the Creative Commons Attribution 4.0 License.
the Creative Commons Attribution 4.0 License.
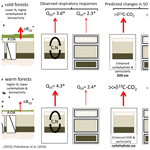
Soil profile connectivity can impact microbial substrate use, affecting how soil CO2 effluxes are controlled by temperature
Frances A. Podrebarac
Sharon A. Billings
Kate A. Edwards
Jérôme Laganière
Matthew J. Norwood
Determining controls on the temperature sensitivity of heterotrophic soil respiration remains critical to incorporating soil–climate feedbacks into climate models. Most information on soil respiratory responses to temperature comes from laboratory incubations of isolated soils and typically subsamples of individual horizons. Inconsistencies between field and laboratory results may be explained by microbial priming supported by cross-horizon exchange of labile C or N. Such exchange is feasible in intact soil profiles but is absent when soils are isolated from surrounding depths. Here we assess the role of soil horizon connectivity, by which we mean the degree to which horizons remain layered and associated with each other as they are in situ, on microbial C and N substrate use and its relationship to the temperature sensitivity of respiration. We accomplished this by exploring changes in C : N, soil organic matter composition (via C : N, amino acid composition and concentration, and nuclear magnetic resonance spectroscopy), and the δ13C of respiratory CO2 during incubations of organic horizons collected across boreal forests in different climate regions where soil C and N compositions differ. The experiments consisted of two treatments: soil incubated (1) with each organic horizon separately and (2) as a whole organic profile, permitting cross-horizon exchange of substrates during the incubation. The soils were incubated at 5 and 15 ∘C for over 430 d. Enhanced microbial use of labile C-rich, but not N-rich, substrates were responsible for enhanced, whole-horizon respiratory responses to temperature relative to individual soil horizons. This impact of a labile C priming mechanism was most emergent in soils from the warmer region, consistent with these soils' lower C bioreactivity relative to soils from the colder region. Specifically, cross-horizon exchange within whole soil profiles prompted increases in mineralization of carbohydrates and more 13C-enriched substrates and increased soil respiratory responses to warming relative to soil horizons incubated in isolation. These findings highlight that soil horizon connectivity can impact microbial substrate use in ways that affect how soil effluxes of CO2 are controlled by temperature. The degree to which this mechanism exerts itself in other soils remains unknown, but these results highlight the importance of understanding mechanisms that operate in intact soil profiles – only rarely studied – in regulating a key soil–climate feedback.
- Article
(4214 KB) -
Supplement
(623 KB) - BibTeX
- EndNote
Increased understanding of the controls on soil respiration, a globally significant flux of CO2 (Bond-Lamberty and Thomson, 2010; Stocker et al., 2013), and its response to temperature is required in developing Earth system models. Global-scale surveys suggest that temperature sensitivity of soil respiration is largely attributed to responses occurring at the level of the whole microbial community, with the greatest temperature sensitivities occurring in high C : N ratio, C-rich soils of high-latitude boreal and arctic ecosystems (Karhu et al., 2014). Congruent with these laboratory studies, temperature sensitivity of soil respiration from field experimental warming studies indicates that the greatest enhancement occurs in high latitude soils (Carey et al., 2016). Variation in high latitude soil responses may be attributed to differences in the microbial mechanisms supported within the soil horizon or collection of horizons assessed. For example, temperature sensitivity of soil respiration can increase with depth in association with a reduction in soil organic matter (SOM) bioreactivity (the ease with which it is degraded biologically). This is consistent with the idea that increased temperature sensitivity is associated with slower turnover and higher energy of activation (Ea) substrates (Conant et al., 2008; Lefevre et al., 2014; Leifeld and Fuhrer, 2005). In boreal forest soils warming appears to enhance bacterial use of labile surface soil C sources and fungal use of deeper slower-turnover soil C pools (Ziegler et al., 2013), with lower bacterial to fungal ratios associated with increases in the temperature sensitivity of soil respiration (Briones et al., 2014).
The association between soil depth or bioreactivity and temperature sensitivity of soil respiration is not ubiquitous (Fang et al., 2005; Liski et al., 1999), nor are such laboratory findings consistently supported by in situ whole-profile investigations of respiration. For example, relative to laboratory results in situ observations reveal consistent heterotrophic respiration of relatively young soil C and elevated Q10 of soil respiration to 100 cm (Hicks Pries et al., 2017). In fact, temperature responses of soil respiration observed within whole soil profiles suggest that deeper soil profiles contribute significantly to the temperature response of soil respiration (Hicks Pries et al., 2017). This raises questions regarding soil profile attributes, such as root and dissolved organic matter inputs or microbial substrate and nutrient exchange, that may control respiratory responses not revealed in commonly used laboratory experiments in which horizons are isolated.
Interactions among soil horizons may be important features driving temperature responses of the C-rich organic layers common in boreal forests. For example, the temperature sensitivity of soil respiration was up to 30 % higher in whole boreal forest organic profiles from a warmer versus colder climate despite the fact that the temperature sensitivity of soil respiration from the individual organic horizons isolated from those same organic profiles did not differ by climate (Laganière et al., 2015; Podrebarac et al., 2016). Differences in soil organic C (SOC) and soil organic N (SON) composition between climate regions were consistent with the differences in soil bioreactivity observed between regions (Fig. 1, left; Laganière et al., 2015). However, the temperature response of respiration was only consistent with soil bioreactivity when soils were incubated as whole soil profiles (Fig. 1, center; Podrebarac et al., 2016). Here the temperature response of the whole organic profile respiration was over 50 % greater than the respiration from the same soils incubated as isolated horizons (Fig. 1, middle). This suggests that the whole soil profile structure enhances microbial use of the different C or N substrates available among horizons in ways that can regulate soil respiratory responses to temperature analogous to responses observed for root exudates (Zhu and Cheng, 2011). Specifically, labile substrates from the less degraded L horizon (litter layer in the Canadian soil classification or Oi in US soil classification) may enhance the use of more complex, high Ea substrates found in the lower F or H horizons (folic and humic layers in the Canadian soil classification or Oa and Oe, respectively, in US soil classification) via microbial priming (Cheng et al., 2014; Finzi et al., 2015; Fontaine et al., 2007, 2011).
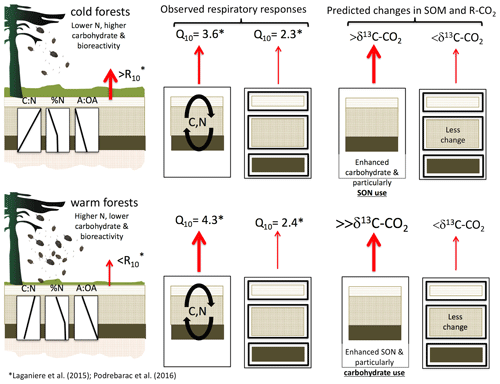
Figure 1Conceptual figure depicting differences in relatively cold and warmer boreal forest organic horizon compositions, including bioreactivity given as C-normalized soil respiration at 10 ∘C (R10; left column), previously reported temperature sensitivities of heterotrophic respiration (Q10) in two incubation designs (“whole” versus “isolated”, left to right in center column; Podrebarac et al., 2016), and hypothesized responses of incubated soils with and without interlayer exchange of microbial C and N substrates (right column). The carbon to nitrogen (C : N) ratio, nitrogen content (as % N), and the alkyl to O-alkyl ratio (A : OA) reflect the decreasing relative content of carbohydrate with depth and in the warm relative to colder climate forest sites and decreasing N content with depth and in the colder sites (left side). Enhanced temperature sensitivity of respiration in the soils incubated as a whole profile relative to those same horizons incubated individually in isolation prompted hypotheses that (1) soils incubated as a whole profile, especially from the warmer forests, would experience greater relative losses of carbohydrates signified by more 13C-enriched respired CO2 (δ13C-CO2), increased ratio of alkyl-C to O-alkyl-C (A : OA), and reductions in C : N relative to the same soils incubated as isolated horizons, signifying a greater use of labile C in support of the enhanced temperature sensitivity of respiration; and that (2) soils incubated as a whole profile, especially from the colder forests, would experience a reduction in % N as total hydrolyzable amino acids, signifying a greater use of soil organic N in support of the enhanced temperature response of soil respiration.
Understanding the presence or absence of cross-horizon substrate exchange and use will help determine the mechanisms driving soil organic matter compositional changes with temperature and those governing the temperature sensitivity of soil respiration. Differences in microbial access to distinct substrates (e.g., labile C- or N-rich compounds) may affect respiratory responses to temperature (Billings and Ballantyne, 2013) given that labile inputs such as rhizosphere C can provide a significant control on soil C and N cycling (Cheng et al., 2014; Finzi et al., 2015). For example, labile substrates mixed into soils can enhance decomposition of extant soil organic matter and the temperature response of soil respiration (Di Lonardo et al., 2019; Wang et al., 2016; Wild et al., 2016). More labile substrates are typically found in more surficial soil horizons, particularly in boreal forest podzols where thick organic horizons include a surface litter horizon. Transport of these labile substrates to zones of slower-turnover organic matter in deeper horizons occurs via mobilization of dissolved organic matter (Kaiser and Kalbitz, 2012; Kalbitz and Kaiser, 2008) or microbial use of neighboring horizons' substrates via hyphae or mycelia (Dijkstra et al., 2013; Fontaine et al., 2011). More fungal-dominated communities in surface horizons may access N-rich, higher Ea substrates from deeper soil horizons, a mechanism found to support priming effects in some soils (Li et al., 2017). In forest soils, increased N availability can enhance substrate use by bacteria relative to fungi and Actinobacteria and can also suppress soil respiration rates (Butnor et al., 2003; Ziegler and Billings, 2011; Maier and Kress, 2000). These N-driven alterations in microbial strategies and the relatively low bacterial to fungal ratios in boreal forest soils (Hogberg and Hogberg, 2002) are often associated with increased temperature sensitivity of microbial activity (Briones et al., 2014). Thus we may expect increased N to enhance temperature sensitivity of soil respiration in boreal forest soils. However, most studies exploring relevant issues leverage isolated soil layers to address this question via incubations, and when horizons are separated, the exchange and availability of labile C and N substrates across horizons are inhibited, potentially altering microbial decomposition processes and their response to temperature.
By following soil C and N use in soils from a boreal forest transect where SOC and SON compositions differ both by depth and climate region (Fig. 1, left side), we addressed two hypotheses describing how the whole soil profile connectivity, or interlayering, affects the temperature response of soil respiration (Fig. 1, center). Firstly, we hypothesized that priming of soil respiration and C loss from slower-turnover F and H horizon soils is induced by microbial use of more labile C from the overlying L horizon (e.g., leaching) and greater N availability accessed by L horizon communities from the deeper F and H horizons (e.g., fungal hyphae). These represent two possible priming mechanisms which may combine to enhance respiratory responses to increased temperature. Secondly, we hypothesize that the evidence for a labile C priming mechanism is most emergent within the warmer region soils given the lower SOC bioreactivity relative to soils from the cold region (Fig. 1, right side). However, we also anticipate that if the priming mechanism is supported by cross-horizon N availability, it would be most emergent within the colder region soils where SON availability is lower relative to the warmer region. Here we investigated whether the elevated Q10 of soil respiration within the whole organic profiles as observed in Podrebarac et al. (2016), was associated with increased use of more labile soil C or N relative to horizons incubated individually. To do so we assessed changes in soil organic matter composition and use via C : N, nuclear magnetic resonance spectroscopy (NMR), and amino acid profiling, as well as δ13C of respiratory CO2. Support for these hypotheses would suggest a potentially important means by which the temperature sensitivity of soil microbes' CO2 release may be governed, which is rarely explored.
2.1 Study area
This study was conducted using soils from the two end-member climate regions of the Newfoundland and Labrador Boreal Ecosystem Latitudinal Transect (NL-BELT) where mean annual temperature differs by approximately 5.2 ∘C and mean annual precipitation is 1074 and 1505 mm for the highest latitude region (hereafter referred to as the cold region) and lowest latitude region (hereafter referred to as the warm region), respectively (Cartwright and Doyles, NL, weather station climate normals between 1981 and 2010; Environment-Canada, 2014; Table S1). Within these two climate regions, three mesic forest sites dominated by mature balsam fir stands (Abies balsamea L.) and underlain by humo-ferric podzols were established. The forest transect sites used here, established in 2011, provide a unique opportunity to determine the impact of the climate history of soil organic matter cycling and the fate of in situ reservoirs (Ziegler et al., 2017).
2.2 Soil sampling
Soil was collected in October 2011 as described in Laganière et al. (2015). Briefly, three soil sampling plots each with a diameter of 10 m were established within each site. A 20×20 cm intact “cake” of the whole profile including the L, F, and H horizons was collected using a sharp knife and a trowel within each soil sampling plot. The Canadian soil classification of L, F, and H horizons is synonymous with Oi, Oe, and Oa sub-horizons, respectively, in the US soil classification and collectively hereafter will be referred to as the “organic profile” or the LFH. Although they are technically horizons of the O layer in the Canadian soil classification, L, F, and H will be referred to as sub-horizons from here on in order to make it easier to associate them with the more commonly used US soil classification. Mean LFH depth is 8.1 ± 0.3 cm (L 1.0 ± 0.0, F 6.1 ± 0.3, H 1.0 ± 0.0 cm) and 8.4 ± 0.4 cm (1.0 ± 0.0, 6.3 ± 0.4, 1.1 ± 0.1 cm for L, F and H, respectively) for the cold and warm regions, respectively. On site, half of the intact organic profile was separated by hand into the L, F, and H horizons and placed in a cooler while field sampling. Samples were transported to the laboratory and stored at 5 ∘C until analysis and experimental setup prior to the incubation as described in Laganière et al. (2015) and Podrebarac et al. (2016). Briefly in preparation for the incubation, L was homogenized by cutting the large soil pieces into 1 cm lengths, whereas F and H were homogenized separately by soil sampling plot through the use of a 6 mm sieve. If present, large roots (>6 mm) were removed. For the incubation, the homogenized soils were pooled by site to yield three site composite samples per region.
2.3 Soil incubations
As described in Laganière et al. (2015) and Podrebarac et al. (2016), the microcosms consist of a plastic tube (5 cm diameter × 15 cm height) with an acid-washed glass wool plug and V notches on one end to enable aeration and drainage of the soil samples (Fig. 2). The selected soil horizon(s) are placed on the glass wool horizon inside the plastic tube, and the tube is placed in a 1 L mason jar with these microcosms allowed to equilibrate for 1 week at 5 ∘C to reduce handling effects (Robertson et al., 1999). Replicate microcosms were then incubated at 5 and 15 ∘C, the average range in temperatures across the study sites in April–August (growing season). To maintain gravimetric water holding capacity at approximately 70 % for between 438 and 482 d, depending on the experiment, the soil moisture was adjusted weekly by adding water to the top of the soil core based on mass loss measured for each microcosm. Laganière et al. (2015) incubated the L, F, and H horizons separately to determine the soil bioreactivity and Q10 of soil respiration specific to each separate horizon, an approach hereafter referred to as the “isolated” experiment. In Podrebarac et al. (2016), the organic profile was reconstructed in the same proportions by mass as found in situ using the same soils used in the isolated experimental treatment, an approach hereafter referred to as the “whole” experiment (Fig. 2). Total soil mass in grams dry weight equivalent was the same across all microcosms (∼ 11 g.d.w.) and between the two experimental treatments. Actual mass of the individual L, F, and H horizons in the whole treatment totaling ∼ 11 g.d.w. was based upon the average proportion of each horizon measured from across sites in all regions (Laganière et al., 2015). In a previous study, intact soil cores of the whole organic horizon were collected at the same time as those used to obtain the homogenized separated horizons. These were incubated to compare respiratory responses among intact profiles, the reconstructed whole profiles, and those incubated as isolated horizons. This confirmed similar soil respiratory responses to temperature between the intact and reconstructed whole profiles indicating that the homogenized soils used in this study behaved similarly to the intact soil profiles from these forest sites (Podrebarac et al., 2016). Furthermore, the overall climate region differences in temperature sensitivity of forest soil respiration observed in the laboratory incubations of the intact and reconstructed whole profiles were found to be in line with field observations of total soil respiration in these forest sites (Podrebarac et al., 2016).
The temperature sensitivity of the soil respiration rates were taken directly from Laganière et al. (2015) and Podrebarac et al. (2016) and reported as Q10 simply calculated as the ratio of cumulative respiration over the course of the entire incubation at 15 ∘C over that measured in the 5 ∘C incubations. This simple approach was chosen because it avoids possible bias introduced when fitting data to a least-squares regression line (Sierra, 2012), enabling us to make a direct comparison of the temperature sensitivity of respiration among treatments within which the soil compositional results from this study can be placed.
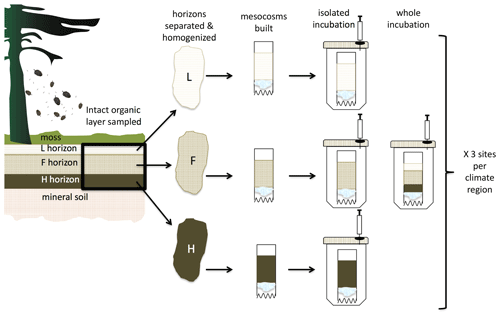
Figure 2Conceptual figure depicting the experimental design. Organic horizon soils were collected as an intact 20×20 cm layer from each of the three field sites in each climate region and separated into the individual L, F, and H (equivalent to the Oi, Oe, and Oa in US soil classification) horizons. After homogenization, soil was used as illustrated to set up two sets of incubations. The treatment labeled “isolated” incubated each soil horizon individually and in isolation. The treatment labeled “whole” incubated the L, F, and H horizons together as a whole profile layered as they are in situ and with the mass of each horizon reflecting the average proportional masses of each within the profiles observed across all the sites.
The whole experiment was incubated in triplicate by site over the more than 438 d incubation and compared with results of Laganière et al. (2015). To represent the organic profile without the cross-horizon exchange that exists naturally in situ in whole horizons, the results of the isolated experiment were summed using the same proportions of the L, F, and H horizons used in the whole experimental treatment. This summed approach is hereafter referred to as the “predicted whole” experiment. The predicted whole values for a given soil metric, such as respiration or percent carbon content (% C) was derived using Eq. (1):
where Xpredicted whole represents the value of X (e.g., % C, respiration rate, C : N) for a whole profile as predicted from measurements of X from the isolated horizons. The “M” refers to the total mass of dry soil, with subscripts designating the isolated horizon (L, F, or H) incubated. Mwhole represents the total soil dry mass for the incubated microcosm of the whole experiment. Using this equation we generated a predicted measurement of each soil metric (e.g., soil nitrogen content as % N, C : N, total hydrolyzable amino acid content, ratio of alkyl-C to O-alkyl-C) for the whole profile without cross-horizon exchange with which to make direct comparisons with values observed for the directly measured whole LFH profiles. As opposed to the instantaneous respiration rates, sampling for soil chemical composition was destructive, and soil C and N composition was not expected to change enough to warrant sampling for soil composition throughout the incubation experiment. Therefore, we limited sampling for soil composition to the end of the incubation experiment. However, we do acknowledge that the most significant differences in respiratory responses to soil temperature among treatments and sites were observed before the end of the incubation period (Fig. S3). Thus, the experimental and site effects observed in soil composition may be conservative.
2.4 Soil respiration and the δ13C of respiratory CO2
The CO2 production rate and δ13C of respiratory CO2 (δ13C-CO2) measured at six and three time points, respectively, occurred over the course of the more than 438 d incubation according to Laganière et al. (2015) and Podrebarac et al. (2016). Briefly, each microcosm was flushed with ambient air before being sealed with an airtight lid with a rubber septum. Prior to gas sampling a volume of N2 was injected into the sealed 1 L microcosm to avoid the generation of a vacuum upon sampling. A gas tight syringe was then used to sample the initial gas sample at the same volume as the injected N2. The final gas sample was collected following the same method after 16 and 4 h, respectively, for 5 and 15 ∘C treatments. The samples collected were injected into evacuated gas tight vials (Labco Limited, Lampeter, UK) and stored (less than 2 weeks) alongside standards until analysis for CO2 concentration using an Agilent 6890A gas chromatograph with a thermal conductivity detector (Agilent Technologies, Santa Clara, CA, USA). The CO2 production rate was calculated as the difference in the headspace CO2 concentration between final and initial gas samples per gram of initial soil C per unit of time (mg C-CO2 g−1 initial C h−1). In the case of the whole experiment, CO2 production was measured on day 0, 7, 42, 96, 149, 243, and 438. The CO2 production rate for the isolated experiment was measured on day 0, 7, 42, 91, 156, 245, and 482 as reported in Laganière et al. (2015). The δ13C-CO2 measured on days 0, 96, and 438 and day 0, 91, and 482, respectively, for the whole and isolated experiments was determined on an Agilent 6890 gas chromatograph (Agilent Technologies, Santa Clara, CA, USA) using a Carboxen 1010 PLOT column (30 m × 0.32 mm × 15 mm; Sigma Aldrich) interfaced to a Delta V+ isotope ratio mass spectrometer (Thermo Finnegan). To determine the δ13C of the CO2 produced via respiration over the entire incubation, a linear extrapolation through measured values over the incubation was used to obtain δ13C of respired CO2 on days not measured. These values were used to estimate the δ13C of the total cumulative CO2 over the entire incubation period using Eq. (2):
where X is the cumulative respiration (mg C-CO2 g−1 initial C) measured for a given sampling time point n. Y is the δ13C of respired CO2 at each sampling time point n.
2.5 Soil chemistry
All initial and final soil samples were analyzed for % C, % N, δ13C, δ15N, total hydrolyzable amino acids (THAAs), and relative differences in the proportion of main C functional groups via nuclear magnetic resonance spectroscopy (NMR). The O-alkyl and di-O-alkyl C proportions were tracked to assess relative changes in carbohydrate content of the soils, a labile source of C. The alkyl-C and more specifically its ratio to O-alkyl C (A : O-A) was used to assess the degradative state of soil C. The A : O-A ratio increases with soil depth and degradative state of plant tissues or soil (Preston et al., 2009, 2000). To track the degree of SON use we followed changes in the % N as THAAs and mole percent (mol %) glycine, given that amino acids make up the bulk of SON and exhibit significant losses relative to total soil N (Philben et al., 2016). Soil samples were air-dried and ground prior to analyses. The % C, % N, δ13C, and δ15N were analyzed with a Carlo Erba NA1500 Series II elemental analyzer (Milan, Italy) coupled to a Delta V Plus isotope ratio mass spectrometer via a ConFlo III interface (Thermo Scientific). Solid-state cross polarization magic-angle-spinning (CP-MAS) experiments were performed using a Bruker AVANCE II 600 MHz spectrometer with a magic-angle-spinning probe for H, C, N, and 2H (MASHCCND). Samples were run at 150.96 MHz (13C) and spun at 20 kHz at 298 K. Experiments run for each replicate sample (n=3) were each deconvoluted using a 19-component model within the “DM fit” base software (Massiot et al., 2002). Chemical shift regions assigned to the following functional groups – alkyl-C (50–0 ppm), amine + methoxy-C (65–45 ppm), O-alkyl-C (90–65 ppm), di-O-alkyl-C (110–90 ppm), aromatic C (145–110 ppm), and carbonyl-C+amide (190–165 ppm) – were expressed as percent of total area resolved (Preston et al., 2009; Wilson et al., 1987).
For the THAA analyses, ground samples (5–10 mg) were added to glass hydrolysis tubes followed by an addition of 1 mL of 6 M HCl. The hydrolysis tubes were sparged with N2, sealed with Teflon-lined caps, and heated to 110 ∘C for 20 h. Each hydrolysis tube was opened, and an aliquot of the hydrolysate was dried under a stream of N2 gas. Samples were then redissolved in 0.01 M HCl, and norvaline was added as an internal standard. Amino acids were recovered from the hydrolysate using solid-phase extraction and derivatized using the EZfaast kit for amino acid analysis (Phenomenex, USA). The derivatized samples were separated by gas chromatography using a Phenomenex ZB-AAA column (110–320 ∘C at 30 ∘C min−1) and quantified with a flame ionization detector using an HP6850 gas chromatograph (Agilent Technologies, Santa Clara, CA, USA). This resulted in 15 quantified amino acids (AAs) including alanine, glycine, valine, leucine, isoleucine, threonine, serine, proline, aspartic acid, hydroxyproline, glutamic acid, phenylalanine, lysine, histidine, and tyrosine. Glutamine and asparagine are converted to glutamic acid and aspartic acid, respectively, during hydrolysis and are included in the measurement of these amino acids. The THAA yield was expressed as a percentage of total soil C or N based on the total of all 15 AAs according to Eq. (3):
where is the C- or N-normalized yield of each AA given in milligrams amino acid per 100 mg C or N, and wt % (C or N)AA is the weight % C or N in the AAs.
2.6 Statistical analyses
The initial soil measures (C : N, % N of THAAs, mol % glycine, % alkyl-C, alkyl-C : O-alkyl-C ratio (A : OA), % di-O-alkyl-C, δ13C, and δ15N) were analyzed using a two-way ANOVA to test the effects of climate region, horizon, and their interaction term to quantify meaningful differences in soil properties prior to these experiments. These are expected to support previous observations of lower N, elevated carbohydrates (lower A : OA and % alkyl, higher % di-O-alkyl-C), and higher bioreactivity in the colder relative to warmer region forest soils (Fig. 1, left side). Previous work investigated the impact of the climate region on the soil respiratory responses to temperature (Laganière et al., 2015; Podrebarac et al., 2016). This work indicated no real difference in respiratory responses to temperature between the colder versus warmer forest soils when horizons were incubated in isolation. In contrast, and consistent with the differences in bioreactivity and soil chemical properties, a larger temperature response was observed in the warmer relative to colder forest soils when soils were incubated as a whole organic profile as layered in situ (Fig. 1, center). This study focuses on the mechanisms controlling those different responses, which appear dependent upon the layering of the soil horizons. As such, we focus on the impact of the intact nature of whole soil horizons on the temperature responses of soil respiration and its relationship to C and N substrate use. To investigate how microbial use of C and N components of the SOM pools relate to the previously reported Q10 of soil respiration (Laganière et al., 2015; Podrebarac et al., 2016), the soil mass loss, loss of C and N, change in soil composition (Δ of C : N, δ13C, δ15N, THAAs, NMR-resolved C chemistry), and the δ13C-CO2 were tracked over the incubation within the (1) isolated experiment, (2) whole experiment, and (3) predicted whole experiment based on the isolated experimental results. Given our two hypotheses, we expected to observe a more 13C-enriched respired CO2, greater relative losses of carbohydrates signified by increased % alkyl and/or A : OA and decreased % di-O-Alkyl, and reductions in C : N in the whole soil relative to the isolated soil incubations, signifying microbial use of labile C resulting in the enhancement of observed temperature sensitivity of CO2 release. We also expected to observe greater reduction in % N as THAAs and increase in mol % glycine in the whole relative to the isolated soil incubations, signifying microbial use of SON that promoted the enhanced temperature sensitivity. Finally, we anticipated that the enhanced use of labile C and SON would be most emergent within the warm and cold forests, respectively, as a result of the climate region differences in soil C and N (Fig. 1, right side). The difference between the initial and final soil composition metric (given as final−initial = Δ) was calculated, with negative values indicating a decline in the value over the incubation (e.g., a Δ of −10 for soil C : N indicates that C : N decreased by 10 units). Due to large standard errors associated with some of the changes in composition, Student's t test was used to determine if the initial and final soil values were significantly different from each other (i.e., if Δ was different from zero). Because of a significant effect of region on multiple response variables (see “Results”), we performed region-specific tests to assess mechanisms responsible for respiratory responses. For the isolated experiment, the effect of incubation temperature, horizon, and their interaction was assessed using a two-way ANOVA in which levels of temperature were 5 and 15 ∘C and horizons were defined as L, F, and H within each climate region. Using both the isolated and whole horizon treatments we explored the effect of incubation temperature, experimental type (i.e., whole, isolated), and their interaction using a two-way ANOVA within the climate region. Here, a significant effect of experimental type denotes some impact of cross-horizon exchange on SOM processing.
For tests in which residuals did not meet the assumptions of normality and homoscedasticity, data were log10-transformed prior to testing (Zar, 1999). The Tukey's honestly significance differences test was used to determine which combination of treatments or effects were significantly different. All statistical analyses were performed using R with a significance threshold set at 0.05 (R-Core-Team, 2017, 2014).
3.1 Initial soil organic matter chemistry and nitrogen differ between the cold and warm regions
The initial soil N content in the present study was greater in the warm relative to the cold region (p<0.0001), consistent with soil sampled in earlier studies from the same climate regions (Philben et al., 2016). The initial soil C : N ranged from 32 to 54. These ratios did not exhibit any region by horizon effects (p=0.1272) but were lowest in the warm region (p<0.0001) and decreased with depth (p<0.0001; Fig. 3a). The initial % N as THAAs ranged from 38 % to 43 %, was similar in soils for the two climate regions, and decreased with depth (p=0.0011; Fig. 3b) in a manner consistent with increasing soil organic N degradation with depth (Philben et al., 2016). Congruent with this feature, mol % glycine increased with depth (p<0.0001) and was elevated in the warmer region soils (p=0.0035) and to a greater extent within the H horizon (p=0.0126), and cold region soils exhibited a lower soil organic N degradative state relative to the warm region (Fig. 3c;. Philben et al., 2016). The initial % alkyl-C ranged from 27 % to 33 % and exhibited a region by horizon interaction (p=0.0443), revealing higher values in F relative to L and H relative to F horizons for the warm region (Fig. 3d). Higher % alkyl-C was observed in L and H of the warmer region (p=0.0301) that increased with depth (p=0.0137). The initial % di-O-alkyl-C, ranging from 7 % to 9 %, was elevated in the cold region soils (p<0.0001; Fig. 3e) but exhibited no other trends. The A : O-A ratio exhibited a regional (p=0.0014) effect only with an elevated ratio in warm relative to cold region soils (Fig. 3f).
3.2 Losses of soil mass and declines in soil C and N concentrations were enhanced by increased temperature and to a greater extent in the cold region soils
Over the more than 438 d incubation, mass, % C, and % N losses were greatest in the 15 ∘C incubation and for the cold region soils (Table 1). In the isolated experiment, mass loss ranged from 16 ± 1 % to 39 ± 5 % and 16 ± 3 % to 30 ± 2 % (mean ± standard error), respectively, for the cold and warm regions. The % C loss ranged from 21 ± 3 % to 82 ± 4 % in the cold region soils with 82 % loss at 15 ∘C in the F sub-horizon. The warm region soils exhibited a % C loss that ranged from 24 ± 5 % to 46 ± 3 %. The % N loss in the cold region soils ranged from 11 ± 9 % to 45 ± 5 % and was greatest in the 15 ∘C treatment. In the warm region % N loss ranged from 13 ± 3 % to 52 ± 23 % and did not exhibit a temperature effect (p=0.227). In the whole organic profile (whole experiment) and the predicted whole experiment soils, mass loss was greatest at 15 ∘C and ranged from 16 ± 2 % to 37 ± 1 % and 12 ± 2 % to 28 ± 2 %, respectively, for the cold and warm regions. In the warm region, mass loss was greater in the predicted experiment relative to the whole profile experiment (p=0.011). The effect of temperature and experiment was observed for % C loss in the cold and warm regions, with the greatest % C losses having occurred at 15 ∘C and in the predicted whole experiment. The % N loss in these whole profile experiments ranged from 11 ± 4 % to 38 ± 5 % in the cold climate soils in which greater loss was observed at 15 ∘C, yet no experimental effect was observed (p=0.119). The warm climate soils exhibited a similar range in % N loss from 13 ± 3 % to 40 ± 13 % with no temperature (p=0.195) or experimental effect (p=0.064).
Table 1The mean (standard error) of mass, % C, and % N loss within each experiment in which individual horizons were incubated in isolation from each other (isolated), then calculated as whole profile values based upon those isolated horizon results (predicted) and given also as the actual measured incubation results for whole organic profiles (measured). The effect of temperature (T), horizon (H), and interaction term (T×H) are given for the isolated experimental results (top). The effect of T, experiment (E), and interaction term (T×E) are given for the tests conducted across both the predicted and measured whole profile experimental treatments (bottom). Significance (α=0.05) is denoted in bold.
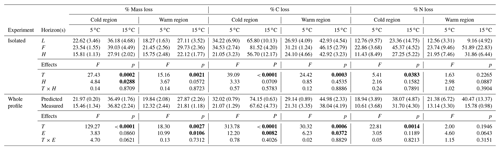
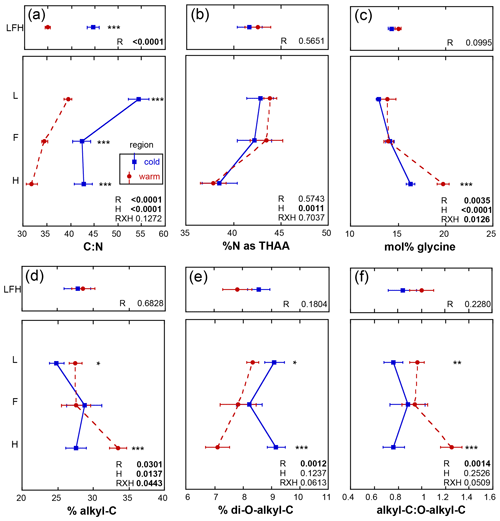
Figure 3Initial mean values ± standard error for soil metrics from each of the three sites given as the whole organic profile (LFH; upper panels) and individual horizons (L, F, H) from the isolated experiment (lower panels). An analysis of variance was used to assess the effect of region (R) within the organic profile, and the effect of R, horizon (H), and their interaction within the individual horizons in which the significance of the effect of R within the horizon is denoted with the following asterisks: p≤0.0001, p≤0.001, * p≤0.05, and n.s. (not significant): p>0.05.
3.3 The δ13C of the cumulative respired CO2 increased in whole-profile incubations and with incubation temperature
The δ13C of respired CO2 increased throughout the incubation period. However, an exception to this includes all the soil horizons from the cold region incubated within the isolated experiment and, consequently, the predicted whole experimental treatment (Fig. 4). The δ13C of respired CO2 over the course of the incubation ranged from −29 ‰ to −24 ‰, with more 13C-enriched respired CO2 released from the deeper soil horizons and soils exposed to the higher incubation temperature. Overall the effect of temperature (p=0.0024), experiment (p<0.0001), and their interaction (p<0.0001) was observed in the cold region soils, while only the effect of experiment (p=0.0010) was observed in the warm region soils. Regardless of region or temperature, the whole experiment exhibited more 13C-enriched respired CO2, consistent with the impact of increased temperature across all soils and experiments. For example, the whole experiment incubations at both temperatures exhibited a 3 ‰–4 ‰ increase in δ13C-CO2 relative to both the initial and the isolated experiment at 5 ∘C (Fig. 4b). In contrast, the cold region soils incubated as individual L, F, and H horizons exhibited a ∼ 3 ‰ increase in δ13C-CO2 in the 15 ∘C relative to both the initial values and the 5 ∘C incubations (Fig. 4a). These results indicate that the whole profile structure enhanced the respiration of more 13C-enriched substrates to an extent similar to that observed with increased incubation temperature. Furthermore, the respiration of more 13C-enriched substrates was associated with higher Q10 of soil respiration regardless of climate region.
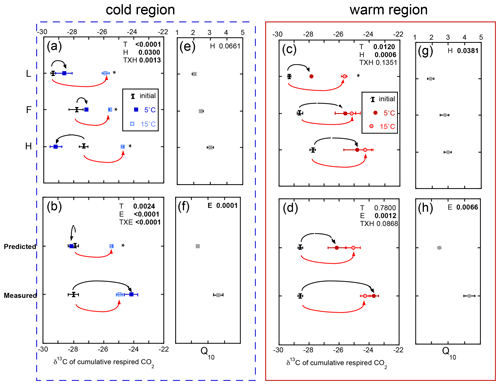
Figure 4The δ13C of the total cumulative respired CO2 comparing individual horizons incubated in isolation (isolated experiment; L, F, and H; a, c), calculated as a whole profile from those isolated horizons (predicted), and measured directly as a whole-profile (measured; b, d). The corresponding temperature sensitivity (Q10; e, f, g, h) of the total cumulative respiration for the same incubation period. Values are provided separately for the soils from the cold (blue, left) and warm regions (red, right). Values are given as the mean of the three sites ± standard error with the initial bulk soil δ13C included for reference (I). The effects of temperature (T), horizon (H), or experiment (E) and their interaction term (T×H or T×E) are given for all three effects, with significance (α=0.05) denoted in bold. Any significant effect of temperature within horizon (a, c) or within experiment (b, d) is denoted with an asterisk.
Though initial soil organic matter δ13C and δ15N differed by climate region, the changes in soil δ13C and δ15N over the course of the incubation were relatively small (typically less than 1 ‰) across all horizons and with both temperature and experimental types (Table S2). No temperature, horizon, or experimental effect was observed in the changes in bulk soil of δ13C and δ15N in these soils.
3.4 Degradation of soil nitrogen occurred to a greater extent in the cold relative to the warm region soils but exhibit no response to whole profile structure
The magnitude of change in C : N (ΔC : N) over the course of the experiment decreased with soil depth regardless of region (Fig. 5a–d). Increased temperature resulted in a greater decrease in soil C : N with decreases of 3–14 and 0–9 observed in the cold and warm regions, respectively, over the course of the entire incubation (Fig. 5a–d). In the cold region where the soil C bioreactivity is generally greater (Laganière et al., 2015), the effect of incubation temperature on C : N was similar across both the predicted and measured whole experiments, suggesting that soil profile structure was not an important factor for this variable. However, in the warm region soils, the decrease in C : N was evident in the individual L and H horizons, as well as the whole profile experiment but not when calculated as a whole profile from the individual horizons. Although a temperature effect was noted within all soil profile experimental treatments of the cold and warm region soils, no effect of soil profile experimental treatment was detected for the ΔC : N within either region. Change in % C as THAAs was only noted in the F horizon of the warm region soils incubated at 15 ∘C (Fig. S1). No effects of temperature, horizon, or experiment were observed for changes in % C as THAAs. Change in % N as THAAs was variable but exhibited temperature effects consistent with the ΔC : N in the cold climate whole profile soils whether incubated as a whole profile or predicted from the isolated horizons (Fig. 6a–d). Changes in mol % glycine were also consistent with these observations of soil organic N processing in the cold region soil (Fig. 6e–h). The mol % glycine, an indicator of greater microbial reworking of soil organic N (Dauwe and Middelburg, 1998; Hedges et al., 1994; Philben et al., 2016), increased with temperature in the L and H horizons, as well as the whole organic profiles from the cold region. In the warm region soils, temperature effects were only noted in the isolated experiment in which decreases in mol % glycine were observed in the 5 ∘C relative to 15 ∘C incubation temperatures (p=0.0028). This temperature effect was observed within the H horizon (p=0.0058), indicating a relative increase in final mol % glycine following incubation at the higher relative to lower incubation temperature.
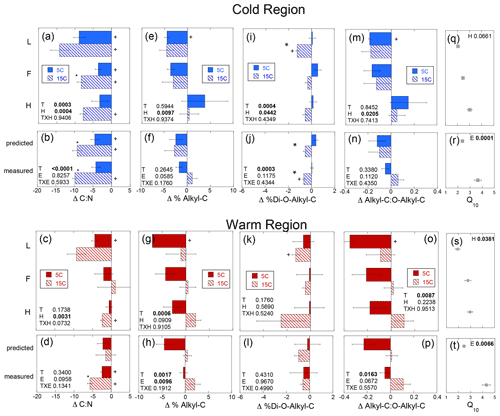
Figure 5Change in the soil C to N ratio (Δ C : N), percent of soil carbon as alkyl-C (Δ % Alkyl-C) and as di-O-alkyl-C (Δ % Di-O-Alkyl-C), and the ratio of alkyl-C to O-alkyl-C (Δ Alkyl-C : O-Alkyl-C) given as the difference between the absolute final minus initial values for the soils incubated as individual horizons in isolation from each other (upper panels labeled by horizon: L, F, and H) and those calculated as whole profile values based upon those isolated horizon results (predicted) and those measured directly from the incubation of whole organic profiles (measured). These results are given for both the cold (a, b, e, f, i, j, m, n) and warm regions (c, d, g, h, k, l, o, p). The corresponding temperature sensitivities of the total cumulative respiration for the same incubation period (Q10; cold region, q and r; warm region, s and t) taken from Podrebarac et al. (2016) are provided for reference. All values provided are the mean of the three sites ± the standard error with a significant change from 0 denoted by symbol “+”. Any significant (α≤0.05) effect of temperature (T), horizon (H), or experiment (E) or interaction term (T×H or T×E) is denoted in bold. A significant effect of temperature within the horizon or within the experiment is denoted by an asterisk.
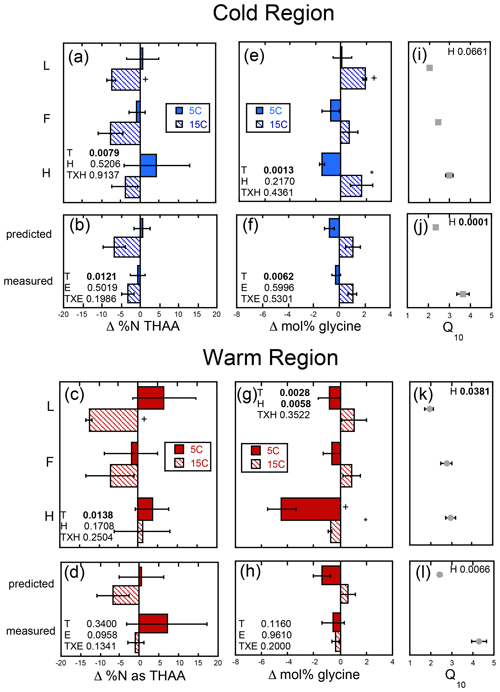
Figure 6The change in soil % N as total hydrolyzable amino acid (Δ % N as THAAs) and mole percent glycine (Δ mol % glycine) given as the difference between the absolute final minus initial values for the soils incubated as individual horizons in isolation from each other (upper panels labeled by horizon: L, F, and H) and those calculated as whole profile values based upon those same isolated horizon results (predicted) and those measured directly from the incubation of whole organic profiles (measured). These results are given for both the cold (a, b, e, f) and warm regions (c, d, g, h), and the corresponding temperature sensitivity of the total cumulative respiration for the total incubation period (Q10; cold region, i and j; warm region, k and l) taken from Podrebarac et al. (2016) are provided for reference. All values provided are the mean of the three sites ± the standard error with a significant change from 0 denoted by symbol “+”. Any significant (α≤0.05) effect of temperature (T), horizon (H), or experiment (E) or interaction term (T×H or T×E) is denoted in bold. A significant effect of temperature within horizon or within experiment is denoted by an asterisk.
3.5 Change in soil carbon chemistry was affected by both incubation temperature and whole soil profile structure
Changes in the alkyl-C to O-alkyl-C ratio (ΔA : O-A) were observed within the L horizons from both climate regions. However, temperature effects on the ΔA : O-A (p=0.0087) were noted only in the warm region soils and horizon effects (p=0.021) only in the cold climate soils (Fig. 5m–p). Unexpectedly, % alkyl-C decreased (Fig. 5e–h), while % O-alkyl-C increased in the warm region soils over the incubation at 5 ∘C resulting in the decreases in the A : O-A ratio (Fig. 5m–p). Decomposition of vascular plant tissues typically results in increases in A : O-A as a result of losses in carbohydrates (rich in o-alkyl-C) and relative retention of plant aliphatics (rich in alkyl-C) (Preston et al., 2009, 2000). This unexpected finding is perhaps a result of the significant surface inputs of moss tissues rich in structural carbohydrates that can be resistant to decomposition (Turetsky et al., 2008; Hájek et al., 2011; Philben et al., 2018). The cold region soils exhibited a horizon effect on the change in % O-alkyl-C (p=0.026) and ΔA : O-A (p=0.021) such that % O-alkyl-C generally increased and A : O-A decreased in the L sub-horizon, while % O-alkyl-C decreased and A : O-A increased in the H sub-horizon over the incubation (Fig. 5e). In the warm region soils, increased incubation temperature appeared to have eliminated these unexpected changes in % alkyl-C, % O-alkyl-C, and the A : O-A ratio. No change in % alkyl-C, % O-alkyl, or A : O-A was noted over the course of the 15 ∘C incubation. However, the unexpected trend of decreasing A : O-A and % alkyl-C was not observed in the warm climate soils incubated as whole soil profiles. An effect of both temperature (p=0.0017) and experiment type (i.e., whole versus isolated) (p=0.0096) was noted in the change in % alkyl-C over the incubation period. This was observed as an increase in % alkyl-C within the whole profile experiment only when incubated at 15 ∘C as opposed to the reduction in % alkyl-C at 5 ∘C and no change at 15 ∘C in the predicted whole profile (Fig. 5e–h). No experimental effects were noted for either the changes in A : O-A (p=0.067) or % O-alkyl-C (p=0.143) within the whole soil profiles from the warm region. The % di-O-alkyl-C exhibited a decrease only following the 15 ∘C incubation of the L horizons from both climate regions (Fig. 5i, k). However, the isolated horizons from the cold region exhibited both a temperature (p=0.0004) and horizon (p=0.044) effect, indicating decreases in % di-O-alkyl-C with warming primarily within the L horizon. Further, a temperature effect (p=0.0003), observed as a decrease in % di-O-alkyl-C, was also observed in the whole soil profiles from the cold region regardless of experiment type (Fig. 5j). The relative changes in the other carbon types as detected via 13C-NMR were generally below detection. However, we did note increases in % aromatic C over the course of the 5 ∘C incubation of the warm region soils, consistent with the proportional decreases as observed in % alkyl-C (data not shown).
Prior work at these same sites demonstrated how the temperature response of soil respiration can be enhanced in warmer relative to colder mesic boreal forest region soils but only when horizons were incubated together and layered as a whole soil profile, congruent with field observations of soil respiration in the same forest sites (Fig. 1, center; Podrebarac et al., 2016). Isolated horizons of the same soils, under the same conditions, exhibited lower respiratory responses to increased temperature and no regional climate differences despite clear differences in soil bioreactivity between the two climate regions (Laganière et al., 2015; Podrebarac et al., 2016). These previous studies suggest that some mechanism facilitated by the connectivity among soil horizons occurring within whole profiles can enhance the temperature sensitivity of soil respiration over weekly to monthly timescales. Here, we explore the potential role of soil priming as a mechanism supporting the enhanced temperature responses of respiration in these whole soil profiles. We found evidence for labile C but not labile N priming of microbial activities, supported by soil horizon connectivity that indirectly stimulates respiratory responses to increased temperature (Fig. 1, right). Additionally, the data indicate that the labile C priming mechanism is most emergent in the warmer region soils, consistent with lower SOC bioreactivity in the warmer region relative to soil from the colder region.
4.1 Enhancement of microbial labile carbon use supports an indirect mechanism for increased temperature sensitivity of soil respiration in whole organic soil profiles
The inclusion of relatively faster-turnover soil substrates from the surface L horizons with the slower-turnover pools within the lower F and H horizons promoted microbial use of more bioreactive substrates and an enhanced soil respiratory response to temperature in the whole soil profiles studied. Enhanced temperature sensitivity of microbial activity supported indirectly through increased availability of labile substrates, or a priming effect, has been observed with root exudates and other fresh plant inputs (Curiel Yuste et al., 2004; Zhu and Cheng, 2011), but, to the best of our knowledge, it has not yet been linked to soil profile connectivity. These whole soil profiles exhibited increases in the δ13C of respired CO2 and relatively enhanced decomposition of carbohydrates compared to soil horizons incubated in isolation. These increases were associated with the enhanced temperature response of respiration in the whole organic profiles not predicted by the response of the same individual horizons incubated in isolation. Being most strongly observed in the warm region forest soils in which composition metrics indicate initially lower bioreactivity, the enhanced temperature sensitivity of respiration with the whole soil profile structure appears to be largely supported by labile C priming as we had hypothesized. These respiratory responses to cross-horizon exchange were observed throughout the incubation (Podrebarac et al., 2016) and not just at the end when we were able to observe soil chemical composition. This highlights the challenges for in situ profile studies for which a combination of short- and longer-term investigations are needed to capture labile C priming influences on soil substrate use and respiratory responses to temperature.
Enhanced carbohydrate use suggests that catabolism of more bioreactive, 13C-enriched substrates supported the use of lower Ea substrates, helping to explain the elevated Q10 of soil respiration within the whole profile soils relative to the sum of the individual horizons from the same profiles. Soil substrates are not uniformly available to microbes, and both temperature and changes in the suite of available substrates can alter the catabolism or incorporation of soil substrates by microbes (Bölscher et al., 2017; Fontaine et al., 2007; Frey et al., 2013; Streit et al., 2014; Zogg et al., 1997). During incubation, catabolism of more recent, fast-turnover soil inputs relative to the bulk soil can occur along with an increased proportion of older, slower-turnover soil substrates incorporated into microbial biomass (Blagodatskaya et al., 2011). The increased δ13C of respired CO2 observed within the whole profile experiment, independent of region or incubation temperature, was congruent with increases in δ13C of respired CO2 observed with incubation temperature in the isolated horizons (Fig. 4). These increases exceed those typically attributed to isotopic discrimination associated with respiration (<1 ‰; Breecker et al., 2015; Czimczik and Trumbore, 2007) and likely resulted from enhanced use of more 13C-enriched substrates, increased reuse of bioreactive C pools incorporated into microbial biomass, and/or shifts in microbial composition of the active community (Blagodatskaya et al., 2011).
Congruent with the increased δ13C of respired CO2, the relative retention of alkyl-C observed in the warmer region soils was likely a consequence of the enhanced use of carbohydrates which are relatively 13C-enriched components of soil organic matter (Benner et al., 1987; Hobbie and Werner, 2004). When the soil horizons were incubated at 5 ∘C as isolated horizons, we observed the opposite of the typical relative loss of carbohydrates (reduced O-alkyl-C) and retention of plant waxes (retained alkyl-C) associated with the decomposition of vascular plant tissues (Preston et al., 2000, 2009). The unexpected trend of decreasing alkyl-C and A : O-A, observed in the 5 ∘C incubation of the cold and warm region L horizons, was notably absent in the 15 ∘C incubations of warm region soils (Fig. 5). The experimental effect on the change in alkyl-C indicated that the whole horizon structure enhanced decomposition which is more typical of increased carbohydrate use which was further enhanced with increased temperature. The lack of change in % alkyl-C or % O-alkyl-C within the cold region soils likely resulted from the larger relative concentration of carbohydrates (Fig. 3e, f), a consequence of the greater moss contributions to these soils relative to the warm forest soils (Kohl et al., 2018). The cold region profiles lack the increase in A : O-A with depth observed in the warm region profiles and typically observed in vascular-plant-dominated soils including boreal forests (Kane et al., 2010). Therefore, the increases in δ13C-CO2 associated with temperature and the proximity of horizons within the whole profile may still have been due to enhanced mineralization of carbohydrates in the cold region soils not clearly detected in bulk changes in the chemistry of the SOM.
Microbial use or catabolism of lower Ea compounds (e.g., carbohydrates) in support of microbial responses to increasing temperature likely enhanced substrate assimilation and use efficiency in the whole profile soils. The enhanced labile substrate use and temperature response of soil respiration in the whole profile incubations coincided with lower soil C losses (Table 1) as compared with the isolated horizons. This could be explained by a priming effect within these soil profiles enhancing the use of more complex higher Ea substrates consistent with respiratory temperature responses closer to intrinsic values relative to those of isolated horizons lacking this priming effect (Davidson and Janssens, 2006). Root exudates have been found to increase the availability or use of complex, high Ea substrates via a priming effect (Bingeman et al., 1953; Cheng et al., 2014) by accelerating SOM decomposition via co-metabolism or the increased production of polymer-degrading enzymes breaking down macromolecules and generating more soluble molecules (Schimel and Weintraub, 2003; Wallenstein and Weintraub, 2008; Zhu and Cheng, 2011). Similarly, the whole soil profiles likely promoted availability of a diversity of substrates and activity of more diverse microbes than in isolated horizons, supporting co-metabolism or increased polymer-degrading enzyme activity (Basler et al., 2015), as has been noted with litter additions to soil (Malik et al., 2016).
The whole profile structure studied here results in the contact between communities with high fungal to bacterial ratios (F:B) within carbohydrate-rich L horizons with communities exhibiting low F:B within less carbohydrate-rich F and H horizons (F:B L>F>H in these forest soils; Kohl et al., 2015). Relative to bacteria, fungi can exhibit greater substrate use efficiencies (Bölscher et al., 2016; Kallenbach et al., 2016), and their hydrolytic activities may support the cross-horizon enhancement of substrate use, including higher Ea substrates in these organic horizons, congruent with the reduced respiration rates and enhanced carbohydrate decomposition observed in the whole profile soils. By initiating key steps in the decomposition of more complex soil organic matter (Paterson et al., 2008), fungi can enhance the decomposition of higher Ea substrates found within the F and H layers and likely to a greater extent when incubated in contact with the fungal-rich L layer. Enhanced fungal enzyme activity is not exclusive of enhanced bacterial respiration and use of carbohydrates in these soil profiles; rather enhanced bacterial relative to fungal respiration could explain the increases in δ13C of respired CO2 observed in the whole profiles (Dijkstra et al., 2006; Glaser and Amelung, 2002). This suggests that enhancement in bacterial relative to fungal respiration, and specifically bacterial catabolism of carbohydrates, occurred in the whole soil profiles, supporting the idea of a labile C priming effect.
4.2 Enhanced temperature sensitivity of respiration is not associated with enhanced N use in whole soil profiles
Soil N availability can impact the soil microbial community, its substrate use and growth efficiency (Blagodatskaya et al., 2014; Mooshammer et al., 2014), and priming effects supported by fungi (Dijkstra et al., 2013). Therefore, we additionally hypothesized that enhanced soil N availability supported by the whole soil profile structure has the potential to enhance the temperature response of soil respiration given differences in soil N content and microbial community composition by horizon. Soil N content also differed by climate region in these forests, providing us with an opportunity to assess the role of soil N exchange and use across a climate-relevant range of soil N availability in this boreal forest region (Philben et al., 2016). Substrate use could therefore be influenced by exchange across horizons as both fungal abundance and soil organic N concentration and composition vary with depth in most soil profiles and particularly in the boreal forest organic horizons explored here (Fig. 1, left side; Kohl et al., 2015; Philben et al., 2016).
Despite the observed temperature effects on soil N losses being consistent with enhanced N2O production with warming observed in these organic horizons (Buckeridge et al., 2020) and clear differences in availability of soil N represented by the two climate region soils, we observed no difference in soil N use between soils incubated as a whole profile versus as isolated horizons. In particular the lower N availability of the cold region soils, indicated by lower initial % N and higher C : N (Fig. 3), suggests that if we were to see an enhancement in soil organic N use, it would likely have been observed in the cold region soils as noted. However, use of soil organic N in the colder region with increased incubation temperature was not impacted by whether soil horizons were incubated in isolation or connected as a whole profile. The observed changes in % N, C : N, and indicators of amino acid degradation (% N as THAAs, mol % glycine) were similar in both soil profile treatments of the cold region soils (Fig. 6). Congruent with the greater bioreactivity and soil C respiratory losses observed in the cold relative to warm region soils (Laganière et al., 2015), decreases in soil C : N were primarily observed in the cold region soils in which soil C : N was initially higher and temperature effects were noted regardless of whether soils were incubated as isolated horizons or as a whole profile. This contrasted with the warm region soils in which the change in C : N only differed with incubation temperature in the whole profiles, consistent with the carbohydrate losses.
We observed some increased degradation of amino acids in the isolated horizons during the incubation. For example, decreases in % N as THAAs were detected in the isolated L horizons from both the warm and cold regions, indicating that we were able to detect soil N use over the incubation period used, and we found that the greater availability of N in the surface L horizons supports enhanced SON use with increased temperature but without necessarily impacting overall N use in the whole soil profiles. These results suggest that the enhanced temperature sensitivity of soil respiration observed in the whole profiles relative to the sum of their isolated horizons is not due to changes in N substrate use but rather solely changes in C substrate use facilitated by the whole profile structure. This is likely attributed to enhanced use of labile, high C : N substrates in the surface L horizons facilitating or priming efficient use of slower-turnover soil organic matter in the lower horizons. Interestingly, this did not lead to enhanced use of SON, indicating the efficient use and recycling of N or a priming of primarily C-rich substrates in lower soil horizons.
These findings highlight that soil horizon connectivity can impact microbial substrate use in ways that affect how soil effluxes of CO2 are controlled by temperature. Partially supporting our two hypotheses, we first demonstrate how inter-layering of soil horizons as a whole profile, and as found in situ, supports cross-horizon exchange enhancing the use of labile C (but not N) and respiratory responses to temperature (Fig. 1). Secondly, we found this feature was most emergent in the warmer forest soils, as predicted and consistent with these soils' lower C bioreactivity relative to soils from the colder region. Thus we reveal an additional mechanism that can control in situ respiratory responses to changing temperature, an important soil–climate feedback. This result further explains discrepancies between laboratory and in situ observations of the temperature sensitivity of soil respiration highlighting the role of in situ processes that must be captured to better predict this feedback within Earth system models. Given that recent photosynthates, e.g., root exudates, can contribute similarly to soil respiration across the surface and to deep horizons (Pumpanen et al., 2009), the role of a priming mechanism suggested by our study is worthy of investigating in deeper mineral soil profiles in which the enhanced temperature sensitivity of soil respiration is supported by soil C of recent origin (Hicks Pries et al., 2017). Deeper soil profiles are key to uncovering the full soil response to climate change, and understanding controls on those responses requires an increased understanding of the cross-horizon exchange processes suggested by this study. For example, root inputs and hydrologic regimes transferring dissolved organic matter and nutrients, as well as regulating redox conditions, represent relevant factors likely controlling interactive effects of cross-horizon exchange on soil C use. The degree to which this mechanism exerts itself in other soils remains unknown, but these results highlight the importance of understanding priming mechanisms that operate within whole soil profiles – only rarely studied – in regulating respiratory responses to changing temperature.
All data are included in the paper's tables and the Supplement.
The supplement related to this article is available online at: https://doi.org/10.5194/bg-18-4755-2021-supplement.
Authors JL, SAB, and SEZ contributed to the general conceptions of the study. SAB, KAE, JL, and SEZ designed the sampling. KAE, JL, and SEZ contributed to field sample collections, while incubation experiment setup and sampling were conducted by FAP and JL. FAP, JL, and MJN contributed to sample analyses, including soil CO2 fluxes, sample extractions, and preparations for isotope and elemental analyses, NMR, and amino acids. FAP conducted data and statistical analyses. FAP and SEZ jointly wrote the manuscript which received edits from all co-authors.
The authors declare that they have no conflict of interest.
Publisher’s note: Copernicus Publications remains neutral with regard to jurisdictional claims in published maps and institutional affiliations.
We thank Darrell Harris, Andrea Skinner, and Thalia Soucy Giguere for fieldwork assistance and Rachelle Dove, Jamie Warren, and Catie Young for laboratory assistance. NMR analyses were conducted by Céline Schneider and δ13C of CO2 was analyzed by Geert Van Biesen in the CREAIT network at Memorial University. Funding was generously provided by NSERC-CRSNG (SPG#479224; DG#2018-05383); NSERC CREATE Program; Canadian Forest Service, Natural Resources Canada; Forestry and Agrifoods Agency, Government of Newfoundland and Labrador; and the Canada Research Chairs Programme.
This research has been supported by the Natural Sciences and Engineering Research Council of Canada (grant nos. SPG#479224 and DG#2018-05383), the Forest and Agrifoods Agency, Government of Newfoundland and Labrador (grant no. SPG#479224), and Natural Resources Canada (grant no. SPG#479224).
This paper was edited by Michael Bahn and reviewed by Birgit Wild and one anonymous referee.
Alster, C. J., Fischer, von, J. C., Allison, S. D., and Treseder, K. K.: Embracing a new paradigm for temperature sensitivity of soil microbes, Glob. Change Biol., 26, 3221–3229, https://doi.org/10.1111/gcb.15053, 2020.
Basler, A., Dippold, M., Helfrich, M., and Dyckmans, J.: Microbial carbon recycling – An underestimated process controlling soil carbon dynamics – Part 1: A long-term laboratory incubation experiment, Biogeosciences, 12, 5929–5940, https://doi.org/10.5194/bg-12-5929-2015, 2015.
Benner, R., Fogel, M., Spargue, K., and Hodson, R.: Depletion of 13C in lignin and its implications for stable carbon isotope studies, Nature, 329, 708–710, https://doi.org/10.1038/329708a0, 1987.
Billings, S. A. and Ballantyne, F.: How interactions between microbial resource demands, soil organic matter stoichiometry, and substrate reactivity determine the direction and magnitude of soil respiratory responses to warming, Glob. Change Biol., 19, 90–102, https://doi.org/10.1111/gcb.12029, 2013.
Bingeman, C. W., Varner, J. E., and Martin, W. P.: The Effect of the Addition of Organic Materials on the Decomposition of an Organic Soil, Soil Sci. Soc. Am. J., 17, 34–38, https://doi.org/10.2136/sssaj1953.03615995001700010008x, 1953.
Blagodatskaya, E., Yuyukina, T., Blagodatsky, S., and Kuzyakov, Y.: Three-source-partitioning of microbial biomass and of CO2 efflux from soil to evaluate mechanisms of priming effects, Soil Biol. Biochem., 43, 778–786, https://doi.org/10.1016/j.soilbio.2010.12.011, 2011.
Blagodatskaya, E., Blagodatsky, S., Anderson, T.-H., and Kuzyakov, Y.: Microbial growth and carbon use efficiency in the rhizosphere and root-free soil, PLoS ONE, 9, e93282, https://doi.org/10.1371/journal.pone.0093282, 2014.
Bond-Lamberty, B. and Thomson, A.: A global database of soil respiration data, Biogeosciences, 7, 1915–1926, https://doi.org/10.5194/bg-7-1915-2010, 2010.
Bölscher, T., Wadso, L., Borjesson, G., and Herrmann, A. M.: Differences in substrate use efficiency: impacts of microbial community composition, land use management, and substrate complexity, Biol. Fert. Soils, 52, 547–559, https://doi.org/10.1007/s00374-016-1097-5, 2016.
Bölscher, T., Paterson, E., Freitag, T., Thornton, B., and Herrmann, A. M.: Temperature sensitivity of substrate-use efficiency can result from altered microbial physiology without change to community composition, Soil Biol. Biochem., 109, 59–69, https://doi.org/10.1016/j.soilbio.2017.02.005, 2017.
Breecker, D. O., Bergel, S., Nadel, M., Tremblay, M. M., Osuna-Orozco, R., Larson, T. E., and Sharp, Z. D.: Minor stable carbon isotope fractionation between respired carbon dioxide and bulk soil organic matter during laboratory incubation of topsoil, Biogeochemistry, 123, 83–98, https://doi.org/10.1007/s10533-014-0054-3, 2015.
Briones, M. J. I., McNamara, N. P., Poskitt, J., Crow, S. E., and Ostle, N. J.: Interactive biotic and abiotic regulators of soil carbon cycling: evidence from controlled climate experiments on peatland and boreal soils, Glob. Change Biol., 20, 2971–2982, https://doi.org/10.1111/gcb.12585, 2014.
Buckeridge, K. M., Banerjee, S., Siciliano, S. D., and Grogan, P.: The seasonal pattern of soil microbial community structure in mesic low arctic tundra, Soil Biol. Biochem., 65, 338–347, https://doi.org/10.1016/j.soilbio.2013.06.012, 2013.
Butnor, J. R., Johnsen, K. H., Oren, R., and Katul, G. G: Reduction of forest floor respiration by fertilization on both carbon dioxide‐enriched and reference 17‐year‐old loblolly pine stands, Glob. Change Biol., 9, 849–861, https://doi.org/10.1046/j.1365-2486.2003.00630.x, 2003.
Carey, J. C., Tang, J., Templer, P. H., Kroeger, K. D., Crowther, T. W., Burton, A. J., Dukes, J. S., Emmett, B., Frey, S. D., Heskel, M. A., Jiang, L., Machmuller, M. B., Mohan, J., Panetta, A. M., Reich, P. B., Reinsch, S., Wang, X., Allison, S. D., Bamminger, C., Bridgham, S., Collins, S. L., de Dato, G., Eddy, W. C., Enquist, B. J., Estiarte, M., Harte, J., Henderson, A., Johnson, B. R., Larsen, K. S., Luo, Y., Marhan, S., Melillo, J. M., Peuelas, J., Pfeifer-Meister, L., Poll, C., Rastetter, E., Reinmann, A. B., Reynolds, L. L., Schmidt, I. K., Shaver, G. R., Strong, A. L., Suseela, V., and Tietema, A.: Temperature response of soil respiration largely unaltered with experimental warming, P. Natl. Acad. Sci. USA, 113, 13797–13802, 2016.
Cheng, W., Parton, W. J., Gonzalez-Meler, M. A., Phillips, R., Asao, S., McNickle, G. G., Brzostek, E., and Jastrow, J. D.: Synthesis and modeling perspectives of rhizosphere priming, New Phytol., 201, 31–44, https://doi.org/10.1111/nph.12440, 2014.
Conant, R. T., Steinweg, J. M., Haddix, M. L., Paul, E. A., Plante, A. F., and Six, J.: Experimental warming shows that decomposition temperature sensitivity increases with soil organic matter recalcitrance, Ecology, 89, 2384–2391, https://doi.org/10.1890/08-0137.1, 2008.
Curiel Yuste, J., Janssens, I. A., Carrara, A., and Ceulemans, R.: Annual Q10 of soil respiration reflects plant phenological patterns as well as temperature sensitivity, Glob. Change Biol., 10, 161–169, https://doi.org/10.1111/j.1529-8817.2003.00727.x, 2004.
Czimczik, C. I. and Trumbore, S. E.: Short-term controls on the age of microbial carbon sources in boreal forest soils, J. Geophys. Res.-Biogeo., 112, JG000389, https://doi.org/10.1029/2006JG000389, 2007.
Dauwe, B. and Middelburg, J. J.: Amino acids and hexosamines as indicators of organic matter degradation state in North Sea sediments, Limnol. Oceangr., 43, 782–798, https://doi.org/10.4319/lo.1998.43.5.0782, 1998.
Davidson, E. A. and Janssens, I. A.: Temperature sensitivity of soil carbon decomposition and feedbacks to climate change, Nature, 440, 165–173, https://doi.org/10.1038/nature04514, 2006.
Di Lonardo, D. P., de Boer, W., Zweers, H., and van der Wal, A.: Effect of the amount of organic trigger compounds, nitrogen and soil microbial biomass on the magnitude of priming of soil organic matter, edited by: Wu, F., PLoS ONE, 14, e0216730, https://doi.org/10.1371/journal.pone.0216730, 2019.
Dijkstra, F. A., Carrillo, Y., Pendall, E., and Morgan, J. A.: Rhizosphere priming: a nutrient perspective, Front. Microbiol., 4, 00216, https://doi.org/10.3389/fmicb.2013.00216, 2013.
Dijkstra, P., Ishizu, A., Doucett, R., Hart, S. C., Schwartz, E., Menyailo, O. V., and Hungate, B. A.: 13C and 15N natural abundance of the soil microbial biomass, Soil Biol. Biochem., 38, 3257–3266, https://doi.org/10.1016/j.soilbio.2006.04.005, 2006.
Fang, C. M., Smith, P., Moncrieff, J. B., and Smith, J. U.: Similar response of labile and resistant soil organic matter pools to changes in temperature, Nature, 436, 881–881, https://doi.org/10.1038/nature04044, 2005.
Finzi, A. C., Abramoff, R. Z., Spiller, K. S., Brzostek, E. R., Darby, B. A., Kramer, M. A., and Phillips, R. P.: Rhizosphere processes are quantitatively important components of terrestrial carbon and nutrient cycles, Glob. Change Biol., 21, 2082–2094, https://doi.org/10.1111/gcb.12816, 2015.
Fontaine, S., Barot, S., Barré, P., Bdioui, N., Mary, B., and Rumpel, C.: Stability of organic carbon in deep soil layers controlled by fresh carbon supply, Nature, 450, 277–280, https://doi.org/10.1038/nature06275, 2007.
Fontaine, S., Henault, C., Aamor, A., Bdioui, N., Bloor, J. M. G., Maire, V., Mary, B., Revaillot, S., and Maron, P. A.: Fungi mediate long term sequestration of carbon and nitrogen in soil through their priming effect, Soil Biol. Biochem., 43, 86–96, https://doi.org/10.1016/j.soilbio.2010.09.017, 2011.
Frey, S. D., Lee, J., Melillo, J. M., and Six, J.: The temperature response of soil microbial efficiency and its feedback to climate, Nat. Clim. Change, 3, 1–4, https://doi.org/10.1038/nclimate1796, 2013.
Glaser, B. and Amelung, W.: Determination of 13C natural abundance of amino acid enantiomers in soil: methodological considerations and first results, Rapid Commun. Mass Spectrom., 16, 891–898, https://doi.org/10.1002/rcm.650, 2002.
Hájek, T., Ballance, S., Limpens, J., Zijlstra, M., and Verhoeven, J. T. A.: Cell-wall polysaccharides play an important role in decay resistance of Sphagnum and actively depressed decomposition in vitro, Biogeochemistry, 103, 45–57, https://doi.org/10.1007/s10533-010-9444-3, 2011.
Hedges, J. I., Cowie, G. L., Richey, J. E., Quay, P. D., Benner, R., Strom, M., and Forsberg, B. R.: Origins and processing of organic matter in the Amazon River as indicated by carbohydrates and amino acids, Limnol. Oceangr., 39, 743–761, https://doi.org/10.4319/lo.1994.39.4.0743, 1994.
Hicks Pries, C. E., Castanha, C., Porras, R. C., and Torn, M. S.: The whole-soil carbon flux in response to warming, Science, 355, 1420–1423, https://doi.org/10.1126/science.aal1319, 2017.
Hobbie, E. and Werner, R.: Intramolecular, compound-specific, and bulk carbon isotope patterns in C3 and C4 plants: a review and synthesis, New Phytol., 161, 371–385, doi.org/10.1046/j.1469-8137.2004.00970.x, 2004.
Hogberg, M. N. and Hogberg, P.: Extramatrical ectomycorrhizal mycelium contributes one-third of microbial biomass and produces, together with associated roots, half the dissolved organic carbon in a forest soil, New Phytol., 154, 791–795, 2002.
Kaiser, K. and Kalbitz, K.: Cycling downwards – dissolved organic matter in soils, Soil Biol. Biochem., 52, 29–32, https://doi.org/10.1016/j.soilbio.2012.04.002, 2012.
Kalbitz, K. and Kaiser, K.: Contribution of dissolved organic matter to carbon storage in forest mineral soils, J. Plant Nutr. Soil Sci., 171, 52–60, https://doi.org/10.1002/jpln.200700043, 2008.
Kallenbach, C. M., Frey, S. D., and Grandy, A. S.: Direct evidence for microbial-derived soil organic matter formation and its ecophysiological controls, Nat. Commun., 7, 13630, https://doi.org/10.1038/ncomms13630, 2016.
Kane, E. S., Hockaday, W. C., Turetsky, M. R., Masiello, C. A., Valentine, D. W., Finney, B. P., and Baldock, J. A.: Topographic controls on black carbon accumulation in Alaskan black spruce forest soils: implications for organic matter dynamics, Biogeochemistry, 100, 39–56, https://doi.org/10.1007/s10533-009-9403-z, 2010.
Karhu, K., Auffret, M. D., Dungait, J. A. J., Hopkins, D. W., Prosser, J. I., Singh, B. K., Subke, J.-A., Wookey, P. A., Ågren, G. I., Sebastià, M.-T., Gouriveau, F., Bergkvist, G., Meir, P., Nottingham, A. T., Salinas, N., and Hartley, I. P.: Temperature sensitivity of soil respiration rates enhanced by microbial community response, Nature, 513, 81–84, https://doi.org/10.1038/nature13604, 2014.
Kohl, L., Laganière, J., Edwards, K. A., Billings, S. A., Morrill, P. L., Van Biesen, G., and Ziegler, S. E.: Distinct fungal and bacterial δ13C signatures as potential drivers of increasing δ13C of soil organic matter with depth, Biogeochemistry, 124, 13–26, https://doi.org/10.1007/s10533-015-0107-2, 2015.
Kohl, L., Philben, M., Edwards, K. A., Podrebarac, F. A., Warren, J., and Ziegler, S. E.: The origin of soil organic matter controls its composition and bioreactivity across a mesic boreal forest latitudinal gradient, Glob. Change Biol., 24, e458–e473, https://doi.org/10.1111/gcb.13887, 2018.
Laganière, J., Podrebarac, F., Billings, S. A., Edwards, K. A., and Ziegler, S. E.: A warmer climate reduces the bioreactivity of isolated boreal forest soil horizons without increasing the temperature sensitivity of respiratory CO2 loss, Soil Biol. Biochem., 84, 177–188, https://doi.org/10.1016/j.soilbio.2015.02.025, 2015.
Lefevre, R., Barré, P., Moyano, F. E., Christensen, B. T., Bardoux, G., Eglin, T., Girardin, C., Houot, S., Kätterer, T., van Oort, F., and Chenu, C.: Higher temperature sensitivity for stable than for labile soil organic carbon – Evidence from incubations of long-term bare fallow soils, Glob. Change Biol., 20, 633–640, https://doi.org/10.1111/gcb.12402, 2014.
Leifeld, J. and Fuhrer, J.: The Temperature Response of CO2 Production from Bulk Soils and Soil Fractions is Related to Soil Organic Matter Quality, Biogeochemistry, 75, 433–453, https://doi.org/10.1007/s10533-005-2237-4, 2005.
Li, Q., Tian, Y., Zhang, X., Xu, X., Wang, H., and Kuzyakov, Y.: Labile carbon and nitrogen additions affect soil organic matter decomposition more strongly than temperature, Appl. Soil Ecol., 114, 152–160, https://doi.org/10.1016/j.apsoil.2017.01.009, 2017.
Liski, J., Ilvesniemi, H., Mäkelä, A., and Westman, C. J.: CO2 emissions from soil in response to climatic warming are overestimated – The decomposition of old soil organic matter is tolerant of temperature, AMBIO, 28, 171–174, 1999.
Maier, C. A. and Kress, L. W.: Soil CO2 evolution and root respiration in 11 year-old loblolly pine (Pinus taeda) plantations as affected by moisture and nutrient availability, Can. J. Forest Res., 30, 347–359, https://doi.org/10.1139/x99-218, 2000
Malik, A. A., Chowdhury, S., Schlager, V., Oliver, A., Puissant, J., Vazquez, P. G. M., Jehmlich, N., Bergen, von, M., Griffiths, R. I., and Gleixner, G.: Soil Fungal : Bacterial Ratios Are Linked to Altered Carbon Cycling, Front. Microbiol., 7, 1247, https://doi.org/10.3389/fmicb.2016.01247, 2016.
Massiot, D., Fayon, F., Capron, M., King, I., Le Calve, S., Alonso, B., Durand, J. O., Bujoli, B., Gan, Z. H., and Hoatson, G.: Modelling one- and two-dimensional solid-state NMR spectra, Magn. Reson. Chem., 40, 70–76, https://doi.org/10.1002/mrc.984, 2002.
Min, K., Buckeridge, K., Ziegler, S. E., Edwards, K. A., Bagchi, S., and Billings, S. A.: Temperature sensitivity of biomass-specific microbial exo-enzyme activities and CO2 efflux is resistant to change across short- and long-term timescales, Glob. Change Biol., 25, 1793–1807, https://doi.org/10.1111/gcb.14605, 2019.
Mooshammer, M., Wanek, W., Hämmerle, I., Fuchslueger, L., Hofhansl, F., Knoltsch, A., Schnecker, J., Takriti, M., Watzka, M., Wild, B., Keiblinger, K. M., Zechmeister-Boltenstern, S., and Richter, A.: Adjustment of microbial nitrogen use efficiency to carbon: Nitrogen imbalances regulates soil nitrogen cycling, Nat. Commun., 5, 1–7, https://doi.org/10.1038/ncomms4694, 2014.
Paterson, E., Osler, G., Dawson, L. A., Gebbing, T., Sim, A., and Ord, B.: Labile and recalcitrant plant fractions are utilised by distinct microbial communities in soil: Independent of the presence of roots and mycorrhizal fungi, Soil Biol. Biochem., 40, 1103–1113, https://doi.org/10.1016/j.soilbio.2007.12.003, 2008.
Pawar, S., Dell, A. I., Savage, V. M., and Knies, J. L.: Real versus Artificial Variation in the Thermal Sensitivity of Biological Traits, Am. Nat., 187, E41–E52, https://doi.org/10.1086/684590, 2016.
Pennington, S. C., McDowell, N. G., Megonigal, J. P., Stegen, J. C., and Bond-Lamberty, B.: Localized basal area affects soil respiration temperature sensitivity in a coastal deciduous forest, Biogeosciences, 17, 771–780, https://doi.org/10.5194/bg-17-771-2020, 2020.
Philben, M., Ziegler, S. E., Edwards, K. A., Kahler III, R., and Benner, R.: Soil organic nitrogen cycling increases with temperature and precipitation along a boreal forest latitudinal transect, Biogeochemistry, 127, 397–410, https://doi.org/10.1007/s10533-016-0187-7, 2016.
Philben, M., Butler, S., Billings, S. A., Benner, R., Edwards, K. A., and Ziegler, S. E.: Biochemical and structural controls on the decomposition dynamics of boreal upland forest moss tissues, Biogeosciences, 15, 6731–6746, https://doi.org/10.5194/bg-15-6731-2018, 2018.
Pietikainen, J., Pettersson, M., and Baath, E.: Comparison of temperature effects on soil respiration and bacterial and fungal growth rates, FEMS Microbiol. Ecol., 52, 49–58, https://doi.org/10.1016/j.femsec.2004.10.002, 2005.
Podrebarac, F. A., Laganière, J., Billings, S. A., Edwards, K. A., and Ziegler, S. E.: Soils isolated during incubation underestimate temperature sensitivity of respiration and its response to climate history, Soil Biol. Biochem., 93, 60–68, https://doi.org/10.1016/j.soilbio.2015.10.012, 2016.
Popper, Z. A. and Fry, S. C.: Primary cell wall composition of bryophytes and charophytes, Ann. Bot., 91, 1–12, https://doi.org/10.1093/aob/mcg013, 2003.
Preston, C. M., Trofymow, J. T., and Working Group, T. C. I. D.: Variability in litter quality and its relationship to litter decay in Canadian forests, Can. J. Bot., 78, 1269–1287, https://doi.org/10.1139/b00-101, 2000.
Preston, C. M., Nault, J. R., and Trofymow, J. A.: Chemical Changes During 6 Years of Decomposition of 11 Litters in Some Canadian Forest Sites, Part 2. 13C Abundance, Solid-State 13C NMR Spectroscopy and the Meaning of “Lignin”, Ecosystems, 12, 1078–1102, https://doi.org/10.1007/s10021-009-9267-z, 2009.
Pumpanen, J. S., Heinonsalo, J., Rasilo, T., Hurme, K.-R., and Ilvesniemi, H.: Carbon balance and allocation of assimilated CO2 in Scots pine, Norway spruce, and Silver birch seedlings determined with gas exchange measurements and 14C pulse labelling, Trees-Struct. Funct., 23, 611–621, https://doi.org/10.1007/s00468-008-0306-8, 2009.
R Core Team: A Language and Environment for Statistical Computing, https://www.R-project.org/ (last access: 17 June 2018), 2017.
Rinnan, R. and Baath, E.: Differential Utilization of Carbon Substrates by Bacteria and Fungi in Tundra Soil, Appl. Environ. Microb., 75, 3611–3620, https://doi.org/10.1128/AEM.02865-08, 2009.
Robertson, G. P., Coleman, D. C., Sollins, P., and Bledsoe, C. S (Eds.): Standard soil methods for long-term ecological research, Oxford Press, London, 1999.
Rytioja, J., Hilden, K., Yuzon, J., Hatakka, A., de Vries, R. P., and Makela, M. R.: Plant-polysaccharide-degrading enzymes from basidiomycetes, Microbiol. Mol. Biol. Rev., 78, 614–649, https://doi.org/10.1128/MMBR.00035-14, 2014.
Schadt, C. W., Martin, A. P., Lipson, D. A., and Schmidt, S. K.: Seasonal dynamics of previously unknown fungal lineages in tundra soils, Science, 301, 1359–1361, https://doi.org/10.1126/science.1086940, 2003.
Schimel, J. P. and Weintraub, M. N.: The implications of exoenzyme activity on microbial carbon and nitrogen limitation in soil: a theoretical model, Soil Biol. Biochem., 35, 549–563, https://doi.org/10.1016/S0038-0717(03)00015-4, 2003.
Schipper, L. A., Hobbs, J. K., Rutledge, S., and Arcus, V. L.: Thermodynamic theory explains the temperature optima of soil microbial processes and high Q10 values at low temperatures, Glob. Change Biol., 20, 3578–3586, https://doi.org/10.1111/gcb.12596, 2014.
Sierra, C. A.: Temperature sensitivity of organic matter decomposition in the Arrhenius equation: some theoretical considerations, Biogeochemistry, 108, 1–15, https://doi.org/10.1007/s10533-011-9596-9, 2012.
Stocker, T. F., Qin, D., Plattner, G. K., Tignor, M. M. B., Allen, S. K., Boschung, J., Nauels, A., Xia, Y., Bex, V., and Midgley, P. M.: Climate change 2013 the physical science basis: Working Group I contribution to the fifth assessment report of the intergovernmental panel on climate change, edited by: Intergovernmental Panel on Climate Change, Cambridge University Press, Cambridge, 2013.
Streit, K., Hagedorn, F., Hiltbrunner, D., Portmann, M., Saurer, M., Buchmann, N., Wild, B., Richter, A., Wipf, S., and Siegwolf, R. T. W.: Soil warming alters microbial substrate use in alpine soils, Glob. Change Biol., 20, 1327–1338, https://doi.org/10.1111/gcb.12396, 2014.
Turetsky, M. R., Crow, S. E., Evans, R. J., Vitt, D. H., and Wieder, R. K.: Trade-offs in resource allocation among moss species control decomposition in boreal peatlands, J. Ecol., 96, 1297–1305, https://doi.org/10.1111/j.1365-2745.2008.01438.x, 2008.
Wallenstein, M. D. and Weintraub, M. N.: Emerging tools for measuring and modeling the in situ activity of soil extracellular enzymes, Soil Biol. Biochem., 40, 2098–2106, https://doi.org/10.1016/j.soilbio.2008.01.024, 2008.
Wang, Q., He, N., Yu, G., Gao, Y., Wen, X., Wang, R., Koerner, S. E., and Yu, Q.: Soil microbial respiration rate and temperature sensitivity along a north-south forest transect in eastern China: Patterns and influencing factors, J. Geophys. Res.-Biogeo., 121, 399–410, https://doi.org/10.1002/2015JG003217, 2016.
Wild, B., Gentsch, N., Čapek, P., Diáková, K., Alves, R. J. E., Bárta, J., Gittel, A., Hugelius, G., Knoltsch, A., Kuhry, P., Lashchinskiy, N., Mikutta, R., Palmtag, J., Schleper, C., Schnecker, J., Shibistova, O., Takriti, M., Torsvik, V. L., Urich, T., Watzka, M., Šantrůčková, H., Guggenberger, G., and Richter, A.: Plant-derived compounds stimulate the decomposition of organic matter in arctic permafrost soils, Sci. Rep., 6, 25607, https://doi.org/10.1038/srep25607, 2016.
Wilson, M. A., Vassallo, A. M., Perdue, E. M.. and Reuter, J. H.: Compositional and Solid-State Nuclear Magnetic Resonance Study of Humic and Fulvic Acid Fractions of Soil Organic Matter, Anal. Chem., 59, 551–558, https://doi.org/10.1021/ac00131a004, 1987.
Zhu, B. and Cheng, W.: Rhizosphere priming effect increases the temperature sensitivity of soil organic matter decomposition, Glob. Change Biol., 17, 2172–2183, https://doi.org/10.1111/j.1365-2486.2010.02354.x, 2011.
Zar, J. H.: Biostatistical Analysis, Prentice Hall, New York, USA, 1999.
Ziegler, S. E. and Billings, S. A.: Soil nitrogen status as a regulator of carbon substrate flows through microbial communities with elevated CO2, J. Geophys. Res., 116, G01011, https://doi.org/10.1029/2010JG001434, 2011.
Ziegler, S. E., Billings, S. A., Lane, C. S., Li, J., and Fogel, M. L.: Warming alters routing of labile and slower-turnover carbon through distinct microbial groups in boreal forest organic soils, Soil Biol. Biochem., 60, 23–32, https://doi.org/10.1016/j.soilbio.2013.01.001, 2013.
Ziegler, S. E., Benner, R., Billings, S. A., Edwards, K. A., Philben, M., Zhu, X., and Laganière, J.: Climate Warming Can Accelerate Carbon Fluxes without Changing Soil Carbon Stocks, Front. Earth Sci., 5, 2, https://doi.org/10.3389/feart.2017.00002, 2017.
Zogg, G. P., Zak, D. R., Ringelberg, D. B., White, D. C., MacDonald, N. W., and Pregitzer, K. S.: Compositional and Functional Shifts in Microbial Communities Due to Soil Warming, Soil Sci. Soc. Am. J., 61, 475–481, https://doi.org/10.2136/sssaj1997.03615995006100020015x, 1997.