the Creative Commons Attribution 4.0 License.
the Creative Commons Attribution 4.0 License.
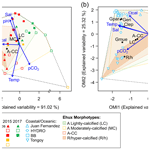
Abundances and morphotypes of the coccolithophore Emiliania huxleyi in southern Patagonia compared to neighbouring oceans and Northern Hemisphere fjords
Francisco Díaz-Rosas
Catharina Alves-de-Souza
Emilio Alarcón
Eduardo Menschel
Humberto E. González
Rodrigo Torres
Peter von Dassow
Coccolithophores are potentially affected by ongoing ocean acidification, where rising CO2 lowers seawater pH and calcite saturation state (Ωcal). Southern Patagonian fjords and channels provide natural laboratories for studying these issues due to high variability in physical and chemical conditions. We surveyed coccolithophore assemblages in Patagonian fjords during late spring 2015 and early spring 2017. Surface Ωcal exhibited large variations driven mostly by freshwater inputs. High-Ωcal conditions (max. 3.6) occurred in the Archipelago Madre de Dios. Ωcal ranged from 2.0–2.6 in the western Strait of Magellan and 1.5–2.2 in the inner channel and was subsaturating (0.5) in Skyring Sound. Emiliania huxleyi was the only coccolithophore widely distributed in Patagonian fjords (> 96 % of total coccolithophores), only disappearing in the Skyring Sound, a semi-closed mesohaline system. Correspondence analysis associated higher E. huxleyi biomasses with lower diatom biomasses. The highest E. huxleyi abundances in Patagonia were in the lower range of those reported in Norwegian fjords. Predominant morphotypes were distinct from those previously documented in nearby oceans but similar to those of Norwegian fjords. Moderately calcified forms of E. huxleyi A morphotype were uniformly distributed throughout Patagonia fjords. The exceptional R/hyper-calcified coccoliths, associated with low Ωcal values in Chilean and Peruvian coastal upwellings, were a minor component associated with high Ωcal levels in Patagonia. Outlying mean index (OMI) niche analysis suggested that pH and Ωcal conditions explained most variation in the realized niches of E. huxleyi morphotypes. The moderately calcified A morphotype exhibited the widest niche breadth (generalist), while the R/hyper-calcified morphotype exhibited a more restricted realized niche (specialist). Nevertheless, when considering an expanded sampling domain, including nearby southeast Pacific coastal and offshore waters, even the R/hyper-calcified morphotype exhibited a higher niche breadth than other closely phylogenetically related coccolithophore species. The occurrence of E. huxleyi in naturally low pH–Ωcal environments indicates that its ecological response is plastic and capable of adaptation.
- Article
(6959 KB) -
Supplement
(5901 KB) - BibTeX
- EndNote
Coccolithophores are small unicellular phytoplankton (3–30 µm) bearing intricate plates called coccoliths formed of calcite, the more stable form of calcium carbonate (CaCO3) (Monteiro et al., 2016). Coccolithophores can carry out a substantial portion of CaCO3 precipitation in pelagic systems (Broecker and Clark, 2009), which may enhance organic matter export by CaCO3 ballasting (Klaas and Archer, 2002), while also lowering alkalinity (Zondervan et al., 2001) and contributing to the carbonate counter pump (Passow and Carlson, 2012). Thus, understanding how coccolithophores respond to environmental stressors, such as ocean acidification (OA) due to rising pCO2, is necessary for predicting future ocean biogeochemistry.
Chemically, calcite stability in seawater can be parameterized using its saturation state, defined as Ωcal = [Ca2+] × [CO]/Kspcal (where Kspcal is the solubility constant for calcite), which is decreased by OA. When Ωcal<1, calcite dissolution is thermodynamically favoured, whereas calcite precipitation is favoured when Ωcal > 1. Most of the ocean surface is predicted to remain supersaturated with respect to calcite in the future ocean (Feely et al., 2009), although some coastal zones may experience Ωcal<1 in the euphotic zone either due to increased pCO2 in areas of naturally high CO2 upwelling (e.g., Franco et al., 2018) or due to freshening (e.g., Tynan et al., 2014). Furthermore, drops in Ωcal may negatively affect biological calcite production rates even before becoming undersaturated (Doney et al., 2009).
In contrast to other calcifying organisms, coccolith formation occurs intracellularly in Golgi-derived vesicles, involving sustained fluxes of Ca2+ and dissolved inorganic carbon (primarily HCO) from the external medium and high H+ efflux to maintain cellular pH homeostasis (reviewed by Taylor et al., 2017). Which extracellular carbonate chemistry parameter most influences intracellular coccolithophore calcification is debated, e.g., whether Ωcal or more complex relationships involving HCO, H+, and CO2 (Bach et al., 2015; Cyronak et al., 2016; Kottmeier et al., 2016; Gafar et al., 2018). Additionally, OA can have contrasting effects, with increased CO2 availability potentially benefiting photosynthesis but high H+ negatively affecting metabolisms besides calcification (Kottmeier et al., 2016; Paul and Bach, 2020). A wide range of calcification and growth responses to OA have been reported in laboratory studies of coccolithophores, mostly using the cosmopolitan and most abundant species Emiliania huxleyi (reviewed in Meyer and Riebesell, 2015). With some notable exceptions (e.g., Iglesias-Rodriguez et al., 2008), most culture studies showed reduced calcification rates of E. huxleyi in response to simulated OA, while there is no clear trend on growth rates. In a mesocosm experiment using a Norwegian fjord community, increased pCO2 levels (> 500 µatm) resulted in lower growth rates of E. huxleyi, preventing it from blooming (Riebesell et al., 2017). This result contrasted with a long-term observational study showing a steady increase in coccolithophore stocks (related to diatoms and dinoflagellates) from pre-industrial to present-day high pCO2 levels in the North Atlantic (Rivero-Calle et al., 2015), although both studies predict a decrease in net calcification.
Morphological variability in E. huxleyi has been reported with several morphotypes described so far with different degrees of calcification of the coccoliths, such as fusion of coccolith elements or calcite overgrowth (Young et al., 2003). Morphotype-A coccoliths have a grill central area and tend to be moderately calcified, while morphotypes B and C have more lightly calcified distal shield elements, and the central area is either a plate or open (type O) (Young and Westbroek, 1991; Hagino et al., 2011). Additional morphotypes, or morphotype sub-classes, include B/C (intermediate in coccolith size between B and C) and R (Reticulofenestra-like), considered an A morphotype where distal shield elements are mostly or completely fused (Hagino et al., 2011). However, the readily identifiable qualitative distinctions may not easily translate into quantitative differences in the calcite produced per cell in the ecosystem, as for example, due to variability in the rate of coccoliths produced per cell (Johnsen and Bollmann, 2020).
Nevertheless, cultured isolates maintain their morphotype classifications even under variable environmental conditions that can alter total calcite production and even lead to coccolith malformation (e.g., Young and Westbroek, 1991; Langer et al., 2011; Müller et al., 2015; von Dassow et al., 2018; Mella-Flores et al., 2018), suggesting a genetic determination of coccolith morphology. One genetic marker has been associated with morphological variability in E. huxleyi. The calcium-binding protein GPA has been potentially associated with E. huxleyi coccolith deposition (Corstjens et al., 1998). Although the function of this protein is unclear, the 3′ untranslated region (non-coding) showed consistent differences between morphotypes, with all morphotypes A and R showing alleles (coccolith morphology motifs) CMM I, III, or IV and B, B/C, and C morphotypes showing CMM II (Schroeder et al., 2005; Krueger-Hadfield et al., 2014). The uronic acid content of coccolith-associated polysaccharides also varies among strains, and the one R morphotype tested was much higher in this character than most of the other A morphotypes (Rickaby et al., 2016). It is likely that further comparative biochemical analyses following Rickaby et al. (2016) and/or associating comparative genomics analyses (e.g., studies such as Read et al., 2013; von Dassow et al., 2015; Bendif et al., 2019) with morphometric analyses may identify genetic markers associated with sub-types within the broader A and B morphotypes. However, mitochondrial phylogenies classify E. huxleyi into a warmer water clade and a colder water clade, and each clade contains both A (including R) and B (or B/C or O) morphotypes (Hagino et al., 2011; Bendif et al., 2014), and B/C morphotypes also occurred in different genetic groups defined by microsatellite markers (Krueger-Hadfield et al., 2014), although another microsatellite study did find a separation between A and B/C morphotypes (Cook et al., 2013). Therefore, different morphologies likely correspond to stable genetically determined phenotypes that might reflect adaptations selected to specific conditions within a taxon whose recent evolution has been as a single biological species (Filatov, 2019).
In a global survey, Beaufort et al. (2011) found a general pattern of decreasing calcification with increasing pCO2 and a concomitant decrease in CO. Interestingly, calcite mass variability was predominantly the result of assemblage shifts both among closely related species and among morphotypes of the same species, from predominance of large and heavily calcified Gephyrocapsa oceanica cells, through intermediate moderately calcified E. huxleyi (A morphotype), to more lightly calcified E. huxleyi cells (B/C or C morphotype). In the subtropical and tropical eastern South Pacific, an exceptionally hyper-calcified R morphotype of E. huxleyi (henceforth referred to as R/hyper-calcified, showing the complete fusion of distal shield elements and partial or complete calcite overgrowth of the coccolith central area) was dominant in coastal upwelling waters with relatively high pCO2 and low Ωcal levels, causing an inversion in the trend of calcite-per-coccolith vs. Ωcal seen in the rest of the ocean (Beaufort et al., 2011; von Dassow et al., 2018). Likewise, Smith et al. (2012) observed an increase in the proportion of E. huxleyi, corresponding to an “overcalcified” morphotype (with complete overgrowth of the coccolith central area but without fusion of distal shield elements, referred to hereon as A-CC for covered central area) that occurred during the winter decline of Ωcal in the Bay of Biscay (North Atlantic). These results suggested that perhaps the A-CC and R/hyper-calcified morphotypes are likely resistant to low Ωcal. However, while the B/C morphotype was reported to be especially sensitive to low Ωcal compared to moderately calcified and overcalcified strains of morphotype A (Müller et al., 2015), other experimental results did not find a higher resistance of the R/hyper-calcified subtype to high CO2 and low Ωcal when compared to moderately calcified strains of morphotype A isolated from neighbouring waters (von Dassow et al., 2018). These results highlight that it is unclear how the physiological effects of OA on coccolithophores in culturing conditions translate to community-level responses in the field.
Fjord systems provide especially interesting natural laboratories for observing how coccolithophores may be affected by environmental conditions due to high spatial and seasonal variability in chemical and biotic conditions. In the Norwegian fjord system and the neighbouring North Sea, E. huxleyi has been very well studied for over a century, where it forms dense spring blooms but is also prominent throughout the year (Birkenes and Braarud, 1952; Berge, 1962; Kristiansen et al., 1994; Fernandez et al., 1996; Egge et al., 2015). Southern Patagonia, on the Pacific side of South America, hosts the largest network of fjords and channels in the world. Aquatic ecosystems of southwest Patagonia (50–55∘ S) are dominated by the transition between oceanic and estuarine–brackish waters. Denser, saltier, nitrate- and phosphate-rich but silicate-poor Sub-Antarctic Surface Water (SAASW) intrudes below nitrate- and phosphate-poor but silicic-acid-rich surface waters influenced by substantial freshwater inputs (copious precipitation, rivers, glacier melt; Dávila et al., 2002; Sievers and Silva, 2008; Torres et al., 2014). Generally, surface waters with low salinity and low alkalinity are undersaturated in dissolved CO2 during the spring and summer seasons (Torres et al., 2011). The Archipelago Madre de Dios (AMD) is an interesting exception to this pattern, where extreme precipitation/runoff in the “limestone” basin on the western AMD produces surface waters with low salinity and high alkalinity while maintaining low dissolved silicate compared with the eastern basin (Torres et al., 2020). These gradients create a unique contrast for exploring the influence of chemical conditions on the ecology of calcified phytoplankton, as changes in Ωcal are driven mainly by freshening rather than upwelling of high pCO2.
In contrast to the Norwegian fjord system, E. huxleyi blooms have not been reported in Patagonian fjords; however information on coccolithophores in these waters is scarce. A study documenting coccolithophores in the Strait of Magellan found that this group represented a minor fraction of the small-sized phytoplankton (Zingone et al., 2011), but other published studies have not specifically sampled for coccolithophores. The Patagonian shelf on the Atlantic side experiences large E. huxleyi blooms (Poulton et al., 2011, 2013), but satellite observations suggest coccolithophore blooms are of lower intensity in the Pacific waters to the west (Hopkins et al., 2019). These observations raise the question of how coccolithophore communities on the western coast and fjords–channels of Patagonia compare with nearby oceans and with fjord systems in the Northern Hemisphere. Here, we evaluated how physical, chemical, and biological features influence the distribution, abundance, and biomass of coccolithophores as well as the proportions of E. huxleyi morphotypes of varying calcification levels throughout southern Patagonia fjords. In particular, three research questions were addressed. (i) What coccolithophore assemblages and E. huxleyi morphotypes are present in fjords and channels of southern Patagonia? (ii) How do these morphotypes and the co-occurring phytoplankton (mostly diatoms) vary with physical and chemical factors? (iii) Do the abundance and relative composition of E. huxleyi morphotypes reflect populations in adjacent Pacific, Atlantic, or Southern Ocean waters or instead exhibit similarities to the Norwegian fjord system?
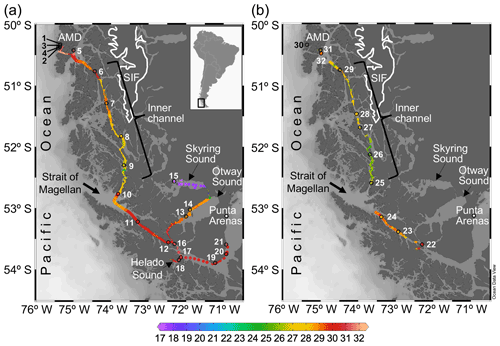
Figure 1Maps of southern Patagonia showing the study sites and stations sampled during the austral late spring 2015 (a) and early spring 2017 (b). Salinity recorded at the surface during the two cruises is plotted. The approximate perimeter of the Southern Ice Fields (SIF) is depicted. A zoom into the Archipelago Madre de Dios (AMD) zone with salinity and Ω calcite surface values recorded in 2015 is provided in Fig. S1. Maps were produced by Ocean Data View (Schlitzer, 2018).
2.1 Sampling
Two cruises were conducted on board the vessel M/N Forrest during the late austral spring 2015 (26 November to 3 December) and early austral spring 2017 (22 to 28 September) in southern Chilean Patagonia (∼ 50–54∘ S, 71–76∘ W). The sampling track of 2015 was from the Archipelago Madre de Dios (AMD), crossing the inner channel (IC) to the western part of the Strait of Magellan (WSM), and entering eastward into the Otway and Skyring sounds (OS, SS) to end up near Punta Arenas (Fig. 1a). The 2017 sampling was from the interior WSM, crossing the IC, and ending in the AMD zone (Fig. 1b). In both cruises, surface water (< 5 m) was pumped continuously on board every 15–20 min for determination of salinity and temperature with a YSI-30 termosalinometer (Yellow Springs, OH, USA) and pCO2 with a Qubit-S157 CO2 analyzer (Kingston, Ontario, Canada). The CO2 analyzer was calibrated daily with 0 ppm CO2 (air treated with soda lime) and 403 ppm air–CO2 mixture standard (Indura, Chile). Surface samples for determination of the planktonic assemblages and chemical variables (i.e., concentration of macronutrients, opal, total chlorophyll a, and the carbonate system parameters) were collected at discrete sampling stations (Fig. 1a–b). Twenty-one stations were sampled in 2015: five in the AMD (Sts. 1–5), four in the IC (Sts. 6–9), nine in the WSM (Sts. 10–12, 16–21), two in the OS (Sts. 13 and 14), and one in the SS (St. 15). Eleven stations were sampled in 2017: three in the AMD (Sts. 30–32), five in the IC (Sts. 25–29), and three in the WSM (Sts. 22–24).
Conductivity, temperature, and depth (CTD) vertical profiles were additionally obtained at selected localities on both cruises. In 2015, three casts were performed into the AMD zone and one cast into the SS using a CTD Seabird 19 plus (Sea-Bird Scientific, Bellevue, WA, USA) equipped with photosynthetically available radiation (PAR) and oxygen sensors. Two profiles were performed in 2017 in the AMD zone, by the deployment of a CTD Seabird 25 plus with PAR and oxygen sensors. The depth of 1 % of penetration of PAR (euphotic zone) was calculated from maximum surface PAR values. CTD profile binning was 1 m. In both years, samples for the determination of plankton assemblages and chemical variables were obtained at discrete depths using 5 L Niskin bottles to which the CTD profiles were attached (depths pre-determined from prior studies in the region, aiming to adequately sample the surface mixed layer, pycnocline, and vertical variation in chlorophyll fluorescence). Complete environmental and biological data are provided in the Supplement (Tables S1–S4).
2.2 Plankton assemblages
Samples for the determination of planktonic organisms through the Utermöhl (1958) method were collected only in 2015. For that, duplicate 100 mL water samples were pre-filtered through 200 µm Nitex mesh, fixed with a formaldehyde–glutaraldehyde solution (1 % formaldehyde, 0.05 % glutaraldehyde, 10 mM borate pH 8.5) and stored at 4 ∘C. In the laboratory, water samples were brought to room temperature, gently homogenized, and sedimented into 100 mL chambers for 24–48 h before counting and identification. The absolute abundances of the microplankton (20–200 µm in size) and coccolithophores (∼ 6 µm in diameter) were estimated with an inverted microscope (Olympus CKX41) connected to a digital camera (Motic 5.0). For counts of diatoms, dinoflagellates, and other planktonic cells greater than about 40 µm, the whole chamber was examined at 200× magnification. When large chain-forming diatoms were in high density, between 5–60 randomly selected fields of view were examined at 200× magnification until reaching 100 chains. For counts of small diatoms, naked flagellates (including small flagellates and athecated dinoflagellates), and coccolithophores, between one and four transects (to reach ≥100 cells in total) were analyzed at 400× magnification. Counts of total coccolithophores were performed with a 40× objective with cross-polarized light (Edmund Optics polarizers 54 926 and 53 347).
In both cruises, samples for the identification and quantification of coccolithophores through scanning electron microscopy (SEM) analysis were obtained by filtering 200–300 mL of surface water, immediately after sampling, onto 0.8 µm polycarbonate filters that were subsequently dried at room temperature. For the identification of coccolithophores and E. huxleyi morphotypes, a portion of each dried filter was cut, sputter-coated with gold, and examined in either a Quanta FEI 250 or Quanta FEG 250 SEM (both FEI, Hillsboro, Oregon, USA). As water samples for light microscopy counts were not available for two samples from 2015 (St. 3 and St. 5 at 8 m) and all samples from 2017, total coccolithophore abundances were obtained from SEM counts for those samples. On average, 70 images per filter were captured at 1500× magnification (276×184 µm per frame), covering 3.5 mm2 of the filter area corresponding to 1.8–3.4 mL of water analyzed. The coccolithophore abundances were calculated using the following equation:
To check for differences in coccolithophore counts obtained through sedimentation and inverted light-microscopy versus filtration and SEM examination (hereinafter SEM and Utermöhl counts, respectively), polycarbonate filters from three selected Utermöhl samples (showing higher, medium, and lower coccolithophore abundances) were analyzed with SEM as outlined above. Coccolithophore SEM counts were consistently about twice as high compared to Utermöhl counts (average 1.84), agreeing with the correction factor suggested by Bollmann et al. (2002). Thus, all total coccolithophore counts obtained by the Utermöhl method were multiplied by 1.84 to be comparable to SEM counts. To estimate the absolute abundances at species and morphotype level, the relative abundance of each coccolithophore species or E. huxleyi morphotype determined from SEM counts was multiplied by the absolute abundance of total coccolithophore cells. Saturation curves confirmed that the number of analyzed coccospheres (minimum 40 coccospheres per sample) was enough to capture the specific/morphotype diversity.
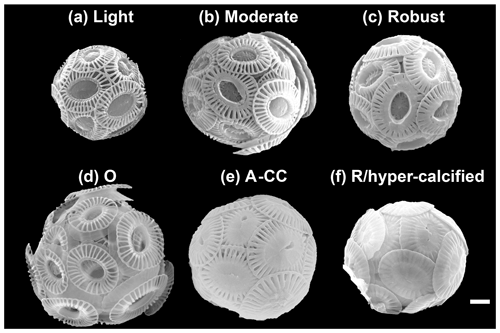
Figure 2The main E. huxleyi morphotypes recorded in the surface waters of southern Patagonia fjords during the austral late spring 2015 and early spring 2017. The lightly, moderately, and robustly calcified A morphotypes (top) and the O, A-CC, and R/hyper-calcified forms (bottom) are shown. For statistical analysis, the moderately and robustly calcified A morphotypes were merged under “moderately calcified A morphotype”, and the few O and C specimens were categorized into the lightly calcified subgroup. Scale bar = 1 µm.
Table 1Classification of E. huxleyi coccospheres based on the calcification level of coccoliths. Mean ± standard deviations of distal shield length and coccosphere diameter of morphotypes observed in Patagonia are given for coccospheres where at least one distal shield was visible and adequately oriented (numbers in parentheses).
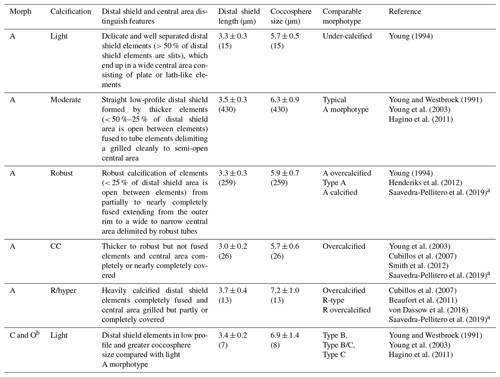
a The Plate Ib–c of Saavedra-Pellitero et al. (2019) are grouped together as “overcalcified” in that reference but are distinguished in the present study as R/hyper-calcified and A-CC morphotypes. Plate Ie of Saavedra-Pellitero et al. (2019) corresponds to overcalcified A morphotype in Young (1994) and the present study. Similarly, the “Type A overcalcified” in Fig. 3c of Cubillos et al. (2007) corresponds to the A-CC morphotype here (as distal shield elements are not fused or only partly fused in most coccoliths) while Fig. 3d of the same reference, identified also as “Type A overcalcified” appears to show both nearly complete fusion of the distal shield element and nearly complete overcalcification covering the central area, thus corresponding to the R/hyper-calcified morphotype in the present study. b B and C morphotypes are distinguished by distal shield diameters > 4.5 or < 3.5 µm, respectively, with B/C being intermediate. O variants have varied distal shield diameters with an empty (open) central area (Hagino et al., 2011). B and B/C were not observed in Patagonia. The lightly calcified E. huxleyi in the dataset of von Dassow et al. (2018) included a continuum of characteristics from A to B and B/C or C.
SEM images taken at 20 000–25 000× magnification were used to categorize E. huxleyi cells in the different morphotypes according to the morphology of distal shield and the central plate of the coccoliths (following Young and Westbroek, 1991; Young et al., 2003; Hagino et al., 2011; von Dassow et al., 2018). Given high morphological similarities in the A morphotype coccoliths with those found by Young (1994) in Norwegian fjords, they were here classified as lightly, moderately, and robustly calcified, based on the morphology of distal shields and central plates (Fig. 2; Table 1). Moreover, two extremely heavily calcified A morphotypes were observed: the A-CC (with closed central area but distal shield elements mostly not fused) and the R/hyper-calcified (Table 1; Fig. 2). These two morphotypes are sometimes grouped as overcalcified (Cubillos et al., 2007; Saavedra-Pellitero et al., 2019). However, we have observed in culture that they remain distinct under different physiological stresses (e.g., Mella-Flores et al., 2018; von Dassow et al., 2018). This analysis assumes discontinuous traits that can be accurately assessed by qualitative analysis. A morphometric study supports this, where coccoliths of what we term the A-CC morphotype cluster well apart from other A morphotype coccoliths in the parameter relative tube length (that is, a small second mode in histograms) (Young et al., 2014). This assumption was also necessary as morphometric analyses in these characters are difficult to measure consistently in field samples and on attached coccoliths. Similarly, due to frequent overlap in coccolith distal shield lengths and coccosphere diameters observed in moderately and robustly calcified A forms (Table 1), we consolidate them into one group (hereafter jointly referred to as “moderately calcified A morphotype”) for statistical analyses. Moreover, we classified the malformed (teratological), incomplete, weakly dissolved, and collapsed coccoliths following the terminology and definitions of Young and Westbroek (1991) and Young (1994). Although these malformed and collapsed coccoliths were observed in < 9 % of the morphotype-A cells, it was almost always possible to classify those abnormal coccospheres into one of the abovementioned morphotypes (Fig. S2). SEM images were also used to measure the orthogonal coccosphere diameters and, when available, coccolith distal shield length (ImageJ software version 1.48 for Mac OS).
Biovolumes (µm3) of E. huxleyi, diatoms, dinoflagellates, and naked flagellates were estimated assuming recommended geometric shapes (Hillebrand et al., 1999; see Table S4). For E. huxleyi, a spherical geometric shape was assumed, and the average of the minimum and maximum diameters was used for biovolume calculations. Biovolume calculations were then converted to carbon biomass by using regression equations proposed by Menden-Deuer and Lessard (2000) for diatoms (pg C cell) and other protists (pg C cell). We assumed a constant cytoplasm diameter to be 60 % of the mean E. huxleyi coccosphere diameter (O'Brien et al., 2013), whereas cytoplasm volumes of 50 % and 78 % were used for diatoms and dinoflagellates, respectively (i.e., total cellular volume minus frustule or theca and vacuole volumes; Sicko-Goad et al., 1984). Absolute abundance data were standardized to cells per litre and multiplied by specific carbon contents per cell (pg cell−1) to derive total carbon biomass (Total C; µg C L−1). We used the biogenic silica concentration (µmol opal L−1) as a proxy of diatom biomass along the 2017 track, as samples for microscopy counts were not available. For this, the bSi concentration was converted into carbon units using the average net silicate-to-carbon ratio of 0.52 (mol mol) found by Brzezinski et al. (2003) in the Southern Ocean. There was a significant linear relationship between diatom carbon biomass estimated with microscopy/allometry and that calculated from bSi concentration in 2015 samples (R2=0.60, p<0.05, slope = 0.8; N=11), with an offset (16 µg C L−1) likely from other contributors to bSi (e.g., silicoflagellates, radiolarians) as well as the contribution of Minidiscus spp. (data not shown) not included in microscopy/allometric carbon estimation. The presence or absence of diatoms was confirmed qualitatively in 2017 by SEM images at 1500× magnification, and a semi-quantitative evaluation was made as follows: low (few valves), intermediate (at least one species with several valves or chains), and high (many species with several cells or chains). It should be kept in mind that there can be substantial variation in diatom carbon biomass estimated by microscopy vs. bSi, due to variability in diatom C:Si ratios (Leblanc et al., 2018).
2.3 Chemical analyses
Macronutrients, opal, total chlorophyll a (chl a), pH, and total alkalinity (AT) were determined as described in Torres et al. (2020). Full carbonate system parameters (including Ωcal) were estimated from pH, AT, salinity, temperature (25 ∘C as input and in situ temperature as output conditions), and pressure (0 dbar as input and depth as output conditions) using CO2Sys Excel macro spreadsheet version 2.1 (Pierrot et al., 2006) with Mehrbach set of solubility constants (Mehrbach et al., 1973) refitted by Dickson and Millero (1987). To extrapolate full carbonate parameters from pCO2 (onboard sensor) and salinity measurements where alkalinity samples were not directly available (due to mismatch in chemical and biological sampling along the IC-WSM 2015 track), the regression curve for the salinity–AT relationship (µmol kg salinity + 101 (R2=0.99, N=186; Torres et al., 2020) was used to derive AT estimated from salinity. It is important to note that this relationship has been stable for over a decade in Patagonia (Torres et al., 2011, 2020). pCO2 values delivered by the onboard sensor (underway sampling) correlated with pCO2 calculated from AT–pH pairs (discrete sampling) in the same 2015 samples (R2=0.56, p<0.001; N=17), with an overestimation of 6 µatm (2 %). The differences between measured and calculated pCO2 values are small compared to the high ranges in the variability of pCO2, salinity, AT, and pH and should not affect the objectives of the present study. Exceptionally, the calculated pCO2 values for SS were overestimated by up to 36 % concerning pCO2 measurements (comparing 15 readings from the sensor with three calculated values). This disagreement could be due to various local factors that increase the sensitivity of calculated pCO2 to AT (Abril et al., 2015) or pH uncertainties due to differences in salinity between buffers and samples. Therefore, it should be kept in mind that in the case of SS the uncertainties in the carbonate system could be more substantial. Finally, in order to calculate the ratio (in mol/µmol) of bicarbonate (HCO) to proton (H+), the HCO was divided by the antilog10 of –pH (total H+ scale).
2.4 Statistical analysis
All statistical analyses were performed in R software using packages freely available on the CRAN repository (R Core Team, 2019). As measurements for nitrate, phosphate, DSi, bSi, and chl a in 2015 were scattered and uncoupled from plankton sampling, these variables were used only descriptively and not included in the statistical analyses.
2.4.1 Environmental gradients
Physical–chemical data obtained from the surface at the discrete sample stations in both 2015 and 2017 were combined in a unique matrix and standardized by subtracting the mean and dividing by the standard deviation (Legendre and Legendre, 2012). We used the varclus function in the “Hmisc” package based on Spearman's correlation to detect redundant environmental variables (N=32). Temperature, salinity, pH, and Ωcal were selected as they are non-redundant based on Spearman's correlation <0.75 (Fig. S3), and they are easiest to interpret from a biological or cell physiological point of view. We also included CO2. It was moderately correlated with pH (Spearman correlation = 0.8) but represents the substrate for photosynthesis and is typically incorporated as a driving variable in ocean acidification studies. HCO may more directly impact sensitivity of coccolithophores in lab measurements (e.g., Kottmeier et al., 2016; Gafar et al., 2018), but it was redundant with the other variables. The selected standardized variables were then used in two separate cluster analyses to recognize groups of sampling stations with similar characteristics in 2015 (N=21) and 2017 (N=11). For that, Euclidean distance matrixes were first calculated based on selected standardized variables (vegdist function in the “vegan” package) and then included in hierarchical cluster analyses based on the Ward method using the hclust function in the basic “stats” package.
2.4.2 Testing for a relationship of Emiliania huxleyi vs. diatoms
To characterize the diatom species associated with the different E. huxleyi morphotypes and other coccolithophore species, we performed a non-metric multidimensional scaling (nMDS) using the metaMDS function in the vegan package, based on the log-transformed [ln (x+1)] coccolithophore and diatom abundances (cells L−1) in Patagonia (only 2015) and the other coastal and oceanic locations (von Dassow et al., 2018) (N=52). The function heatmap of the basic stats package was then used to plot the abundance of the coccolithophore and diatom species related to the clusters based on the nMDS scores of species/morphotypes and samples. As both the nMDS and OMI (see below) analyses suggested a clear separation between Patagonia fjords and the other coastal/oceanic areas, we used the IndVal analysis (Dufrene and Legendre, 1997) to identify indicator species for both areas, based on log-transformed abundances (indval function in “labdsv” package).
We aimed to assess how E. huxleyi and diatom biomasses were related to each other and with the environmental conditions throughout Patagonia fjords. However, the different methods used to estimate diatom biomass in both years precluded the use of E. huxleyi–diatom ratios. Moreover, the use of regression-based analyses was not recommended due to the absence of a linear relationship between E. huxleyi and the different physical–chemical variables. To overcome these limitations, we created three categorical variables for both E. huxleyi and diatom biomasses (low, intermediate, and high) based on their < 25th, 25–75th, and > 75th percentiles, respectively. We then performed a correspondence analysis (CA) using the function cca in the vegan package, based on the presence or absence of these new categoric variables in each sample (N=32), followed by fitting the standardize physical–chemical variables to the CA plot using the envfit function (10 000 permutations).
2.4.3 Niche analysis
We used the outlying mean index (OMI) analysis (Dolédec et al., 2000) to assess how the different physical–chemical variables (selected in Sect. 2.4.1) were associated with the realized niche of the different E. huxleyi morphotypes. The OMI index represents the marginality (i.e., niche position) and measures the distance between the average habitat conditions used by a given population and the average environmental conditions across the study area (represented by the point where the two multivariate axes intersect at zero). The tolerance (Tol) accounts for the dispersion of samples containing organisms of the population from the average environmental condition (i.e., niche breadth), whereas the residual tolerance (RTol) accounts for the proportion of the variability unexplained by the variables included in the analysis (Dolédec et al., 2000). Thereby, a species having a low OMI (species score close to zero, located in the centre of the multivariate space) and high Tol is likely one that utilizes a wider array of resources and maintains populations within a wider variety of conditions (i.e., generalist), when compared with the specialized and less resilient species with a more restricted realized niche associated with high OMI and low Tol that are expected to show lower Tol and may also be associated with lower OMI (Dolédec et al., 2000).
The OMI analysis was performed using the niche function in the “ade4” package (Dray and Dufour, 2007), simultaneously considering the data obtained for 2015 and 2017 (N=32). To compare the patterns observed in Patagonia to other localities in the southeastern Pacific, a complementary OMI analysis was performed, including records of coccolithophore assemblages and E. huxleyi morphotypes from nearby coastal and oceanic waters (published by von Dassow et al., 2018) in addition to the data used in the first analysis (N=64). A 1.84× correction factor (determined as informed in Sect. 2.2) was applied to these data, as coccolithophore counts from von Dassow et al. (2018) were obtained by the Utermöhl method. In both cases, data were arranged in two matrices, one containing the coccolithophore abundances and a second matrix with the standardized physical–chemical variables. Coccolithophore abundances were previously Hellinger-transformed (Legendre and Legendre, 2012). Since Hellinger transformation is obtained by the squared root of relative abundances, the potential biases from comparing data from both SEM and Utermöhl counts were minimized. The statistical significance of the morphotypes/species marginality was tested using the Monte Carlo method included in the ade4 package (10 000 permutations). The envfit function in the vegan package (Oksanen et al., 2007) was then used to fit the five environmental variables to the OMI scores (10 000 permutations).
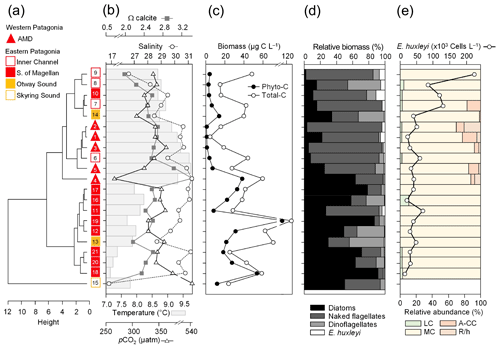
Figure 3Physical and chemical conditions, carbon biomass by microplankton and phytoplankton assemblages, and abundance and calcification level of E. huxleyi recorded in surface waters of southern Patagonia during the late austral spring 2015. (a) Hierarchical clustering on salinity, temperature, pH, pCO2, and Ω calcite surface values on 21 water samples collected for plankton analysis, (b) salinity, temperature, Ω calcite, and pCO2 levels. (c) Total carbon biomass by the nano- and micro-plankton assemblages (Total-C, all items between 5–200 µm in length) and phytoplankton assemblages (Phyto-C, diatoms + coccolithophores). (d) Relative carbon biomass by diatoms, naked flagellates, dinoflagellates, and E. huxleyi. (e) Total abundances of E. huxleyi and relative abundances of four E. huxleyi morphotypes. All samples were taken < 5 m in depth. Stations 5–7, 11–13, and 18–21 were conducted at night. LC: E. huxleyi lightly calcified A morphotype; MC: E. huxleyi moderately calcified A morphotype; A-CC: E. huxleyi A-CC morphotype; R/h: E. huxleyi R/hyper-calcified morphotype.
3.1 Late spring southern Patagonia 2015
The hierarchical clustering based on the surface values of the selected physical–chemical variables in the late austral spring 2015 (i.e., salinity, temperature, Ωcal, pH, and pCO2) showed a clear separation between the sampling station at the Skyring Sound (SS; St. 15) and the other localities (Fig. 3a). The other stations were grouped in two main clusters: one cluster composed of stations in the Archipelago Madre de Dios (AMD) and the inner channel (IC) and a second one composed mainly of stations in the western part of the Strait of Magellan (WSM). Samples from the Otway Sound (OS; Sts. 13–14) were distributed between the two clusters. The cluster separation seemed to be mainly related to temperature and salinity dissimilarities, while stations 4 and 15 differed from others by their relatively low pCO2–high Ωcal and high pCO2–low Ωcal conditions, respectively (Fig. 3b). Surface salinity ranged from > 29 in the AMD and southernmost WSM stations to as low as 17 in the SS (St. 15), with intermediate values throughout the IC and in the OS (range: 26–29; Fig. 3a–b). A north–south gradient of decreasing surface temperature was recorded from 9.0–10.0 ∘C around the AMD zone to 7.1 ∘C near Helado Sound (Sts. 17–18; Figs. 3a–b, S4a). Surface waters were mostly undersaturated relative to atmospheric pCO2 (< 400 µatm) with minimum values at St. 4 in the AMD (241 µatm). CO2 oversaturation was only observed at the SS (542 µatm). Similar to salinity, Ωcal varied widely, ranging from highly oversaturated conditions in the AMD zone (Ωcal range: 2.5–3.6, pH in situ range: 8.03–8.21), intermediate levels in the interior WSM (Ωcal range: 2.4–2.6; pH range: 8.04–8.07), lower levels in the southern IC zone (Ωcal 2.0–2.2, pH ∼ 8.0), and a subsaturated extreme reached at the SS sampled site (Ωcal 0.5, pH 7.73, Figs. 3a–b, S5a). Moderate to low DSi (< 6 µm) and nitrate (NO < 6 µm) were recorded in southern Patagonia, dropping to a minimum in the southern IC zone (Table 2, Fig. S6).
Table 2Physical and chemical conditions and photosynthetic and silicified biomass proxies in the surface waters (< 5 m) of southern Patagonia fjords. Mean, standard deviation (SD), and range of each variable and number of samples for late spring 2015 and early spring 2017 are shown. Only values matching planktonic samples discussed in the text are included, except for chlorophyll a (chl a), opal, nitrate, and silicate 2015 for which values are between 3–28 km decoupled from biological sampling. The mean and SD do not include the Skyring Sound 2015 station as it shows extreme values for all variables (see Table S1). However, the values from that sample are shown in parentheses for comparison. NA: no available data.
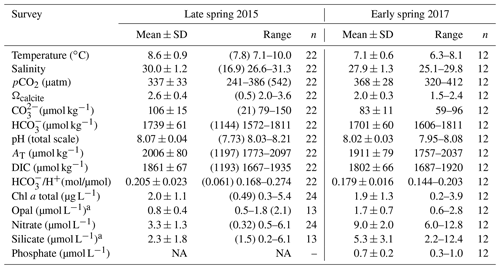
a The 2015 range is from the Archipelago Madre de Dios to the inner channel zone along 50.4–52.6∘ S.
The AMD and IC zones showed relatively low phytoplankton biomasses (< 20 µg C L−1) dominated by naked flagellates (Fig. 3c–d), whereas diatoms were associated with higher phytoplankton biomasses (> 40 µg C L−1) in Eleuterio Channel (St. 4; mostly chains of Chaetoceros spp.) and the WSM (Sts. 12, 16–21; mostly chains of Leptocylindrus spp., Chaetoceros spp., and Thalassiosira spp.). The contribution of dinoflagellates to biomass was highest in IC St. 8, OS Sts. 13–14, and WSM Sts. 12 and 16. Coccolithophores only accounted for 0.2 %–12.8 % of total C biomass (0.1–4.0 µg C L−1), reaching > 6 % only in the southern IC (Sts. 8–10) where diatom biomasses were among the lowest observed. E. huxleyi dominated the coccolithophore assemblages in all samples (> 98 %), with a few Syracosphaera spp. coccospheres (mostly collapsed) found at the AMD (Table S2). E. huxleyi abundances (Fig. 3e) ranged from 0 to 2.76×105 cells L−1, being most abundant in southern IC (> 1.03 × 105 cells L−1 in Sts. 7–10) and only absent from SS (St. 15), which was the only station where conditions were undersaturated with respect to calcite (Ωcal<1). Emiliania huxleyi populations were mostly composed of the moderate A morphotype (which also included cells of the robustly calcified A morphotype; see Methods) (Fig. 3e, Table 3). Few lightly calcified A cells were observed. Among all samples, only eight total O and C and no B or B/C morphotype cells were detected. The heavily A-CC and R/hyper-calcified morphotypes were restricted to the AMD zone.
Table 3Relative percentages of E. huxleyi A morphotypes recorded throughout southern Patagonia fjords. Mean, standard deviation (SD), and maximum and minimum percentages of five E. huxleyi morphotypes recorded in inner surface waters of southern Patagonia (PAT; n=883 cells counted in 23 samples) and down to 50 m in the Archipelago Madre de Dios western zone (AMD; n=1012 cells counted in 27 samples) during the late austral spring 2015 and early spring 2017.

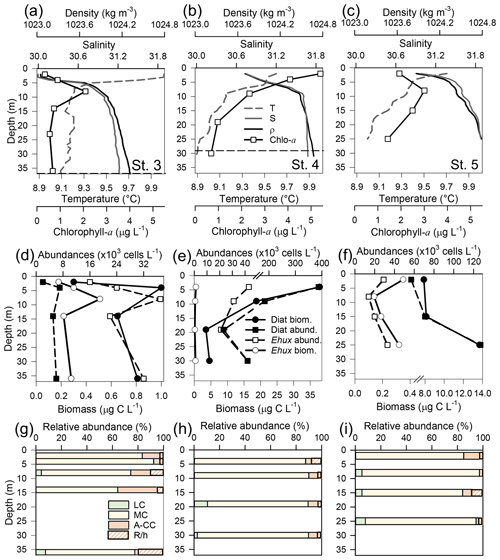
Figure 4Physical, chemical, and biological vertical profiles recorded in the Archipelago Madre de Dios during late austral spring 2015. Temperature, salinity, density, and total chlorophyll a (a–c); abundance and total carbon biomass of E. huxleyi (Ehux) and diatoms (Diat; d–f); and relative abundance of four E. huxleyi morphotypes (g–i) in the W-AMD (St. 3 , a, d, g), between (St. 4, b, e, h), and E-AMD zones (St. 5, c, f, i). Dotted lines in (a–b) indicate depths of 1 % PAR penetration (St. 5 was conducted at night). Morphotype abbreviations as in Fig. 3. See Fig. S7 for additional variables.
Three vertical profiles were performed in the AMD estuarine zone (Fig. 4): one at the western limestone AMD basin (St. 3), one between the western and eastern AMD basins (St. 4), and one at the easternmost basin (St. 5, Figs. 4, S1). All samples were taken within the euphotic zone (1 % PAR), which extended down to 36 and 30 m at Sts. 3 and 4, respectively (St. 5 was conducted at night, Fig. S7a–c). All three sites were sharply stratified in the upper 10 m, with pycnoclines around 5 m. Temperature decreased while salinity increased with depth (Fig. 4a–c). At station 3, Ωcal varied little with depth, remaining in the range 2.5–2.7 (Fig. S7g) even across the shallow pycnocline. At station 4, Ωcal showed the highest values and highest range; AT, pCO2, and HCO increased sharply with depth in the upper 10 m, while Ωcal decreased from over 3.6 to less than 2.9 at 9 m and 2.7 at 29 m (Fig. S7h). Finally, at station 5, Ωcal decreased steadily from 3 at the surface to 2.4 at 25 m (Fig. S7i). Based on total chl-a levels and fluorescence signals, most photosynthetic biomass occurred in the upper 15 m of the water column at Sts. 3–4, peaking at the surface at St. 4 (5.4 µg L−1). However, in station 5, total chl-a levels and fluorescence signals were more constant with depth, dropping proportionally less by 25 m from maximal values, when compared to the other stations. The “silicate” estuarine zone (Sts. 4–5) showed higher photosynthetic biomass and bSi than the limestone site (Figs. 4a–c, S7d–f). Emiliania huxleyi mostly occurred in the well-illuminated upper layer, most notably in the western limestone AMD waters, where diatom abundance and biomass were low compared to the communities recorded at silicate sites (Fig. 4d–f). Although the moderate A morphotype was predominant at all depths in the three stations (Fig. 4g–i), a higher proportion of the A-CC morphotype (up to 31 % relative abundance) was observed in the upper 15 m of the limestone western AMD when compared to the other two stations. The lightly calcified and R/hyper-calcified morphotypes were present in a lower proportion (<10 %).
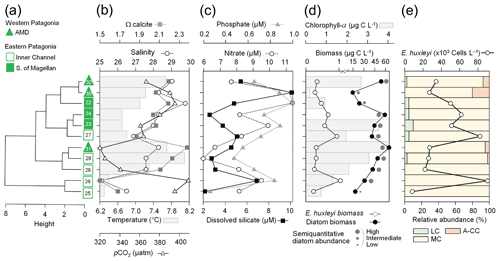
Figure 5Physical, chemical, and nutrient conditions, chlorophyll-a levels, carbon biomass by E. huxleyi and diatoms, and abundances and calcification level of E. huxleyi recorded in surface waters of southern Patagonia during early austral spring 2017. (a) Hierarchical clustering on temperature, salinity, pH, pCO2, and Ω calcite surface values on 11 water samples collected for plankton analysis; (b) salinity, temperature, Ω calcite, and pCO2 levels; (c) nitrate, dissolved silicate, and phosphate levels; (d) total chlorophyll a and total carbon biomass by E. huxleyi and diatoms and semi-quantitative estimation of diatom abundances (SEM); and (e) total abundances of E. huxleyi and relative abundances of three E. huxleyi morphotypes. All samples were taken < 5 m in depth. Stations 25–26 and 30 were conducted at night. Morphotype abbreviations as in Fig. 3.
3.2 Early spring southern Patagonia 2017
The hierarchical clustering based on the physical–chemical conditions in early austral spring 2017 indicated a separation between the WSM and the IC, whereas the three stations in the AMD were distributed between the two clusters (Fig. 5a). Surface salinity ranged from 25 to 30 along the south–north track, with saltier waters (> 28) around the AMD (Sts. 30–32) and southward in the WSM zone (Sts. 22–24) and fresher waters in the southern IC (< 27 at Sts. 25–27) (Fig. 5b). Surface temperatures ranged from a minimum of 6.3 ∘C in the southern IC to > 7.7 ∘C in the AMD and northern IC (max. 8.1 ∘C at St. 29, Figs. 5a–b, S4b). Surface waters were undersaturated with respect to pCO2 at all stations (range: 320–396 µatm) except for station 26 (412 µatm). Lower Ωcal levels prevailed in the southern IC (range: 1.5–1.9) with higher Ωcal in the AMD zone (range: 2.1–2.4; Figs. 5b, S5b). Nitrate, silicate, and phosphate concentrations (Fig. 5c) were mostly in the range 6.0–8.3, 2.2–5.4, and 0.3–0.7 µm, respectively, with the highest levels of nitrate (9.5–12.8 µm) and phosphate (0.8–1.0 µm) at Sts. 22–23, 26, and 30, whereas stations 30 and 26 registered the highest DSi (12 and 7 µm, Fig. 5c, Table 2). Higher photosynthetic biomass (chl a>2.7 µg L−1) was recorded around the eastern AMD (St. 29, 31–32) and WSM station 23, while the western AMD (St. 30), southern IC (Sts. 25–26), and WSM (St. 22) yielded the lowest measured biomass (chl a<1.3 µg L−1; Fig. 5d). Variation in chl a reflected variation in diatom biomass (Fig. 5d).
The dominant coccolithophore taxon during early spring 2017 was again E. huxleyi (> 96 %). Abundances ranged from 1.69×104 to 9.06×104 cells L−1 (Fig. 5e). The E. huxleyi carbon biomass averaged 0.5±0.3 µg C L−1 (in 11 samples), reaching both maximal and minimal values (0.2 and 1 µg C L−1, respectively) in the southern IC (Sts. 25–27). In contrast, the opal-derived diatom carbon biomass averaged 40±17 µg C L−1, with lower values (< 18 µg C L−1) in the AMD at St. 30 and IC at St. 25 (Fig. 5d). While fixed samples for standard microplankton analysis were not available, large chains of Skeletonema spp., Thalassiosira spp., and Chaetoceros spp. were noted as frequent in samples observed by SEM (Fig. S8) and were likely significant contributors to opal. Similar to the 2015 survey, the moderate A morphotype dominated the E. huxleyi assemblages along the 2017 track (Fig. 5e). Cells of the lightly calcified A morphotype were sporadically observed, whereas the highly calcified A-CC and R/hyper-calcified morphotypes were again restricted to the AMD zone (Fig. 6, Table S1; note the low abundances of the R/hyper-calcified morphotype were only detected at other depths, so they do not appear in Fig. 5e).
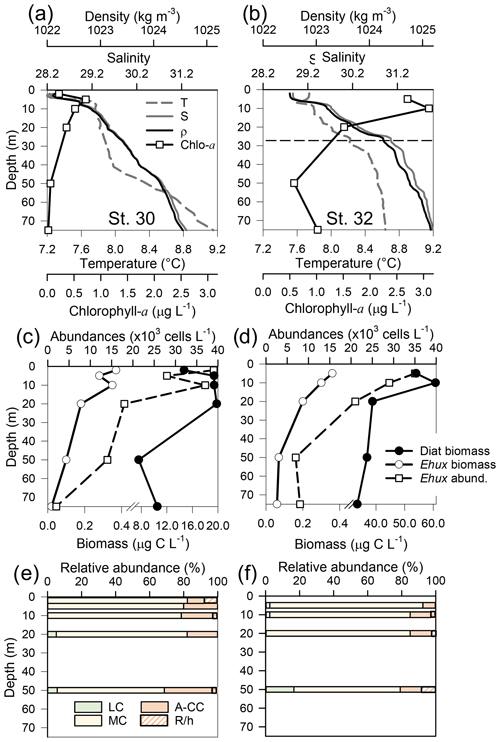
Figure 6Physical, chemical, and biological vertical profiles recorded in the Archipelago Madre de Dios during early austral spring 2017. Temperature, salinity, density, and total chlorophyll a (a–b), abundance of E. huxleyi and total carbon biomass of E. huxleyi (Ehux) and diatoms (Diat; c–d), and relative abundances of four E. huxleyi morphotypes (e–f) in the W-AMD (St. 30 left) and the E-AMD (St. 32 right). Dotted line in (b) indicates depth of 1 % PAR penetration (St. 30 was conducted at night). Morphotype abbreviations as in Fig. 3. See Fig. S9 for additional variables.
Two CTD profiles were performed in the AMD zone: one in the western limestone AMD basin (St. 30) and another profile southwest of Escribano Island at the eastern silicate AMD basin (St. 32) (Fig. 6a, b). The profiles covered the euphotic zone (down to 27 m in St. 32; St. 30 was conducted at night) as well as subsurface layers (25–75 m depth). In both stations, temperature and salinity increased with depth, with maximum density stratification between 5–10 m. In the western AMD profile, Ωcal was low at the surface (2.1) due to the lowest salinity, but rose to a maximum at 5 m due to a minimum in pCO2 and increasing salinity, whereas below 20 m, pCO2 rose and Ωcal dropped (Fig. S9e). At the eastern AMD site, in contrast, Ωcal increased with depth despite increasing pCO2 (Fig. S9f). At both stations, photosynthetic biomass was mainly confined to the upper 25 m of the water column, with chl-a peaks at 5 and 10 m (0.7 and 3.1 µg L−1, respectively) and dropping close to zero below 40 m in the western AMD, while remaining near 1 µg L−1 even at depths ≥50 m in the eastern AMD (Figs. 6a–b, S9a–b). The eastern AMD zone exhibited higher chl a and bSi when compared to the western AMD, despite no depletion of phosphate, nitrate, and DSi being observed at either site (Figs. 6a–b, S9a–d).
At both sites, E. huxleyi dominated the coccolithophore assemblages across all depths, with another six coccolithophore species observed in subsurface AMD waters (Table S3). E. huxleyi and diatom abundances were highest in the surface at both sites (Fig. 6c–d). However, reflecting the chl-a profile, estimated diatom biomass remained relatively high at depth compared to surface values (dropping by only about 40 %), and E. huxleyi abundance and biomass also dropped less with depth in the eastern AMD compared to the western AMD (Fig. 6c–d). In both stations, the composition of E. huxleyi morphotypes was similar at all depths, characterized by the predominance of the moderately calcified morphotype followed by highly calcified A-CC (Fig. 6e–f). The lightly calcified and R/hyper-calcified morphotypes were either undetected or represented a minor fraction of coccolithophore assemblages.
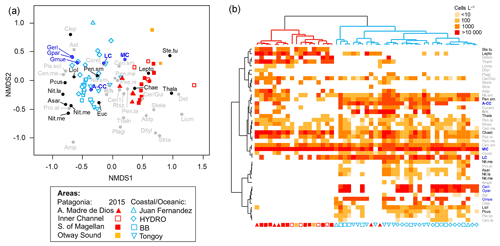
Figure 7(a) Non-metric multidimensional scaling (nMDS) based on coccolithophore and diatom abundances attained in southern Patagonia fjords during late spring 2015 (this study) and other coastal/oceanic areas (data from von Dassow et al., 2018). (b) Heatmap showing abundances of coccolithophore and diatom species used in the nMDS. The horizontal dendrogram (based on the nMDS sample scores) shows a clear separation between Patagonia fjords (red cluster) and coastal/oceanic (blue cluster) areas, whereas the vertical dendrogram (based on the nMDS species scores) indicates the separation of species in two main clusters. Black and blue species labels depict species with significant values in the IndVal analysis. A-CC: Emiliania huxleyi A-CC morphotype; Amphi: Amphiprora spp.; Asar: Asteromphalus sarcophagus; Aster: Asteromphalus spp.; Astp: Asterionellopsis spp.; Cen.la: centric diatoms > 100 µm diameter; Cen.me: centric diatoms 40–100 µm diameter; Cen.sm: centric diatoms < 40 µm diameter; Chae: Chaetoceros spp.; Clep: Calcidiscus leptoporus; Coret: Corethron spp.; Cer/Gui: Cerataulina spp. + Guinardia spp.; Deton: Detonula spp.; Dityl: Ditylum spp.; Eucam: Eucampia spp.; Geri: Gephyrocapsa ericsonii; Gmue: Gephyrocapsa muellerae; Gpar: Gephyrocapsa parvula; LC: Emiliania huxleyi lightly calcified morphotype; Lepto: Leptocylindrus spp.; Licmo: Licmophora spp.; Liol: Lioloma spp.; MC: Emiliania huxleyi moderately calcified morphotype; Nit.la: Nitzschia spp. large > 100 µm length; Nit.me: Nitzschia spp. medium 100–50 µm length; Nit.re: Nitzschia reversa; Nit.sm: Nitzschia spp. < 50 µm length; Pcus: Pseudo-nitzschia cuspidata; Pen.la: pennate diatoms > 100 µm length; Pen.me: pennate diatoms 50–100 µm length; Pen.sm: pennate diatoms < 50 µm length; Pla.so: Planktoniella sol; Plagi: Plagiogrammopsis spp.; Pro.al: Proboscia alata; Pse.ni: Pseudo-nitzschia spp.; R/h: Emiliania huxleyi R/hyper-calcified morphotype; Rhizo: Rhizosolenia spp.; Skele: Skeletonema spp.; Ste.tu: Stephanopyxis turris; Stria: Striatella spp.; Thala: Thalassiosira spp.; Thaln: Thalassionema spp.
3.3 Emiliania huxleyi abundance vs. diatoms
The nMDS depicted a clear separation between the Patagonia fjords and the oceanic/coastal areas regarding the composition of coccolithophorid and diatom assemblages (Fig. 7). The IndVal analysis (Table S5) identified only the E. huxleyi moderately calcified morphotype as an indicator of the fjord locations, along with the diatoms Thalassiosira spp., Stephanopyxis turris, Leptocylindrus spp., and Chaetoceros spp. The coastal/oceanic locations were more characterized by the lightly calcified and A-CC morphotypes and the other coccolithophore species (i.e., G. ericsonii, G. muellerae, G. parvula), as well as the diatoms Lioloma spp., pennate diatoms (< 50 µm length), Nitzschia spp., Pseudo-nitzschia cuspidata, and Asteromphalus sarcophagus.
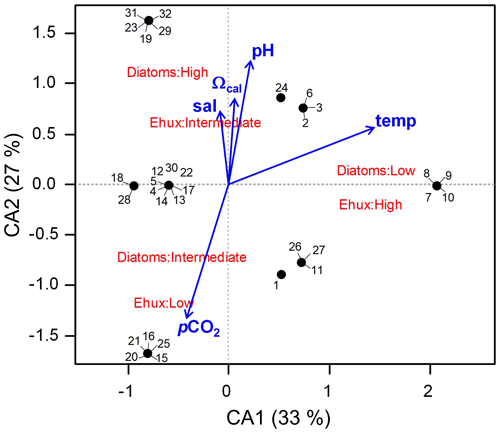
Figure 8Correspondence analysis (CA) assessing the relationship between E. huxleyi and diatom biomasses converted to categoric values (i.e., low, intermediate, and high biomasses) in Patagonia fjords during late spring 2015 and early spring 2017. The envit function of the vegan package (R software) was used to fit the environmental variables to the CA plot (no variable was significant; p>0.05).
The two first axes of the CA accounted for 60 % of the total explained variability and indicated that the highest E. huxleyi and low diatom biomasses were associated with increasing temperatures (Fig. 8). Intermediate E. huxleyi biomasses were associated with high diatom biomasses and increasing gradients of salinity, pH, and Ωcal, whereas low E. huxleyi biomasses were associated with intermediate diatom biomasses and increasing pCO2. However, none of the considered environmental variables had a significant fit in the envfit test.
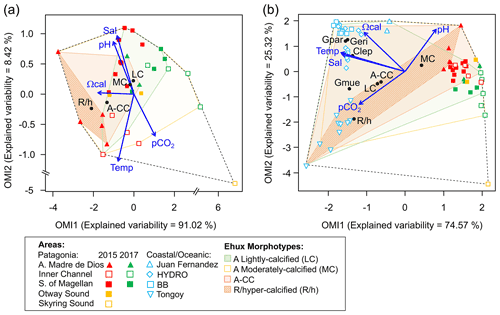
Figure 9Outlying mean index (OMI) niche analysis by E. huxleyi (Ehux) morphotypes populating the surface waters of southern Patagonia and complemented with coccolithophores and Ehux morphotypes from nearby coastal and oceanic waters constrained by environmental conditions. (a) Biplot representing the realized niche of four E. huxleyi morphotypes during late austral spring 2015 and early spring 2017 in Patagonia, where black circles indicate the mean habitat condition used by each morphotype (niche position) and polygons delimit their respective niche breadth (i.e., tolerance). Blue vectors represent the gradients of environmental variables. (b) Realized niches of Ehux morphotypes and other coccolithophore species in Patagonia fjords (this study) and nearby coastal/oceanic waters (data from von Dassow et al., 2018). Polygons of other coccolithophore species in (b) are not shown for simplicity. Temp: temperature; Sal: salinity; LC: E. huxleyi lightly calcified A morphotype; MC: E. huxleyi moderately calcified A morphotype; A-CC: E. huxleyi A-CC morphotype; R/h: E. huxleyi R/hyper-calcified morphotype; Gpar: Gephyrocapsa parvula; Geri: Gephyrocapsa ericsonii; Gmue: Gephyrocapsa muellerae; Clep: Calcidiscus leptoporus.
3.4 Niche analysis of Emiliania huxleyi morphotype responses to environmental conditions
The OMI analysis depicted differences in the realized niches of the E. huxleyi morphotypes throughout Patagonian fjords in 2015 and 2017 (Fig. 9a, Table S6). The OMI plot showed station 15 from 2015 as an outlier, characterized by extremely low salinity and high pCO2. The OMI axis 1 (91.02 % of explained variability) was negatively related to Ωcal, whereas the OMI axis 2 (8.42 % of explained variability) was positively related to salinity and pH and negatively related to temperature and pCO2. The envfit test indicated that all variables had a significant fit (R2>0.88, p<0.01; Table S7). Only the moderate A and R/hyper-calcified morphotypes showed significant OMIs (p<0.05, Table S6). The moderate A morphotype was the most generalist (OMI = 0.07, Tol = 1.23), observed in all samples (except St. 15 in 2015). The R/hyper-calcified morphotype, observed exclusively in the AMD zone, was the most specialized morphotype (OMI = 4.77, Tol = 0.75). The A-CC morphotype (OMI = 1.43, Tol = 1.68), observed in the AMD and northern IC, showed intermediate habitat preferences (Fig. 9a), but the OMI for this morphotype did not meet the threshold for significance (p=0.060). The RTol for the R/hyper-calcified morphotype was 12 % (Table S6), indicating that most variability in its realized niche was accounted for by the environmental variables included in the analysis.
The extended domain OMI analysis (Fig. 9b, Table S8) indicated a clear separation between the Patagonian fjords and coastal and oceanic waters off central and northern Chile and Peru. The OMI axis 1 (74.57 % of explained variability) was negatively related to temperature, salinity, and Ωcal, whereas the OMI axis 2 (25.32 % of explained variability) was positively related to pH and negatively related to pCO2. The envfit test indicated that all variables had a significant fit (R2>0.88, p<0.01; Table S9). All coccolithophore species and E. huxleyi morphotypes showed significant OMIs (p < 0.05, Table S8). The lightly calcified, moderately calcified, and A-CC morphotypes, characterized as the most generalist, showed similar realized niches (OMI = 0.25-0.75, Tol = 2.85–2.96), whereas the R/hyper-calcified form was again the most specific of the E. huxleyi morphotypes (OMI = 5.25, Tol = 1.97) and restricted to the coastal upwelling and AMD zones (Fig. 9b, Table S8). Regarding the other coccolithophore species, G. muellerae was common to both coastal and oceanic areas but still showed a higher degree of specialization (Tol = 1.03) than the E. huxleyi morphotypes, whereas C. leptoporus, G. ericsonii, and G. parvula showed a preference for oceanic conditions with low Tol values (0.15–0.38; Table S8). The R/hyper-calcified morphotype, G. ericsonii, and G. parvula showed very low RTol (< 4.6 %), indicating that most of their realized-niche variation was accounted for by environmental variables in the analysis (Table S8).
4.1 Patagonian coccolithophore communities dominated by E. huxleyi
Emiliania huxleyi was the only coccolithophore widely distributed along the fjords and inner channels of southern Patagonia and always represented > 96 % of total coccolithophore abundance and > 89 % of coccolithophore biomass, during both early and late spring. The low diversity of coccolithophore assemblages, dominated by E. huxleyi, is a common spring–summer feature in both the Patagonian and Norwegian fjord systems. In the case of southern Patagonia, the neighbouring Pacific has higher diversity (Beaufort et al., 2008; Menschel et al., 2016; von Dassow et al., 2018), but the Southern Ocean assemblages also show low diversity dominated by E. huxleyi (Cubillos et al., 2007; Saavedra-Pellitero et al., 2014; Charalampopoulou et al., 2016; Saavedra-Pellitero et al., 2019). The low diversity in southern Patagonian waters thus may partly reflect this latitudinal trend, although more detailed seasonal studies, including sampling along vertical profiles, might reveal significant additional coccolithophore diversity in the Patagonian and Norwegian fjords.
Table 4Comparison of E. huxleyi standing stocks and morphotypes recorded in northern and Patagonia fjord systems and nearby coastal/ocean locations. Temperature and salinity ranges are shown. Only data < 10 m in depth were included. ESP: eastern South Pacific; SO: Southern Ocean; APS: Atlantic Patagonian Shelf; SU: summer; SP: spring; WI: winter; AU: autumn; NA: no available data.
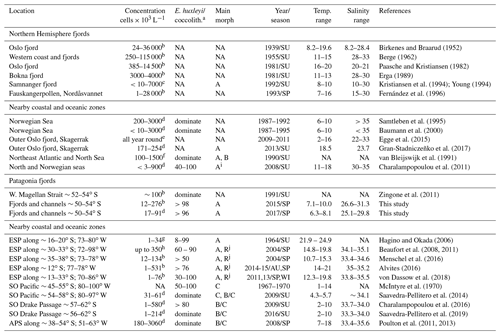
a E. huxleyi as a component of larger coccolithophore assemblages. When information about other coccolithophores was provided, the percentage abundance of E. huxleyi is given or if it was noted that E. huxleyi was dominant. “NA” means no information was provided for the parameter. b Estimated through sedimentation chamber and inverted microscopy. c Estimated by Palmer-Maloney chamber and inverted microscopy. d Estimated through filtration and scanning electron microscopy analysis. e Estimated by high-throughput sequencing and operational taxonomic unit (OTU) analysis, they found E. huxleyi throughout the year but concentrated between summer–autumn. f Cultured strains were identified at morphotype level by using immunofluorescence assay, and abundances were estimated through scanning electron microscopy analysis. g Estimated through filtration and cross-polarized microscope. h Estimated using an automated coccosphere recognition software. i The “characteristic” E. huxleyi morphotype showed in Fig. 4. j The R/hyper-calcified morphotype dominating the E. huxleyi assemblages in neritic zones.
4.2 Abundance of E. huxleyi in Patagonia compared to nearby oceans
During early and late spring, standing stocks of E. huxleyi in the Patagonian fjords and inner seas were moderate compared with those documented in nearby coastal and oceanic regions and within the range of background stocks reported in the Norwegian fjords and North Sea (Table 4 and references therein). The high E. huxleyi abundances typical of spring blooms in the Norwegian fjords were not observed in either early or late spring in the present study despite the similar temperature, salinity, and Ωcal conditions in both fjord systems. No E. huxleyi blooms have been reported in Patagonia fjords, although this might be due to limited observations and methodological issues. For example, many phytoplankton studies in the area (e.g., Alves-de-Souza et al., 2008; González et al., 2013) as well as standard phytoplankton monitoring in the zone (Vivanco and Seguel, 2009) often rely on samples fixed with Lugol's solution acid, which would not preserve coccoliths, or the studies have only focused on larger phytoplankton size classes (e.g., Paredes et al., 2014). Shallow water depth and frequent cloud cover limit satellite observations of particulate inorganic carbon (PIC) within the Patagonian shelf and fjords, but moderate coccolithophore blooms (of lower intensity compared to the North Sea) may occur later in the summer in the Pacific sector offshore of the latitudes sampled here (Hopkins et al., 2019).
4.3 Variation in E. huxleyi with environmental factors
It has been previously proposed that the realized niche of E. huxleyi is partly defined by physical and chemical conditions unfavourable to large diatoms (Tyrrell and Merico, 2004; Smith et al., 2017). During late spring the Patagonia fjords, E. huxleyi reached higher abundances in the southern IC when the temperature was above 8 ∘C and macronutrients and larger diatoms were the lowest, consistent with the pattern previously reported more generally for nano-phytoplankton based on size-fractionated chl a for this geographic area (e.g., Cuevas et al., 2019). However, the CA showed that the lowest levels of E. huxleyi were associated with intermediate levels of larger diatoms, and intermediate levels of E. huxleyi were associated with the highest levels of larger diatoms, suggesting a unimodal relationship between these two planktonic groups, possibly affected by factors not assessed in this study, such as nutrient supply and mixed-layer depth (Margalef, 1978; Cermeño et al., 2011), or predation (Nejstgaard et al., 1997).
The Ωcal – the saturation state of calcite, a parameter often assumed to constrain calcification (e.g., Zondervan et al., 2001; Kleypas et al., 2006; but see Cyronak et al., 2016) – was subject to large spatial variations in surface waters, from relatively high Ωcal levels in the AMD zone (range: 2.1–3.6), to moderate Ωcal in the interior WSM, low Ωcal in the southern IC (range: 1.5-2.2), and subsaturation in the SS (0.5). The range of surface Ωcal recorded along southern Patagonia was comparable to that reported for the Norwegian seas (Jones et al., 2019). Whereas the highest Ωcal values observed at the AMD were not as high as those observed in the global ocean (Takahashi et al., 2014), the lower values at the southern IC were comparable to values reported previously (range: 1.8–2.8) from high-CO2-upwelling conditions in central and northern Chile (Beaufort et al., 2008, 2011; von Dassow et al., 2018). While low surface Ωcal at coastal waters of northern and central Chile are related to the upwelling of high-pCO2–DIC subsurface waters, the freshening (and associated drop in DIC, salinity, and Ca2+ caused by dilution) and latitudinal–seasonal cooling (enhancing CO2 solubility) have major roles in lowering CO and Ωcal in southern Patagonia. These contrasting systems offered the possibility to observe whether the ecological trends related to low Ωcal depend on context.
4.4 Comparison of E. huxleyi morphotypes in Patagonia to nearby oceans and Norwegian fjords
There was some variability in the vertical distribution of the E. huxleyi morphotypes in the water column. The lightly calcified coccoliths appeared associated with subsurface waters in both seasons sampled at the locations, so they might be associated with intrusion of these waters. However, the samples within the euphotic zone were generally similar to each other within a given sample station. Thus, for the purposes of the questions in this study, the use of surface samples to describe morphotype distributions is expected to be reasonable, but use of vertical profiles might have permitted a higher ability to explain variability in the OMI analysis.
The E. huxleyi populations in the Patagonian fjords were morphologically distinct from surrounding coastal or open ocean populations in the eastern South Pacific, the Southern Ocean, and the Atlantic. The Atlantic Patagonian Shelf E. huxleyi populations are reported to be dominated by B/C morphotypes (Poulton et al., 2011, 2013). Southern open ocean populations of E. huxleyi are dominated by B morphotypes (including the B, B/C, C, and O types; Saavedra-Pellitero et al., 2014; Saavedra-Pellitero et al., 2019), and A morphotypes were reported to represent only a small fraction. However, C and O morphotypes were very rare in Patagonian inland waters, and B and B/C morphotypes were undetected. Although the moderately calcified and robustly calcified A morphotypes have also been shown to be present in eastern South Pacific coastal and open ocean waters (von Dassow et al., 2018), the dominance of these A morphotypes was particular to Patagonian interior waters. This conclusion was supported by the IndVal analysis (Table S5), where moderately calcified and robustly calcified A morphotypes were consolidated for final statistical analyses as they are not easily distinguished by objective morphological characters and were present in all samples, and preliminary analysis revealed completely overlapping realized niches. Both the moderately calcified and robustly calcified A morphotypes are also observed as dominant in the Norwegian fjords (Table 4) (Young, 1994). The lightly calcified A morphotype was rare and did not show any clear pattern in its distribution. The A-CC morphotype has been associated with coastal upwelling zones in the Atlantic (Giraudeau et al., 1993; Smith et al., 2012; Henderiks et al., 2012) but not reported from the Norwegian fjords or the Southern Ocean. In both early and late spring, R/hyper-calcified and A-CC E. huxleyi appeared only at the Pacific border of southern Patagonia (AMD zone). Thus, E. huxleyi populations of both Patagonian fjords and Norwegian fjords share a similar morphotype composition.
4.5 Niche analysis of E. huxleyi morphotypes related to carbonate chemistry conditions
The broader niche breadth by the moderately calcified A morphotype contrasted with the marginal niche of the R/hyper-calcified forms in Patagonia (Fig. 9a). The lightly calcified A morphotype also showed a low tolerance (more specialist), but this was not statistically significant. In order to extend the realized niches derived in Patagonia, we complemented the OMI analysis with a sample set of nearby oceanic and coastal sites (data from von Dassow et al., 2018), in some of which the moderately calcified A morphotype, unlike in Patagonia, was less abundant than other E. huxleyi morphotypes and coccolithophore species. According to OMI analysis, the niche differentiation along Patagonia is mostly driven by the pH–Ωcal conditions, but temperature and salinity conditions also become important. In this extended domain, both the moderately calcified A morphotype and the A-CC morphotype appeared to be generalists, with high Tol values (Fig. 9b). The lightly calcified morphotype also appeared to be a generalist in the extended domain. However, we caution that while the lightly calcified E. huxleyi was almost exclusively the lightly calcified A morphotype in Patagonia, there was a continuum of lightly calcified A, B, and B/C morphotypes (and some lightly calcified cells were difficult to classify among these types) in some of the coastal and oceanic sites. Proper differentiation between B, B/C, and C based on coccolith length would require strict morphometrics, which we did not perform due to the difficulty in accurate measurements on full coccospheres of less common morphotypes, especially in low-abundance populations (as coccospheres may lack coccoliths in a correct orientation for accurate measurement). Thus the generalist behaviour of lightly calcified morphotypes in the OMI analysis that combined fjord, coastal, and open ocean sites is likely an artefact. We suspect that lightly calcified A, B, B/C, and C morphotypes might actually each exhibit specialist behaviours in distinct but overlapping niches. In fact, a laboratory study reported that B/C morphotype strains only calcified substantially in a relatively narrow range of carbonate conditions (Müller et al., 2015). In contrast, the very distinct R/hyper-calcified morphotype exhibited restricted preferences in terms of Ωcal, temperature, and salinity but a broad niche in terms of CO2 and pH (Fig. 9b).
The R/hyper-calcified morphotype, in which there is both fusion of distal shield elements and closure or partial closure by overcalcification of the central area, has so far only been reported as prevalent in the high-CO2 and low-pH upwelling zone of the eastern South Pacific (Beaufort et al., 2011; Alvites, 2016; von Dassow et al., 2018), although it has been seen (and reported as rare) in both Australian waters (Cubillos et al., 2007) and the Drake Passage (Saavedra-Pellitero et al., 2019). Experimental findings that the R/hyper-calcified morphotype did not perform better than the moderately calcified A morphotype under high CO2/low pH/low Ωcal (von Dassow et al., 2018) might be explained by the OMI analysis suggesting a possible narrow unimodal response to Ωcal, which would not have been detected in the experiments of von Dassow et al. (2018), where Ωcal values of 1.4 vs. 3.3 were tested in the lab. The studies of Langer et al. (2009) and Müller et al. (2015) did find that R morphotype strains did seem more resistant to high CO2 and low pH than other A morphotypes, either in growth rate or in PIC production. Those studies used either 4-fold higher light levels (Langer et al., 2009) or continuous light (Müller et al., 2015), and low light has been shown to increase the sensitivity to OA, specifically of an R morphotype strain (Rokitta and Rost, 2012), highlighting that the R/hyper-calcified morphotype might be selected by interactions with other variables. Alternatively, the R/hyper-calcified morphotype might be selected by an unidentified condition particular to the southeastern Pacific that correlates with the Ωcal, temperature, and salinity of its realized niche.
The present study shows that the OMI analysis can be useful for identifying how parameters may determine the realized niches of both species and genetically determined phenotypic variants within a species. For example, in the extended domain (Fig. 9b), Ωcal, temperature, and salinity were important in defining the narrowness vs. breadth of niches among the E. huxleyi A morphotype, E. huxleyi R/hypercalcified morphotype, and closely related Gephyrocapsa species, while the habitat centres (niche positions) of the different E. huxleyi morphotypes lined up approximately on a gradient of pH vs. CO2. Calcification rate has been demonstrated to vary among E. huxleyi strains according to maximum photosynthetic rate but also CO concentration at their sites of origin, while maximum photosynthetic rate also varied among the strains with CO2 at the site of origin (Rickaby et al., 2016). Several recent studies have called into question the importance of Ωcal in determining the response of calcifying organisms, with both theoretical and laboratory measurements support that the concentrations of HCO, CO, and H+ are more important (Kottmeier et al., 2016; Bach et al., 2015; Gafar et al., 2018). However, while HCO and CO were formally excluded from the OMI analyses as redundant variables, these vary more with CO2 and pH, respectively, which formed an axis along which the niche of the R/hyper-calcified morphotype was broad, rather than the orthogonal gradient in Ωcal. We caution that calcification rate might not relate in a simple way to the morphotypes observed here. Nevertheless, such trade-offs offer crucial clues into how traits related to calcification may be selected by the environment, resulting in the environmental patterns observed here. It might be worth exploring experimentally whether there is a role for Ωcal separate from other carbonate parameters in selecting the R/hypercalcified morphotype.
The OMI analysis presented here was limited as we focused mostly on the carbonate system, and this may be reflected in that sometimes half of the total variability was not explained by included variables. As mentioned above, several studies have shown that light can impact sensitivity of E. huxleyi to OA (Rokitta and Rost, 2012; Jin et al., 2017), although the effect reported differs in some studies (Zhang et al., 2019). The trade-offs in energy and C balances reported by Rickaby et al. (2016) would suggest that light and mixed-layer depth might also be important parameters to consider in future studies. Nutrient limitation can also modify calcification, although a careful chemostat study suggested that nutrient and CO2 impacts were independent (Müller et al., 2017). The impact of biotic factors was also not assessed with the OMI analysis here, though it is still not clear whether coccoliths might play roles in defence against either grazing or viruses (Harvey et al., 2015; Strom et al., 2018; Johns et al., 2019; Haunost et al., 2020).
A striking result from the OMI analysis was that all the E. huxleyi morphotypes, even the more specialized R/hyper-calcified type, exhibited much greater niche breadth (higher Tol values) than the other coccolithophore species. The three Gephyrocapsa species are very close relatives of E. huxleyi and phylogenetically should be considered congenerics (Bendif et al., 2016, 2019), but all showed lower niche breadth than the E. huxleyi morphotypes. The small G. parvula and G. ericsonii showed Tol values that were more than 10-fold lower than the most specialist E. huxleyi morphotype. Despite the evidence for a genetic underpinning of E. huxleyi morphotypes (Krueger-Hadfield et al., 2014), as well as evidence of a high level of genomic content variability in E. huxleyi (von Dassow et al., 2015), phylogenetic and phylogenomic evidence does not clearly support for it to be split into different species (Bendif et al., 2016; Filatov, 2019). If the ubiquitous taxon is less susceptible to environmental change compared to marginal taxa (i.e., marginality or richness vs. tolerance are inversely correlated; Dolédec et al., 2000; Hernández et al., 2015), the exceptional generalist behaviour exhibited by E. huxleyi compared to other coccolithophores suggests it may be more plastic and more adaptable in the face of environmental change.
The lower values of pH and Ωcal observed here approached levels predicted for higher latitudes of the global ocean at the end of the century under high-emission scenarios such as RCP8.5 (Feely et al., 2009; Hartin et al., 2016). Our results suggest that ongoing changes in ocean chemistry may result in decreases in coccolithophore diversity, leading to more numerical dominance of E. huxleyi compared to other coccolithophores, as well as decreased phenotypic diversity within E. huxleyi. The extended-domain niche analysis in the present study would suggest that C. leptoporus might be less adaptable than E. huxleyi. Some studies found that C. leptoporus was relatively resistant to OA in the lab (Langer et al., 2006), but others reported that it is sensitive and that its PIC POC (particulate organic carbon) ratio, considered important in determining ballast effects, is especially negatively affected by OA. Species such as C. leptoporus can be much more important than E. huxleyi in carbonate export due to their production of much heavier coccolithophores which sink faster and dissolve more slowly (e.g., Ziveri et al., 2007; Menschel et al., 2016; Menschel and González, 2019). Thus, a change to more E. huxleyi-dominated coccolithophore communities might negatively impact carbonate export.
Our study of how E. huxleyi abundances and morphotypes respond to the highly dynamic physical and chemical environments of southern Patagonia yielded seven principal findings.
-
The only coccolithophore that was a regular and ubiquitous component of the phytoplanktonic assemblages throughout the surface waters of the southern Patagonian fjords/channels was E. huxleyi. It occurred under a wide range of carbonate chemistry conditions and was only absent in the Skyring Sound zone where Ωcal<1.
-
Although E. huxleyi never reached more than a small fraction of total plankton carbon biomass (< 13 % of nano- and microplankton assemblages counted by microscopy), it reached moderate abundances (range: 12–276×103 cells L−1) comparable to adjacent coastal and oceanic areas, and within the lower range of stocks reported from Norwegian fjords (1–115 000×103 cells L−1).
-
Emiliania huxleyi abundance was highest ( cells L−1) when assemblages of large diatoms were lowest ( cells L−1), in late spring waters with lower macronutrients, consistent with it being most important in the absence of large diatoms.
-
In terms of morphotypes, the E. huxleyi populations in the southern Patagonian fjords/channels were similar to Norwegian fjords (dominated by moderately calcified and robustly calcified A morphotype) and very distinct from populations previously documented in the Southern Ocean and Drake Passage and the Patagonian Shelf of the Atlantic (where C or B/C morphotypes were reported as dominant) and from the eastern South Pacific coastal upwelling zone, where the R/hypercalcified morphotype dominated.
-
Niche analysis shows that the moderate A morphotype and A-CC morphotypes are generalists, whereas the R/hyper-calcified morphotype has a more marginal (specialized) realized niche.
-
The association of the R/hyper-calcified morphotype to high Ωcal in southern Patagonia, where Ωcal is driven principally by freshwater input, contrasts with its dominance in the upwelling system of central Chile to Peru, where low Ωcal is due to high CO2. This morphotype occupies a narrow range of Ωcal values compared to the A-CC and moderate A morphotypes.
-
The moderate A, A-CC, and R/hyper-calcified E. huxleyi morphotypes all display higher niche breadth (more generalist behaviour) than closely related coccolithophores, suggesting that E. huxleyi may be ecologically more plastic and have more capacity for adaptation in the face of environmental change than other coccolithophores.
All data resulting from this study are available from the corresponding authors upon request. The scanning electron micrograph image datasets can be found at https://doi.org/10.5281/zenodo.4292020 (Díaz-Rosas et al., 2021a). The inverted microscope images used for biovolume and carbon biomass estimations can be found at https://doi.org/10.5281/zenodo.5139161 (Díaz-Rosas et al., 2021b). Abundances of all plankton quantified in 2015 can be found at https://doi.org/10.1594/PANGAEA.936506 (Días-Rosas et al. 2021c). Coccolithophore relative abundances can be found at https://doi.org/10.1594/PANGAEA.936509 (Días-Rosas et al. 2021d). Physical–chemical parameters associated with abundances and biovolumes of distinct E. huxleyi morphotypes can be found at https://doi.org/10.1594/PANGAEA.936505 (Días-Rosas et al. 2021e).
Material for SEM characterization (filter sections) are in Dr. Peter von Dassow's laboratory. laboratory and can be requested.
The supplement related to this article is available online at: https://doi.org/10.5194/bg-18-5465-2021-supplement.
FDR (conceptualization, investigation, data curation, formal analysis, writing – original draft preparation, writing – review and editing) led the study, carried out sampling in the 2015 survey, carried out light and SEM microscopic analysis, conducted characterization of microplankton and E. huxleyi assemblages and morphotype composition, performed biovolume/allometric analysis, analyzed the relationships between E. huxleyi and environmental and biological variables, and wrote the first drafts of the paper. PvD (conceptualization, funding acquisition, supervision, validation, visualization, writing – original draft preparation, writing – review and editing) trained and supervised FDR in E. huxleyi assemblage quantification and characterization, guided analysis strategies, performed extensive re-writes of the text and figures, and provided continuous insights into interpretation of results and how to structure the manuscript. CAdS (formal analysis, supervision, validation, visualization, writing – review and editing) trained and supervised FDR in microplankton light-microscopy qualitative and quantitative analysis; performed nMDS, CA, and OMI niche analysis; guided interpretations of these results; and helped with extensive re-writes of the text and figures. EA (investigation, formal analysis, writing – review and editing) and RT (funding acquisition, investigation, formal analysis, writing – review and editing) performed the analysis of carbonate system parameters, nutrients, opal and chl a and helped characterize the physical environments. EM (investigation, formal analysis, writing – review and editing) carried out sampling and CTD deployment during the 2017 survey and together with HG (funding acquisition, writing – review and editing) provided insights into the interpretation of oceanographic results. All co-authors provided key comments and editing of the final draft of the paper.
The authors declare that they have no conflict of interest.
Publisher's note: Copernicus Publications remains neutral with regard to jurisdictional claims in published maps and institutional affiliations.
The authors thank the captain and crew of the M/N Forrest, Paulina Möller, and Sebastián Cornejo for assisting with onboard parameter recording and CTD deployment during the 2015 survey and Jeremy Young for advice in classification of E. huxleyi morphotypes.
This study was supported by the Comisión Nacional de Investigación Científica y Tecnológica (now Agencia Nacional de Investigación y Desarrollo) through FONDECYT grants 1181614 and 1140385), a doctoral fellowship CONICYT- PCHA/Doctorado Nacional/2013–21130158 to FDR), by the Iniciativa Científica Milenio through the Instituto Milenio de Oceanografía de Chile (grant ICN12_019), and the Centro de Investigación: Dinámica de Ecosistemas Marinos de Altas Latitudes de Chile (grant FONDAP 15150003). Electron microscopy analysis was performed in the Centro de Investigación en Nanotecnología y Materiales Avanzados of the Facultad de Física of the Pontificia Universidad Católica de Chile using a SEM instrument purchased with grant FONDEQUIP EQM150101.
This paper was edited by Clare Woulds and reviewed by Mariem Saavedra-Pellitero and two anonymous referees.
Abril, G., Bouillon, S., Darchambeau, F., Teodoru, C. R., Marwick, T. R., Tamooh, F., Ochieng Omengo, F., Geeraert, N., Deirmendjian, L., Polsenaere, P., and Borges, A. V.: Technical Note: Large overestimation of pCO2 calculated from pH and alkalinity in acidic, organic-rich freshwaters, Biogeosciences, 12, 67–78, https://doi.org/10.5194/bg-12-67-2015, 2015.
Alves-de-Souza, C., González, M., and Iriarte, J.: Functional groups in marine phytoplankton assemblages dominated by diatoms in fjords of southern Chile, J. Plankton Res., 30, 1233–1243, https://doi.org/10.1093/plankt/fbn079, 2008.
Alvites, D.: Variabilidad espacial y calcificación de las comunidades de cocolitofóridos en el sistema de afloramiento costero frente al Callao-Peruí, Tesis de Maestro, Universidad Peruana Cayetano Heredia, 128 pp., 2016.
Bach, L., Riebesell, U., Gutowska, M., Federwisch, L., and Schulz, K.: A unifying concept of coccolithophore sensitivity to changing carbonate chemistry embedded in an ecological framework, Prog. Oceanogr., 135, 125–138, https://doi.org/10.1016/j.pocean.2015.04.012, 2015.
Baumann, K.-H., Andruleit, H., and Samtleben, C.: Coccolithophores in the Nordic Seas: comparison of living communities with surface sediment assemblages, Deep-Sea Res. Pt. II, 47, 1743–1772, https://doi.org/10.1016/S0967-0645(00)00005-9, 2000.
Beaufort, L., Couapel, M., Buchet, N., Claustre, H., and Goyet, C.: Calcite production by coccolithophores in the south east Pacific Ocean, Biogeosciences, 5, 1101–1117, https://doi.org/10.5194/bg-5-1101-2008, 2008.
Beaufort, L., Probert, I., de Garidel-Thoron, T., Bendif, E., Ruiz-Pino, D., Metzl, N., Goyet, C., Buchet, N., Coupel, P., Grelaud, M., Rost, B., Rickaby, R., and de Vargas, C.: Sensitivity of coccolithophores to carbonate chemistry and ocean acidification, Nature, 476, 80–83, https://doi.org/10.1038/nature10295, 2011.
Bendif, E., Probert, I., Carmichael, M., Romac, S., Hagino, K., and de Vargas, C.: Genetic delineation between and within the widespread coccolithophore morpho-species Emiliania huxleyi and Gephyrocapsa oceanica (Haptophyta), J. Phycol., 50, 140–148, https://doi.org/10.1111/jpy.12147, 2014.
Bendif, E., Probert, I., Díaz-Rosas, F., Thomas, D., van den Engh, G., Young, J., and von Dassow, P.: Recent reticulate evolution in the ecologically dominant lineage of coccolithophores, Front. Microbiol., 7, 784, https://doi.org/10.3389/fmicb.2016.00784, 2016.
Bendif, E., Nevado, B., Wong, E., Hagino, K., Probert, I., Young, J., Rickaby, R., and Filatov, D.: Repeated species radiations in the recent evolution of the key marine phytoplankton lineage Gephyrocapsa, Nat. Commun., 10, 4234, https://doi.org/10.1038/s41467-019-12169-7, 2019.
Berge, G.: Discoloration of the sea due to Coccolithus huxley I “bloom”, Sarsia, 6, 27–40, https://doi.org/10.1080/00364827.1962.10410259, 1962.
Birkenes, E. and Braarud, T.: Phytoplankton in the Oslo fjord during a “Coccolithus huxleyi-summer”, Avhandl. Norske Videnskaps – Akd. Oslo. I. Mat. Naturv. Klasse, 2, 1–23, 1952.
Bollmann, J., Cortés, M., Haidar, A., Brabec, B., Close, A., Hofmann, R., Palma, S., Tupas, L., and Thierstein, H.: Techniques for quantitative analyses of calcareous marine phytoplankton, Mar. Micropaleontol., 44, 163–185, https://doi.org/10.1016/S0377-8398(01)00040-8, 2002.
Broecker, W. and Clark, E.: Ratio of coccolith CaCO3 to foraminifera CaCO3 in late Holocene deep sea sediments, Paleoceanography, 24, 1–11, https://doi.org/10.1029/2009PA001731, 2009.
Brzezinski, M., Dickson, M., Nelson, D., and Sambrotto, R.: Ratios of Si, C and N uptake by microplankton in the Southern Ocean, Deep-Sea Res. Pt. II, 50, 619–633, https://doi.org/10.1016/S0967-0645(02)00587-8, 2003.
Cermeño, P., Lee, J.-B., Wyman, K., Schofield, O., and Falkowski, P: Competitive dynamics in two species of marine phytoplankton under non-equilibrium conditions, Mar. Ecol. Prog. Ser., 429, 1928, https://doi.org/10.3354/meps09088, 2011.
Charalampopoulou, A., Poulton, A., Tyrrell, T., and Lucas, M.: Irradiance and pH affect coccolithophore community composition on a transect between the North Sea and the Arctic Ocean, Mar. Ecol. Prog. Ser., 431, 25–43, https://doi.org/10.3354/meps09140, 2011.
Charalampopoulou, A., Poulton, A. J., Bakker, D. C. E., Lucas, M. I., Stinchcombe, M. C., and Tyrrell, T.: Environmental drivers of coccolithophore abundance and calcification across Drake Passage (Southern Ocean), Biogeosciences, 13, 5917–5935, https://doi.org/10.5194/bg-13-5917-2016, 2016.
Cook, S., Jones, R., Vaillancourt, R., and Hallegraeff, G.: Genetic differentiation among Australian and southern ocean populations of the ubiquitous coccolithophore Emiliania huxleyi (Haptophyta), Phycologia, 52, 368–374, https://doi.org/10.2216/12-111.1, 2013.
Corstjens, P., van der Kooij, A., Linschooten, C., Brouwers, G.-J., Westbroek, P., and Vrind-de Jong, E.: GPA, a calcium-binding protein in the coccolithophorid Emiliania huxleyi (Prymnesiophyceae), J. Phycol., 34, 622–630, 1998.
Cubillos, J., Wright, S., Nash, G., de Salas, M., Griffiths, B., Tilbrook, B., Poisson, A., and Hallegraeff, G.: Calcification morphotypes of the coccolithophorid Emiliania huxleyi in the Southern Ocean: changes in 2001 to 2006 compared to historical data, Mar. Ecol. Prog. Ser., 348, 47–54, https://doi.org/10.3354/meps07058, 2007.
Cuevas, A., Tapia, F., Iriarte, J., Gonzaìlez, H., Silva, N., and Vargas, C.: Interplay between freshwater discharge and oceanic waters modulates phytoplankton size-structure in fjords and channel systems of the Chilean Patagonia, Prog. Oceanogr., 173, 103–113, https://doi.org/10.1016/j.pocean.2019.02.012, 2019.
Cyronak, T., Schulz, K., and Jokiel, P.: The Omega myth: what really drives lower calcification rates in an acidifying ocean, ICES J. Mar. Sci., 73, 558–562, https://doi.org/10.1093/icesjms/fsv075, 2016.
Dávila, P., Figueroa, D., and Müller, E.: Freshwater input into the coastal ocean and its relation with the salinity distribution off austral Chile (35–55∘ S), Cont. Shelf Res., 22, 521–534, https://doi.org/10.1016/S0278-4343(01)00072-3, 2002.
Díaz-Rosas, F., Alves-de-Souza, C., Alarcón, E., Menschel, E., González, H., Torres, R., and von Dassow, P.: Scanning electron microscopy image dataset – Abundances and morphotypes of the coccolithophore Emiliania huxleyi in southern Patagonian fjords and channels, Zenodo [data set], https://doi.org/10.5281/zenodo.4292020, 2021a.
Díaz-Rosas, F., Alves-de-Souza, C., Alarcón, E., Menschel, E., González, H., Torres, R., and von Dassow, P.: Inverted microscopy image dataset – Carbon biomass of microplankton assemblages in southern Patagonian fjords and channels, Zenodo [data set], https://doi.org/10.5281/zenodo.5139161, 2021b.
Díaz-Rosas, F., Alves-de-Souza, C., Alarcón, E., Menschel, E., González, H., Torres, R., and von Dassow, P.: Abundances of planktonic items found in southern Patagonia during the austral late-spring 2015, PANGAEA [data set], https://doi.org/10.1594/PANGAEA.936506, 2021c.
Díaz-Rosas, F., Alves-de-Souza, C., Alarcón, E., Menschel, E., González, H., Torres, R., and von Dassow, P.: Abundances of coccolithophores found in southern Patagonia during the austral early-spring 2017, PANGAEA [data set], https://doi.org/10.1594/PANGAEA.936509, 2021d.
Díaz-Rosas, F., Alves-de-Souza, C., Alarcón, E., Menschel, E., González, H., Torres, R., and von Dassow, P.: Physical and chemical parameters and associated abundance and biovolume of Emiliania huxleyi morphotypes recorded in southern Patagonia during the austral late-spring 2015 and early-spring 2017, PANGAEA [data set], https://doi.org/10.1594/PANGAEA.936505, 2021e.
Dickson, A. and Millero, F.: A comparison of the equilibrium constants for the dissociation of carbonic acid in seawater media, Deep-Sea Res., 34, 1733–1743, https://doi.org/10.1016/0198-0149(87)90021-5, 1987.
Dolédec, S., Chessel, D., and Gimaret-Carpentier, C.: Niche separation in community analysis: A new method, Ecology, 81, 2914–2927, https://doi.org/10.2307/177351, 2000.
Doney, S., Fabry, V., Feely, R., and Kleypas, J.: Ocean acidification: the other CO2 problem, Annu. Rev. Mar. Sci., 1, 169–192, https://doi.org/10.1146/annurev.marine.010908.163834, 2009.
Dray, S. and Dufour, A.: The ade4 package: implementing the duality diagram for ecologists, J. Stat. Softw, 22, 1–20, https://doi.org/10.18637/jss.v022.i04, 2007.
Dufrene, M. and Legendre, P.: Species assemblages and indicator species: the need for a flexible asymmetrical approach, Ecol. Monogr., 67, 345–366, https://doi.org/10.2307/2963459, 1997.
Egge, E., Johannessen, T., Andersen, T., Eikrem, W., Bittner, L., Larsen, A., Sandaa, R., and Edvardsen, B.: Seasonal diversity and dynamics of haptophytes in the Skagerrak, Norway, explored by high-throughput sequencing, Mol. Ecol., 24, 3026–3042, https://doi.org/10.1111/mec.13160, 2015.
Erga, S.: Ecological studies on the phytoplankton of Boknafjorden, western Norway, 1. The effect of water exchange processes and environmental factors on temporal and vertical variability of biomass, Sarsia, 74, 161–176, https://doi.org/10.1080/00364827.1989.10413425, 1989.
Feely, R., Doney, S., and Cooley, S.: Ocean acidification: Present conditions and future changes in a high-CO2 world, Oceanography, 22, 36–47, https://doi.org/10.5670/oceanog.2009.95, 2009.
Fernández, E., Marañón, E., Harbour, D., Kristiansen, S., and Heimdal, B.: Patterns of carbon and nitrogen uptake during blooms of Emiliania huxleyi in two Norwegian fjords, J. Plankton Res., 18, 2349–2366, https://doi.org/10.1093/plankt/18.12.2349, 1996.
Filatov, D.: Extreme Lewontin's paradox in ubiquitous marine phytoplankton species, Mol. Biol. Evol., 36, 4–14, https://doi.org/10.1093/molbev/msy195, 2019.
Franco, A., Gruber, N., Frölicher, T., and Artman, L.: Contrasting impact of future CO2 emission scenarios on the extent of CaCO3 mineral undersaturation in the Humboldt Current System, J. Geophys. Res.-Oceans, 123, 2018–2036, https://doi.org/10.1002/2018JC013857, 2018.
Gafar, N., Eyre, B., and Schulz, K.: A conceptual model for projecting coccolithophorid growth, calcification and photosynthetic carbon fixation rates in response to global ocean change, Front. Mar. Sci., 4, 433, https://doi.org/10.3389/fmars.2017.00433, 2018.
Giraudeau, J., Monteiro, P., and Nikodemus, K.: Distribution and malformation of living coccolithophores in the northern Benguela upwelling system off Namibia, Mar. Micropaleontol., 22, 93–110, https://doi.org/10.1016/0377-8398(93)90005-I, 1993.
González, H., Castro, L., Daneri, G., Iriarte, J., Silva, N., Tapia, F., Teca, E., and Vargas, C.: Land-ocean gradient in haline stratification and its effects on plankton dynamics and trophic carbon fluxes in Chilean Patagonian fjords (47–50∘ S), Prog. Oceanogr., 119, 32–47, https://doi.org/10.1016/j.pocean.2013.06.003, 2013.
Gran-Stadniczeñko, S., Šupraha, L., Egge, E., and Edvardsen, B.: Haptophyte diversity and vertical distribution explored by 18∘ S and 28∘ S ribosomal RNA gene metabarcoding and scanning electron microscopy, J. Eukaryot. Microbiol., 64, 514–532, https://doi.org/10.1111/jeu.12388, 2017.
Hagino, K. and Okada, H.: Intra- and infra-specific morphological variation in selected coccolithophore species in the equatorial and subequatorial Pacific Ocean, Mar. Micropaleontol., 58, 184–206, https://doi.org/10.1016/j.marmicro.2005.11.001, 2006.
Hagino, K., Bendif, E., Young, J., Kogame, K., Probert, I., Takano, Y., Horiguchi, T., de Vargas, C., and Okada, H.: New evidence for morphological and genetic variation in the cosmopolitan coccolithophore Emiliania huxleyi (Prymnesiophyceae) from the cox1b-atp4 genes, J. Phycol., 47, 1164–1176, https://doi.org/10.1111/j.1529-8817.2011.01053.x, 2011.
Hartin, C. A., Bond-Lamberty, B., Patel, P., and Mundra, A.: Ocean acidification over the next three centuries using a simple global climate carbon-cycle model: projections and sensitivities, Biogeosciences, 13, 4329–4342, https://doi.org/10.5194/bg-13-4329-2016, 2016.
Harvey, E., Bidle, K., and Johnson, M.: Consequences of strain variability and calcification in Emiliania huxleyi on microzooplankton grazing, J. Plankton Res., 37, 1137–1148, https://doi.org/10.1093/plankt/fbv081, 2015.
Haunost, M., Riebesell, U., and Bach, L.: The calcium carbonate shell of Emiliania huxleyi provides limited protection against viral infection, Front. Mar. Sci., 7, 530757, https://doi.org/10.3389/fmars.2020.530757, 2020.
Henderiks, J., Winter, A., Elbra'chter, M., Feistel, R., van der Plas, A., Nausch, G., and Barlow, R.: Environmental controls on Emiliania huxleyi morphotypes in the Benguela coastal upwelling system (SE Atlantic), Mar. Ecol. Prog. Ser., 448, 51–66, https://doi.org/10.3354/meps09535, 2012.
Hernández, T., Bacher, C., Soudant, D., Belin, C., and Barillé, L.: Assessing phytoplankton realized niches using a French national phytoplankton monitoring network, Estuarine, Coast. Shelf Sci., 159, 15–27, https://doi.org/10.1016/j.ecss.2015.03.010, 2015.
Hillebrand, H., Dürselen, C., Kirschtel, D., Pollingher, U., and Zohary, T.: Biovolume calculation for pelagic and benthic microalgae, J. Phycol., 35, 403–424, https://doi.org/10.1046/j.1529-8817.1999.3520403.x, 1999.
Hopkins, J., Henson, S., Poulton, A., and Balch, W.: Regional characteristics of the temporal variability in the global particulate inorganic carbon inventory, Global Biogeochem. Cy., 33, 1328–1338, https://doi.org/10.1029/2019GB006300, 2019.
Iglesias-Rodriguez, D., Halloran, P., Rickaby, R., Hall, I., Colmenero-Hidalgo, E., Gittins, J., Green, D., Tyrrell, T., Gibbs, S., von Dassow, P., Rehm, E., Armbrust, V., and Boessenkool, K.: Phytoplankton calcification in a high-CO2 world, Science, 320, 336–340, https://doi.org/10.1126/science.1154122, 2008.
Jin, P., Ding, J., Xing, T., Riebesell, U., and Gao, K.: High levels of solar radiation offset impacts of ocean acidification on calcifying and non-calcifying strains of Emiliania huxleyi, Mar. Ecol. Prog. Ser., 568, 47–58, https://doi.org/10.3354/meps12042, 2017.
Johns, Ch., Grubb, A., Nissimov, J., Natale, F., Knapp, V., Mui, A., Fredricks, H., Van Mooy, B., and Bidle, K.: The mutual interplay between calcification and coccolithovirus infection, Environ. Microb., 21, 1896–1915, https://doi.org/10.1111/1462-2920.14362, 2019.
Johnsen, S. and Bollmann, J.: Coccolith mass and morphology of different Emiliania huxleyi morphotypes: A critical examination using Canary Islands material, PLOS ONE, 15, e0230569, https://doi.org/10.1371/journal.pone.0230569, 2020.
Jones, E., Chierici, M., Skjelvan, I., Norli, M., Børsheim, K., Lødemel, H., Sørensen, K., King, A., Lauvset, S., Jackson, K., de Lange, T., Johannsessen, T., and Mourgues, C.: Monitoring ocean acidification in Norwegian seas in 2018, Rapport, Miljødirektoratet, M-1417-2019, 2019.
Klaas, C. and Archer, D.: Association of sinking organic matter with various types of mineral ballast in the deep sea: Implications for the rain ratio, Global Biogeochem. Cy., 16, 1116, https://doi.org/10.1029/2001GB001765, 2002.
Kottmeier, D., Rokitta, S., and Rost, B.: Acidification, not carbonation, is the major regulator of carbon fluxes in the coccolithophore Emiliania huxleyi, New Phytol., 211, 126–137, 2016.
Kleypas, J., Feely, R., Fabry, V., Langdon, C., Sabine, C., and Robbins, L. Impacts of Ocean Acidification on Coral Reefs and Other Marine Calcifiers: A Guide for Future Research. Report of a workshop held 18–20 April 2005, St. Petersburg, FL, sponsored by NSF, NOAA, and the U.S. Geological Survey, 88 pp., 2006.
Kristiansen, S., Thingstad, F., van der Wal, P., Farbrot, T., and Skjoldal, E.: An Emiliania huxleyi dominated subsurface bloom in Samnangerfjorden, western Norway, Importance of hydrography and nutrients, Sarsia, 79, 357–368, https://doi.org/10.1080/00364827.1994.10413567, 1994.
Krueger-Hadfield, S. A., Balestreri, C., Schroeder, J., Highfield, A., Helaouët, P., Allum, J., Moate, R., Lohbeck, K. T., Miller, P. I., Riebesell, U., Reusch, T. B. H., Rickaby, R. E. M., Young, J., Hallegraeff, G., Brownlee, C., and Schroeder, D. C.: Genotyping an Emiliania huxleyi (prymnesiophyceae) bloom event in the North Sea reveals evidence of asexual reproduction, Biogeosciences, 11, 5215–5234, https://doi.org/10.5194/bg-11-5215-2014, 2014.
Langer, G. and Geisen, M.: Species-specific responses of calcifying algae to changing seawater carbonate chemistry, Geochem. Geophys. Geosys., 7, Q09006, https://doi.org/10.1029/2005GC001227, 2006.
Langer, G., Nehrke, G., Probert, I., Ly, J., and Ziveri, P.: Strain-specific responses of Emiliania huxleyi to changing seawater carbonate chemistry, Biogeosciences, 6, 2637–2646, https://doi.org/10.5194/bg-6-2637-2009, 2009.
Langer, G., Probert, I., Nehrke, G., and Ziveri, P.: The morphological response of Emiliania huxleyi to seawater carbonate chemistry changes: an inter-strain comparison, J. Nannoplankton Res., 32, 29–34, ISSN 1210-8049, 2011.
Leblanc, K., Queìguiner, B., Diaz, F., Cornet, V., Michel-Rodriguez, M., Durrieu de Madron, X., Bowler, C., Malviya, S., Thyssen, M., Greìgori, G., Rembauville, M., Grosso, O., Poulain, J., de Vargas, C., Pujo-Pay, M., and Conan, P.: Nanoplanktonic diatoms are globally overlooked but play a role in spring blooms and carbon export, Nat. Commun., 9, 953, https://doi.org/10.1038/s41467-018-03376-9, 2018.
Legendre, P. and Legendre, L.: Numerical Ecology, 3rd English edition, Elsevier Science BV, Amsterdam, 1006 pp., 2012.
McIntyre, A., Bé, A., and Roche, M.: Modern Pacific coccolithophorida: a paleontological thermometer, Trans. N. Y. Acad. Sci., 32, 720–731, https://doi.org/10.1111/j.2164-0947.1970.tb02746.x, 1970.
Margalef, R.: Life-forms of phytoplankton as survival alternatives in an unstable environment, Oceanol. Ac., 1, 493–509, 1978.
Mehrbach, C., Culberson, C., Hawley, J., and Pytkowicx, R.: Measurement of the apparent dissociation constants of carbonic acid in seawater at atmospheric pressure, Limnol. Oceanogr., 18, 897–907, https://doi.org/10.4319/lo.1973.18.6.0897, 1973.
Mella-Flores, D., Machon, J., Contreras-Porcia, L., Mesa-Campbell, S., and von Dassow, P.: Differential responses of Emiliania huxleyi (Haptophyta) strains to copper excess, Cryptogamie, Algologie, 39, 481–509, https://doi.org/10.7872/crya/v39.iss4.2018.481, 2018.
Menden-Deuer, S. and Lessard, E.: Carbon to volume relationships for dinoflagellates, diatoms, and other protist plankton, Limnol. Oceanogr., 45, 569–579, https://doi.org/10.4319/lo.2000.45.3.0569, 2000.
Menschel, E. and González, H.: Carbon and calcium carbonate export driven by appendicularian faecal pellets in the Humboldt current system off Chile, Sci. Rep., 9, 16501, https://doi.org/10.1038/s41598-019-52469-y, 2019.
Menschel, E., Gonzaìlez, H., and Giesecke, R.: Coastal-oceanic distribution gradient of coccolithophores and their role in the carbonate flux of the upwelling system off Concepcioìn, Chile (36∘ S), J. Plankton Res., 38, 798–817, https://doi.org/10.1093/plankt/fbw037, 2016.
Meyer, J. and Riebesell, U.: Reviews and Syntheses: Responses of coccolithophores to ocean acidification: a meta-analysis, Biogeosciences, 12(6), 1671–1682, https://doi.org/10.5194/bg-12-1671-2015, 2015.
Monteiro, F., Bach, L., Brownlee, C., Bown, P., Rickaby, R., Poulton, A., Tyrrell, T., Beaufort, L., Dutkiewicz, S., Gibbs, S., Gutowska, M., Lee, R., Riebesell, U., Young, J., and Ridgwell, A.: Why marine phytoplankton calcify, Sci. Adv., 2, e1501822, https://doi.org/10.1126/sciadv.1501822, 2016.
Müller, M., Trull, T., and Hallegraeff, G.: Differing responses of three Southern Ocean Emiliania huxleyi ecotypes to changing seawater carbonate chemistry, Mar. Ecol. Prog. Ser., 531, 81–90, https://doi.org/10.3354/meps11309, 2015.
Müller, M., Trull, T., and Hallegraeff, G.: Independence of nutrient limitation and carbon dioxide impacts on the Southern Ocean coccolithophore Emiliania huxleyi, ISME J., 11, 1777–1787, https://doi.org/10.1038/ismej.2017.53, 2017.
Nejstgaard, J., Gismervik, I., and Solberg, P.: Feeding and reproduction by Calanus finmarchicus, and microzooplankton grazing during mesocosm blooms of diatoms and the coccolithophore Emiliania huxleyi, Mar. Ecol. Prog. Ser., 147, 197–217, 1997.
O'Brien, C. J., Peloquin, J. A., Vogt, M., Heinle, M., Gruber, N., Ajani, P., Andruleit, H., Arístegui, J., Beaufort, L., Estrada, M., Karentz, D., Kopczyńska, E., Lee, R., Poulton, A. J., Pritchard, T., and Widdicombe, C.: Global marine plankton functional type biomass distributions: coccolithophores, Earth Syst. Sci. Data, 5, 259–276, https://doi.org/10.5194/essd-5-259-2013, 2013.
Oksanen, J., Kindt, R., Legendre, P., O'Hara, B., and Stevens, H.: The vegan package, Community Ecology Package, 10, 631–637, 2007.
Paasche, E. and Kristiansen, S.: Ammonium regeneration by microzooplankton in the Oslofjord, Mar. Biol., 69, 55–63, https://doi.org/10.1007/BF00396961, 1982.
Paredes, M., Montecino, V., Anic, V., Egaña, M., and Guzmán, L.: Diatoms and dinoflagellates macroscopic regularities shaped by intrinsic physical forcing variability in Patagonian and Fuegian fjords and channels (48–56∘ S), Prog. Oceanogr., 129, 85–97, https://doi.org/10.1016/j.pocean.2014.07.002, 2014.
Passow, U. and Carlson, C.: The biological pump in a high CO2 world, Mar. Ecol. Prog. Ser., 470, 249–271, https://doi.org/10.3354/meps09985, 2012.
Paul, A. and Bach, L.: Universal response pattern of phytoplankton growth rates to increasing CO2, New Phytol., 228, 1710–1716, https://doi.org/10.1111/nph.16806, 2020.
Pierrot, D., Lewis, E., and Wallace, D.: MS Excel Program Developed for CO2 System Calculations. ORNL/CDIAC-105a, Carbon Dioxide Information Analysis Center, Oak Ridge National Laboratory, U.S. Department of Energy, Oak Ridge, Tennessee, https://doi.org/10.3334/CDIAC/otg.CO2SYS_XLS_CDIAC105a, 2006.
Poulton, A., Young, J., Bates, N., and Balch, W.: Biometry of detached Emiliania huxleyi coccoliths along the Patagonian Shelf, Mar. Ecol. Prog. Ser., 443, 1–17, https://doi.org/10.3354/meps09445, 2011.
Poulton, A., Painter, S., Young, J., Bates, N., Bowler, B., Drapeau, D., Lyczsckowski, E., and Balch, W.: The 2008 Emiliania huxleyi bloom along the Patagonian Shelf: Ecology, biogeochemistry, and cellular calcification, Global Biogeochem. Cy., 27, 1023–1033, https://doi.org/10.1002/2013GB004641, 2013.
R Core Team: R: A language and environment for statistical computing. R Foundation for Statistical Computing, Vienna, Austria, available at: http://www.R-project.org/ (last access: 16 November 2020), 2019.
Read, B., Kegel, J., Klute, M., Kuo, A., Lefebvre, S., Maumus, F., Mayer, Ch., Miller, J., Monier, A., Salamov, A., Young, J., Aguilar, M., Claverie, J.-M-, Frickenhaus, S., Gonzalez, K., Herman, E., Lin, Y.-Ch., Napier, J., Ogata, H., Sarno, A., Shmutz, J., Schroeder, D., de Vargas, C., Verret, F., von Dassow, P., Valentin, K., Van de Peer, Y., Wheeler, G., Emiliania huxleyi Annotation Consortium, Dacks, J., Delwiche, Ch., Dyhrman, S., Glöckner, G., John, U., Richards, T., Worden, A., Zhang, X., and Grigoriev, I.: Pan genome of the phytoplankton Emiliania underpins its global distribution, Nature, 499, 209–213, https://doi.org/10.1038/nature12221, 2013.
Rickaby, R., Hermoso, M., Lee, R., Rae, B., Heureux, A., Balestreri, C., Chakravarti, L., Schroeder, D., and Brownlee, C.: Environmental carbonate chemistry selects for phenotype of recently isolated strains of Emiliania huxleyi, Deep-Sea Res. Pt. II, 127, 2840, https://doi.org/10.1016/j.dsr2.2016.02.010, 2016.
Riebesell, U., Bach, L., Bellerby, R., Monsalve, R., Boxhammer, T., Czerny, J., Larsen, A., Ludwig, A., and Schulz, K.: Competitive fitness of a predominant pelagic calcifier impaired by ocean acidification, Nat. Geosci., 10, 19–23, https://doi.org/10.1038/ngeo2854, 2017.
Rivero-Calle, S., Gnanadesikan, A., Del Castillo, C., Balch, W., and Guikema, S.: Multidecadal increase in North Atlantic coccolithophores and the potential role of rising CO2, Science, 350, 1533–1537, https://doi.org/10.1126/science.aaa8026, 2015.
Rokitta, S. and Rost, B.: Effects of CO2 and their modulation by light in the life-cycle stages of the coccolithophore Emiliania huxleyi, Limnology and Oceanography, 57, 607–618, https://doi.org/10.4319/lo.2012.57.2.0607, 2012.
Saavedra-Pellitero, M., Baumann, K., Flores, J., and Gersonde, R.: Biogeographic distribution of living coccolithophores in the Pacific sector of the Southern Ocean, Mar. Micropaleontol., 109, 1–20, https://doi.org/10.1016/j.marmicro.2014.03.003, 2014.
Saavedra-Pellitero, M., Baumann, K.-H., Fuertes, M. Á., Schulz, H., Marcon, Y., Vollmar, N. M., Flores, J.-A., and Lamy, F.: Calcification and latitudinal distribution of extant coccolithophores across the Drake Passage during late austral summer 2016, Biogeosciences, 16, 3679–3702, https://doi.org/10.5194/bg-16-3679-2019, 2019.
Samtleben, C., Schäfer, P., Andruleit, H., Baumann, A., Baumann, K., Kohly, A., Matthiessen, J., Schröder-Ritzrau, A., and “Synpal” Working Group: Plankton in the Norwegian-Greenland Sea: from living communities to sediment assemblages - an actualistic approach, Geol. Rundsch., 84, 108–136, https://doi.org/10.1007/BF00192245, 1995.
Schlitzer, R.: Ocean Data View, available at: https://odv.awi.de/ (last access: 27 November 2020), 2018.
Schroeder, D., Biggi, G., Hall, M., Davy, J., Martínez, J., Richardson, A., Malin, G., and Wilson, W.: A genetic marker to separate Emiliania huxleyi (Prymnesiophyceae) morphotypes, J. Phycol., 41, 874–879, https://doi.org/10.1111/j.1529-8817.2005.04188.x, 2005.
Sicko-Goad, L., Schelske, C., and Stoermer, E.: Estimation of intracellular carbon and silica content of diatoms from natural assemblages using morphometric techniques, Limnol. Oceanogr., 29, 1170–1178, https://doi.org/10.4319/lo.1984.29.6.1170, 1984.
Sievers, H. and Silva, N.: Water masses and circulation in austral Chilean channels and fjords, in: Progress in the oceanographic knowledge of Chilean interior waters, from Puerto Montt to Cape Horn, edited by: Silva, N. and Palma, S., Comité Oceanográfico Nacional, Pontificia Universidad Católica de Valparaiso, 53–58, 2008.
Smith, H., Tyrrell, T., Charalampopoulou, A., Dumousseaud, C., Legge, O., Birchenough, S., Pettit, L., Garley, R., Hartman, S., Hartman, M., Sagoo, N., Daniels, C., Achterberg, E., and Hydes, D.: Predominance of heavily calcified coccolithophores at low CaCO3 saturation during winter in the Bay of Biscay, P. Natl. Acad. Sci. USA, 109, 8845–8849, https://doi.org/10.1073/pnas.1117508109, 2012.
Smith, H. E. K., Poulton, A. J., Garley, R., Hopkins, J., Lubelczyk, L. C., Drapeau, D. T., Rauschenberg, S., Twining, B. S., Bates, N. R., and Balch, W. M.: The influence of environmental variability on the biogeography of coccolithophores and diatoms in the Great Calcite Belt, Biogeosciences, 14, 4905–4925, https://doi.org/10.5194/bg-14-4905-2017, 2017.
Strom, S., Bright, K., Fredrickson, K., and Cooney, E.: Phytoplankton defenses: Do Emiliania huxleyi coccoliths protect against microzooplankton predators?, Limnol. Oceanogr., 63, 617–627, https://doi.org/10.1002/lno.10655, 2018.
Takahashi, T., Sutherland, S., Chipman, D., Goddard, J., Ho, Ch., Newberger, T., Sweeney, C., and Munro, D.: Climatological distributions of pH, pCO2, total CO2, alkalinity, and CaCO3 saturation in the global surface ocean, and temporal changes at selected locations, Mar. Chem., 164, 95–125, https://doi.org/10.1016/j.marchem.2014.06.004, 2014.
Taylor, A., Brownlee, C., and Wheeler, G.: Coccolithophore cell biology: Chalking up progress, Annu. Rev. Mar. Sci., 9, 283–310, https://doi.org/10.1146/annurev-marine-122414-034032, 2017.
Torres, R., Pantoja, S., Harada, N., González, H., Daneri, G., Frangopulos, M., Rutllant, J., Duarte, C., Rúiz-Halpern, S., Mayol, E., and Fukasawa, M.: Air-sea CO2 fluxes along the coast of Chile: From CO2 outgassing in central northern upwelling waters to CO2 uptake in southern Patagonian fjords, J. Geophys. Res., 116, C09006, https://doi.org/10.1029/2010JC006344, 2011.
Torres, R., Silva, N., Reid, B., and Frangopulos, M.: Silicic acid enrichment of subantarctic surface water from continental inputs along the Patagonian archipelago interior sea (41–56∘ S), Prog. Oceanogr., 129, 50–61, https://doi.org/10.1016/j.pocean.2014.09.008, 2014.
Torres, R., Reid, B., Frangopulos, M., Alarcón E., Márquez M., Haussermann, V., Försterra, G., Pizarro, G., Iriarte, J., and Gonzalez, H.: Freshwater runoff effects on the production of biogenic silicate and chlorophyll-a in western Patagonia archipelago (50-51∘ S), Estuarine, Coast. Shelf Sci., 241, 106597, https://doi.org/10.1016/j.ecss.2020.106597, 2020.
Tynan, E., Tyrrell, T., and Achterberg, E.: Controls on the seasonal variability of calcium carbonate saturation states in the Atlantic gateway to the Arctic Ocean, Mar. Chem., 158, 1–9, https://doi.org/10.1016/j.marchem.2013.10.010, 2014.
Tyrrell, T. and Merico, A.: Emiliania huxleyi: bloom observation and the conditions that induce them, in Coccolithophores, edited by: Thierstein, H. and Young, J., Springer, Berlin, Heidelberg, Germany, 75–97, https://doi.org/10.1007/978-3-662-06278-4_4, 2004.
Utermöhl, H.: Vervollkommnung der quantitativen phytoplankton-methodik, Mitteilungen. Internationale Vereinigung für theoretische und angewandte Limnologie, 9, 1–38, 1958.
van Bleijswijk, J., van der Wal, P., Kempers, R., Veldhuis, M., Young, J., Muyzer, G., Vrind-de Jong, E., and Westbroek, P.: Distribution of two types of Emiliania huxleyi (Prymnesiophyceae) in the northeast Atlantic region as determined by immunofluorescence and coccolith morphology, J. Phycol., 27, 566–570, https://doi.org/10.1111/j.0022-3646.1991.00566.x, 1991.
Vivanco, X. and Seguel, M.: Manual Técnico – Curso teórico-práctico para el muestreo, identificación y enumeración de Alexandrium catenella y otros taxa nocivos, Instituto de Fomento Pesquero, Puerto Montt, Chile, available at: https://www.ifop.cl/marearoja/wp-content/uploads/sites/2/2016/07/Manual_Tecnico_curso_Marzo_2009-mod.pdf (last access: 18 November 2020), 2009.
von Dassow, P., John, U., Ogata, H., Probert, I., Bendif, E., Kegel, J., Audic, S., Wincker, P., Da Silva, C., Claverie, J., Doney, S., Glover, D., Mella, D., Herrera, Y., Lescot, M., Garet-Delmas, M., and de Vargas, C.: Life-cycle modification in open oceans accounts for genome variability in a cosmopolitan phytoplankton, ISME J., 9, 1365–1377, https://doi.org/10.1038/ismej.2014.221, 2015.
von Dassow, P., Díaz-Rosas, F., Bendif, E. M., Gaitán-Espitia, J.-D., Mella-Flores, D., Rokitta, S., John, U., and Torres, R.: Over-calcified forms of the coccolithophore Emiliania huxleyi in high-CO2 waters are not preadapted to ocean acidification, Biogeosciences, 15, 1515–1534, https://doi.org/10.5194/bg-15-1515-2018, 2018.
Young, J. and Westbroek, P.: Genotypic variation in the coccolithophorid species Emiliania huxleyi, Mar. Micropaleontol., 18, 5–23, https://doi.org/10.1016/0377-8398(91)90004-P, 1991.
Young, J.: Variation in Emiliania huxleyi coccolith morphology in samples from the Norwegian EHUX experiment, 1992, Sarsia, 79, 417–425, https://doi.org/10.1080/00364827.1994.10413573, 1994.
Young, J., Geisen, M., Cros, L., Kleijne, A., Sprengel, C., Probert, I., and Ostergaard, J.: A guide to extant coccolithophore taxonomy, Journal of Nannoplankton Research Special Issue, 1, 1–125, ISSN 1210-8049, 2003.
Young, J. R., Poulton, A. J., and Tyrrell, T.: Morphology of Emiliania huxleyi coccoliths on the northwestern European shelf – is there an influence of carbonate chemistry?, Biogeosciences, 11, 4771–4782, https://doi.org/10.5194/bg-11-4771-2014, 2014.
Zhang, Y., Fu, F., Hutchins, D., and Gao, K.: Combined effects of CO2 level, light intensity, and nutrient availability on the coccolithophore Emiliania huxleyi, Hydrobiologia, 842, 127–141, https://doi.org/10.1007/s10750-019-04031-0, 2019.
Zingone, A., Sarno, D., Siano, R., and Marino, D.: The importance and distinctiveness of small-sized phytoplankton in the Magellan Straits, Polar Biol., 34, 1269–1284, https://doi.org/10.1007/s00300-010-0937-2, 2011.
Ziveri, P., Bernardi, B., Baumann, K.-H., Stoll, H., and Mortyn, G.: Sinking of coccolith carbonate and potential contribution to organic carbon ballasting in the deep ocean, Deep-Sea Res., 54, 659–675, https://doi.org/10.1016/j.dsr2.2007.01.006, 2007.
Zondervan, I., Zeebe, R., Rost, B., and Riebesell, U.: Decreasing marine biogenic calcification: A negative feedback on rising atmospheric pCO2, Global Biogeochem. Cy., 15, 507–516, https://doi.org/10.1029/2000GB001321, 2001.