the Creative Commons Attribution 4.0 License.
the Creative Commons Attribution 4.0 License.
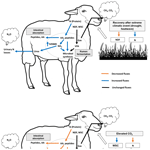
Effects of elevated CO2 and extreme climatic events on forage quality and in vitro rumen fermentation in permanent grassland
Vincent Niderkorn
Annette Morvan-Bertrand
Aline Le Morvan
Angela Augusti
Marie-Laure Decau
Catherine Picon-Cochard
The aim of this study was to analyze changes in botanical and chemical composition, as well as in vitro rumen fermentation characteristics of an upland grassland exposed to climate changes in controlled CO2 concentration, air temperature and precipitation conditions. Grassland was exposed to a future climate scenario coupled with CO2 treatments (390 and 520 ppm) from the beginning of spring. During summer, an extreme climatic event (ECE; 2 weeks of a +6 ∘C increase in temperature, together with severe drought) was applied and then followed by a recovery period. Three cutting dates were considered, i.e. in April, June and November. The results indicate that increases in greenness, nitrogen (N) content and changes in water-soluble carbohydrate profile in association with botanical composition changes for the November cut lead to higher in vitro dry matter degradability (IVDMD) in the rumen. The neutral detergent fiber : nitrogen (NDF:N) ratio appeared to be a key driver of forage quality, which was affected in opposite ways by elevated CO2 and ECE, with a strong impact on rumen fermentation. Atmospheric CO2 concentration in interaction with ECE tended to affect IVDMD, indicating that the effects of elevated CO2 and ECE may partly offset each other. Our findings indicate that the various factors of climate change need to be considered together in order to properly characterize their effects on forage quality and use by ruminants.
- Article
(1874 KB) - Full-text XML
-
Supplement
(368 KB) - BibTeX
- EndNote
Global livestock production has increased rapidly and substantially in recent decades as a result of world population growth and a shift towards diets with a higher animal protein content in many countries (Tilman and Clark, 2014). The livestock sector is singled out as a significant contributor to global human-induced greenhouse gas (GHG) emissions (Gerber et al., 2013), particularly through energy and protein losses in the form of enteric methane (CH4) and ammonia (NH3), urea, and nitrous oxide (N2O) released during ruminant digestion. Using 100-year-timescale global warming potentials of 34 for CH4 and 298 for N2O (compared to CO2) (IPCC, 2013), the livestock supply chains emit an estimated total of 7.1 Gt CO2 eq. yr−1, with ruminants by far the largest contributors (Gerber et al., 2013). Recent studies show that atmospheric CH4 levels have grown alarmingly rapidly in recent years (Nisbet et al., 2019) and that livestock CH4 emissions may even have been underestimated (Wolf et al., 2017). Reducing GHG emissions is a crucial challenge for Earth system governance, and there is significant potential for mitigation in the ruminant sector (Herrero et al., 2016).
The bulk of the ruminant diet consists of plant material in the form of forage. Globally, grasses comprised 48 % (2.3 billion tons) of the total biomass used by livestock (4.7 billion tons) in 2000 (Herrero et al., 2013). Forage quality is a major actionable lever for decreasing GHG emissions as variability in forage nutritive value has been shown to generate variability in the CH4 emission levels from ruminants (Thornton and Herrero, 2010). Forage quality is closely linked to ingestibility and digestibility, both of which are largely dependent on the nature and concentrations of the major forage macronutrients, such as structural and non-structural carbohydrates or crude proteins (Fig. 1). Digestibility is the main driver of net dietary energy, rumen microbial synthesis and production of volatile fatty acids (VFAs), which are the main sources of energy for the ruminant (INRA, 2018). On the other hand, digestible organic matter (OM) is the best indicator of CH4 emissions as it is produced by carbohydrate fermentation (Fig. 1; INRA, 2018). In the specific case of permanent grasslands, forage quality is mainly driven by botanical composition, i.e. a large diversity of self-seeded grasses and dicotyledons and broad variability in phenology and competitiveness, and is affected by agricultural practices (Rossignol et al., 2014; Andueza et al., 2016).
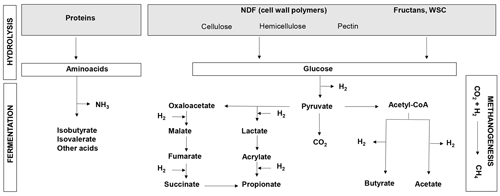
Figure 1Overview of protein and carbohydrate metabolism in the rumen. WSCs: water-soluble carbohydrates; NDF: neutral detergent fiber (cell wall carbohydrates); CH4: methane; CO2: carbon dioxide; NH3: ammonia; H2: hydrogen.
Forage quality can also be impacted by elevated temperatures and by the intensity and frequency of extreme climatic events (ECEs) such as droughts and heat waves, which are projected to increase (Planton et al., 2008). Lee et al. (2017) modeled the variation in nutritive value of forage species growing across a range of bioclimatic zones and showed that higher temperatures reduce forage nutritive value likely due to changes in species identity, physiology and phenology. They also found that CH4 production may increase by 0.9 % with a 1 ∘C temperature rise and by 4.5 % with a 5 ∘C rise. The effects of interactions between ECEs and elevated CO2 on grassland ecology and forage quality are not well understood, especially for permanent grasslands (Dumont et al., 2015). There is a need to address the gap in knowledge on the drivers of forage quality under projected climatic conditions in order to adapt grass-based ruminant systems to the context of global climate change.
The aim of this study was to analyze changes in botanical and chemical composition of plants from an upland grassland when exposed to an elevated atmospheric CO2 level combined or not with an ECE (drought combined with heat wave) in controlled conditions. The impact of these changes on ruminant digestion was investigated by determining in vitro rumen fermentation parameters. The hypothesis tested was that combined drivers of global change (elevated CO2 and ECEs) may have different effects on forage characteristics and digestion by ruminants, with potential offsets.
2.1 Experimental design
The experimental design was previously described in Roy et al. (2016) and Volaire et al. (2020). The present study tested the forage quality response of an upland-grassland plant community near Saint-Genès-Champanelle (central France) to future climate scenarios projected for the 2050s (Ciais et al., 2005). For the representative year 2045 given by the ARPEGEv4 atmosphere–ocean general circulation model under the A2-CO2 emissions scenario (Nakicenovic et al., 2000) and using a multivariate statistical downscaling methodology (Boé et al., 2006) to generate projections over an 8×8 km grid, the projected annual means for air temperature and precipitation at Saint-Genès-Champanelle were 10.9 ∘C and 770 mm, respectively. These values correspond to +2.3 ∘C and −33 mm compared to the mean air temperature and precipitation measured over the 1990–2009 period. Here, we chose to apply less precipitation and higher temperatures than under the prevailing current climate from the start of the experiment and for all treatments (all monoliths were exposed to future climate scenario) in order to test and compare the effects of elevated CO2 and increased ECEs under these drier and warmer conditions. The baseline climate conditions of this experiment were therefore drier and warmer than at the origin site (Saint-Genès-Champanelle). Furthermore, we tested and compared the atmospheric CO2 concentration forecasted for the 2050s (according to the A2 scenario), i.e. 520 ppm, against the CO2 concentration of 390 ppm measured in 2010.
In June 2009, 1 m2 monoliths (n=48) formed by undisturbed soil and vegetation from an extensively managed upland semi-natural grassland were excavated to 60 cm depth. The origin site (Redon; 45∘43′ N, 03∘01′ E; 800 ) is located near Saint-Genès-Champanelle and is a long-term fertile (clover rich) grassland managed by a combination of grazing (three to five grazing periods) and cutting (one cut per year). One organic fertilization occurs at the end of the winter period. The average botanical composition of the plant communities was initially dominated by C3 perennial grasses (60 %), legumes (35 %) and forbs (5 %). At the start of the experiment, five species accounted for 70 % of species composition: Trifolium repens, Lolium perenne, Holcus lanatus, Agrostis tenuis and Alopecurus pratensis. The origin-site soil is a cambisol of 59.5 % sand, 19.2 % silt and 21.3 % clay, with a pH of 5.9. Once excavated, the 48 monoliths were transferred to the INRAE research station (Clermont Ferrand; 45∘46′ N, 03∘08′ E; 350 ) where the soil water content (SWC) was maintained at near 80 % of original field capacity between natural precipitation and additional irrigation.
At the beginning of 2010, the monoliths were transported to the CNRS Ecotron near Montpellier (43∘40′ N, 03∘52′ E). Four monoliths were randomly allocated to each of the 12 Ecotron macrocosms. The macrocosms were exposed from April 2010 to early March 2011 to the future climate scenario forecasted for 2050, according to the ARPEGEv4 model, and to current CO2 concentration. From mid-March 2011 to November 2012, six randomly selected macrocosms were exposed to 520 ppm CO2 and the other six to 390 ppm CO2.
For each CO2 concentration treatment, a first phase of the ECE treatment (reduction of 50 % precipitation) was applied on three out of six monoliths from 25 June to 21 July. A second phase of the ECE treatment (no irrigation and a 3.4 ∘C increase in air temperature per the year 2050 scenario) was applied from 22 July to 4 August. This temperature increase corresponded to a 7.1 ∘C higher temperature than the mean temperature from 2000 to 2009 at the same period and was above the 14 consecutive hottest days of summer 2003. From 5 to 31 August, irrigation was progressively applied in the treatment with the ECE to allow the same cumulative precipitation as in the non-ECE-treated monoliths. From the end of August until the beginning of November, all macrocosms were exposed to the 2050 climate conditions. From April 2010 to November 2011, the Ecotron climate-regulation system monitored hourly means of air temperature and humidity, as well as daily means of precipitation and CO2 concentration. Each of the four experimental treatments combining both CO2 and ECE treatments was replicated three times. Further details on the experimental conditions can be found in Roy et al. (2016). In each of the 48 monoliths, SWC was continuously measured at soil depths of 7, 20 and 50 cm using time domain reflectometry (TDR) probes (IMKO, Ettlingen, Germany) and averaged across soil depths and monoliths in order to get one value per macrocosm. In addition, to match with data on forage quality, SWC was averaged across regrowth periods before the cuts, i.e. from 1 to 26 April, from 27 April to 9 June and from 22 September to 3 November.
2.2 Plant materials, cutting and botanical composition
In 2011, on three dates (26 April, 9 June, 3 November), aboveground biomass was harvested on a fixed center-square (0.5×0.5 m) in each monolith using a precision mower (6 cm cutting height). The April cut was the expression of winter and spring growth, and no treatments were applied except for 1 month of CO2 treatment, whereas the June cut was the expression of spring and early summer growth and CO2 treatment, and the November cut was the expression of summer and fall growth and included the CO2 treatment, the ECE and the recovery phase.
The cut material was weighed to determine fresh aboveground biomass and then separated into three subsamples: the first subsample was oven-dried at 60 ∘C for 72 h and used for dry matter (DM) determination and chemical analyses; the second subsample was freeze-dried and used for the in vitro rumen fermentation assay; the third subsample was used to sort out green, dead and flower biomass and determine the botanical composition of the green material. Species were sorted by hand, oven-dried (60 ∘C, 72 h) and weighed separately in order to calculate relative abundance for each species and then define the functional groups, i.e. grasses, legumes and forbs (shown as relative abundance).
2.3 In vitro rumen fermentation assay
All experimental procedures were performed according to the European Union Directive 2010/63/EU, reviewed by the local institutional review board (C2E2A, “Comité d'Ethique pour l'Expérimentation Animale en Auvergne”) and approved under French Ministry for Research authorization no. CE 69-12.
For each macrocosm and each cutting date, a representative sample was reconstituted with cut freeze-dried material from the four monoliths, weighted according to the values of aboveground biomass measured on each monolith (thereby pooling the four monoliths, n=12). The rumen fermentation assay was performed on the 12 samples taken on each cutting date, three times over a period of 2 weeks.
Freeze-dried plant material (600±0.5 mg) was transferred in 120 mL serum bottles, pre-warmed at 39±0.5 ∘C and flushed with N2 to eliminate the oxygen. A total of 40 mL buffered rumen fluid was then added to the serum bottle, and the bottle was hermetically sealed with a butyl rubber stopper and aluminum crimp seals. The buffered rumen fluid was prepared as follows. Rumen contents were collected before the morning feeding from three cannulated sheep fed daily with 1200 g of a diet composed of 80 % permanent grassland hay and 20 % concentrate mix. Rumen contents from the three sheep were mixed in the same proportions in a container and squeezed through two layers of cheesecloth (800 µm mesh size) to obtain the fluid used as inoculum for the in vitro rumen fermentation assay. Strained rumen fluid was diluted in an anaerobic buffer solution (phosphate : carbonate, 1:2 ) as described by Goering and Van Soest (1970) and modified by Niderkorn et al. (2011). Initial pH of the buffered rumen fluid was All bottles were incubated in a shaking water bath at 39±0.5 ∘C, and blanks without any plant substrate (only buffered rumen fluid) were included. At t=0, samples of buffered rumen fluid were taken to determine the VFAs and NH3 concentrations in the medium before incubation.
After 24 h of incubation, the volume of gas produced in the headspace of the serum bottles was determined using a pressure transducer (Theodorou et al., 1994), and gas samples were taken for the determination of CH4 and CO2 concentrations. The entire contents of the bottle were then transferred into a pre-weighed 50 mL Falcon tube, and the pH was immediately measured. Tubes were centrifuged at 3400×g for 10 min at 4 ∘C, and samples of supernatant were taken for determination of VFAs and NH3 concentrations (Niderkorn et al., 2011). To recover all the non-degraded particles, the bottle was washed twice with distilled water, and the washing water was transferred into the Falcon tube. Tubes were again centrifuged at 3400×g for 10 min at 4 ∘C, and after removal of the supernatant, the residue was used for DM determination.
2.4 Analytical procedures
Plant substrates and leftovers after fermentation (residues) were analyzed to determine DM by oven-drying at 60 ∘C for 72 h and OM by ashing at 550 ∘C for 6 h in a muffle furnace. In-plant neutral detergent fiber (NDF) content was determined according to the method described by Van Soest et al. (1991) using an ANKOM fiber analyzer (ANKOM Technology Corporation, Fairport, NY). The leaf carbon (C) and nitrogen (N) contents were determined at the INRAE-Nancy isotopic analysis platform using a stable isotope-ratio mass spectrometer (IsoPrime 100, IsoPrime, Manchester, UK). Water-soluble carbohydrates (WSCs) were successively extracted from dry powder with 80 % ethanol and water, according to Benot et al. (2019). Supernatants were pooled and evaporated under vacuum to eliminate ethanol and water and to thus concentrate the samples. The residue was dissolved in water and passed through ion exchange resins to remove charged compounds before high-performance liquid chromatography (HPLC) analysis. WSCs were separated on a cation exchange column (Sugar-PAK I, 300×6.5 mm, Millipore Waters Milford, MA) and detected using a refractometer (see Benot et al., 2019, for more details). Condensed tannin (CT) content was determined using the colorimetric HCl–butanol method (Grabber et al., 2013). Pepsin-cellulase OM digestibility was evaluated according to the method described by Aufrère and Michalet-Doreau (1988). In vitro DM degradability (IVDMD) was determined as the difference between the DM of plant material before the fermentation and the DM of fermentation residue after 24 h of fermentation. The CH4 and CO2 concentrations in gas samples were determined by gas chromatography using a 3000A Micro GC system (Agilent Technologies, France). Total and individual VFAs (acetate, propionate, butyrate, valerate, caproate, isobutyrate, isovalerate) in the supernatant were measured by gas chromatography, and NH3 was determined using the Berthelot reaction (Park et al., 2009).
2.5 Statistical analysis
All variables related to the chemical composition of plant communities and in vitro rumen fermentation parameters were analyzed using a mixed model (MIXED procedure, SAS Enterprise Guide 5.1, SAS Institute Inc., Cary, NC). Each macrocosm was considered as an experimental unit. For the April and June cuts, CO2 concentration was used as a fixed effect, and the effects of ECE treatment and CO2–ECE interaction were added as fixed effects for the November cut, with the macrocosm used as a random factor. For each variable analyzed, data were subjected to three covariance structures: compound symmetry, autoregressive order 1 and unstructured covariance. The covariance that resulted in the smallest Akaike's information criterion was retained for analysis. Fractions (relative abundances) were transformed by the arcsine of the square root before the analysis of variance. Significance was set at p≤0.05, and trend was set at . Relationships between aboveground biomass characteristics, chemical composition and in vitro rumen fermentation parameters were analyzed with non-parametric Spearman correlation tests.
The measured values of temperature and atmospheric CO2 concentration throughout the experiment for the different treatments were reported in Roy et al. (2016). When the ECE was imposed, mean daily air temperature peaked at 25 ∘C. As expected, the ECE strongly affected SWC during the stress period (Table 1, p<0.001). There was a significant effect of CO2–ECE interaction during the period preceding the November cut (Table 1, p=0.014), reflecting a higher SWC for the control at 520 ppm CO2 compared to the other treatments.
Table 1Factor effects (p values) on soil volumetric water content (SWC) at two levels of CO2 concentration (390 and 520 ppm) with or without an extreme climatic event (ECE).

The d.f (numden) signifies degrees of freedom (numerator and denominator). In bold: p<0.05; in italics: .
Table 2Factor effects (p values) on aboveground mass, fractions of green material, flower and dead material, relative abundance of functional groups, and relative abundance of the 10 most abundant species and other species (<15 %) in plant communities exposed to two levels of CO2 concentration (390 and 520 ppm) with or without an extreme climatic event (ECE) at three different cutting dates.
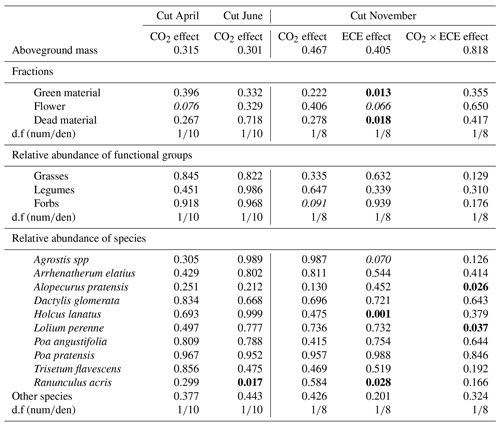
The d.f (numden) signifies degrees of freedom (numerator and denominator). In bold: p<0.05; in italics: .
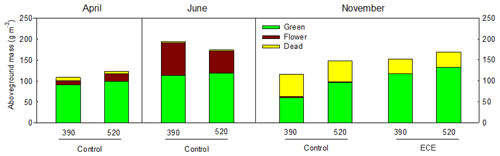
Figure 2Aboveground biomass of green material, dead material and flowers measured before (April, June) and after (November) the extreme climatic event (ECE) exposed to four climate scenarios: 390 or 520 ppm atmospheric CO2 concentration without (control) or combined with the ECE; n=3.
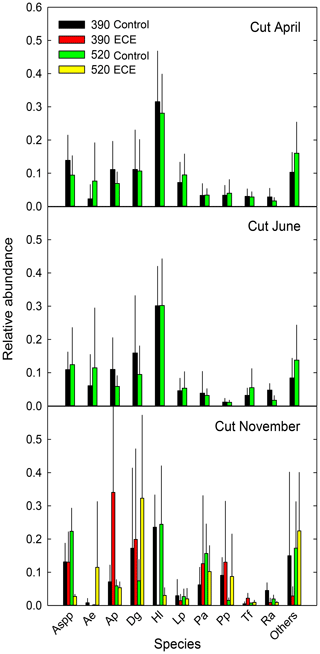
Figure 3Relative abundance of the 10 most abundant species present in the community measured before (April, June) and after (November) the extreme climatic event (ECE) grown under four climate scenarios: 390 or 520 ppm atmospheric CO2 concentration without (control) or combined with the ECE. Aspp: Agrostis spp.; Ae: Arrhenatherum elatius L.; Ap: Alopecurus pratensis L.; Dg: Dactylis glomerata L.; Hl: Holcus lanatus L.; Lp: Lolium perenne L.; Pa: Poa angustifolia L.; Pp: Poa pratensis L.; Tf: Trisetum flavescens L.; Ra: Rumex acetosa L.; Others: <15 % of species; n=3.
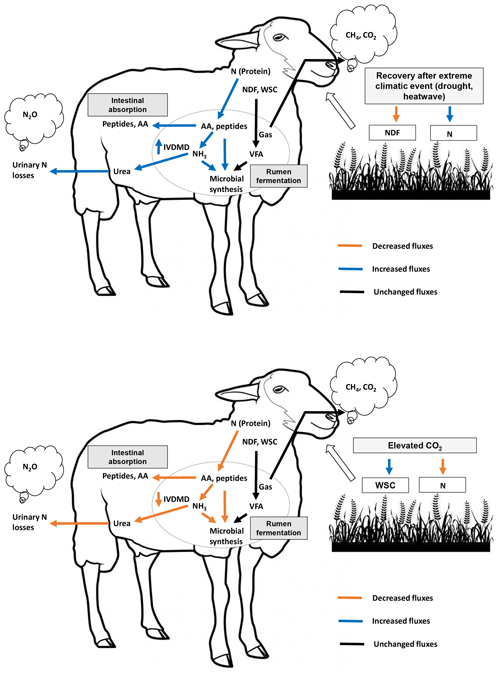
Figure 4Schematic overview of treatment effects (recovery after an extreme climatic event, ECE, and elevated atmospheric CO2 concentration) on forage quality, digestive use of macronutrients by the ruminant and atmospheric greenhouse gas emissions. WSCs: water-soluble carbohydrates; N: nitrogen; NDF: neutral detergent fiber (cell wall carbohydrates); AAs: amino acids; VFAs: volatile fatty acids; CH4: methane; CO2: carbon dioxide; NH3: ammonia; N2O: nitrous oxide; IVDMD: in vitro dry matter degradability.
3.1 Aboveground biomass characteristics and chemical composition
Aboveground biomass did not differ significantly among treatments. The November cut had more green material (p=0.013) and less dead material (p=0.018) with the ECE than in the control (Tables 2 and S1 in the Supplement and seasonal pattern shown in Fig. 2). There were very few differences among treatments in terms of relative abundances of functional groups or species (Table 2, Tables S1 and S2 in the Supplement, Fig. 3) due to large variability among the macrocosms, except for Holcus lanatus which decreased dramatically after the ECE (p=0.001). There was a seasonality-driven cutting date effect on aboveground biomass, fractions of green and dead materials and flowers (p<0.001), and relative abundances of several species but without a significant cutting date and CO2 effect (Table S2).
The N content in the aboveground biomass was significantly lower at 520 ppm CO2 concentration compared to 390 ppm (Tables 3 and 4). This was shown in cuts from every season: April (−11 %, p<0.001), June (−9 %, p=0.003) and November (−21 %, p=0.007). Increasing the CO2 concentration caused an increase in OM content in April (+1 %, p=0.033) and a decrease in NDF content in June (−3 %, p=0.002), as well as increased C:N ratio and NDF:N ratio at the three cutting dates (p<0.05). After ECE recovery in November, there were strong increases in N content (+54 %, p<0.001), sucrose content (+31 %, p=0.022) and fructose content (+23 %, p=0.031). The ECE significantly decreased NDF content (−7 %, p=0.027), C:N ratio (−34 %, p<0.001) and NDF:N ratio (−39 %, p<0.001) and increased pepsin-cellulase OM digestibility (+14 %, p=0.005). There was no effect of the CO2–ECE interaction on chemical composition of the aboveground biomass (p>0.05). There was a significant cutting date effect on all the chemical composition parameters except C content, with a significant cutting date and CO2 effect on N content (p<0.001), C:N ratio (p<0.001) and NDF:N ratio (p<0.001) (Table S3 in the Supplement). By taking all data into account (36 observations), many significant relationships were observed between aboveground biomass characteristics and chemical composition of forage (Table S4 in the Supplement). Chemical composition was strongly driven by green material percentage, as well as plant species abundances, especially those of Alopecurus pratensis, Holcus lanatus and Lolium perenne (Fig. S1 in the Supplement).
3.2 In vitro rumen fermentation characteristics
Estimated IVDMD was significantly lower (−3 %, p=0.041) in plants exposed to 520 ppm CO2 concentration compared to 390 ppm for the April cut and tended to be lower for the November cut (p=0.075) (Tables 3 and 4). Increasing the level of CO2 drastically decreased the NH3 concentration in the incubation medium for all cuts (−21 %, p=0.014; −31 %, p=0.001; and −34 %, p=0.005, respectively), decreased the proportion of valerate for the cuts of April and June (−7 %, p=0.016 and p=0.017, respectively), and increased the acidification for the November cut (+11 %, p=0.007). Increasing the level of CO2 also tended to increase total VFA concentration (p=0.056) and decrease the proportion of isovalerate for the April cut (p=0.062), as well as decrease the proportion of isobutyrate for the November cut (p=0.063). The ECE treatment very significantly increased NH3 concentration in the incubation medium (+90 %, p<0.001), IVDMD (+10 %, p=0.001), and the proportions of propionate, valerate and isovalerate (+4 %, p=0.008; +21 %, p=0.004; and +25 %, p=0.006, respectively), and it tended to decrease the proportion of acetate (p=0.064), whereas the acetate : propionate ratio, which is related to the fermentation pathways in the rumen, decreased (−5 %, p=0.013). The CO2–ECE interaction tended to have effects on IVDMD (p=0.053) and isovalerate concentration (p=0.067). There was a cutting date effect (seasonality) on all in vitro rumen fermentation parameters except isovalerate concentration and CO2:CH4 ratio, with a significant cutting date and CO2 effect on NH3, valerate and isovalerate concentrations (Table S3).
By taking all data into account, many strong significant relationships were observed between in vitro rumen fermentation characteristics and chemical composition of forage (Table S4). For example, IVDMD was negatively correlated with NDF and NDF:N ratio and positively correlated with fructan content. The NH3 emission was positively correlated with aboveground biomass N content (Table S4, Fig. S1 in the Supplement). As expected due to the link between chemical composition and aboveground biomass characteristics, significant correlations were observed between in vitro rumen fermentation characteristics and plant species abundances, especially those of Lolium perenne, Holcus lanatus and Alopecurus pratensis (Table S4, Fig. S1). Acidification and CH4 emission appeared to be strongly positively driven by the abundance of Lolium perenne (Table S4), while NH3 emission was driven by that of Alopecurus pratensis (Fig. S1). The strong negative relationship between Holcus lanatus abundance and isovalerate production (Fig. S1) suggests that the increase in isovalerate production following the ECE was due to the decline of Holcus lanatus.
Table 3Chemical composition and in vitro rumen fermentation parameters of plant communities exposed to two levels of CO2 concentration (390 and 520 ppm) with or without an extreme climatic event (ECE) at three different cutting dates.
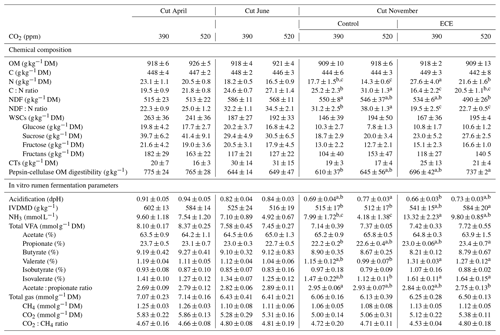
Data shown are means ± standard deviation. a,b,c For the November
cut, means in a given row with different letters are significantly different
(p<0.05).
OM: organic matter; C: carbon; N: nitrogen; NDF: neutral detergent fiber;
WSCs: water-soluble carbohydrates; CTs: condensed tannins; IVDMD: in vitro dry matter
degradability; DM: dry matter; VFAs: volatile fatty acids.
4.1 Elevated CO2 and ECE modify aboveground biomass and botanical composition
In this experiment, aboveground biomass was not affected by elevated CO2 or an ECE probably because the control was actually exposed to drier and warmer conditions compared to the current ones (referred to as the mean of the 1990–2009 period). These conditions were applied to allow us to compare the effect of elevated CO2 and ECE under the projected year 2050 climate scenario. The fact that the control was under little stress may have limited biomass growth and may explain why no difference was observed between treatments with and without an ECE, as expected. Although there was no overall effect of elevated CO2 on the plant fractions, we nevertheless observed a significant increase in green mass for the control in November. This can be related to increases in leaf area index and canopy photosynthesis linked with higher SWC under elevated CO2 as indicated by additional measurements made in the same experiment (Roy et al., 2016).
There was no effect of the ECE on aboveground biomass for the November cut, although it had more green tissue and less dead tissue compared to the control. This could be attributed to a strong increase in the shoot N pool driven primarily by an effect of the ECE on the belowground compartment (Roy et al., 2016). These authors showed that ECEs strongly increased the root N pool, thereby increasing N availability. The CO2–ECE interaction suggests that SWC before the November cut in the 520 ppm CO2 treatment was reduced with the ECE and was enhanced in the control compared to the other treatments. This could be explained by enhanced leaf area index, photosynthesis, greenness and C sequestration leading to higher water extraction from the soil after an ECE, corresponding to higher recovery under elevated CO2 (Roy et al., 2016).
Table 4Factor effects (p values) on chemical composition and in vitro rumen fermentation parameters of plant communities exposed to two levels of CO2 concentration (390 and 520 ppm) with or without an extreme climatic event (ECE) at three different cutting dates.
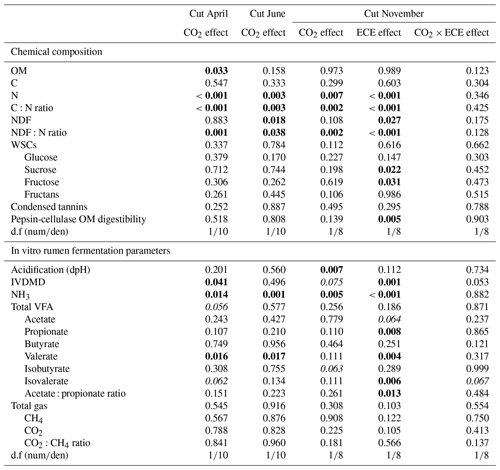
OM: organic matter; C: carbon; N: nitrogen; NDF: neutral detergent fiber; WSCs: water-soluble carbohydrates; CTs: condensed tannins; IVDMD: in vitro dry matter degradability; VFAs: volatile fatty acids; d.f (numden): degrees of freedom (numerator and denominator). In bold: p<0.05; in italics: .
The lack of significant differences in relative abundances of functional groups and species can be attributed to the huge variability in ecosystem responses to elevated CO2 and ECE. We did, however, observe a notable change due to the high vulnerability to the ECE of Holcus lanatus, which mostly disappeared. Volaire et al. (2020) recently showed that soluble carbohydrate metabolism, particularly fructans and sucrose, plays a role in the lack of Holcus lanatus recovery from the ECE.
4.2 Elevated CO2 and ECE modify the chemical composition of aboveground biomass
We observed contrastive effects of elevated CO2 and ECE on the chemical composition of aboveground biomass. The lower N concentration under elevated CO2 compared to the control was shown for all the cutting dates and is consistent with findings from meta-analyses addressing climate change effects on grassland (Dumont et al., 2015; Dellar et al., 2018). Note that this lower N concentration was not accompanied by significant changes in legume proportions, which could have been an explanatory factor due to their high N content. The reduction in N content may therefore reflect a combination of increased growth and changes in photosynthetic N use efficiency (Leakey et al., 2009). Even though NDF concentration was only affected for the June cut, the NDF:N ratio increased at elevated CO2 concentrations for all cutting dates but without a negative impact on pepsin-cellulase OM digestibility. This parameter could have been affected as a high concentration of partially digestible fiber (NDF) and a limiting N concentration can be detrimental for the microbial ecosystem in the rumen (Sinclair et al., 1995). These results are globally well in line with those described in a recent study on mixed grass prairie (Augustine et al., 2018) and a meta-analysis on the effects of climate change on pasture quality in Europe (Dellar et al., 2018).
We observed a clear increase in N concentrations in aboveground biomass from the November cut. ECE-driven dehydration of plant material may have resulted in the asynchrony between plants and soil microbial community functioning. High plant litter and microbial detritus during the ECE are both sources of energy for microbial recovery in soil during rehydration, which is faster than in plants (Hofer et al., 2017). Microbial mineralization of the OM produces inorganic N that plants can only uptake if they have recovered. In addition, it has been shown that the maintenance of root exudates during drought may be one of the factors that enable aboveground recovery, since root functionality ensures increased N availability (Karlowsky et al., 2018). The flush of N can explain the increase in N and sucrose content in the aboveground biomass due to high photosynthesis and transpiration indicated by lower SWC (Roy et al., 2016). An increase in fructose content, as observed here after the ECE, is usually linked to hydrolysis of fructans (Simpson and Bonnett, 1993), but we did not find any significant decrease in fructan content in this experiment. These ECE-driven changes led to a much lower (∼50 %) NDF:N ratio than in the control. A low NDF:N ratio, in addition to effects on some sugars, is particularly beneficial in terms of forage quality, as confirmed by higher pepsin-cellulase OM digestibility due to an increase in readily degradable nutrients providing increased amounts of energy and nitrogen for rumen microbial synthesis (Nocek and Russell, 1988).
4.3 Changes in chemical composition affect rumen fermentation parameters
The changes in chemical composition in aboveground biomass following the ECE strongly affected rumen fermentation parameters. The lower NDF content led naturally to increased IVDMD as some fibers, especially lignin, are known to be indigestible (Jung and Allen, 1995). This is consistent with the increase in pepsin-cellulase OM digestibility, a parameter that is closely correlated with in vivo digestibility (Aufrère and Michalet-Doreau, 1988). Interestingly, despite an increase in IVDMD following the ECE, we did not find any of the usually observed increased total gas production, including CH4 (Getachew et al., 2004). This could mean that a potential increase in energy available for the animal was not accompanied by more energy losses and pollutant emissions. This could be due to the changes observed in VFA profiles (acetate, propionate and valerate) indicating changes in fermentation pathways. In particular, we observed a decrease in the acetate : propionate ratio, which is known to be related to hydrogen availability and CH4 production in the rumen (Russell, 1998). The increase in N concentration after the ECE, which may be partly linked to the increase in the abundance of Alopecurus pratensis, also led to an increase in ruminal NH3, which is a main end product of protein degradability through amino acid deamination. The increase in isovalerate with the ECE, which appears to be related to the decrease in Holcus lanatus abundance, also indicates increased protein degradation as this branched-chain VFA results from deamination of branched-chain amino acids such as leucine (Menahan and Schultz, 1964). Part of the NH3 produced is incorporated into the rumen microbial biomass, but the surplus is transformed into urea, which gets excreted into the environment and thus drives N loss and polluting emissions. Indeed, the fraction of urinary N not used by soil microbes and plants is transformed into N2O, a potent GHG, during microbial processes of nitrification and denitrification (Firestone et al., 1980).
The changes in chemical composition under elevated CO2 affected rumen fermentation parameters in a different manner compared to the ECE. Interestingly, we observed contrastive effects according to cutting date. Elevated CO2 decreased IVDMD for the April cut but then increased IVDMD in November following the ECE, resulting in a trend towards a CO2–ECE interaction. This could mean that the ECE counteracts the negative effect of elevated CO2 on IVDMD likely due to the decrease in NDF concentration. Note, however, that we never observed any significant change in VFA production, which is one of the main drivers of energetic value for the animal as VFAs provide more than 70 % of ruminant energy supply (Bergman, 1990). For all cutting dates, the decrease in N content in aboveground biomass under elevated CO2 led to lower NH3 concentrations in the rumen for the reasons given above.
Analysis of the correlation matrix (data not shown) showed a positive correlation between CT content in aboveground biomass and CO2:CH4 ratio in fermentation gas (r=0.51, p=0.002) and negative correlations between CT content and IVDMD (, p=0.024) and total VFA production (, p=0.015). The antimethanogenic effect of CTs and the reduction of IVDMD are consistent with the literature (meta-analysis by Jayanegara et al., 2012). However, in this experiment, mean CT content values were higher following the ECE compared to the control, but the difference was not significant.
Figure 4 provides a schematic illustrative overview of the results obtained in this experiment, showing the impact of the ECE and elevated CO2 on ruminant digestive degradation of plant macronutrients (carbohydrates and protein). Our findings suggest that the ECE, by increasing aboveground biomass N content, increased N metabolism in the rumen, probably with a positive effect on rumen microbial synthesis. This, along with the lower in-plant NDF content and higher sucrose and fructose contents, may explain the observed increase in IVDMD. In contrast, the elevated atmospheric CO2 concentration reduced aboveground biomass N content and ruminal N metabolism, thereby resulting in a negative impact on IVDMD.
This study shows that different drivers of climate change, i.e. elevated atmospheric CO2 concentration and ECEs (drought and heat wave), have contrastive impacts on forage quality through their effects on plant characteristics. An ECE was followed by an increase in greenness and in N and water-soluble carbohydrate contents in the aboveground biomass produced during the regrowth stage, resulting in higher OM digestibility. Taken together, our results point to the NDF:N ratio as a major driver of forage quality which is highly likely to be affected differently by elevated CO2 and ECE, both of which will have strong impacts on rumen fermentation. In addition, our results on the CO2–ECE interaction indicate that elevated CO2 may limit the ECE-driven gain of IVDMD.
No data sets were used in this article.
The supplement related to this article is available online at: https://doi.org/10.5194/bg-18-4841-2021-supplement.
CPC designed the study, AMB, ALM, AA, MLD, VN and CPC contributed to the acquisition, analysis and interpretation of data. VN wrote the manuscript with contributions from all co-authors.
The authors declare that they have no conflict of interest.
Publisher's note: Copernicus Publications remains neutral with regard to jurisdictional claims in published maps and institutional affiliations.
This study benefited from human resources (CNRS staff) and technical resources allocated to the ECOTRONS Research Infrastructure and from the French government-sponsored “Investissement d'Avenir” funding (AnaEE-France ANR-11-INBS-0001). We thank the technical staff of INRAE Herbipole and UREP groups for extracting the intact soil monoliths; the Ecotron team, Olivier Darsonville, Lionel Thiery and Marine Zwicke for their technical help during this experiment; Didier Macheboeuf for his expertise in in vitro rumen fermentation; and Pierre-Jean Haupais for his contribution to soluble carbohydrate analysis. Angela Augusti and Marie-Lise Benot received post-doctorate positions through an INRAE scientific package (2010–2014). Angela Augusti was also supported by the European FP7 ExpeRT Transnational Access program.
This research has been supported by the FP7 in Food, Agriculture and Fisheries and Biotechnology (Animal Change project; grant no. 266018). This study was supported by the European Community FP7 funding (Animal Change project, grant agreement number 266018, 2007–2013) and an ANR project grant (VALIDATE).
This paper was edited by Paul Stoy and reviewed by two anonymous referees.
Andueza, D., Rodrigues, A. M., Picard, F., Rossignol, N., Baumont, R., Cecato, U., and Farruggia, A.: Relationships between botanical composition, yield and forage quality of permanent grasslands over the first growth cycle, Grass Forage Sci., 71, 366–378, https://doi.org/10.1111/gfs.12189, 2016.
Aufrere, J. and Michalet-Doreau, B.: Comparison of methods for predicting digestibility of feeds, Anim. Feed Sci. Tech., 20, 203–218, https://doi.org/10.1016/0377-8401(88)90044-2, 1988.
Augustine, D. J., Blumenthal, D. M., Springer, T. L., LeCain, D. R., Gunter, S. A., and Derner, J. D.: Elevated CO2 induces substantial and persistent declines in forage quality irrespective of warming in mixedgrass prairie, Ecol. Appl., 28, 721–735, https://doi.org/10.1002/eap.1680, 2018.
Benot, M. L., Morvan-Bertrand, A., Mony, C., Huet, J., Sulmon, C., Decau, M. L., Prud'Homme, M. P., and Bonis, A.: Grazing intensity modulates carbohydrate storage pattern in five grass species from temperate grasslands, Acta Oecol., 95, 108–115, https://doi.org/10.1016/j.actao.2018.11.005, 2019.
Bergman, E. N.: Energy contributions of volatile fatty acids from the gastrointestinal tract in various species, Physiol. Rev., 70, 567–590, https://doi.org/10.1152/physrev.1990.70.2.567, 1990.
Boé, J., Terray, L., Habets, F., and Martin, E.: A simple statistical-dynamical downscaling scheme based on weather types and conditional resampling, J. Geophys. Res.-Atmos., 111, D23, https://doi.org/10.1029/2005JD006889, 2006.
Ciais, P., Reichstein, M., Viovy, N., Granier, A., Ogée, J., Allard, V., Buchmann, N., Aubinet, M., Bernhofer, C., Carrara, A., Chevallier, F., De Noblet, N., Friend, A., Friedlingstein, P., Grünwald, T., Heinesch, B., Keronen, P., Knohl, A., Krinner, G., Loustau, D., Manca, G., Matteucci, G., Miglietta, F., Ourcival, J. M., Pilegaard, K., Rambal, S., Seufert, G., Soussana, J. F., Sanz, M. J., Schulze, E. D., Vesala, T., and Valentini, R.: Europe-wide reduction in primary productivity caused by the heat and drought in 2003, Nature, 437, 529–533, https://doi.org/10.1038/nature03972, 2005.
Dellar, M., Topp, C. F. E., Banos, G., and Wall, E.: A meta-analysis on the effects of climate change on the yield and quality of European pastures, Agr. Ecosyst. Environ., 265, 413–420, https://doi.org/10.1016/j.agee.2018.06.029, 2018.
Dumont, B., Andueza, D., Niderkorn, V., Lüscher, A., Porqueddu, C., and Picon-Cochard, C.: A meta-analysis of climate change effects on forage quality in grasslands: specificities of mountain and Mediterranean areas, Grass Forage Sci., 70, 239–254, https://doi.org/10.1111/gfs.12169, 2015.
Firestone, M. K., Firestone, R. B., and Tiedje, J. M.: Nitrous oxide from soil denitrification: factors controlling its biological production, Science, 208, 749–751, https://doi.org/10.1126/science.208.4445.749, 1980.
Gerber, P. J., Steinfeld, H., Henderson, B., Mottet, A., Opio, C., Dijkman, J., Falcucci, A., and Tempio, G.: Tackling climate change through livestock: a global assessment of emissions and mitigation opportunities, Food and Agriculture Organization of the United Nations (FAO), Rome, 2013.
Getachew, G., Robinson, P. H., DePeters, E. J., and Taylor, S. J.: Relationships between chemical composition, dry matter degradation and in vitro gas production of several ruminant feeds, Anim. Feed Sci. Tech., 111, 57–71, https://doi.org/10.1016/S0377-8401(03)00217-7, 2004.
Grabber, J. H., Zeller, W. E., and Mueller-Harvey, I.: Acetone enhances the direct analysis of procyanidin-and prodelphinidin-based condensed tannins in Lotus species by the butanol–HCl–iron assay, J. Agr. Food Chem., 61, 2669–2678, https://doi.org/10.1021/jf304158m, 2013.
Goering, H. K. and Van Soest, P. J.: Forage Fiber Analyses (Apparatus, Reagents, Procedures, and Some Applications), Agric. Handbook No. 379.ARS-USDA, Washington, DC, 1970.
Herrero, M., Havlík, P., Valin, H., Notenbaert, A., Rufino, M. C., Thornton, P. K., Blümmel, M., Weiss, F., Grace, D., and Obersteiner, M.: Biomass use, production, feed efficiencies, and greenhouse gas emissions from global livestock systems, P. Natl. Acad. Sci. USA, 110, 20888–20893, https://doi.org/10.1073/pnas.1308149110, 2013.
Herrero, M., Henderson, B., Havlík, P., Thornton, P. K., Conant, R. T., Smith, P., Wirsenius, S., Hristov, A. N., Gerber, P., Gill, M., Butterbach-Bahl, K., Valin, H., Garnett, T., and Stehfest, E.: Greenhouse gas mitigation potentials in the livestock sector, Nat. Clim. Change, 6, 452–461, https://doi.org/10.1038/nclimate2925, 2016.
Hofer, D., Suter, M., Buchmann, N., and Lüscher, A.: Nitrogen status of functionally different forage species explains resistance to severe drought and post-drought overcompensation, Agr. Ecosyst. Environ., 236, 312–322, https://doi.org/10.1016/j.agee.2016.11.022, 2017.
INRA: INRA feeding system for ruminants, Wageningen Academic Publishers, Wageningen, NLD, 2018.
IPCC: Working group I contribution to the fifth assessment report of the intergovernmental panel on climate change, in: Climate Change 2013 – The Physical Science Basis by Intergovernmental Panel on Climate Change, edited by: Stocker, T. F., Qin, D., Plattner, G. K., Tignor, M., Allen, S. K., Boschung, J., Nauels, A., Xia, Y., Bex, V., and Midgley, P. M., Cambridge University Press, Cambridge, UK and New York, NY, USA, https://doi.org/10.1017/CBO9781107415324, 2013.
Jayanegara, A., Leiber, F., and Kreuzer, M.: Meta-analysis of the relationship between dietary tannin level and methane formation in ruminants from in vivo and in vitro experiments, J. Anim. Physiol. An. N., 96, 365–375. https://doi.org/10.1111/j.1439-0396.2011.01172.x, 2012.
Jung, H. G. and Allen, M. S.: Characteristics of plant cell walls affecting intake and digestibility of forages by ruminants, J. Anim. Sci., 73, 2774–2790, https://doi.org/10.2527/1995.7392774x, 1995.
Karlowsky, S., Augusti, A., Ingrisch, J., Akanda, M. K. U., Bahn, M., and Gleixner, G.: Drought-induced accumulation of root exudates supports post-drought recovery of microbes in mountain grassland, Front. Plant Sci., 9, 1593, 1–16, https://doi.org/10.3389/fpls.2018.01593, 2018.
Leakey, A. D., Ainsworth, E. A., Bernacchi, C. J., Rogers, A., Long, S. P., and Ort, D. R.: Elevated CO2 effects on plant carbon, nitrogen, and water relations: six important lessons from FACE, J. Exp. Bot., 60, 2859–2876, https://doi.org/10.1093/jxb/erp096, 2009.
Lee, M. A., Davis, A. P., Chagunda, M. G. G., and Manning, P.: Forage quality declines with rising temperatures, with implications for livestock production and methane emissions, Biogeosciences, 14, 1403–1417, https://doi.org/10.5194/bg-14-1403-2017, 2017.
Menahan, L. A. and Schultz, L. H.: Metabolism of leucine and valine within the rumen, J. Dairy Sci., 47, 1080–1085, https://doi.org/10.3168/jds.S0022-0302(64)88849-4, 1964.
Nakicenovic, N., Alcamo, J., Grubler, A., Riahi, K., Roehrl, R. A., Rogner, H. H., and Victor, N.: Special report on emissions scenarios (SRES), a special report of Working Group III of the intergovernmental panel on climate change, Cambridge University Press, UK, 2000.
Niderkorn, V., Baumont, R., Le Morvan, A., and Macheboeuf, D.: Occurrence of associative effects between grasses and legumes in binary mixtures on in vitro rumen fermentation characteristics, J. Anim. Sci., 89, 1138–1145, https://doi.org/10.2527/jas.2010-2819, 2011.
Nisbet, E. G., Manning, M. R., Dlugokencky, E. J., Fisher, R. E., Lowry, D., Michel, S. E., Lund Myhre, C., Platt, S. M., Allen, G., Bousquet, P., Brownlow, R., Cain, M., France, J. L., Hermansen, O., Hossaini, R., Jones, A. E., Levin, I., Manning, A. C., Myhre, G., Pyle, J. A., Vaughn, B. H., Warwick, N. J., and White, J. W. C.: Very strong atmospheric methane growth in the 4 years 2014–2017: Implications for the Paris Agreement, Glob. Biogeochem. Cy., 33, 318–342, https://doi.org/10.1029/2018GB006009, 2019.
Nocek, J. E. and Russell, J.: Protein and energy as an integrated system. Relationship of ruminal protein and carbohydrate availability to microbial synthesis and milk production, J. Dairy Sci., 71, 2070–2107, https://doi.org/10.3168/jds.S0022-0302(88)79782-9, 1988.
Park, G., Oh, H., and Ahn, S.: Improvement of the ammonia analysis by the phenate method in water and wastewater, B. Korean Chem. Soc., 30, 2032–2038, https://doi.org/10.5012/bkcs.2009.30.9.2032, 2009.
Planton, S., Déqué, M., Chauvin, F., and Terray, L.: Expected impacts of climate change on extreme climate events, C. R. Geosci., 340, 564–574, https://doi.org/10.1016/j.crte.2008.07.009, 2008.
Rossignol, N., Andueza, D., Carrère, P., Cruz, P., Duru, M., Fiorelli, J. L., Michaud, A., Plantureux, S., Pottier, E., and Baumont, R.: Assessing population maturity of three perennial grass species: influence of phenology and tiller demography along latitudinal and altitudinal gradients, Grass Forage Sci., 69, 534–548, https://doi.org/10.1111/gfs.12067, 2014.
Roy, J., Picon-Cochard, C., Augusti, A., Benot, M. L., Thiery, L., Darsonville, O., Landais, D., Piel, C., Defossez, M., Devidal, S., Escape, C., Ravel, O., Fromin, N., Volaire, F., Milcu, A., Bahn, M., and Soussana, J. F.: Elevated CO2 maintains grassland net carbon uptake under a future heat and drought extreme, P. Natl. Acad. Sci. USA, 113, 6224–6229, https://doi.org/10.1073/pnas.1524527113, 2016.
Russell, J. B.: The importance of pH in the regulation of ruminal acetate to propionate ratio and methane production in vitro, J. Dairy Sci., 81, 3222–3230, https://doi.org/10.3168/jds.S0022-0302(98)75886-2, 1998.
Sinclair, L. A., Garnsworthy, P. C., Newbold, J. R., and Buttery, P. J.: Effects of synchronizing the rate of dietary energy and nitrogen release in diets with a similar carbohydrate composition on rumen fermentation and microbial protein synthesis in sheep, J. Agric. Sci., 124, 463–472, https://doi.org/10.1017/S0021859600073421, 1995.
Simpson, R. J. and Bonnett, G. D.: Fructan exohydrolase from grasses, New Phytol., 123, 453–469, https://doi.org/10.1111/j.1469-8137.1993.tb03757.x, 1993.
Theodorou, M. K., Williams, B. A., Dhanoa, M. S., McAllan, A. B., and France, J.: A simple gas production method using a pressure transducer to determine the fermentation kinetics of ruminant feeds, Anim. Feed Sci. Tech., 48, 185–197, https://doi.org/10.1016/0377-8401(94)90171-6, 1994.
Thornton, P. K. and Herrero, M.: Potential for reduced methane and carbon dioxide emissions from livestock and pasture management in the tropics, P. Natl. Acad. Sci. USA, 107, 19667–19672, https://doi.org/10.1073/pnas.0912890107, 2010.
Tilman, D. and Clark, M.: Global diets link environmental sustainability and human health, Nature, 515, 518–522, https://doi.org/10.1038/nature13959, 2014.
Van Soest, P. J., Robertson, J. B., and Lewis, B. A.: Methods for dietary fiber, neutral detergent fiber, and nonstarch polysaccharides in relation to animal nutrition, J. Dairy Sci., 74, 3583–3597, https://doi.org/10.3168/jds.S0022-0302(91)78551-2, 1991.
Volaire F., Morvan-Bertrand, A., Prudhomme, M. P., Benot, M. L., Augusti, A., Zwicke, M., Roy, J., Landais, D., and Picon-Cochard, C.: Non-structural carbohydrate metabolism drives the resilience of perennial grass species after extreme summer drought and heat under elevated CO2, J. Exp. Bot., 71, 370–385, https://doi.org/10.1093/jxb/erz424, 2020.
Wolf, J., Asrar, G. R., and West, T. O.: Revised methane emissions factors and spatially distributed annual carbon fluxes for global livestock, Carbon Balance Manag., 12, 16, https://doi.org/10.1186/s13021-017-0084-y, 2017.