the Creative Commons Attribution 4.0 License.
the Creative Commons Attribution 4.0 License.
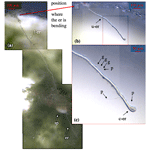
Permanent ectoplasmic structures in deep-sea Cibicides and Cibicidoides taxa – long-term observations at in situ pressure
Jutta E. Wollenburg
Jelle Bijma
Charlotte Cremer
Ulf Bickmeyer
Zora Mila Colomba Zittier
Deep-sea Cibicidoides pachyderma (forma mundulus) and related Cibicidoides spp. were cultured at in situ pressure for 1–2 d, or 6 weeks to 3 months. During that period, fluorescence analyses following BCECF-AM (2′,7′-bis(2-carboxyethyl)-5-(and-6)-carboxyfluorescein acetoxymethyl ester) or calcein (bis[N,N-bis(carboxymethyl)aminomethyl]-fluorescein) labelling revealed a persisting cytoplasmic sheet or envelope surrounding the Cibicidoides tests. Thus, the Cibicidoides shell can be considered as an internal rather than an external cell structure. A couple of days to a week after being transferred into high-pressure aquaria and adjusted to a pressure of 115 bar, the foraminifera changed from a mobile to a more or less sessile living mode. During this quasi-sessile way of life, a series of comparably thick static ectoplasmic structures developed that were not resorbed or remodelled but, except for occasional further growth, remained unchanged throughout the experiments. Three different types of these permanent structures were observed. (a) Ectoplasmic “roots” were common in adult C. pachyderma, C. lobatulus, and C. wuellerstorfi specimens. In our experiments single ectoplasmic roots grew to a maximum of 700 times the individuals' shell diameter and were presumably used to anchor the specimen in an environment with strong currents. (b) Ectoplasmic “trees” describe rigid ectoplasmic structures directed into the aquarium's water body and were used by the foraminifera to climb up and down these ectoplasmic structures. Ectoplasmic trees have so far only been observed in C. pachyderma and enabled the tree-forming foraminifera to elevate itself above ground. (c) Ectoplasmic “twigs” were used to guide and hold the more delicate pseudopodial network when distributed into prevailing currents and were, in our experiments, also only developed in C. pachyderma specimens. Relocation of a specimen usually required it to tear apart and leave behind the rigid ectoplasmic structures and eventually also the envelope surrounding the test. Apparently, these rigid structures could not be resorbed or reused.
- Article
(7109 KB) - Full-text XML
- BibTeX
- EndNote
Our knowledge on form and functioning of ectoplasmic extensions in benthic foraminifera is based on laboratory observations of a few shallow-water species under atmospheric pressure. In 1835 Félix Dujardin published a series of short papers where he not only noticed that the investigated animals were not micro-cephalopods but also that these animals interacted with filaments, which he called rhizopoda, and with the environment, which is why he proposed the name Rhizopoda for the group (Dujardin, 1835a, b, c, d). Subsequent studies describe complex networks of branching and anastomosing pseudopodia that are rapidly and alternately extended and withdrawn into the surrounding environment (Bowser and Travis, 2002; Hedley, 1964; Lee and Anderson, 1991; Lee, 1985; Schultze, 1854). The almost continuously remodelling pseudopodia are used for motility, attachment, food collection, the formation of cysts, growth, and certain aspects of reproduction (Goldstein, 1999; Heinz, 2005; Travis et al., 2002; Tyszka et al., 2019).
Numerous cytoplasmic particles give the pseudopodia a granular appearance when viewed under the light microscope (Goldstein, 1999; Hedley, 1964; Schultze, 1854). The main components of granule are mitochondria, (secretory, excretory, and storage) vesicles or vacuoles, and occasionally symbionts (Bowser and Travis, 2002; Goldstein, 1999; Hedley, 1964; Lee, 1985). Independently of whether pseudopodia modify their shape or are in a stationary state, they display constant bidirectional streaming (Bowser and Travis, 2002; Rinaldi, 1964). Coupled to this cytoplasmic streaming, particles are transported bidirectional along the extracellular surfaces of pseudopodia (Bowser et al., 1984; Bowser and DeLaca, 1985). Foraminifera use this extracellular conveyor belt to collect particles for agglutination or nutrition (Bowser and Travis, 2002).
The majority of foraminifera of the genus Cibicides (e.g. C. refulgens, C. antarcticus) and a significant proportion of Cibicidoides species (e.g. C. lobatulus, C. wuellerstorfi, and C. pachyderma with the morphotypes C. pachyderma, C. kullenbergi, and C. mundulus; see Schweizer, 2009, for the genetic versus morphological classification) are epibenthic (Jorissen et al., 1995; Linke and Lutze, 1993; Lutze, 1989; Nyholm, 1962), although rose-bengal-stained specimens are occasionally found at 1–4 cm sediment depth (e.g. Hunt and Corliss, 1993; Wollenburg and Mackensen, 1998b). However, an affinity of Cibicides and Cibicidoides species to settle in places exposed to currents has been inferred from the preferential colonization of elevated structures exposed to currents or on filter-feeding invertebrates (e.g. Alexander and DeLaca, 1987; Linke and Lutze, 1993; Schönfeld, 2002). Although facultative grazing on phytodetritus and bacteria on the sediment is proposed for some species such as Cibicides pachyderma (Alexander and DeLaca, 1987) the majority of Cibicides and Cibicidoides species are assumed to be passive suspension feeders (Lipps, 1983) trapping phytodetritus by deployment of a pseudopodial network in the prevailing current.
The main target of this study was C. pachyderma, of which we continuously observed 57 specimens under in situ pressure, temperature, and current activity conditions over a time span of 3 months. Daily observations allowed us to shed light on the development of temporary and lasting ectoplasmic extensions in C. pachyderma, one of the most important species for palaeo-reconstructions of the deep sea.
To determine if the observed ectoplasmic structures are unique to C. pachyderma or common to the related genera Cibicides and Cibicidoides, 40 C. lobatulus and 3 C. wuellerstorfi specimens were cultured at corresponding conditions and visually inspected daily to weekly for a time period of 6 weeks. To prove that shells were covered by living cytoplasm, in addition, fluorescence studies on the ectoplasmic envelope of C. lobatulus were carried out for 1–3 d.
Table 1Basic parameters of the culture experiments. Oxygen and pH values were measured with a combined O2 and pH measuring device (WTW Multi 3620 IDS) and respective O2 (WTW FDO® 925) and pH (SenTix® 980) sensors three times per week. Fine-grained siliceous oxide (1–5 µm) was used as artificial sediment in one out of four aquaria in the C. pachyderma (∗) and in all aquaria of the C. lobatulus/C. wuellerstorfi culture experiments.
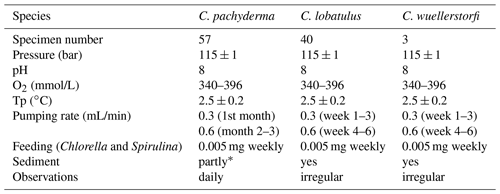
Central to this study are more or less daily observations on permanent ectoplasmic structures in 57 C. pachyderma specimens that were cultured for 3 months during the “experiment (1)” of 2017 (Wollenburg et al., 2018). In 2018, we complemented this data set by daily to weekly observations on permanent ectoplasmic structures in 40 C. lobatulus and 3 C. wuellerstorfi specimens cultured for 6 weeks using the same set-up and experimental design as for C. pachyderma (Table 1). In 2019 fluorescence studies on the ectoplasmic envelope of C. lobatulus were carried out for 1–3 d.
High-pressure culturing with small aquaria, like we have used during these experiments, requires keeping a stock of foraminifera at atmospheric pressure for some weeks or months in advance. The decision in favour of Cibicidoides pachyderma and C. lobatulus species was made as both species live from the shelf to water depths > 1000 m and can, thus, be cultured at atmospheric conditions until they are used in high-pressure experiments. Although it has been shown that barophilic C. wuellerstorfi is able to survive depressurization for weeks and can reproduce when subsequently cultured at in situ pressure (Wollenburg et al., 2015), so far there is no proof that the cell functioning is not altered under such conditions.
During the RV Polarstern expedition PS101 in 2016, pebbles from surface sediments were collected with a multicorer (MUC) at 79∘27.09′ N, 7∘30.93′ E, 856 m water depth, and used as stock for the Cibicidoides pachyderma experiment (Wollenburg et al., 2018). During the RV G.O. Sars expedition GS2018108 (July–August 2018), pebbles with attached living C. lobatulus and C. wuellerstorfi specimens were collected at 900 m water depth on the Norwegian continental slope (68∘00′ N, 15∘00′ E). Pebbles of both expeditions were transferred in large lid-covered petri dishes and used as stock cultures for all observations (see Wollenburg et al., 2018, for handling of the stock cultures). From these stock pebbles, specimens with strong cytoplasm staining were detached with a cactus-spine under a stereomicroscope, temporarily stored in small (ø 3 cm) seawater-filled petri dishes in the cold laboratory, and then transferred into the high-pressure aquaria.
High-pressure culturing observations on C. pachyderma were performed from February to May 2017 (Wollenburg et al., 2018), observations on C. lobatulus and C. wuellerstorfi from August to October 2018, and confocal microscope investigations from October to December 2020.
For this study, a total of 200 L sterile-filtered (0.2 µm mesh) North Sea water was adjusted to a salinity of ∼ 35, by addition of 1 g Marine Hobby sea salt per litre and practical salinity unit (psu) offset, and to a pH of 8.0 under atmospheric pressure. The normal culture seawater (160 L) was tagged with calcein (4,5-bis((N,N-bis(carboxymethyl)amino)methyl)fluorescein) (200 mg/L) to allow for identification of newly precipitated calcite (Wollenburg et al., 2018). To observe ectoplasmic structures under fluorescence light (excitation wavelength of 470 nm, emission wavelength > 490 nm) required rinsing the aquaria with unlabelled seawater from the remaining sterile-filtered batch of 40 L. This was done every 2–3 weeks for 2 d. Tagged and non-tagged seawater was stored in multiple 10 L Schott glass bottles with Bola connections in a cold room and refrigerator running at 2.5 ∘C. A high-pressure pump (ProStar218, Agilent Technologies) was used to supply a continuous one-way isobaric and isocratic seawater flow through the serially arranged aquaria running at an experimental pressure of 115 bar. Weekly, with a second high-pressure pump, 0.005 mg of dried Chlorella and Spirulina algae dispersed in seawater were pumped in each individual aquarium containing foraminifera (Wollenburg et al., 2018).
Cibicidoides specimens and the development of momentary and durable ectoplasmic extensions were observed under a Zeiss Axio Zoom V16 microscope, and pictures were taken with an Axiocam 506 colour camera.
In 2019, 1 to 3 d long high-pressure (100 bar) fluorescence measurements with C. lobatulus were performed. For these investigations, C. lobatulus specimens from the 2018 stock were transferred in a ∼ 10 mL aquarium with windows on both sides and installed in a portable cooling table running at 1.5 ∘C. A volume of 0.6 mL/min of non-labelled culturing water was directed through the high-pressure aquarium. For examination, a Confocal Leica TCS SP5 II equipped with a HCX PL Fluotar objective (10x 0.30) and an argon laser (λex = 488 nm) was used. Fluorescence emission was measured at 494–504 nm. The assessment and evaluation of the images were done with the software LAS AF Lite (Leica Camera AG). A stock solution of BCECF-AM (2′,7′-bis(2-carboxyethyl)-5-(and-6)-carboxyfluorescein acetoxymethyl ester) in DMSO (1 mg/mL in dimethylsulfoxide) was mixed and stored at −20 ∘C. Prior to the staining procedure, control observations were made to check for foraminiferal autofluorescence. At used microscope settings there was no autofluorescence of C. lobatulus specimens prior to staining. For incubation, the selected specimens were transferred into a petri dish with 2 mL seawater and exposed to 5 µmol/L_BCECF-AM. The incubation medium was then gently stirred with a small brush to distribute the dye evenly. The petri dish was covered and stored at 4 ∘C for 19 h (incubation time). The properties of BCECF-AM allow us to conduct a non-terminal live–dead screening procedure (Bernhard et al., 1995). The nonfluorescent membrane permeable BCECF-AM enters an organism and has to be converted to fluorescent BCECF via intracellular hydrolases; thus, the cell has to be alive to exhibit fluorescence. After incubation, specimens were transferred into the high-pressure aquaria and gradually adjusted to a pressure of 100 bar over a period of 6 h. The observations were conducted right after the aimed pressure was reached, after 24 h, and after 48 h. The settings from the control measurement were used to record the fluorescence activity in the cytoplasm of the C. lobatulus specimens. As the Cibicidoides test proved to be too thick to be penetrated by the argon laser, only ectoplasmic features could be investigated with the confocal microscope.
As the refraction index of foraminiferal cytoplasm approximates that of water, pseudopodia and other cytoplasmic extensions are usually observed with inverted microscopes once they are in contact with or close to the thin glass bottom of the observational dishes (e.g. Bowser and Travis, 2002; Cedhagen and Frimanson, 2002; Röttger, 1982; Travis et al., 2002). High-pressure culturing requires a thick glass and a certain interior aquarium height, in our case both measuring 4 mm. In these aquaria thin pseudopodia could only be observed occasionally when a specimen positioned itself or the respective ectoplasmic structure close to the aquarium's window. Therefore, our results do not comprise a comprehensive documentation of the fine branched parts of the pseudopodial network but essentially of the thicker ectoplasmic structures.
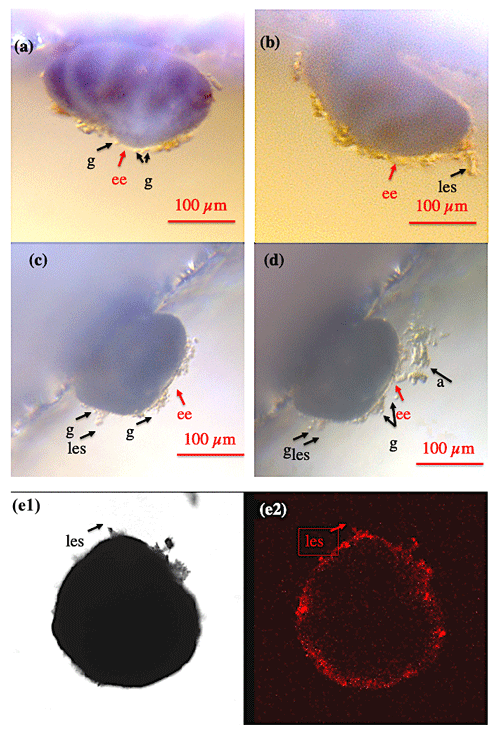
Figure 1Shell envelope I. (a–b) Shell envelope (ee) of a Cibicidoides pachyderma specimen revealing multiple granule (g) and initial static ectoplasmic structures (les). (c–d) Shell envelope of a C. pachyderma specimen 24 h before (c) and during feeding (d). During feeding multiple mobile granule and attached algae (a) indicate a pseudopodial network presumably originating in the shell envelope. (e-1–e-2) BCECF-AM incubated C. lobatulus specimen viewed under normal transmitted light (e-1) and laser excitation exhibiting the BCECF-AM fluorescence (e-2). As C. lobatulus specimens possess a thick shell, only the shell envelope, an initial lasting ectoplasmic structure (les), and especially granule reveal bright red fluorescence.
3.1 Shell envelope
At all times, all Cibicidoides tests were covered by a thin to thick continuous layer of ectoplasm (envelope), making the shell an internal rather than an external cellular structure (Figs. 1–2). The shell envelopes showed numerous granules and in this respect resembled the appearance of pseudopodia (Fig. 1a–d). Although at an extremely low speed (significantly less than < 10 µm per 10 min), the envelope-inherent granules gradually changed their position over time. A coherent ectoplasmic structure of the shell envelope is corroborated by BCECF-AM staining/confocal microscope analyses (Fig. 1e1–e2). Extension of pseudopodia from the shell envelope became apparent when algae adhered to these filaments during feeding (Fig. 1c–d), whereas hours to days after feeding a significant portion of the fed algae were found covering parts of the shell envelope. We assume that the shell envelope initiates the formation of the agglutinated cyst that covers Cibicidoides tests during shell precipitation/growth or in waters of low pH (De Nooijer et al., 2009; Wollenburg et al., 2018). Similarly, a pure algae half cyst formed during a period of 6 weeks on the spiral side of an adult C. lobatulus (Fig. 2a–b). Figure 2a shows a bright shell envelope covering the umbilical side of the specimen and the algae cyst with ectoplasmic contributions on the spiral side. After 6 weeks, the half cyst was shed but still showed parts of what we assume to be ectoplasmic remains (Fig. 2b). Occasionally (n=2) also abandoned ectoplasmic envelopes were observed, supporting the idea that the cytoplasmic envelope serves as matrix for the cyst formation (Figs. 2c–d).
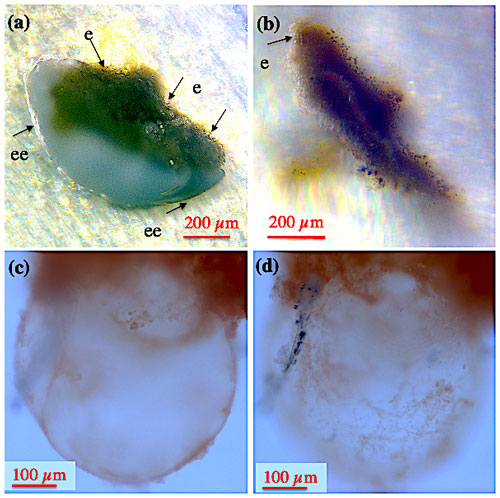
Figure 2Shell envelope II. (a) Shell envelope apparent on the umbilical side of an adult C. lobatulus specimen, whereas an algae half cyst was formed over a period of 6 weeks over the spiral side. (b) The half cyst 1–2 d after it has been abandoned (cyst was shed during the weekend). (c–d) Abandoned shell envelope of a C. pachyderma specimen retrieved after the termination of the Cibicidoides pachyderma experiment. Panels (c) and (d) show the same cyst but different focussing. “ee” denotes ectoplasmic envelope; “e” denotes remains of ectoplasm.
3.2 Static ectoplasmic structures
Within 24 h after transfer into the aquaria and adjustment to a pressure of 115 bar, the first type of thick static ectoplasmic structures, ectoplasmic “roots”, appeared in about 50 % of juvenile and most adult specimens (Figs. 3–9). In 68 out of 100 specimens ectoplasmic roots were observed. In an unknown proportion of the rest (32 specimens), such structures might have existed but due to the large working distance and/or a less optimal observational position of the specimens in the aquarium were not noticed. Juvenile Cibicidoides specimens were more mobile than adults (Wollenburg et al., 2018), and likely, therefore, the formation of ectoplasmic roots was often delayed. Three days and 2 weeks after transfer, the first ectoplasmic “twigs” and “trees”, respectively, were formed, directing into the water column. All static ectoplasmic structures may have shown continued growth but otherwise changed little over the 3 months of observation. In one case braided ectoplasmic roots even persisted after the termination of the experiment when the two involved specimens were rinsed in deionized water and dried (Fig. 5g). We never observed that these structures were in whole or in part resorbed.
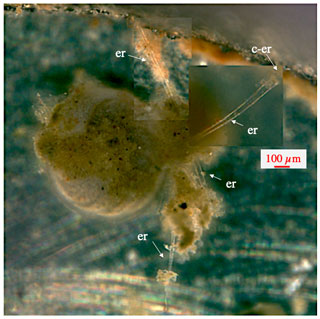
Figure 3Ectoplasmic roots of C. wuellerstorfi. Starting with the lower ones, ectoplasmic roots were developed over a period of 1 week and remained unchanged for the remaining 5 weeks of the experiment. “er” denotes ectoplasmic root; “c-er” denotes contact zone of ectoplasmic root with the aquarium glass.
3.2.1 Ectoplasmic “roots”
The most frequent static ectoplasmic structures were root-like, extending along the bottom or adhering to the window of the aquarium (Figs. 3–5). Where the ectoplasmic root came close to the aquarium glass, thereby reducing the distance to the microscope objective, pseudopodia and bidirectional streaming on the outside of the respective ectoplasmic root could be observed (Fig. 4). Ectoplasmic roots were attached to the aquarium glass via thickened endings (Figs. 3–4). The typical ectoplasmic root had a mean thickness of roughly 30 µm, and often two roots were twisted to form thicker braid-like structures (Fig. 5). Presumably limited by the dimension of our aquaria, a maximum root length of roughly 5 mm was observed (Figs. 4–5). Over the course of the experiments, the number of ectoplasmic roots increased and some showed ongoing growth (Fig. 4). Figure 5a shows a twisted ectoplasmic root with a total length of 400 µm on the left and a shorter straight root of approx. 100 µm on the right side of C. pachyderma specimen 1 (Sp. 1). Both structures had formed in the course of a night. During the following day, Sp. 1 flipped over so that the test periphery was facing the aquarium floor and moved to the filter ring. There the smaller single ectoplasmic root continued to grow and branch (Fig. 5b–c). Finally, this ectoplasmic root of Sp. 1 combined with the ectoplasmic root of a neighbouring specimen (Sp. 2) and formed a single braid-like ectoplasmic root (Fig. 5d). For the remaining 2 months, the two individuals moved along this braided root like on rails and positioned themselves sometimes closer to and sometimes further away from each other. Hereby, specimen 2 remained under the filter ring for most of the time.
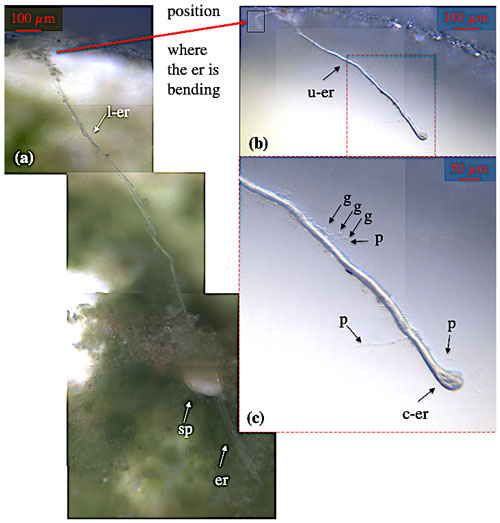
Figure 4Ectoplasmic roots of C. lobatulus. (a) Cibicidoides lobatulus specimen (sp) embedded in algae with two ectoplasmic roots (er) extending on the bottom of the aquarium. At one point, the northern ectoplasmic root bends upward at the aquarium's wall; thus, it is differentiated in a lower (l-er) and an upper (u-er) part. Panel (b) shows the upper part of the northern ectoplasmic root. Panel (c) shows the u-er at higher magnification revealing granule (g), pseudopodia (p), and a broad contact zone (c-er) where the ectoplasmic root is attached to the aquarium's window.
3.2.2 Ectoplasmic “trees”
Thick, robust, and permanent ectoplasmic structures, very similar to ectoplasmic roots but extending into the water column, were termed ectoplasmic “trees”. Tree-forming Cibicidoides pachyderma specimens could climb up these structures to raise themselves above the bottom. Interestingly, similar structures were not observed in any of the investigated C. lobatulus and C. wuellerstorfi specimens. Distinct ectoplasmic trees were observed in 6 of the 50 studied C. pachyderma specimens; others might have been overlooked as the experimental set-up just allows a vertical view of the aquarium.
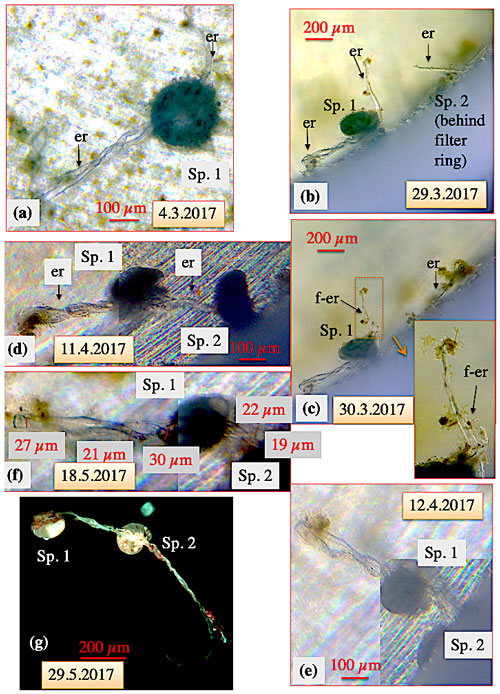
Figure 5Ectoplasmic roots of C. pachyderma (specimens 1 and 2). (a) Six days after being transferred into the high-pressure aquarium, overnight a twisted ectoplasmic root formed on the left and a short simple root on the right side of the test of specimen 1 (Sp. 1). (b) Thereafter, Sp. 1 moved towards the filter ring and finally positioned itself close to an ectoplasmic root of specimen 2 (Sp. 2; situated under the filter ring) on 29 March. (c) The next day, the right-hand ectoplasmic root of specimen 1 started to fray. (d) Several days later, during a weekend, specimen 2 resurfaced from below the filter ring and its left-hand ectoplasmic root was combined with the frayed right-hand root of specimen 1 to a joined twisted or braided ectoplasmic root. (e) The joined braided ectoplasmic root of specimens 1 and 2 (positioned under the filter ring) on 12 April. (f) Thickness measurements of the joined braided ectoplasmic root. (g) Fluorescence picture of the braided ectoplasmic root of Sp. 1 and 2 immediately after termination of the experiment (excitation wavelength 470 nm, emission wavelength 490 nm). The emitted bright greenish calcein fluorescence of the ectoplasmic root likely indicates recent cytoplasmic activity. “er” denotes ectoplasmic root; “f-er” denotes frayed ectoplasmic root. After termination of the experiment, gently washing the specimens over a 30 µm mesh, and drying the residue, both specimens were still attached via the joined braided ectoplasmic root with a final length of at least 5 mm (g).
Whereas ectoplasmic roots were eventually formed within 24 h after transfer into the aquaria, it took about 2 weeks before the first ectoplasmic trees were formed (Fig. 6). Rather than moving with the foraminifera, as described for the roots of some specimens, ectoplasmic trees were fixed in the aquaria. They reached a maximum height of approx. 2 mm, and the foraminifera could climb freely along these tree-like structures (Fig. 6a–c). Regularly spaced short and obviously adhesive side branches (Fig. 6a), probably with tiny pseudopodia (that are rarely visible in our set-up), collected suspended algae from the inflow current during feeding. As a result ectoplasmic trees looked like loosely agglutinated structures later in the experiment (Fig. 6c-1–c2).
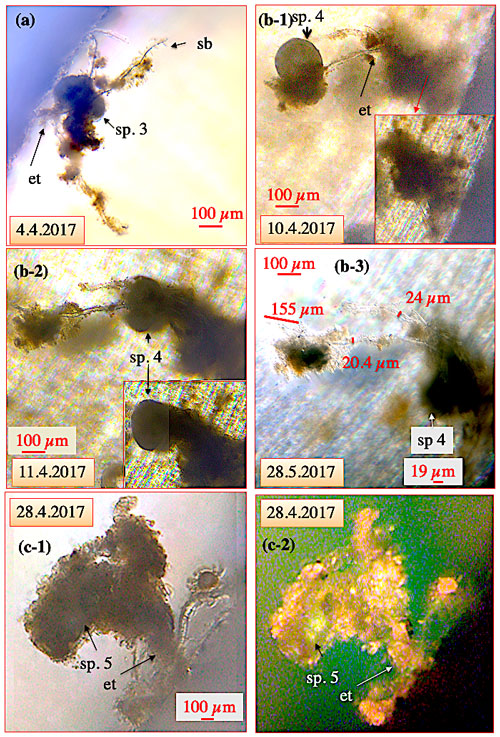
Figure 6Ectoplasmic trees of C. pachyderma. (a) Ectoplasmic tree of C. pachyderma specimen (sp.) 3 with three thick branches originating from a single “stem” fixed to the aquarium wall. Cibicidoides pachyderma sp. 3 was positioned approx. 100 µm away from the wall with no contact to the bottom of the aquarium. (b-1–b-3) Ectoplasmic tree of C. pachyderma sp. 4 fixed to the aquarium's bottom and extending at least 2 mm into the water column. (b-1) On 10 April, specimen 4 had climbed to the top of the ectoplasmic tree. (b-2) The next day, the specimen had moved to the middle section of the ectoplasmic tree. Panel (b-3) shows, as an example, specimen 4 at the bottom of the ectoplasmic tree on 28 May. Furthermore, thickness measurements on the tree structures are provided. (c-1–c-2) Ectoplasmic tree of C. pachyderma sp. 5. Algae adhering to the adhesive side branches of the ectoplasmic tree obscure the ectoplasmic nature when viewed under normal light (c-1). Panel (c-2) shows the same ectoplasmic tree under fluorescent light, allowing a better visibility of the tree and the specimen's position. The bright greenish calcein fluorescence of the cytoplasm illustrates the elevated position of specimen 5 within the accumulated algae. “et” denotes ectoplasmic tree; “sb” denotes side branches.
3.2.3 Ectoplasmic “twigs” and pseudopodial network
Thick ectoplasmic structures extending into the water were termed ectoplasmic “twigs” if the shape and position with respect to the test remained essentially permanent during the experiment (Figs. 7–8). However, ectoplasmic twigs are the least static of the three described ectoplasmic structures and were only observed in C. pachyderma specimens so far. Ectoplasmic twigs are directed above the umbilical side into the water column; thus, in our experiments they could only be observed in specimens that had attached themselves on an, in respect to the observation, ideal position on the aquarium's wall. In 16 of the 50 observed C. pachyderma specimens, ectoplasmic twigs were observed. The first ectoplasmic twigs appeared 3 d after transfer of C. pachyderma specimens into the aquaria (Fig. 7a). Additional structures were eventually added over time (Fig. 7a, b), but the original structure was usually not modified (Figs. 7–8). Provided with the same short and obviously adhesive side branches as ectoplasmic trees (Fig. 6), the ectoplasmic twigs probably support a more delicate pseudopodial network (Figs. 7–8). In our experiment, C. pachyderma specimens exhibited a strong rheotaxis. In this context it was observed that a specimen had positioned itself at the hole of the filter ring (where the food entered the aquarium). After this position was occupied the specimen developed a series of crescent-shaped ectoplasmic twigs (Fig. 8). From the area in which the ectoplasmic twigs were developed, the species directed an anastomosing pseudopodial network into the inflowing water current during feeding (Figs. 8–10). In doing so, the instrumentally visible collection area increased the specimen's test size by at least 20 times. Hereby, both the pseudopodial network and the respective supportive ectoplasmic twigs obviously allowed the animal to collect food from the water current (Figs. 8–10). When we shut down the pumps and, thus, the current activity for some minutes (on 26 May 2017, 25 h after feeding), the pseudopodial network, visualized by adhering algae, collapsed (Fig. 8b), whereas the ectoplasmic twigs kept their original shape (Fig. 8). The shape of the specimen's ectoplasmic twigs was affected neither by the presence or absence of the current nor by the speed of it (∼ 0.1–5 cm/min, Wollenburg et al., 2018).
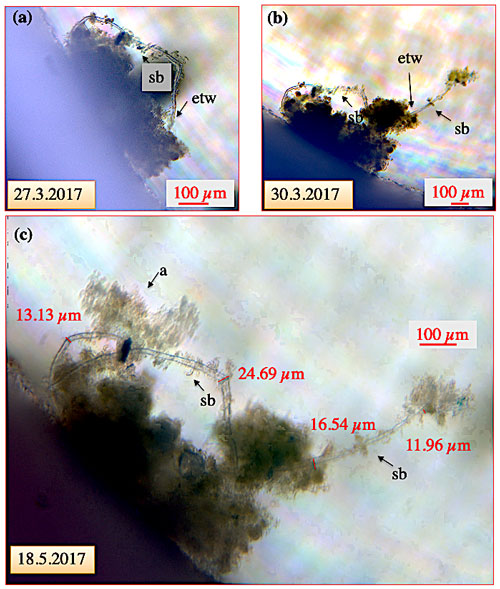
Figure 7Ectoplasmic twigs of C. pachyderma specimen 8. (a) For 3 d, the specimen had gathered algal detritus around its shell envelope and simultaneously developed a loop-like ectoplasmic twig with a total length of ∼ 700 µm from the periphery to the opposite side. (b) Three days later, an ∼ 500 µm measuring extension directing into the water column was added to the loop-like twig. Both structures persisted for the remaining weeks of the experiment. (c) On 15 May, dispersion of algae into the aquarium allowed the specimen to collect additional algae onto the ectoplasmic twig. The algae mass remained in this position and was not ingested during the experiment. “etw” denotes ectoplasmic twig; “a” denotes algae; “sb” denotes side branch.
For the specimen positioned at the hole in the filter ring, the development and extension of pseudopodia directing into the water current during feeding was immediate (Fig. 10); however, the transport of collected algae towards the shell was extremely slow. Seven hours after feeding, algae were still sticking to the pseudopodia and ectoplasmic twigs, and no or only low amounts of fresh algae had reached the shell interior (Fig. 10f). Slow food ingestion was also reflected by the extremely slow propagation of anastomoses over time. An anastomosis propagated less than 150 µm within 24 h (Fig. 10). During and following feeding, the number of granules in the ectoplasmic envelope, the ectoplasmic twigs, and pseudopodia were significantly increased.
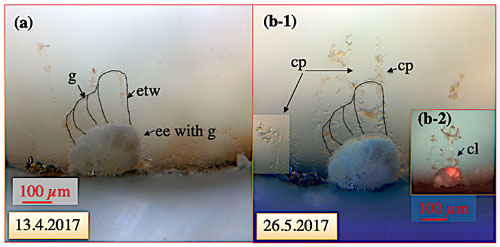
Figure 8Crescent-shaped ectoplasmic twigs of C. pachyderma specimen 1 positioned at the hole of the sinter ring, i.e. at the inflow of water and algal food into this aquarium. (a) Specimen viewed under normal light when no food was added to the inflow revealing bow-like ectoplasmic twigs. (b) Thirty-five days later, the pumps were stopped1 to investigate the stability of the ectoplasmic twigs and the pseudopodial network at zero current activity but stable high-pressure conditions. Stable ectoplasmic twigs and collapsed pseudopodial (cp) network under normal light (b-1) and fluorescent light (b-2). The red colour of especially older test parts results from ingested Spirulina and Chlorella algae stored in food vacuoles of the cytoplasm. “ee” denotes ectoplasmic envelope; “etw” denotes ectoplasmic twig; “g” denotes granule; “cl” denotes calcein-stained cytoplasmic lacuna in the etw and cp. 1 A shut-off valve following downstream the overflow valve prohibited a pressure drop in the high-pressure aquaria when the pumps were shut.
3.2.4 Torn ectoplasmic remains
When Cibicidoides specimens that were virtually sessile for weeks changed position, their static ectoplasmic structures could obviously not be resorbed. These structures were either pulled along by the specimens, as shown for the ectoplasmic roots in Fig. 5, or torn off. Over the duration of the experiment, numerous ectoplasmic roots and twigs, or what is supposed to be parts of such structures, were flushed to the aquarium's window (Fig. 11). We had to increase the current speed through the aquaria sporadically to get rid of the torn biomass and clear the view. When we opened the aquaria after termination of the experiments, we found torn ectoplasmic roots with no signs of shrinking or collapsing. Since static ectoplasmic structures can obviously not be resorbed, any relocation is accompanied by material loss for a specimen.
It was also observed that algae (dispersed from the water inflow) adhering to the static ectoplasmic envelope, twigs, trees, and less marked roots, remained almost at the same position throughout the experiment or until the respective structure was torn off (Figs. 6–7).
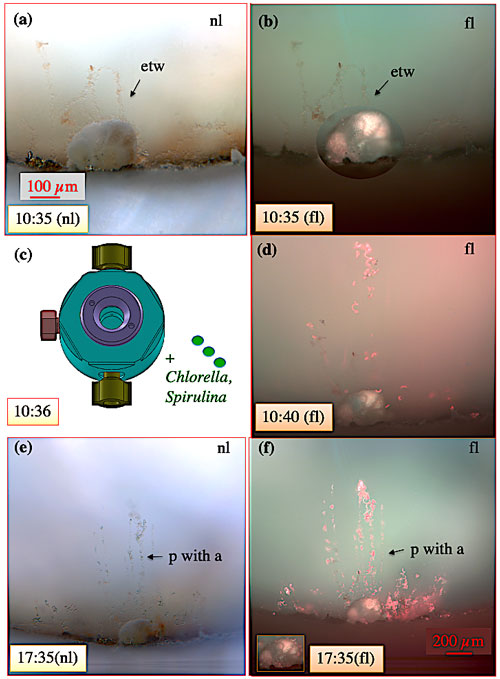
Figure 9Pseudopodial network of C. pachyderma specimen 1 during feeding on 13 April 2017. Specimen 1 before, during, and after feeding with 0.5 µg dried Spirulina and Chlorella algae. The bright red colour of dispersed algae under fluorescent light provides an excellent tool to document the passage and uptake of algae in the pseudopodia and cytoplasm. (a–b) Specimen 1 prior feeding. (c) Schematic illustration of the aquaria indicating the start of feeding. (d) Specimen 1 during feeding. (e–f) Seven hours after feeding. “etw” denotes ectoplasmic twig; “p” denotes pseudopod; “a” denotes algae; “nl” denotes normal light; “fl” denotes fluorescence light. Numbers state the respective time on 13 April.
4.1 Ectoplasmic envelope
This study describes the shell of Cibicidoides spp. as an internal “skeleton” rather than an external feature. Already in Schultze's work from 1854 (Schultze, 1854) an ectoplasmic sheet can be suspected to cover the illustrated Elphidium macellum (as Polystomella strigilatum) test plate IV, Fig. 1). Cushman (1928) even stated that in many foraminifera the shell would be an internal one. But he did not list any species in which this is the case. In studies on foraminiferal calcification processes in planktonic foraminifera, Spiroloculina hyalina, Ammonia sp., and Amphistegina lessonii, a protective cytoplasmic envelope is described as a structure restricted to times and areas when/where new shell material is precipitated (Angell, 1980; Bé et al., 1979; de Nooijer et al., 2014; Erez, 2003; Tyszka et al., 2019). In our observations, an ectoplasmic envelope covered the tests of the investigated Cibicidoides specimens at all times, and for shell growth a supplementary surrounding sediment cyst had to develop (Wollenburg et al., 2018). Thus, it is currently unclear whether a permanent ectoplasmic envelope as we have observed it for Cibicidoides spp. is developed in only some foraminifera taxa or has simply been overlooked in others. The ectoplasmic sheet described for Heterostegina depressa (Röttger, 1973, 1982) visually resembles the sheets surrounding Cibicidoides specimens and as in our experiments had to be during rapid relocation.
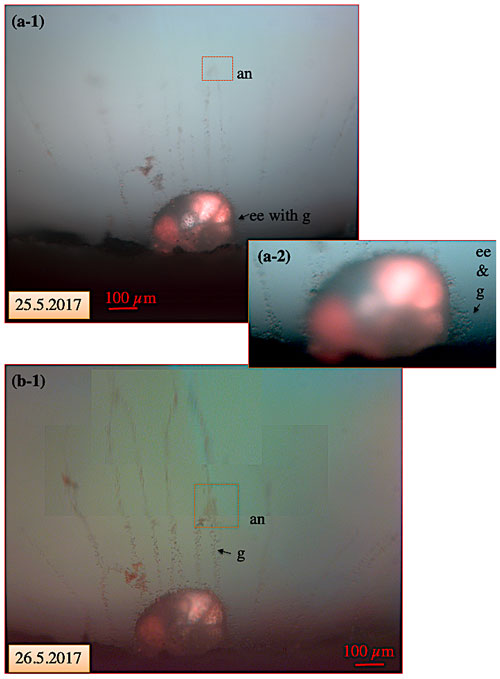
Figure 10Pseudopodial network of C. pachyderma specimen 1 under fluorescent light on 25 and 26 May. Movement of an anastomosis within 24 h after feeding. (a-1) In the course of the experimental running time, a visually increasing amount of algae (intensified red colour of cytoplasm; compare to Fig. 9) had accumulated in the specimen's cytoplasm. A red square indicates the position of a slowly moving anastomosis in the pseudopodial network. Panel (a-2) shows the test at higher magnification revealing the presence of numerous granules in the ectoplasmic envelope and twigs. (b) Twenty-four hours later, the anastomosis had moved by approximately 150 µm towards the shell. “an” denotes anastomosis; “ee” denotes ectoplasmic envelope; “g” denotes granule.
4.2 Ectoplasmic extensions – pseudopodial network
Only for a few shallow-water benthic foraminifera, information on ectoplasmic extensions to interact with the environment has been published (Bowser and Travis, 2002; Travis et al., 2002). Hereby, the typical ectoplasmic extensions described are pseudopodia characterized by their forceful and rapid extension enabled by actin filaments and extremely dynamic microtubule systems (Bowser et al., 1988; Goleń et al., 2020; Travis and Bowser, 1986; Travis et al., 2002). Anastomosing, i.e. the fusing of two neighbouring pseudopodia, is abundant and rapidly propagating. Furthermore, a rapid bidirectional transport of both granules and surface-attached particles has been described for the pseudopodia of shallow-water foraminifera. Giving tribute to the granular appearance, the term “granuloreticulopodia” is widely used for this pseudopodial network and separates it from the globular and lamellar pseudopodia involved in chamber formation (Goleń et al., 2020; Tyszka et al., 2019).
Our study shows that at in situ pressure the pseudopodial network of the examined Cibicidoides taxa extends into the water current and exhibits branching and anastomoses, resembling the pseudopodial network of shallow-water foraminifera. However, in the investigated specimens, granules, anastomoses, and attached particles moved very slow and could be observed for hours, sometimes even days or weeks, with little noticeable movement (Figs. 9–10). In C. pachyderma sp. 1 of Figs. 8–10, for example, it took about 6 weeks before a significant ingestion of dispersed algae inside the shell could be noticed (Figs. 9–10).
The rate at which cells can form projections, like pseudopodia, and transport granules and adhering particles is, in part, limited by the rate at which the cell assembles new or reorganizes existing actin filaments (Bowser et al., 1988; Goleń et al., 2020; Travis and Bowser, 1986; Travis et al., 2002; Tyszka et al., 2019). This ATP-consuming process is obviously much faster in shallow-water foraminifera than in deep-water Cibicides and Cibicidoides taxa. Presumably due to the large working distance in our high-pressure aquarium set-up, fluorescent SiR-actin labelling failed in our confocal studies so far. Therefore, we can just speculate that the ATP demand to form pseudopodia and perform bidirectional streaming increases with hydrostatic pressure and/or at sites of high current activity.
4.3 Ectoplasmic extensions – permanent extensions
Besides pseudopodia, this study describes for the first time non-retractable static ectoplasmic structures that, depending on their characteristics, were named ectoplasmic roots, trees, and twigs. Ectoplasmic roots developed in most specimens and all species investigated. Hereby, a minimum of two mutually opposing ectoplasmic roots developed soon after the start of the experiments. However, over the course of the experiments, the number of ectoplasmic roots increased, and most showed ongoing growth. Ectoplasmic roots are long branchless structures extending along the bottom or adhering to the window of the aquarium. Together with pseudopodia emerging from the ectoplasmic root, these structures likely act as anchors to stabilize the foraminiferal shell in an area of high current activity. Ectoplasmic roots are likely the “naked” variant of the agglutinated tubes of C. lobatulus described from shallow-water occurrences (Nyholm, 1962). We assume that similar to the sedimentary cyst covering the ectoplasmic envelope (see above), deposition of current-collected sediment particles on top of ectoplasmic roots leads to an increased robustness and protection of these structures.
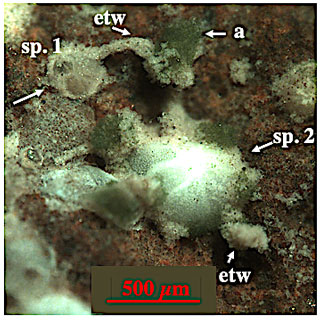
Figure 12Epilithic C. lobatulus specimen from off Svalbard. A joined “agglutinated” tube, here equated with ectoplasmic twigs, is developed between specimen 1 and 2. Algae are accumulated halfway down the tube. “etw” denotes ectoplasmic twig; “a” denotes algae. Picture courtesy of Julia Wukovits (September 2020).
Ectoplasmic trees are thick, robust, and branching structures that, other than roots, direct into the water column (Fig. 6). Over the course of weeks in the experiments, ectoplasmic trees were only formed by C. pachyderma specimens. Fixed to the aquarium bottom, these protruding structures reached heights of around 2 mm. Ectoplasmic trees likely serve as scaffolding on which the foraminifera can modify or optimize its position with respect to the prevailing current.
Ectoplasmic twigs are thick structures extending into the water column whose shape and position with respect to the specimen's test remain largely unchanged. However, they are the least static ones of the three described ectoplasmic structures. Ectoplasmic twigs are perhaps a stabilizing and protective framework that maintains a delicate pseudopodial network when distributed into a current. However, further studies are required to prove our assumptions. In our high-pressure experiments, ectoplasmic twigs were only observed in C. pachyderma specimens, yet recent observations on shallow-water C. lobatulus show “agglutinated” tubes directing into the water column (Fig. 12) that resemble ectoplasmic twigs. In Fig. 12 we see a joint “agglutinated” tube between specimen 1 (juvenile C. lobatulus) and 2 (adult C. lobatulus) with freshly (picture was taken following a feeding experiment) accumulated algae halfway. On specimen 2 a second “agglutinated” tube directs into the water column. From our experience with cyst formation and algae aggregation, we assume that these “agglutinated” tubes are sediment-covered ectoplasmic twigs. Whether C. lobatulus just develops ectoplasmic twigs at shallow-water/low-pressure sites or whether they were too thin to be detected with our instrumental set-up in our experiments with this species remains unclear. However, the picture of these freshly fed shallow-water C. lobatulus specimens supports our assumption that the formation of rigid ectoplasmic twigs assists a food-gathering pseudopodial network.
We observed that static ectoplasmic structures did not change in response to current speed and that they could not be resorbed or retracted. It was also observed that algae (dispersed from the water inflow) adhering to the static ectoplasmic envelope, twigs, trees, and less marked roots remained almost at the same position throughout the experiment or until the respective structure was torn off (Figs. 6–7). This might suggest that, in the absence of sediment particles in the current, the foraminifera try to stabilize lasting ectoplasmic structures by the continuous accumulation of algae (see also below).
In the field, the pseudopodial network of C. antarcticus is assumed to be guided by agglutinated tubes extending from the foraminiferal shell into the water column (Alexander and DeLaca, 1987; Hancock et al., 2015). In our experiments the ectoplasmic trees and twigs accumulated algae over time but likely would also have accumulated sediments if provided by the inflowing current. Hypothetically, accumulation of sediment particles on ectoplasmic twigs and trees over longer periods could result in structures that resemble the agglutinated tubes described for C. antarcticus (Alexander and DeLaca, 1987) or shallow-water C. lobatulus (Fig. 12).
The tubes of C. antarcticus are made up of silt- and clay-sized minerals, diatom frustules, fine organic detritus, and occasionally sponge spicules. However, although described as agglutinated structures, the tubes collapsed when the respective foraminifera was taken out of the water (Alexander and DeLaca, 1987). As no analyses on the particle combining cement were made, it is quite possible that the described agglutinated tubes are sediment-covered ectoplasmic structures. In our study provided artificial quartz substrate was not used for agglutination or accumulation on the static ectoplasmic roots, trees, or twigs, whereas dispersed algae were collected from the inflowing current and deposited on these structures. As we had no dispersed minerals in the circulating current, it can only be assumed that they would also adhere to the lasting ectoplasmic structures described.
4.4 Ectoplasmic extensions – biological and evolutionary aspects of permanent extensions and outlook for future research
Bowser and Travis (2002) speculated that evolutionarily the pseudopodium may have derived from the eukaryotic flagellum because nearly all foraminifera possess flagellated gametes (Goldstein, 1999). Both flagella and pseudopodia rely on microtubules as a supporting and locomotive framework. Flagella possess an elaborate cross-linking apparatus designed to produce a highly regulated bending form, whereas in shallow-water foraminifera microtubules are constantly transported within the tethered framework of pseudopodia, allowing a less rigid but highly flexible motile function. Although, pseudopodia emerged from the static ectoplasmic structures, due to the stiffness of roots, trees, and twigs, they rather resemble flagella than pseudopodia. Yet, future transmission electron analyses or confocal microscope investigations at atmospheric pressure (Goleń et al., 2020; Tyszka et al., 2019) are needed to understand the cellular structure of these lasting ectoplasmic extensions. Application of fluorescent dyes for confocal microscope investigations in high-pressure aquaria is often limited by the large working distance hampering for example a noticeable emission from SiR-actin labelling.
The static ectoplasmic features described are long-lasting and, thus, presumably energy saving structures of taxa living under significant hydrostatic pressure and current activity. They likely anchor the specimen at low energetic costs in a highly turbulent environment. Furthermore, twigs and trees likely protect a delicate pseudopodial network that, in a habitat with unpredictable food supply, has to be immediately developed and extended. However, movement of anastomoses, adhering algae, and bidirectional streaming in the pseudopodial network were extremely slow during our observations, suggesting a much slower ingestion time than has been described for shallow-water foraminifera (Bowser et al., 1984; Bowser and Travis, 2002; Wollenburg et al., 2018). This may be the reason why, for example, C. wuellerstorfi in the Nordic Seas and Arctic Ocean is restricted to times and areas of high food supply but is insensible to sudden primary production/carbon export pulses (Wollenburg and Kuhnt, 2000; Wollenburg et al., 2001; Wollenburg and Mackensen, 1998a).
This is the first report investigating ectoplasmic structures and dynamics in Cibicidoides species under in situ pressure. In the present study, a protective ectoplasmic envelope completely covered all Cibicidoides shells at any time, suggesting that the shell is an endo- rather than ectoplasmatic feature.
Our further findings indicate that the life of these deep-sea foraminifera is characterized by energy-saving, long-lasting, static ectoplasmic structures that allow these rheotactic species to position themselves at sites of high current activities. Roots are thick and robust ectoplasmic structures that anchor the specimens on current exposed substrates. They might continue to grow but otherwise could not be reshaped. Ectoplasmic trees are stationary structures that are directed into the water column, allowing the foraminifera to climb this structure and thereby elevate itself above ground.
Ectoplasmatic twigs provide a supportive rigid framework from which or around which a delicate food-gathering pseudopodial network emerges.
When the specimen changed its location, the stationary ectoplasmic trees and one or the other ectoplasmic root were torn off. Thus, relocation is associated with a loss of ectoplasm and an additional energy demand required for the formation of new lasting ectoplasmic structures to secure the specimen at its new location. Whereas the deployment of a pseudopodial network into an inflowing current with algae is immediate, the propagation of collected algae towards the shell is extremely slow. Perhaps for this reason Cibicidoides taxa are poor indicators of primary production pulses.
We assume that the static shape and slow remodelling of trees, twigs, and roots as well as the slow formation of anastomoses and surface transport arises from an adaptation to a high-current-activity habitat with unpredictable food fluxes driven by energetic optimization. This assumption and the possibility of a different microtubule system in deep-sea pseudopodia have to be addressed in future studies.
All data supporting the results of this article are shown in the figures and tables of the article.
JEW designed and performed large parts of the research, analysed the data, cultured the foraminifera, wrote the paper, and prepared the graphics. ZMCZ assisted in the high-pressure experiments and observations under the Axio Zoom and assisted in writing. CC and UB carried out the confocal microscope investigations and assisted in writing. JB assisted in writing.
The authors declare that they have no conflict of interest.
Publisher’s note: Copernicus Publications remains neutral with regard to jurisdictional claims in published maps and institutional affiliations.
We thank the chief scientist Antje Boetius and the captain and crew of RV Polarstern for their fantastic work during cruise PS101. Special thanks go to Linn Schmidtman, who did a wonderful job in collecting the foraminiferal material during this expedition. A big thank you goes to Erik Wurz, who collected stones with foraminifera during the RV G.O. Sars expedition GS2018108 (July–August 2018) for this study. During her stay at the AWI Julia Wukovits took the wonderful picture of living C. lobatulus that she allowed us to use in this publication. We are extremely grateful for the excellent work of the AWI workshop and especially to Erich Dunker, who was always at our side designing and redesigning the high-pressure aquaria and most of the corresponding equipment. We thank Johannes Lemburg, who in 2018 replaced Erich Dunker and designed the wonderful confocal high-pressure aquaria and supportive systems.
The valuable comments of the Susan Goldstein and an anonymous reviewer helped to improve the manuscript. We thank them for their effort and kind remarks.
This study was funded by a DFG grant to Jutta E. Wollenburg (WO742-2), a joint DFG–ANR grant
to Jelle Bijma (project B2SeaCarb; BI 432/10-1) and the CoPter project (AWI
Strategiefond), and through the SponGES “Deep-sea Sponge Grounds Ecosystems of
the North Atlantic: an integrated approach towards their preservation and
sustainable exploitation” project, under H2020 – the EU Framework Programme for
Research and Innovation (grant agreement no. 679849).
The article processing charges for this open-access publication were covered by the Alfred Wegener Institute, Helmholtz Centre for Polar and Marine Research (AWI).
This paper was edited by Hiroshi Kitazato and reviewed by Susan T. Goldstein and one anonymous referee.
Alexander, S. P. and DeLaca, T. E.: Feeding adaptations of the foraminiferan Cibicides refulgens living epizoi-cally and parasitically on the Antarctic scallop Adamussium colbecki, Biol. Bull., 173, 136–159, 1987.
Angell, R. W.: Test morphogenesis (chamber formation) in the foraminifer Spiroloculina hyalina Schulze, J. Foramin. Res., 10, 89–101, 1980.
Bé, A. W. H., Hemleben, C., Anderson, O. R., and Spindler, M.: Chamber formation in planktonic foraminifera, Micropaleontology, 25, 294–307, 1979.
Bernhard, J. M., Newkirk, S. G., and Bowser, S. S.: Towards a Non-Terminal Viability Assay for Foraminiferan Protists, J. Eukaryot. Microbiol., 42, 357–367, 1995.
Bowser, S. S. and DeLaca, T. E.: Rapid intracellular motility and dynamic membrane events in an Antarctic foraminifer, Cell Biol. Int. Rep., 9, 901–910, 1985.
Bowser, S. S. and Travis, J. L.: Reticulopodia: Structural and behavioural basis for the suprageneric placement of Granuloreticulosan protists, J. Foramin. Res., 32, 440–442, 2002.
Bowser, S. S., Israel, H. A., McGee-Russell, S. M., and Rieder, C. L.: Surface transport properties of reticulopodia: Do intra-cellular and extracellular motility share a common mechanism?, Cell Biol. Int. Rep., 8, 1051–1063, 1984.
Bowser, S. S., Travis, J. L., and Rieder, C. L.: Microtubules associate with actin-containing filaments at discrete sites along the ventral surface of Allogromia reticulopods, J. Cell Sci., 89, 297–307, 1988.
Cedhagen, T. and Frimanson, H.: Temperature dependence of pseudopodial organelle transport in seven species of foraminifera and its functional consequences, J. Foramin. Res., 32, 434–439, 2002.
Cushman, J. A.: Foraminifera; their classification and economic use, Cushman Laboratory for Foraminiferal, Research, Sharon, Mass., 491 pp., 1928.
De Nooijer, L., Toyofuku, T., and Kitazato, H.: Foraminifera promote calcification by elevating their intracellular pH, P. Natl. Acad. Sci. USA, 106, 15374–15378, 2009.
de Nooijer, L. J., Spero, H. J., Erez, J., Bijma, J., and Reichart, G. J.: Biomineralization in perforate foraminifera, Earth-Sci. Rev., 135, 48–58, 2014.
Dujardin, G.: Observations nouvelles sur les Céphalopodes microscopiques, Annales des Sciences Naturelles, Paris (Seconde Série, Zoologie), 3, 108–109, 1835a.
Dujardin, G.: Observations nouvelles sur les prétendus Céphalopodes microscopiques, Annales des Sciences Naturelles, Paris (Seconde Série, Zoologie), 3, 312–316, 1835b.
Dujardin, G.: Observations sur les rhizopodes et les infusoires, L'Académie des Sciences Paris, Comptes Rendus, 1, 343–377, 1835c.
Dujardin, G.: Recherches sur les organismes inférieures, Annales des Sciences Naturelles, Zoologie, 4, 347–377, 1835d.
Erez, J.: The Source of Ions for Biomineralization in Foraminifera and Their Implications for Paleoceanographic Proxies, Rev. Mineral. Geochem., 54, 115–149, 2003.
Goldstein, S. J.: Foraminifera: A biological overview, in: Modern Foraminifera, edited by: Gupta Sen, B. K., Kluwer Academic Publishers, Dordrecht, 37–55, 1999.
Goleń, J., Tyszka, J., Bickmeyer, U., and Bijma, J.: SiR-actin-labelled granules in foraminifera: patterns, dynamics, and hypotheses, Biogeosciences, 17, 995–1011, https://doi.org/10.5194/bg-17-995-2020, 2020.
Hancock, L. G., Walker, S. E., Pérez-Huerta, A., and Bowser, S. S.: Population Dynamics and Parasite Load of a Foraminifer on Its Antarctic Scallop Host with Their Carbonate Biomass Contributions, PLOS ONE, 10, e0132534, https://doi.org/10.1371/journal.pone.0132534, 2015.
Hedley, R. H.: The Biology of Foraminifera, in: International Review of General and Experimental Zoology, edited by: Felts, W. J. L. and Harrison, R. J., Elsevier, 45 pp., 1964.
Heinz, P., Geslin, E., and Hemleben, C.: Laboratory observations of benthic foraminiferal cysts, Mar. Biol. Res., 1, 149–159, 2005.
Hunt, A. S. and Corliss, B. H.: Distribution and microhabitats of living (stained) benthic foraminifera from the Canadian Arctic Archipelago, Mar. Micropaleontol., 20, 321–345, 1993.
Jorissen, F. J., de Stigter, H. C., and Widmark, J. G. V.: A conceptual model explaining benthic foraminiferal microhabitats, Mar. Micropaleontol., 26, 3–15, 1995.
Lee, A. J. and Anderson, O. R.: The biology of foraminifera, Academic Press, University of California, 368 pp., 1991.
Lee, J. J.: An illustrated guide to protozoa, Society of Protozoologists, Lawrence, Kansas, 629 pp., 1985.
Linke, P. and Lutze, G. F.: Microhabitat preferences of benthic foraminifera – a static concept or a dynamic adaptation to optimize food acquisition?, Mar. Micropaleontol., 20, 215–234, 1993.
Lipps, J. H.: Biotic interactions in benthic foraminifera, in: Biotic interactions in recent and fossil benthic communities, edited by: Teves, M. J. J. and McCall, P. L., Topics in Geobiology, Springer, Boston, MA, 331–376, 1983.
Lutze, G. F., Thiel, H.: Epibenthic foraminifera from elevated microhabitats: Cibicides wuellerstorfi and Planulina ariminnensis, J. Foramin. Res., 19, 153–158, 1989.
Nyholm, K. G.: Morphogenesis and Biology of the Foraminifer Cibicides Lobatulus, Almqvist & Wiksell, 157–196, 1962.
Rinaldi, R. A. and Jahn, T. L.: Shadowgraphs of protoplasmic movement in Allogromia laticollaris and a correlation of this movement to striated muscle contraction, Protoplasma, 58, 369–390, 1964.
Röttger, R.: Die Ektoplasmahülle von Heterostegina depressa (Foraminifera:Nummulitidae), Mar. Biol., 21, 127–138, 1973.
Röttger, R.: The Larger Foraminifer Heterostegina depressa – Organization and Growth of the Megalospheric Generation, IWF, https://doi.org/10.3203/IWF/C-1451, 1982.
Schönfeld, J.: A new benthic foraminiferal proxy for near-bottom current velocities in the Gulf of Cadiz, northeastern Atlantic Ocean, Deep-Sea Res. Pt. I, 49, 1853–1875, 2002.
Schultze, M. J. S.: Über den Organismus der Polythalamien (Foraminiferen), nebst Bemerkungen über die Rhizopoden im Allgemeinen, Leipzig, 88 pp., 1854.
Schweizer, M., Pawlowski, J., Kouwenhoven, T., van der Zwaan, B.: Molecular phylogeny of common cibicidids and related rotaliida (foraminifera) based on small subunit rDNA sequences, J. Foramin. Res., 39, 300–315, 2009.
Travis, J. L. and Bowser, S. S.: A new model of reticulopodial motility and shape: Evidence for a microtubule-based motor and an actin skeleton, Cell Motility, 6, 2–14, 1986.
Travis, J. L., Welnhofer, E. A., and Orokos, D. D.: Autonomous reorganization of foraminiferan reticulopodia, J. Foramin. Res., 32, 425–433, 2002.
Tyszka, J., Bickmeyer, U., Raitzsch, M., Bijma, J., Kaczmarek, K., Mewes, A., Topa, P., and Janse, M.: Form and function of F-actin during biomineralization revealed from live experiments on foraminifera, P. Natl. Acad. Sci. USA, 116, 4111, https://doi.org/10.1073/pnas.1810394116, 2019.
Wollenburg, J. E. and Kuhnt, W.: The response of benthic foraminifers to carbon flux and primary production in the Arctic Ocean, Mar. Micropaleontol., 40, 189–231, 2000.
Wollenburg, J. E., Kuhnt, W., and Mackensen, A.: Changes in Arctic Ocean paleoproductivity and hydrography during the last 145 kyr: the benthic foraminiferal record, Paleoceanography, 16, 65–77, 2001.
Wollenburg, J. E. and Mackensen, A.: Living benthic foraminifers from the central Arctic Ocean: faunal composition, standing stock and diversity, Mar. Micropaleontol., 34, 153–185, 1998a.
Wollenburg, J. E. and Mackensen, A.: On the vertical distribution of living (rose bengal stained) benthic foraminifers in the Arctic Ocean, J. Foramin. Res., 28, 268–285, 1998b.
Wollenburg, J. E., Raitzsch, M., and Tiedemann, R.: Novel high-pressure culture experiments on deep-sea benthic foraminifera – Evidence for methane seepage-related δ13C of Cibicides wuellerstorfi, Mar. Micropaleontol., 117, 47–64, 2015.
Wollenburg, J. E., Zittier, Z. M. C., and Bijma, J.: Insight into deep-sea life – Cibicidoides pachyderma substrate and pH-dependent behaviour following disturbance, Deep-Sea Res. Pt. I, 138, 34–45, 2018.