural analogues of climate change scenarios predicted by the IPCC (2014) (Fig. 6). Therefore the PFS can be considered a very useful spot for large-scale and long-term adaptation experiments, as seen in other CO2 systems (e.g. Ricevuto et al., 2014; Uthicke et al., 2019). Moreover, the acidified system of La Palma is highlighted by the absence of bubbling, since the volcanic degasification takes place in the aquifers and not directly on the coast as in other acidified systems of volcanic origin (e.g. Hall-Spencer et al., 2008; Fabricius et al., 2011) (Fig. 5). This could give us new insights into the effect of acidification in situ avoiding the effects of bubbling (González-Delgado and Hernández, 2018). Nevertheless, several caveats for future prediction experiments should be considered, here as well as in other naturally acidified systems, especially those related to increased alkalinity values in the submarine discharge.
First, there is a clear tidal influence; this is an important force that controls the acidified brackish water discharges. Although a fluctuation in the emission is observed, normal ocean conditions can occur for a short time, about 2–4 h d−1, during high tide and depending on the oceanic conditions (Viotti et al., 2019). The pHT is severely affected by the location, reaching down to ∼7.2 in the emission points, so a careful selection of the study sites is recommended, depending on the study objectives (Fig. 6). This tidal phenomenon has also been reported in other acidified natural systems such as Puerto Morelos in Mexico (Crook et al., 2012) and Ischia (Kerrison et al., 2011). However, the pH time fluctuation can be used to our advantage, as a daily and seasonal fluctuation in the pH is normal in coastal habitat environments (Hofmann et al., 2011). So, it could be considered very useful to incorporate pH variability in ocean acidification studies as environmental fluctuations that can have a large impact on marine organisms (Hofmann et al., 2011).
Second, one of the most common concerns with CO2 seeps and SGD areas is the presence of other gases or elements associated with volcanic emissions, such as nitrogen (N2), mercury (Hg) or methane (CH4) (e.g. Fabricius et al., 2011; Boatta et al., 2013; Aiuppa et al., 2021). Although there are no traces of the presence of volcanic elements such as methane or sulfates that are harmful to marine organisms in the seawater of the PFS (Hernández et al., 2016), there is an extra supply of different elements such as Mg that comes from groundwater (Soler-Liceras, 2007). Groundwater has 10 times more magnesium than normal, but when mixed with seawater, the supply is considerably lower compared to CO2. Nevertheless, Mg plays an important role in the calcification of marine organisms that have magnesite–calcite, such as echinoderms (Weber, 1969) and some Bryozoa species (Smith et al., 2006). Similarly, Hernández et al. (2016) found an increase in silicates in the nearby area of Las Cabras. In these cases, Si could participate in the calcification of diatoms (Paasche, 1973) as well as of many sponges (Smith et al., 2013). The increase in these essential elements for certain calcifying species can allow their survival and growth in the PFS while buffering the effects of acidification (Smith et al., 2016; Ma et al., 2009). Therefore, measurements of other metals in seawater should be considered in the following studies.
The high concentration of bicarbonate in the brackish waters also implies an extra contribution of alkalinity and carbonate that can buffer the effect of acidification in the area, so it is necessary to take this into account when making predictions of the future. These values together with calcium content are especially important factors in the case of the saturation state for both calcite and aragonite, which shows high values for seawater with low pH values. Hence, despite the fact that we are dealing with a subtropical ecosystem, the values obtained in both saturation states are more similar to the predictions for a tropical ecosystem, such as the values found in Papua New Guinea seeps (Fabricius et al., 2011; IPCC, 2014).
Finally, the area is not very large and only one type of rocky benthic habitat, the most typical community of the Canary Islands, is present at the PFS (Sangil et al., 2018). Therefore, all conclusions derived from this acidified system should be interpreted with caution and acknowledging local effects. Hence, it is crucial to establish a collaborative network of researchers who are working in other naturally acidified systems worldwide to have a more realistic interpretation of future ocean scenarios.
The Echentive lagoons are an oversaturated carbonate system. Like hydrothermal alkalinity vents (Martin et al., 2008), they could help us to understand early life on Earth from the Precambrian, 4000 million years ago, when the atmosphere was rich in CO2 (Kasting, 1993; Nakamura and Kato, 2004) (Fig. 6). These studies could allow us to disentangle the adaptation and evolution of marine life to the changing carbonate conditions over time (Gattuso et al., 1998).
Additionally, to our knowledge, this is the first time that a brackish water discharge altered by volcanic activity has been studied. Each studied beach with a contribution of 150 t CO2 yr−1 becomes an important source of carbon into the sea. Correspondingly, Playa del Faro is emitting 28 kg CO2 d−1 in each tidal flow to the atmosphere. This may seem very scarce compared to volcanic eruptions such as the most recent in the Canaries that occurred on the neighbouring island, El Hierro, in 2010, which was emitting kg d−1, and now the emissions of the PFS are unappreciated (Santana-Casiano et al., 2016). However, the flux of CO2 from La Palma seems to have started before the islands were conquered in 1493 (Soler-Liceras, 2007), being in a more advanced degassing phase than El Hierro with fewer emissions, and continued over time. Therefore, if we consider its timescale, La Palma becomes a significant CO2 source. For all these reasons, the PFS and the lagoons are an interesting area for future hydrological and oceanographic research, helping in new studies focusing on groundwater fluxes, the oceanic water cycle and oceanic carbon fluctuation (Moore, 2010; Santana-Casiano et al., 2016; Mulligan et al., 2019).
The studies carried out show the existence of continuous natural acidification on the southern coast of La Palma. This acidification process is caused by two natural phenomena: the discharge of submarine brackish waters from the aquifer and the magmatic emissions of CO2 gas. Therefore, the monitoring of both sources is important not only from a biological point of view but also from an atmospheric, oceanographic, volcanologist and hydrological perspective. The groundwater discharges found on Playa del Faro and Los Porretos (PFS) have similar chemical properties (even when alkalinity does not remain constant) that create a natural pH gradient analogous to future ocean conditions. Consequently, they can be used as natural laboratories to predict the effects of OA on the functioning of future oceans. In addition, the interior Echentive lagoons where the chemical alterations are intensified present the conditions capable of disentangling how life has persisted during higher-atmospheric-CO2 periods on planet Earth.
All measures obtained and used in this work are available in the Supplement.
The supplement related to this article is available online at: https://doi.org/10.5194/bg-18-1673-2021-supplement.
Sampling and data analysis were performed by all authors. SGD and JCH led the paper writing, and all authors contributed to the interpretation of the results and writing.
The authors declare that they have no conflict of interest.
We thank the officers, crew and researchers of the R/V Ángeles Alvariño from the Instituto Español de Oceanografía (IEO) for their help during the sampling process in March 2018, especially Eugenio Fraile and Francisco Domingo (from the VULCANA-II-0318 project). Also, we want to thank Adrián Castro for his help during the water sample analysis in the laboratory of the QUIMA group (ULPGC) and Enrique Lozano Bilbao from the University of La Laguna for his comments and feedback. Finally, we very much appreciate all the help offered by the Fuencaliente town hall (La Palma).
This research received a grant from the Fundación Biodiversidad of the Ministerio para la Transición Ecológica y el Reto Demográfico of the Spanish Government and help from the Ministerio de Economía y Competitividad through the ATOPFe project (CTM2017-83476).
This paper was edited by Peter Landschützer and reviewed by Celeste Sánchez-Noguera, Sylvain Agostini and one anonymous referee.
Agostini, S., Wada, S., Kon, K., Omori, A., Kohtsuka, H., Fujimura, H., Tsuchiyaa, Y., Satoa, T., Shinagawaa, H., Yamadaa, Y., and Inaba, K.: Geochemistry of two shallow CO2 seeps in Shikine Island (Japan) and their potential for ocean acidification research, Reg. Stud. Mar. Sci., 2, 45–53, 2015.
Aiuppa, A., Hall-Spencer, J. M., Milazzo, M., Turco, G., Caliro, S., and Di Napoli, R.: Volcanic CO2 seep geochemistry and use in understanding ocean acidification, Biogeochemistry, 152, 93–115, 2021.
Boatta, F., D'Alessandro, W., Gagliano, A. L., Liotta, M., Milazzo, M., Rodolfo-Metalpa, R., Hall-Spencer, J. M., and Parello, F.: Geochemical survey of Levante Bay, Vulcano Island (Italy), a natural laboratory for the study of ocean acidification, Mar. Pollut. Bull. 73, 485–494, 2013.
Burnett, W. C., Aggarwal, P. K., Aureli, A., Bokuniewicz, H., Cable, J. E., Charette, M. A., Kontar, E., Krupa, S., Kulkarni, K. M., Loveless, A., and Moore, W. S.: Quantifying submarine groundwater discharge in the coastal zone via multiple methods, Sci. Total Environ. 367, 498–543 https://doi.org/10.1016/j.scitotenv.2006.05.009, 2006.
Calvet, F., Cabrera, M. C., Carracedo, J. C., Mangas, J., Recio, C., and Travé, A.: Beachrocks from the island of La Palma (Canary Islands, Spain), Mar. Geol., 197, 75–93, https://doi.org/10.1016/S0025-3227(03)00090-2, 2003.
Carracedo, J. C., Rodríguez-Badiola, E., Guillou, H., de la Nuez, J. D. L., and Pérez-Torrado, F. J.: Geology and volcanology of La Palma and El Hierro, Western Canaries, Estudios Geologicos, 57, 5–6, 2001.
Charette, M.A., Lam, P.J., Lohan, M. C., Kwon, E. Y., Hatje, V., Jeandel, C., Shiller, A. M., Cutter, G. A., Thomas, A., Boyd, P. W., and Homoky, W. B.: Coastal ocean and shelf-sea biogeochemical cycling of trace elements and isotopes: lessons learned from GEOTRACES, Philos. T. Roy. Soc. A, 374, 20160076, https://doi.org/10.1098/rsta.2016.0076, 2016.
Crook, E. D., Potts, D., Rebolledo-Vieyra, M., Hernandez, L., and Paytan, A.: Calcifying coral abundance near low-pH springs: implications for future ocean acidification, Coral Reefs, 31, 239–245, https://doi.org/10.1007/s00338-011-0839-y, 2012.
Dando, P. R., Stüben, D., and Varnavas, S. P.: Hydrothermalism in the Mediterranean sea, Prog. Oceanogr., 44, 333–367, https://doi.org/10.1016/S0079-6611(99)00032-4, 1999.
Dickson, A. G., Sabine, C. L., and Christian, J. R.: Guide to best practices for ocean CO2 measurements, North Pacific Marine Science Organization Sidney, British Columbia, available at: http://hdl.handle.net/11329/249 (last access: 17 June 2019), 191 pp., 2007.
Fabricius, K .E., Langdon, C., Uthicke, S., Humphrey, C., Noonan, S., De'ath, G., Okazaki, R., Muehllehner, N., Glas, M. S., and Lough, J. M.: Losers and winners in coral reefs acclimatized to elevated carbon dioxide concentrations, Nat. Clim. Change, 1, 165–169, 2011.
Foo, S. A., Byrne, M., Ricevuto, E., and Gambi, M. C.: The carbon dioxide vents of Ischia, Italy, a natural system to assess impacts of ocean acidification on marine ecosystems: an overview of research and comparisons with other vent systems, Oceanogr. Mar. Biol. Annu. Rev., 56, 237–310, 2018.
Gattuso, J. P., Frankignoulle, M., Bourge, I., Romaine, S., and Buddemeier, R. W.: Effect of calcium carbonate saturation of seawater on coral calcification. Global Planet. Change, 18, 37–46, https://doi.org/10.1016/S0921-8181(98)00035-6, 1998.
González-Dávila, M., Santana-Casiano, J. M., and González-Dávila, E. F.: Interannual variability of the upper ocean carbon cycle in the northeast Atlantic Ocean, Geophys. Res. Lett., 34, L07608, https://doi.org/10.1029/2006GL028145, 2007.
González-Delgado, S. and Hernández, J. C.: The importance of natural acidified systems in the study of ocean acidification: what have we learned?, Adv. Mar. Biol., 80, 57–99, https://doi.org/10.1016/bs.amb.2018.08.001, 2018.
González-Delgado, S., Hernández, J. C., Epherra, L., Hernández, C., and Alfonso, B.: Effect of a natural CO2 gradient on egg characteristics of Arbacia lixula, 16th International echinoderm Conference, Nagoya, Japan, 28 May–1 June 2018, 165 pp., 2018a.
González-Delgado, S., Hernández, J. C., Wangensteen, O., Alfonso, B., and Soto, A.: Changes in echinoderm populations due to a natural CO2 gradient. Program & Abstracts: 16th International echinoderm Conference, Nagoya, Japan, 28 May–1 June 2018, 58 pp., 2018b.
González-Delgado, S., Sangil, C., Wangensteen, O. S., Hernández, C. A., Soto, A. Z., Alfonso, B., Mariani S., and Hernández, J. C.: Persistent natural CO2 disturbances increase taxonomic diversity of a benthic community, in preparation, 2021.
Hall-Spencer, J. M., Rodolfo-Metalpa, R., Martin, S., Ransome, E., Fine, M., Turner, S. M., Rowley, S. J., Tedesco, D., and Buia, M. C.: Volcanic carbon dioxide vents show ecosystem effects of ocean acidification, Nature, 454, 96–99, https://doi.org/10.1038/nature07051, 2008.
Hall-Spencer, J. M. and Harvey, B. P.: Ocean acidification impacts on coastal ecosystem services due to habitat degradation, Emerg. Top. Life Sci., 3, 197–206, https://doi.org/10.1042/ETLS20180117, 2019.
Hernández, C. A., Epherra, L., Alfonso, B., González-Delgado, S., Hernández, J. C. Characterization of a CO2 vent in La Palma (Canary Islands) and its effects on the calcified structures of Arbacia lixula. Program & Abstracts: 16th International echinoderm Conference, Nagoya, Japan, 28 May–1 June 2018, 63 pp., 2018.
Hernández, C. A., Sangil, C., and Hernández, J. C.: A new CO2 vent for the study of ocean acidification in the Atlantic, Mar. Pollut. B. 109, 419–426, https://doi.org/10.1016/j.marpolbul.2016.05.040, 2016.
Hofmann, G. E., Smith, J. E., Johnson, K. S., Send, U., Levin, L. A., Micheli, F., Paytan, A., Price, N. N., Peterson, B., Takeshita, Y., Matson, P. G., Crook, E. D., Kroeker, K. J., Gambi, M. C., Rivest, E. B., Frieder, C. A., Yu, P. C., and Martz, T. R.: High-frequency dynamics of ocean pH: a multi-ecosystem comparison, Plos One, 6, e28983, https://doi.org/10.1371/journal.pone.0028983, 2011.
IPCC: Core Writing Team, Pachauri, R. K., and Meyer, L. A.: Climate Change 2014: Synthesis Report. Contribution of Working Groups I, II and III to the Fifth Assessment Report of the Intergovernmental Panel on Climate Change, Geneva, Switzerland, 151 pp., 2014.
Jeandel, C.: Overview of the mechanisms that could explain the `Boundary Exchange'at the land–ocean contact. Philos. T. Roy. Soc. A., 374, 20150287, https://doi.org/10.1098/rsta.2015.0287, 2016.
Kasting, J. F.: Earth's early atmosphere, Science, 259, 920–926, https://doi.org/10.1126/science.11536547, 1993.
Kerrison, P., Hall-Spencer, J. M., Suggett, D. J., Hepburn, L. J., and Steinke, M.: Assessment of pH variability at a coastal CO2 vent for ocean acidification studies, Estuar. Coast. Shelf Sci., 94, 129–137, 2011.
Kroeker, K. J., Kordas, R. L., Crim, R. N., and Singh, G. G.: Meta-analysis reveals negative yet variable effects of ocean acidification on marine organisms, Ecol. Lett., 13, 1419–1434, https://doi.org/10.1111/j.1461-0248.2010.01518.x, 2010.
Ma Y., Aichmayer B., Paris O., Fratzl P., Meibom A., Metzler R. A., Politi Y., Addadi L., Gilbert P. U., and Weiner S.: The grinding tip of the sea urchin tooth exhibits exquisite control over calcite crystal orientation and Mg distribution, P. Natl. Acad. Sci. USA, 106, 6048–6053, https://doi.org/10.1073/pnas.0810300106, 2009.
Marrero, R., López, D. L., Hernández, P. A., and Pérez, N. M.: Carbon dioxide discharged through the las Cañadas aquifer, Tenerife, Canary Islands, Pure Appl. Geophys., 165, 147–172, https://doi.org/10.1007/s00024-007-0287-3, 2008.
Martin, W., Baross, J., Kelley, D., and Russell, M. J.: Hydrothermal vents and the origin of life, Nat. Rev. Microbiol., 6, 805, https://doi.org/10.1038/nrmicro1991, 2008.
Migliaccio, O., Pinsino, A., Maffioli, E., Smith, A. M., Agnisola, C., Matranga, V., Nonnis, S., Tedeschi, G., Byrne, M., Gambi, M. C., and Palumbo, A. Living in future ocean acidification, physiological adaptive responses of the immune system of sea urchins resident at a CO2 vent system, Sci. Total Environ., 672, 938–950, 2019.
Mintrop, L., Pérez, F. F., González-Dávila, M., Santana-Casiano, J. M., and Körtzinger, A.: Alkalinity determination by potentiometry: Intercalibration using three different methods, Cienc. Mar., 26, 23–37, http://hdl.handle.net/10261/25136 (last access: 1 February 2020), 2000.
Moore, W. S.: The effect of submarine groundwater discharge on the ocean, Ann. Rev. Mar. Sci. 2, 59–88, https://doi.org/10.1146/annurev-marine-120308-081019, 2010.
Mulligan, A. E., Charette, M. A., Tamborski, J. J., and Moosdorf, N.: Submarine Groundwater Discharge, in: Encyclopedia of Ocean Sciences, edited by: Cochran, J. K., Yager, P. L., and Bokuniewicz, H. J., Reference Module in Earth Systems and Environmental Sciences, Elsevier, the Netherlands, 108–119, https://doi.org/10.1016/B978-0-12-409548-9.11482-4, 2019.
Nagelkerken, I., Russell, B. D., Gillanders, B. M., and Connell, S. D.: Ocean acidification alters fish populations indirectly through habitat modification, Nat. Clim. Change, 6, 89–93, 2016.
Nakamura, K. and Kato, Y.: Carbonatization of oceanic crust by the seafloor hydrothermal activity and its significance as a CO2 sink in the Early Archean, Geochim. Cosmochim. Ac., 68, 4595–4618, https://doi.org/10.1016/j.gca.2004.05.023, 2004.
Olivé, I., Silva, J., Lauritano, C., Costa, M. M., Ruocco, M., Procaccini, G., and Santos, R.: Linking gene expression to productivity to unravel long-and short-term responses of seagrasses exposed to CO2 in volcanic vents, Sci. Rep.-UK, 7, 42278, https://doi.org/10.1038/srep42278, 2017.
Paasche, E.: Silicon and the ecology of marine plankton diatoms. II. Silicate-uptake kinetics in five diatom species, Mar. Biol., 19, 262–269, https://doi.org/10.1007/BF02097147, 1973.
Padrón, E., Pérez, N. M., Rodríguez, F., Melián, G., Hernández, P. A., Sumino, H., Padilla, G., Barrancos, J., Dionis, S., Notsu, K., and Calvo, D.: Dynamics of carbon dioxide emissions from Cumbre Vieja volcano, La Palma, Canary Islands. B. Volcanol., 77, 28, https://doi.org/10.1007/s00445-015-0914-2, 2015.
Pérez, C.: Effects of a Natural CO2 Gradient on Benthic Coastal Populations, Degree Project, University of La Laguna, available at: https://riull.ull.es/xmlui/handle/915/6758 (last access: 6 July 2019), 2017.
Pierrot, D., Lewis, E., and Wallace, D. W. R.: MS Excel program developed for CO2 system calculations, in: ORNL/CDIAC-105a. Carbon Dioxide Information Analysis Center, Oak Ridge National Laboratory, US Department of Energy, Oak Ridge, Tennessee, 2006.
Ricevuto, E., Kroeker, K. J., Ferrigno, F., Micheli, F., and Gambi, M. C.: Spatio-temporal variability of polychaete colonization at volcanic CO2 vents indicates high tolerance to ocean acidification, Mar. Biol., 161, 2909–2919, 2014.
Sangil, C., Clemente, S., and Francisco, L. C.: Ambientes litorales marginales en las islas Canarias: estructura y composición de las comunidades bentónicas en las Lagunas de Echentive (La Palma), Vieraea, 36, 143–162, 2008 (in Spanish).
Sangil, C., Martins, G. M., Hernández, J. C., Alves, F., Neto, A. I ., Ribeiro, C., León-Cisneros, K., Canning-Clode, J., Rosas-Alquicira, E., Mendoza, J. C., and Titley, I.: Shallow subtidal macroalgae in the North-eastern Atlantic archipelagos (Macaronesian region): a spatial approach to community structure, Eur. J. Phycol., 53, 83–98, https://doi.org/10.1080/09670262.2017.1385098, 2018.
Santana-Casiano, J. M., Fraile-Nuez, E., González-Dávila, M., Baker, E. T., Resing, J. A., and Walker, S. L.: Significant discharge of CO2 from hydrothermalism associated with the submarine volcano of El Hierro Island, Sci. Rep.-UK, 6, 25686, https://doi.org/10.1038/srep25686, 2016.
Smith, A. M., Berman, J., Key Jr., M. M., and Winter, D. J.: Not all sponges will thrive in a high-CO2 ocean: Review of the mineralogy of calcifying sponges, Palaeogeogr. Palaeocll., 392, 463–472, https://doi.org/10.1016/j.palaeo.2013.10.004, 2013.
Smith, A. M., Clark, D. E., Lamare, M. D., Winter, D. J., and Byrne, M.: Risk and resilience: variations in magnesium in echinoid skeletal calcite, Mar. Ecol. Prog. Ser., 561, 1–16, https://doi.org/10.3354/meps11908, 2016.
Smith, A. M., Key Jr, M. M., and Gordon, D. P.: Skeletal mineralogy of bryozoans: taxonomic and temporal patterns, Earth-Sci. Rev., 78, 287–306, https://doi.org/10.1016/j.earscirev.2006.06.001, 2006.
Soler-Liceras, C.: La historia de la Fuente Santa, Editorial Turquesa, Santa Cruz de Tenerife, 2007 (in Spanish).
Szymczycha, B., Maciejewska, A., Winogradow, A., and Pempkowiak, J.: Could submarine groundwater discharge be a significant carbon source to the southern Baltic Sea?, Oceanologia, 56, 327–347, https://doi.org/10.5697/oc.56-2.327, 2014.
Tarasov, V. G., Gebruk, A. V., Mironov, A. N., and Moskalev, L. I.: Deep-sea and shallow water hydrothermal vent communities: two different phenomena?, Chem. Geol., 224, 5–39, https://doi.org/10.1016/j.chemgeo.2005.07.021, 2005.
Uthicke, S., Deshpande, N. P., Liddy, M., Patel, F., Lamare, M., and Wilkins, M. R.: Little evidence of adaptation potential to ocean acidification in sea urchins living in “Future Ocean” conditions at a CO2 vent, Ecol. Evol., 9, 10004–10016, 2019.
Viotti, S., Sangil, C., Hernández, C. A., and Hernández, J. C.: Effects of long-term exposure to reduced pH conditions on the shell and survival of an intertidal gastropod, Mar. Environ. Res., 152, 104789, https://doi.org/10.1016/j.marenvres.2019.104789 , 2019.
Wanninkhof, R.: Relationship between wind speed and gas exchange over the ocean revisited, Limnol. Oceanogr.-Meth., 12, 351–362, https://doi.org/10.1029/92JC00188, 2014.
Weber J. N.: The incorporation of magnesium into the skeletal calcites of echinoderms, Am. J. Sci., 267, 537–566, https://doi.org/10.2475/ajs.267.5.537, 1969.
The requested paper has a corresponding corrigendum published. Please read the corrigendum first before downloading the article.
- Article
(5482 KB) - Full-text XML
- Corrigendum
-
Supplement
(3093 KB) - BibTeX
- EndNote