the Creative Commons Attribution 4.0 License.
the Creative Commons Attribution 4.0 License.
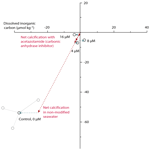
Carbonic anhydrase is involved in calcification by the benthic foraminifer Amphistegina lessonii
Siham de Goeyse
Alice E. Webb
Gert-Jan Reichart
Lennart J. de Nooijer
Marine calcification is an important component of the global carbon cycle. The mechanism by which some organisms take up inorganic carbon for the production of their shells or skeletons, however, remains only partly known. Although foraminifera are responsible for a large part of the global calcium carbonate production, the process by which they concentrate inorganic carbon is debated. Some evidence suggests that seawater is taken up by vacuolization and participates relatively unaltered in the process of calcification, whereas other results suggest the involvement of transmembrane transport and the activity of enzymes like carbonic anhydrase. Here, we tested whether inorganic-carbon uptake relies on the activity of carbonic anhydrase using incubation experiments with the perforate, large benthic, symbiont-bearing foraminifer Amphistegina lessonii. Calcification rates, determined by the alkalinity anomaly method, showed that inhibition of carbonic anhydrase by acetazolamide (AZ) stopped most of the calcification process. Inhibition of photosynthesis either by 3-(3,4-Dichlorophenyl)-1,1-dimethylurea (DCMU) or by incubating the foraminifera in the dark also decreased calcification rates but to a lesser degree than with AZ. Results from this study show that carbonic anhydrase plays a key role in biomineralization of Amphistegina lessonii and indicates that calcification of those perforate, large benthic foraminifera might, to a certain extent, benefit from the extra dissolved inorganic carbon (DIC), which causes ocean acidification.
- Article
(1882 KB) -
Supplement
(860 KB) - BibTeX
- EndNote
Fossil fuel burning and land use changes have been steadily increasing atmospheric CO2 levels. About one-third of the added carbon has been taken up by the ocean (Sabine and Tanhua, 2010), and the resulting increase in seawater dissolved carbon dioxide and associated acidification are lowering the saturation state of seawater with respect to calcite and hence likely affect marine calcifiers. Even a modest impact on the production of carbonate shells and skeletons may have important consequences for the global carbon cycle. Foraminifera are responsible for almost 25 % of the total marine calcium carbonate production (Langer, 2008), and their response to ongoing acidification is therefore important to predict future marine inorganic-carbon cycling. Despite its relevance for future CO2 scenarios, it is still unclear how increased pCO2 in seawater will affect foraminiferal calcification. Previous research has shown discrepancies in their results: in some cases higher pCO2 increased the growth rate of benthic foraminifera, while in other cases calcification decreased or halted (Haynert et al., 2014; Hikami et al., 2011).
Addition of CO2 to seawater not only reduces saturation state with respect to calcite but also increases the total dissolved inorganic carbon (DIC) concentration. At surface seawater pH, the dominant DIC species is , and many marine calcifiers are shown to employ transmembrane bicarbonate ion transporters (e.g., coccolithophores: Brownlee et al., 2015; Mackinder et al., 2011; scleractinian corals: Cai et al., 2016; Giri et al., 2019; Zoccola et al., 2015), which may also be the case for foraminifera. If so, ocean acidification would be detrimental as this shifts the carbonate system from to CO2. Alternatively, CO2 may be the inorganic-carbon source of choice for benthic foraminifera as it diffuses relatively easily through lipid membranes. The latter uptake mechanism would facilitate foraminiferal calcification as ongoing CO2 dissolution increases total DIC and hence the availability of building blocks for chamber formation. Since this uptake mechanism is crucial for calcification in a rapidly changing ocean and because it is essentially unknown how foraminifera take up inorganic carbon, it remains difficult to predict the reaction of foraminifera to ongoing environmental change. It was recently suggested that CO2 uptake by benthic foraminifera is achieved through proton pumping (Glas et al., 2012; Toyofuku et al., 2017). The outward proton flux increases the pCO2 directly outside the site of calcification (SOC) through conversion of bicarbonate into carbon dioxide. The elevated pH at the foraminifers' site of calcification (Bentov et al., 2009; de Nooijer et al., 2009) and reduced pH outside the cell thus result in a strong inward–outward pCO2 gradient, promoting inward CO2 diffusion. If calcification in foraminifera relies on this inward CO2 diffusion, the conversion from outside the test may be a limiting step for ongoing calcite precipitation. This process may be catalyzed by an enzymatic conversion by carbonic anhydrase (CA), which is present in many prokaryotes and virtually all eukaryotes (Hewett-Emmett and Tashian, 1996; Lionetto et al., 2016). This enzyme is essential in calcification in many organisms, including corals, sponges and coccolithophores (Bertucci et al., 2013; Medaković, 2000; Müller et al., 2013; Le Roy et al., 2014; Wang et al., 2017). Also for foraminiferal calcification it has been hypothesized that CA is used to enhance inorganic-carbon uptake. Indirect evidence for such a role in calcification comes from the observed slope between the carbon and oxygen isotopes (Chen et al., 2018), but direct evidence is, however, still missing.
To test whether carbonic anhydrase is involved in biomineralization of perforate, benthic foraminifera, we incubated calcifying specimens of Amphistegina lessonii with acetazolamide (AZ), a membrane-impermeable inhibitor of this enzyme (Elzenga et al., 2000; Moroney et al., 1985). Calcification and respiration were determined by measuring changes in alkalinity and DIC of the incubated seawater over the course of the experiment. An additional experiment was conducted in parallel to test whether CA is directly involved in perforate foraminiferal calcification or whether the effect is indirect via photosynthesis. The latter would imply that CA drives photosynthesis by the symbionts and that observed effects would be due to reduced photosynthesis impairing calcification through reduced energy transfer from the symbionts to the foraminifer.
2.1 Foraminifera and incubations
Surface sediments were collected from the Indo-Pacific coral reef aquarium in Burgers' Zoo (Arnhem, the Netherlands; Ernst et al., 2011). The sediments were kept at 24 ∘C, with a day–night cycle of 12 h ∕ 12 h. Living specimens of Amphistegina lessonii showing a dark cytoplasm and pseudopodial activity were manually selected using a fine brush under a stereomicroscope and transferred to Petri dishes. They were fed with freeze-dried Dunaliella salina and incubated in North Atlantic seawater (salinity: 36). After 1 week, viable specimens were collected and divided over eight experimental conditions, each of them consisting of three groups (Fig. 1). Each group consisted of 40–60 specimens with a similar size distribution (initial diameter: 140 to 1400 µm; shown in Fig. S1 in the Supplement). Foraminifera were placed in airtight glass vials of 80 mL (24 ∘C, 12 h daylight cycle) for 5 d. Illumination was approximately 180 µmol photons m−2 s−1 during the 12 h of light.
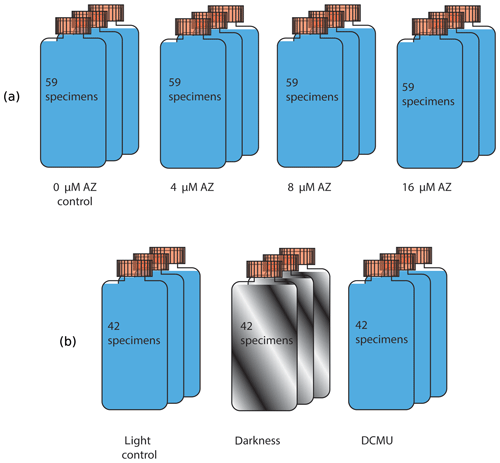
Figure 1A total of 59 specimens were placed in one culture vial, with three replicate vials for each concentration of acetazolamide (upper row). Similarly, 42 specimens were incubated under light, in the dark and with the inhibitor DCMU (lower row).
In the first experiment, the impact of acetazolamide (AZ) on calcification was tested. A stock solution was prepared by dissolving AZ (Sigma-Aldrich) in dimethyl sulfoxide (DMSO; 0.05 % v∕v) at a final concentration of 90 mM. It has been shown that DMSO at concentrations of 10 %–20 % v∕v does not impair calcification (Moya et al., 2008), so the effect of this solvent is not reported here separately. The AZ stock solution was diluted with seawater from the North Atlantic to achieve AZ concentrations of 4, 8 and 16 µM, which were used to incubate the foraminifera. In a second experiment, inhibition of photosynthesis was tested by (1) addition of 3-(3,4-Dichlorophenyl)-1,1-dimethylure (DCMU; Tóth et al., 2005; Velthuys, 1981) and (2) darkness. DCMU was added to seawater at a final concentration of 6 µM, whereas covering the vials with aluminum foil prevented light-dependent reaction and hence photosynthesis in a second set of incubations (Fig. 1).
2.2 Alkalinity, DIC and nutrient analysis
To quantify calcification and respiration, total alkalinity (TA) and the concentration of dissolved inorganic carbon [DIC] were determined at the beginning and end of every incubation. This method was chosen above other growth method measurements such as sample weighing or counting chamber addition as it allows a quantification of the amount of calcite formed during the actual experiment. Total alkalinity was analyzed immediately at the end of each experiment, whereas subsamples to determine nutrient concentrations and DIC analyses were stored at −20 ∘C (nutrients) and 4 ∘C (DIC). The samples for DIC analyses were poisoned with mercury chloride (DIC) until analysis. These samples first passed a 0.2 µm syringe filter.
Alkalinity measurements were performed using an automated spectrophotometric alkalinity system (ASAS), as described by Liu et al. (2015). Briefly, 60 mL of seawater is placed in a borosilicate vial and automatically titrated with a solution of 0.1 M HCl. Before the start of the titration, 45 µL of bromocresol purple (10 mmol L−1) was added to the seawater, and pH changes were followed by spectrophotometry. Certified reference material (CRM; Dickson, 2001; Scripps Institution of Oceanography) was analyzed at the beginning of every series (5–10 samples) of measurements. Reproducibility of the obtained TA was ∼ 3 µmol kg−1 (SD), based on 50 measurements of untreated seawater.
Nutrient samples were analyzed on a QuAAtro continuous flow analyzer (SEAL Analytical, GmbH, Norderstedt, Germany) following GO-SHIP protocol (Hydes et al., 2010). DIC was measured on an autoanalyzer TRAACS 800 spectrophotometric system, as described in Stoll et al. (2001).
2.3 Calcification rate
Changes in DIC and alkalinity between start and end of the experiments were used to calculate the net respiration and calcification (Fig. 2). Total measured alkalinity is defined as the contribution of the following anions:
Concentrations of boron and silicon were neglected as the first one is constant and the second one is present at a low abundance. In order to account for the alkalinity change related to the inorganic-carbon system only, we subtracted the combined concentrations of the nutrients from the measured alkalinity so that the observed alkalinity over time is defined as
Respnet is defined as the difference between respiration and photosynthesis. Here, we consider the respiration of the holobiont (foraminifera and its symbionts), which is calculated by
Since other processes, e.g., respiration by bacteria, may affect the TA and [DIC] during the incubations, vials were carefully checked for the presence of biofilms. There was no sign of such activity in any of the treatments, so changes in TA and [DIC] are attributed to the foraminifera and their symbionts.
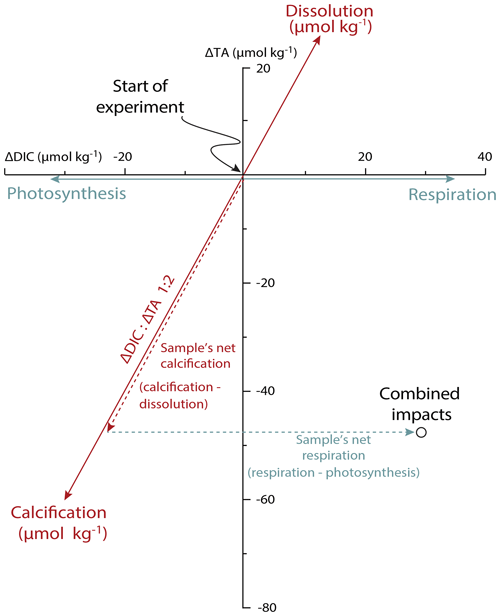
Figure 2Calcification and net respiration of foraminifera deduced from changes in DIC and total alkalinity over time. The blue vectors show the impact of photosynthesis and respiration (impacting DIC); the red arrows show the impact of calcification and calcite dissolution (impacting both DIC and TA in a 1 : 2 ratio). Observed changes for each incubation should be decomposed into two vectors: a contribution of calcification (dashed red arrow) and the net effect of respiration and photosynthesis (dashed blue arrow). The approach is indicated here for a hypothetical incubation.
3.1 Carbonic anhydrase inhibition
Without acetazolamide, TA decreased on average by 53 µmol kg−1 and DIC by 38 µmol L−1 during the incubation (Table 1). This corresponds to 2.74 g L−1 of precipitated calcite. Contrastingly, when the seawater contained acetazolamide (even at the lowest concentration of 4 µM), alkalinity and DIC did not change or decreased only marginally during the incubation (less than 0.4 g L−1 of calcite precipitated). When comparing the changes in TA and DIC between treatments, calcification is minimized by the AZ, and net respiration slightly increases (Fig. 3). The concentration of AZ has no discernible effect on the magnitude of changes in calcification or respiration.
The number of chambers added by the foraminifera shows that the average number of chambers added decreases after addition of AZ (Table 3). Whereas many specimens in the control vials added two chambers, almost all calcification after addition of AZ resulted in the addition of only one chamber.
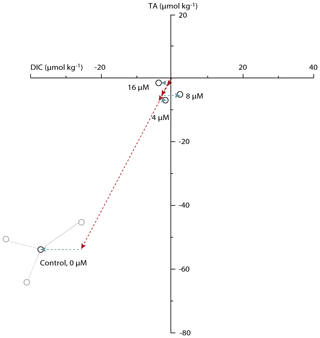
Figure 3Changes in total alkalinity versus DIC for all concentrations of acetazolamide (AZ) used. Every black circle represents the average change in DIC–TA for one triplicate of incubations. The three gray circles show the measured DIC–TA combination for each of the triplicate measurements within the control treatment. For the three additions of AZ, replicates never differed more than 8 µmol kg−1 from the average for DIC and never more than 5 µmol kg−1 from the average for TA. Arrows show the calcification (red) and net respiration (blue) effects.
3.2 Photosynthesis inhibition
When photosynthesis was not impaired (light control), alkalinity decreased within the vials by 70 µmol L−1, and DIC increased by 21 µmol L−1 (Table 2). Given the relative standard deviations, this is similar to the changes in TA and DIC in the control vials for the AZ experiments. These changes correspond to approximatively 3.75 g L−1 of precipitated calcite. In contrast, when foraminifera were cultivated in the dark or in the presence of the photosynthesis inhibitor DCMU, DIC increased by 16 and 42 µmol L−1, respectively, whereas the total alkalinity decrease was only 19 and 11 µmol L−1, respectively, which corresponds to less than a third of the amount of calcite precipitated when photosynthesis was not hampered (Fig. 4). Changes in DIC–TA are also reflected in the number of chambers added to the incubated foraminifera: with DCMU or AZ added and in the dark, specimens added fewer chambers than the control group (Table 3). Some of the smaller specimens incubated during the experiment were not retrieved from the vial, explaining the missing specimens (Table 3). The foraminifera incubated with an inhibitor have more broken chambers than the others.
Table 2Total alkalinity and DIC changes for every triplicate. Confidence interval: 1 SD (taking biological variability into account).
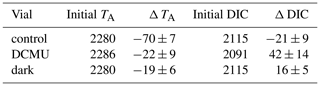
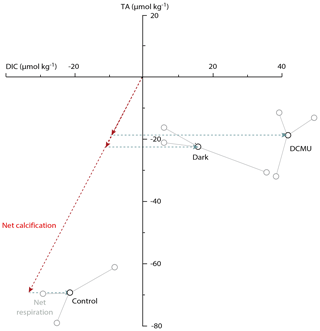
Figure 4Changes in total alkalinity versus that in DIC for incubations in light–dark alternation (control), in the dark and with the photosynthetic inhibitor DCMU. Every black circle represents the average change in TA and DIC between the initial and the final values for each triplicate. The three gray circles show the measured DIC–TA combination for each of the triplicate measurements within every of the three treatments. For the “dark” and “DCMU” treatments, the individual DIC–TA combinations are connected to the average value. Arrows show the calcification (red) and net respiration (blue) effects.
4.1 Growth rates and the effect of AZ
In the control experiments (incubations with unaltered seawater), foraminiferal calcification resulted in a decrease in alkalinity of the culture media by approximately 53 µmol L−1 over a period of 5 d (Table 1). On average, this equals a growth rate of 1.0 µg per individual per day which is low when compared to some previously reported rates for foraminiferal calcification (∼ 6–60 µg per individual per day; Evans et al., 2018; Glas et al., 2012; Keul et al., 2013). These studies, however, all used different species than the one incubated here. Previous research using Amphistegina spp. reported growth rates of 3–9 and 2.6–4 µg per individual per day (ter Kuile and Erez, 1984; ter Kuile and Erez, 1987), respectively, while Hallock et al. (1986) reported rates of 0.3–6.6 µg per individual per day depending on the light intensity. Segev and Erez (2006) reported growth rates similar to those observed in our study (0.53–1.0 µg per individual per day) based on changes in dry weight. The growth rates reported here fall in the lower range of those previously reported, which may be due to the average size of our specimens, the light intensity used and/or the short duration of our experiment.
Addition of AZ caused a 20-fold decrease in calcification rates (Fig. 3) while increasing net respiration. The concentration of the inhibitor (4–16 µM) did not affect the magnitude by which net calcification decreased, nor does it appear to affect the increase in net respiration (Fig. 3). The accompanying decrease in the number of chambers added per specimen (Table 3) suggests that AZ did not decrease the survival rates of the incubated specimens but affected the rate of chamber addition in all specimens equally. The inhibition of calcification caused by AZ suggests that carbonic anhydrase plays a crucial role in perforate foraminiferal biomineralization. With the inhibitor present, specimens produced little to no calcite (Fig. 3), indicating that biomineralization either relies on CA or is negatively impacted through an effect of CA on photosynthesis. Whether calcification depends directly on extracellular carbonic anhydrase (eCA) or whether calcification depends on photosynthesis and thereby indirectly on CA can be inferred from comparing the two sets of experiments (Fig. 1).
4.2 Effect of photosynthesis on calcification
The inhibition of photosynthesis with DCMU and darkness decreases calcification comparably (Fig. 3). Simultaneously, net respiration increases after addition of DCMU, and so does blocking light (Fig. 4). The similarity in the effect of darkness and DCMU indicates that photosynthesis has an effect on calcification in these perforate foraminifera. It was previously suggested that light, irrespective of photosynthesis, enhances calcification in foraminifera (Erez, 2003). Since the latter study used the planktonic, low-Mg calcite Globigerinoides sacculifer, the discrepancy between results may be caused by differences in the process involved in calcification between these species. For example, it has been suggested that calcification may involve seawater transport (Erez, 2003; Segev and Erez, 2006) as well as transmembrane transport (Nehrke et al., 2013; Toyofuku et al., 2017), of which the relative contribution may vary between groups of foraminifera.
Foraminiferal calcification and endosymbiont photosynthesis both require inorganic carbon. Therefore, it seems reasonable to suggest that those two mechanisms are competing with each other for inorganic carbon, as was shown by ter Kuile et al. (1989a, b). However, our results show that preventing photosynthesis by the symbionts actually decreases foraminiferal calcification. This implies that benefits from photosynthesis outweigh an eventual competition with calcification, which is in agreement with results from Duguay (1983) and Hallock (1981), who showed that uptake of both calcium and inorganic carbon into the cell is enhanced by light. As the foraminifera were in the dark 12 h a day, it might also be that DIC is shared over time, being used for calcification during the dark phase and CO2 being used for calcification during the light phase.
It was shown that photosynthetic symbionts provide energy to their foraminiferal hosts (Lee, 2001) and that calcification in some foraminifera is enhanced by the photosymbiont's activity (e.g., Hallock, 2000; Stuhr et al., 2018). This was seen already by Muller (1978), for example, who reported increased carbon fixation by the foraminifer A. lessonii in the light compared to uptake of carbon in the dark. A positive effect of higher CO2 level on calcification through enhanced photosynthesis is known as “fertilization effect” (Ries et al., 2009). A positive effect of photosynthesis on calcification has been observed previously for other marine calcifiers as well. For example, in coccolithophores, decreasing CO2 can hamper calcification through reduced photosynthesis (Mackinder et al., 2010). Utilization of photosynthate as an organic template for calcification may explain this observation. We hypothesize here that a similar effect may explain decreased calcification in foraminifera as a consequence of inhibited photosynthesis (Fig. 3), as hypothesized by Toler and Hallock (1998). If so, the type of organic molecules produced by the foraminifer's endosymbionts and their fluxes will need to be assessed to test the extent of the dependency of calcification on photosynthesis. However, it has been shown that symbiotic dinoflagellates can trigger the activity of carbonic anhydrase from their host organisms (giant clams and sea anemones; Leggat et al., 2003; Weis, 1991; Weis and Reynolds, 2002; Yellowlees et al., 2008), thereby explaining how photosynthesis enhances calcification. Alternatively, increased activity of CA in the symbiont may also promote the flux of products to the host and thereby promote calcification indirectly. Since there are many (perforate) foraminiferal species that do not have photosynthetic symbionts, the effect of inhibiting CA in these species may provide additional information on the role played by CA in calcification.
4.3 Role of CA in calcification
In calcifiers other than foraminifera, carbonic anhydrase plays a direct role in calcification. For example, in giant clams (Chew et al., 2019), gastropods (Le Roy et al., 2012) and oysters (Wang et al., 2017), CA helps to concentrate inorganic carbon in the fluid from which calcium carbonate precipitates. In scleractinian corals, CA promotes conversion of metabolic CO2 into bicarbonate after the carbon dioxide diffused into the subcalicoblastic space (Bertucci et al., 2013). Although the inorganic carbon would take the same route in the absence of CA, the hydration of CO2 is relatively slow and ion fluxes and calcification rates would be a fraction of what they are with the catalytic activity of CA. This role of CA fits with the localization of (membrane-bound) CA observed at the walls of the calicoblastic cells by immunolabeling (Moya et al., 2008). In addition, by facilitating an inward flux of inorganic carbon, involvement of CA can explain the covariation of oxygen and carbon isotopes in coral aragonite (Chen et al., 2018; Uchikawa and Zeebe, 2012).
In larger benthic foraminifera, CA likely plays different roles: it helps in concentrating CO2 by the symbionts and aids foraminiferal calcification. It is also likely that cytoplasmic CAs – involved for instance in intracellular pH regulation – also affect calcification. The molecular types of CA that are involved and their precise location still remain to be investigated within the larger benthic foraminifera. In addition, the type of symbionts or their absence may affect inorganic-carbon uptake so that the result obtained here may only partially apply to foraminifera in general. Analogous to other calcifying organisms and based on existing models of foraminiferal calcification, we hypothesize that extracellular CA helps to convert into CO2 directly outside the calcifying chamber. This would help to further increase the pCO2 outside the foraminifer in addition to the shift in inorganic-carbon chemistry resulting from active proton pumping and subsequent low pH (Glas et al., 2012; de Nooijer et al., 2009; Toyofuku et al., 2017). Although not directly targeted by our experimental approach as the inhibitor we used is membrane-impermeable, it is likely that a form of CA within the calcifying fluid increases the rate by which the diffused CO2 is converted into bicarbonate.
The involvement of extracellular CA in calcification may explain why perforate foraminifera can be relatively resilient to ocean acidification. It also remains to be investigated whether Tubothalamea, which produce their calcite in a fundamentally different way (Mikhalevich, 2013; Pawlowski et al., 2013), use CA similarly. If they rely on CA for conversion of to CO2 and take up inorganic carbon by diffusion of CO2, additional dissolved atmospheric CO2 may be beneficial for calcification in foraminifera. If they exclusively rely on bicarbonate ions, a reduction in pH would lower the [] and thereby hamper calcification. Manipulation of the inorganic-carbon speciation in relation to calcification and the aid of enzymes therein will allow the prediction of rates of calcification as a function of ongoing ocean acidification.
The alkalinity anomaly method allowed us to quantify growth rates in incubation experiments, equalling addition of 1 µg per individual per day. Calcification and photosynthesis in the benthic foraminifer Amphistegina lessonii and its symbionts both depend on carbonic anhydrase (CA), as shown after inhibition by acetazolamide (AZ). Since the inhibitor is membrane-impermeable, the CA may well be localized at the outside of the foraminifer's cell membrane. Our results also show that inhibiting photosynthesis by DCMU or incubation in darkness reduces calcification similarly. This suggests that not light, but photosynthesis itself promotes calcification in symbiont-bearing perforate foraminifera. We also suggest that CA plays a role in concentrating inorganic carbon for calcification, possibly by promoting conversion of bicarbonate into carbon dioxide outside the foraminifer.
The data on which this publication is based can be found at https://doi.org/10.4121/uuid:afcdcdc1-2591-4822-bade-806119cdd724 (De Goeyse et al., 2020).
The supplement related to this article is available online at: https://doi.org/10.5194/bg-18-393-2021-supplement.
SdG and LJdN designed the experiment, and SdG carried it out. SdG and AEW analyzed the seawater inorganic chemistry. SdG and LJdN analyzed the data and prepared the manuscript with contributions from all coauthors.
The authors declare that they have no conflict of interest.
We would like to thank Karel Bakker for DIC measurements. We kindly thank Max Janse (Burgers' Zoo, Arnhem) for providing stock specimens of Amphistegina lessonii and Kirsten Kooijmans and Michele Grego (NIOZ) for providing cultures of Dunaliella salina. We thank Takashi Toyofuku, the three anonymous reviewers and the editors for their helpful comments and all subsequent improvements.
This research has been supported by the Netherlands Earth System Science Centre (NESSC; Dutch Ministry of Education, Culture and Science, OCW; grant no. 024.002.001) and the NWO Open Competition program (Pasodoble Grant).
This paper was edited by Hiroshi Kitazato and reviewed by Takashi Toyofuku and three anonymous referees.
Bentov, S., Brownlee, C., and Erez, J.: The role of seawater endocytosis in the biomineralization process in calcareous foraminifera, P. Natl. Acad. Sci. USA, 106, 21500–21504, https://doi.org/10.1073/pnas.0906636106, 2009.
Bertucci, A., Moya, A., Tambutté, S., Allemand, D., Supuran, C. T., and Zoccola, D.: Carbonic anhydrases in anthozoan corals – A review, Bioorgan. Med. Chem., 21, 1437–1450, https://doi.org/10.1016/j.bmc.2012.10.024, 2013.
Brownlee, C., Wheeler, G. L., and Taylor, A. R.: Coccolithophore biomineralization: New questions, new answers, Semin. Cell Dev. Biol., 46, 11–16, https://doi.org/10.1016/j.semcdb.2015.10.027, 2015.
Cai, W.-J. J., Ma, Y., Hopkinson, B. M., Grottoli, A. G., Warner, M. E., Ding, Q., Hu, X., Yuan, X., Schoepf, V., Xu, H., Han, C., Melman, T. F., Hoadley, K. D., Pettay, D. T., Matsui, Y., Baumann, J. H., Levas, S., Ying, Y., and Wang, Y.: Microelectrode characterization of coral daytime interior pH and carbonate chemistry, Nat. Commun., 7, 11144, https://doi.org/10.1038/ncomms11144, 2016.
Chen, S., Gagnon, A. C., and Adkins, J. F.: Carbonic anhydrase, coral calcification and a new model of stable isotope vital effects, Geochim. Cosmochim. Ac., 236, 179–197, https://doi.org/10.1016/j.gca.2018.02.032, 2018.
Chew, S. F., Koh, C. Z. Y., Hiong, K. C., Choo, C. Y. L., Wong, W. P., Neo, M. L., and Ip, Y. K.: Light-enhanced expression of Carbonic Anhydrase 4-like supports shell formation in the fluted giant clam Tridacna squamosa, Gene, 683, 101–112, https://doi.org/10.1016/j.gene.2018.10.023, 2019.
De Goeyse, S., Webb, A. E., Reichart, G.-J., and de Nooijer, L. J.: Dissolved organic carbon and total alkalinity values presented in article: “Carbonic anhydrase is involved in benthic foraminiferal calcification”, 4TU.ResearchData, https://doi.org/10.4121/uuid:afcdcdc1-2591-4822-bade-806119cdd724, 2020.
de Nooijer, L. J., Toyofuku, T., and Kitazato, H.: Foraminifera promote calcification by elevating their intracellular pH, P. Natl. Acad. Sci. USA, 106, 15374–15378, https://doi.org/10.1073/pnas.0904306106, 2009.
Dickson, A. G.: Reference materials for oceanic CO2 measurements, Oceanography, 14, 21–22, 2001.
Duguay, L. E.: Comparative laboratory and field studies on calcification and carbon fixation in foraminiferal-algal associations, J. Foramin. Res., 13, 252–261, 1983.
Elzenga, J. T. M., Prins, H. B. A., and Stefels, J.: The role of extracellular carbonic anhydrase activity in inorganic carbon utilization of Phaeocystis globosa (Pyrmnesiophyceae): A comparison with other marine algae using isotopic disequilibrium technique, Limnol. Oceanogr., 45, 372–380, https://doi.org/10.4319/lo.2000.45.2.0372, 2000.
Erez, J.: The Source of Ions for Biomineralization in Foraminifera and Their Implications for Paleoceanographic Proxies, Rev. Mineral. Geochem., 54, 115–149, https://doi.org/10.2113/0540115, 2003.
Ernst, S., Janse, M., Renema, W., Kouwenhoven, T., Goudeau, M.-L., and Reichart, G.-J.: Benthic foraminifera in a large Indo-Pacific coral reef aquarium, J. Foramin. Res., 41, 101–113, https://doi.org/10.2113/gsjfr.41.2.101, 2011.
Evans, D., Müller, W., and Erez, J.: Assessing foraminifera biomineralisation models through trace element data of cultures under variable seawater chemistry, Geochim. Cosmochim. Ac., 236, 198–217, https://doi.org/10.1016/j.gca.2018.02.048, 2018.
Giri, S. J., Swart, P. K., and Pourmand, A.: The influence of seawater calcium ions on coral calcification mechanisms: Constraints from boron and carbon isotopes and B∕Ca ratios in Pocillopora damicornis, Earth Planet. Sc. Lett., 519, 130–140, https://doi.org/10.1016/j.epsl.2019.05.008, 2019.
Glas, M. S., Fabricius, K. E., de Beer, D., and Uthicke, S.: The O2, pH and Ca2+ Microenvironment of Benthic Foraminifera in a High CO2 World, PLoS ONE, 7, e50010, https://doi.org/10.1371/journal.pone.0050010, 2012.
Hallock, P.: Light dependence in Amphistegina, J. Foramin. Res., 11, 40–46, https://doi.org/10.2113/gsjfr.11.1.40, 1981.
Hallock, P.: Larger Foraminifera as Indicators of Coral-Reef Vitality, Springer, Boston, MA, USA, 121–150, 2000.
Hallock, P., Forward, L. B., and Hansen, H. J.: Influence of environment on the test shape of Amphistegina, J. Foramin. Res., 16, 224–231, https://doi.org/10.2113/gsjfr.16.3.224, 1986.
Haynert, K., Schönfeld, J., Schiebel, R., Wilson, B., and Thomsen, J.: Response of benthic foraminifera to ocean acidification in their natural sediment environment: a long-term culturing experiment, Biogeosciences, 11, 1581–1597, https://doi.org/10.5194/bg-11-1581-2014, 2014.
Hewett-Emmett, D. and Tashian, R. E.: Functional diversity, conservation, and convergence in the evolution of the α-, β-, and γ-carbonic anhydrase gene families, Mol. Phylogenet. Evol., 5, 50–77, https://doi.org/10.1006/mpev.1996.0006, 1996.
Hikami, M., Ushie, H., Irie, T., Fujita, K., Kuroyanagi, A., Sakai, K., Nojiri, Y., Suzuki, A., and Kawahata, H.: Contrasting calcification responses to ocean acidification between two reef foraminifers harboring different algal symbionts, Geophys. Res. Lett., 38, 22, https://doi.org/10.1029/2011GL048501, 2011.
Hydes, D. J., Aoyama, M., Aminot, A., Bakker, K., Becker, S., Coverly, S., Daniel, A., Dickson, A. G., Grosso, O., Kerouel, R., Van Ooijen, J., Sato, K., Tanhua, T., Woodward, M., and Zhang, J.-Z.: Determination of dissolved nutrients (N, P, Si) in seawater with high precision and inter-comparability using gas-segmented continuous flow analysers, in: The GO-SHIP Repeat Hydrography Manual : A Collection of Expert Reports and guidelines. IOCCP Report No 14, ICPO Publication Series No. 134, version 1, 1–87 (online), available at: http://archimer.ifremer.fr/doc/00020/13141/ (last access: November 2020), 2010.
Keul, N., Langer, G., de Nooijer, L. J., and Bijma, J.: Effect of ocean acidification on the benthic foraminifera Ammonia sp. is caused by a decrease in carbonate ion concentration, Biogeosciences, 10, 6185–6198, https://doi.org/10.5194/bg-10-6185-2013, 2013.
Langer, M. R.: Assessing the contribution of foraminiferan protists to global ocean carbonate production, J. Eukaryot. Microbiol., 55, 163–169, https://doi.org/10.1111/j.1550-7408.2008.00321.x, 2008.
Lee, J. J.: Living Sands: Symbiosis between Foraminifera and Algae, in: Symbiosis, Kluwer Academic Publishers, Dordrecht, the Netherlands, 491–506, 2001.
Leggat, W., Buck, B. H., Grice, A., and Yellowlees, D.: The impact of bleaching on the metabolic contribution of dinoflagellate symbionts to their giant clam host, Plant Cell Environ., 26, 1951–1961, https://doi.org/10.1046/j.0016-8025.2003.01111.x, 2003.
Le Roy, N., Marie, B., Gaume, B., Guichard, N., Delgado, S., Zanella-Cléon, I., Becchi, M., Auzoux-Bordenave, S., Sire, J. Y., and Marin, F.: Identification of Two Carbonic Anhydrases in the Mantle of the European Abalone Haliotis tuberculata (Gastropoda, Haliotidae): Phylogenetic Implications, J. Exp. Zool. Part B, 318, 353–367, https://doi.org/10.1002/jez.b.22452, 2012.
Le Roy, N., Jackson, D. J., Marie, B., Ramos-Silva, P., and Marin, F.: The evolution of metazoan α-carbonic anhydrases and their roles in calcium carbonate biomineralization, Front. Zool., 11, 75, https://doi.org/10.1186/s12983-014-0075-8, 2014.
Lionetto, M. G., Caricato, R., Giordano, M. E., and Schettino, T.: The Complex Relationship between Metals and Carbonic Anhydrase: New Insights and Perspectives., Int. J. Mol. Sci., 17, 127, https://doi.org/10.3390/ijms17010127, 2016.
Liu, X., Byrne, R. H., Lindemuth, M., Easley, R., and Mathis, J. T.: An automated procedure for laboratory and shipboard spectrophotometric measurements of seawater alkalinity: Continuously monitored single-step acid additions, Mar. Chem., 174, 141–146, https://doi.org/10.1016/j.marchem.2015.06.008, 2015.
Mackinder, L., Wheeler, G., Schroeder, D., Riebesell, U., and Brownlee, C.: Molecular mechanisms underlying calcification in coccolithophores, Geomicrobiol. J., 27, 585–595, https://doi.org/10.1080/01490451003703014, 2010.
Mackinder, L., Wheeler, G., Schroeder, D., von Dassow, P., Riebesell, U., and Brownlee, C.: Expression of biomineralization-related ion transport genes in Emiliania huxleyi, Environ. Microbiol., 13, 3250–3265, https://doi.org/10.1111/j.1462-2920.2011.02561.x, 2011.
Medaković, D.: Carbonic anhydrase activity and biomineralization process in embryos, larvae and adult blue mussels Mytilus edulis L., Helgoland Mar. Res., 54, 1–6, https://doi.org/10.1007/s101520050030, 2000.
Mikhalevich, V. I.: New insight into the systematics and evolution of the foraminifera, Micropaleontology, 59, 493–527, 2013.
Moroney, J. V., Husic, H. D., and Tolbert, N. E.: Effect of Carbonic Anhydrase Inhibitors on Inorganic Carbon Accumulation by Chlamydomonas reinhardtii, Plant Physiol., 79, 177–183, https://doi.org/10.1104/pp.79.1.177, 1985.
Moya, A., Tambutté, S., Bertucci, A., Tambutté, E., Lotto, S., Vullo, D., Supuran, C. T., Allemand, D., and Zoccola, D.: Carbonic anhydrase in the scleractinian coral Stylophora pistillata: Characterization, localization, and role in biomineralization, J. Biol. Chem., 283, 25475–25484, https://doi.org/10.1074/jbc.M804726200, 2008.
Muller, P. H.: 14Carbon fixation and loss in a foraminiferal-algal symbiont system, J. Foramin. Res., 8, 35–41, https://doi.org/10.1007/s005350300016, 1978.
Müller, W. E. G., Schröder, H. C., Schlossmacher, U., Neufurth, M., Geurtsen, W., Korzhev, M., and Wang, X.: The enzyme carbonic anhydrase as an integral component of biogenic Ca-carbonate formation in sponge spicules, FEBS Open Bio, 3, 357–362, https://doi.org/10.1016/j.fob.2013.08.004, 2013.
Nehrke, G., Keul, N., Langer, G., de Nooijer, L. J., Bijma, J., and Meibom, A.: A new model for biomineralization and trace-element signatures of Foraminifera tests, Biogeosciences, 10, 6759–6767, https://doi.org/10.5194/bg-10-6759-2013, 2013.
Pawlowski, J., Holzmann, M., and Tyszka, J.: New supraordinal classification of Foraminifera: Molecules meet morphology, Mar. Micropaleontol., 100, 1–10, https://doi.org/10.1016/j.marmicro.2013.04.002, 2013.
Ries, J. B., Cohen, A. L., and McCorkle, D. C.: Marine calcifiers exhibit mixed responses to CO2-induced ocean acidification, Geology, 37, 1131–1134, https://doi.org/10.1130/G30210A.1, 2009.
Sabine, C. L. and Tanhua, T.: Estimation of Anthropogenic CO2 Inventories in the Ocean, Annu. Rev. Mar. Sci., 2, 175–198, https://doi.org/10.1146/annurev-marine-120308-080947, 2010.
Segev, E. and Erez, J.: Effect of Mg/Ca ratio in seawater on shell composition in shallow benthic foraminifera, Geochem. Geophy. Geosy., 7, 8, https://doi.org/10.1029/2005GC000969, 2006.
Stoll, M. H. C., Bakker, K., Nobbe, G. H., and Haese, R. R.: Continuous-flow analysis of dissolved inorganic carbon content in seawater, Anal. Chem., 73, 4111–4116, https://doi.org/10.1021/ac010303r, 2001.
Stuhr, M., Blank-Landeshammer, B., Reymond, C. E., Kollipara, L., Sickmann, A., Kucera, M., and Westphal, H.: Disentangling thermal stress responses in a reef-calcifier and its photosymbionts by shotgun proteomics, Sci. Rep., 8, 1–13, https://doi.org/10.1038/s41598-018-21875-z, 2018.
ter Kuile, B. and Erez, J.: In situ growth rate experiments on the symbiont-bearing foraminifera amphistegina lobifera and amphisorus hmprichii, J. Foramin. Res., 14, 262–276, https://doi.org/10.2113/gsjfr.14.4.262, 1984.
ter Kuile, B. and Erez, J.: Uptake of inorganic carbon and internal carbon cycling in symbiont-bearing benthonic foraminifera, Mar. Biol., 94, 499–509, 1987.
ter Kuile, B., Erez, J., and Padan, E.: Competition for inorganic carbon between photosynthesis and calcification in the symbiont-bearing foraminifer Amphistegina lobifera, Mar. Biol., 103, 253–259, https://doi.org/10.1007/BF00543355, 1989a.
ter Kuile, B., Erez, J., and Padan, E.: Mechanisms for the uptake of inorganic carbon by two species of symbiont-bearing foraminifera, Mar. Biol., 103, 241–251, https://doi.org/10.1007/BF00543354, 1989b.
Toler, S. K. and Hallock, P.: Shell malformation in stressed Amphistegina populations: Relation to biomineralization and paleoenvironmental potential, Mar. Micropaleontol., 34, 107–115, https://doi.org/10.1016/S0377-8398(97)00043-1, 1998.
Tóth, S. Z., Schansker, G., and Strasser, R. J.: In intact leaves, the maximum fluorescence level (FM) is independent of the redox state of the plastoquinone pool: A DCMU-inhibition study, BBA-Bioenergetics, 1708, 275–282, https://doi.org/10.1016/j.bbabio.2005.03.012, 2005.
Toyofuku, T., Matsuo, M. Y., de Nooijer, L. J., Nagai, Y., Kawada, S., Fujita, K., Reichart, G.-J., Nomaki, H., Tsuchiya, M., Sakaguchi, H., and Kitazato, H.: Proton pumping accompanies calcification in foraminifera, Nat. Commun., 8, 14145, https://doi.org/10.1038/ncomms14145, 2017.
Uchikawa, J. and Zeebe, R. E.: The effect of carbonic anhydrase on the kinetics and equilibrium of the oxygen isotope exchange in the CO2–H2O system: Implications for δ18O vital effects in biogenic carbonates, Geochim. Cosmochim. Ac., 95, 15–34, https://doi.org/10.1016/j.gca.2012.07.022, 2012.
Velthuys, B. R.: Electron-dependent competition between plastoquinone and inhibitors for binding to photosystem II, FEBS Lett., 126, 277–281, https://doi.org/10.1016/0014-5793(81)80260-8, 1981.
Wang, X., Wang, M., Jia, Z., Song, X., Wang, L., and Song, L.: A shell-formation related carbonic anhydrase in Crassostrea gigas modulates intracellular calcium against CO2 exposure: Implication for impacts of ocean acidification on mollusk calcification, Aquat. Toxicol., 189, 216–228, https://doi.org/10.1016/j.aquatox.2017.06.009, 2017.
Weis, V. M.: The Induction of Carbonic Anhydrase in the Symbiotic Sea Anemone Aiptasia pulchella, Biol. Bull., 180, 496–504, https://doi.org/10.2307/1542351, 1991.
Weis, V. M. and Reynolds, W. S.: Carbonic Anhydrase Expression and Synthesis in the Sea Anemone Anthopleura elegantissima Are Enhanced by the Presence of Dinoflagellate Symbionts, Physiol. Biochem. Zool., 72, 307–316, https://doi.org/10.1086/316674, 2002.
Yellowlees, D., Rees, T. A. V., and Leggat, W.: Metabolic interactions between algal symbionts and invertebrate hosts, Plant Cell Environ., 31, 679–694, https://doi.org/10.1111/j.1365-3040.2008.01802.x, 2008.
Zoccola, D., Ganot, P., Bertucci, A., Caminiti-Segonds, N., Techer, N., Voolstra, C. R., Aranda, M., Tambutté, E., Allemand, D., Casey, J. R., and Tambutté, S.: Bicarbonate transporters in corals point towards a key step in the evolution of cnidarian calcification, Sci. Rep., 5, 1–11, https://doi.org/10.1038/srep09983, 2015.