the Creative Commons Attribution 4.0 License.
the Creative Commons Attribution 4.0 License.
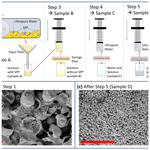
Isolation of subpollen particles (SPPs) of birch: SPPs are potential carriers of ice nucleating macromolecules
Julia Burkart
Jürgen Gratzl
Teresa M. Seifried
Paul Bieber
Hinrich Grothe
Within the last years pollen grains have gained increasing attention due to their cloud-forming potential. Especially the discovery that ice nucleating macromolecules (INMs) or subpollen particles (SPPs) obtained from pollen grains are able to initiate freezing has stirred up interest in pollen. INMs and SPPs are much smaller and potentially more numerous than pollen grains and could significantly affect cloud formation in the atmosphere. However, INMs and SPPs are not clearly distinguished. This has motivated the present study, which focuses on birch pollen and investigates the relationship between pollen grains, INMs, and SPPs. According to the usage of the term SPP in the medical fields, we define SPPs as the starch granules contained in pollen grains. We show that these insoluble SPPs are only obtained when fresh pollen grains are used to generate aqueous extracts from pollen. Due to the limited seasonal availability of fresh pollen grains, almost all studies have been conducted with commercial pollen grains. To enable the investigation of the SPPs we develop an alternative extraction method to generate large quantities of SPPs from commercial pollen grains. We show that INMs are not bonded to SPPs (i.e. can be washed off with water). Further, we find that purified SPPs are not ice nucleation active: after several times of washing SPPs with ultrapure water the ice nucleation activity completely disappears. To our knowledge, this is the first study to investigate the ice nucleation activity of isolated SPPs. To study the chemical nature of the INMs, we use fluorescence spectroscopy. Fluorescence excitation–emission maps indicate a strong signal in the protein range (maximum around λex = 280 nm and λem = 330 nm) with all ice nucleation active samples. In contrast, with purified SPPs the protein signal is lost. We also quantify the protein concentration with the Bradford assay. The protein concentration ranges from 77.4 µg mL−1 (highly concentrated INMs) to below 2.5 µg mL−1 (purified SPPs). Moreover, we investigate the connection between proteins and ice nucleation activity by treating the ice nucleation active samples with subtilisin A and urea to unfold and digest the proteins. After this treatment the ice nucleation activity clearly diminished. The results indicate a linkage between ice nucleation activity and protein concentration. The missing piece of the puzzle could be a glycoprotein which exhibits carboxylate functionalities, can bind water in tertiary structures, and displays degeneration and unfolding of its secondary structure due to heat treatment or reaction with enzymes. Even though purified SPPs are not ice nucleation active they could act as carriers of INMs and distribute those in the atmosphere.
- Article
(4480 KB) - Full-text XML
-
Supplement
(458 KB) - BibTeX
- EndNote
Aerosol particles in the atmosphere are climatically relevant if they affect cloud microphysics and formation of precipitation or directly scatter solar radiation. The aerosol effect on clouds is still one of the largest uncertainties in our climate system (Boucher, 2013), and the role of bioaerosols including pollen is far from being understood (Fröhlich-Nowoisky et al., 2016). Pollen are a subset of bioaerosols such as bacteria, viruses, algae, fungi, and plant debris found abundantly in the atmosphere during the blooming season of plants. Pollen is the male gametophyte that contains vegetative and generative cells essential for the fertilization and reproduction of a plant. In particular, wind pollinated plants such as early flowering trees (e.g. birch) and grasses produce pollen in large quantities and rely on wind for dispersal and transportation to the female plant part, where fertilization occurs. Pollen are thus distributed in the lower atmosphere and can also travel long distances when meteorological conditions are favourable (Damialis et al., 2017; Sofiev et al., 2012; Skjøth et al., 2007). Typical concentrations of pollen grains of a specific type range from 10 m−3 to a few hundred or even thousand per cubic metre on a daily average. Peak concentrations in the vicinity of plants can assume a few hundred thousand per cubic metre (Jochner et al., 2015; Šikoparija et al., 2018). Forests of the Northern Hemisphere are estimated to be large contributors to atmospheric pollen concentrations; e.g. birch pollen concentrations outside the canopy of birch forests are in the range of 10 000 m −3 during spring (Williams and Després, 2017). The ability of pollen to induce liquid droplet and ice formation has been documented in several studies already years ago. Entire pollen grains were shown to act as giant cloud condensation nuclei (CCN) (Pope, 2010) and also as ice nucleating particles (INPs) (Diehl et al., 2002, 2001; von Blohn et al., 2005). A summary of the role of pollen and other biological particles in cloud physics is given by Möhler and colleagues (Möhler et al., 2007). However, due to their large sizes (10–100 µm) leading to short residence times in the atmosphere, their impact on cloud microphysics was estimated to be negligible on a global scale (Hoose et al., 2010a, b).
Only discoveries of recent years have demonstrated that not only does the entire pollen grain have the ability to initiate ice formation, but the presence of macromolecules originating from pollen grains is sufficient to induce heterogeneous ice nucleation (Pummer et al., 2012, 2015). In their study, Pummer and co-workers (Pummer et al., 2012) suspended entire birch pollen grains in water for several hours. The solution was then decanted and filtrated yielding what is called pollen washing water. The washing water is shown to induce ice formation at similar temperatures to the entire pollen grains. The ice activity of pollen grains is thus attributed to the existence of ice nucleating macromolecules (INMs) that can be separated from pollen grains (Pummer et al., 2015). Such INMs can also be obtained from other parts of a plant, e.g. branches, leaves, and barks of a birch (Felgitsch et al., 2018). Aqueous extracts of pollen grains obtained in a similar manner were used in several other studies to investigate the cloud-forming potential of many different types of pollen grains (Gute and Abbatt, 2020; Gute et al., 2020; Mikhailov et al., 2019; O'Sullivan et al., 2015; Steiner et al., 2015). In these studies, such aqueous extracts are often referred to as subpollen particles (SPPs). The term SPP originates in the medical sciences and is commonly used to describe starch granules with allergenic potential that are contained in pollen grains (Bacsi et al., 2006; Schäppi et al., 1999; Sénéchal et al., 2015). The term starch granule refers to the main component starch, which is a polysaccharide and functions as an energy storage unit in plant cells (Baker and Baker, 1979; Buléon et al., 1998; Hancock and Tarbet, 2000). In studies related to atmospheric sciences, the term is used in a less defined and sometimes confusing way. Aqueous extracts of pollen grains are simply referred to as SPPs even though they might not contain any particulate material, and particles created artificially by atomizing solutions of aqueous pollen extracts are denoted SPPs.
A recent model simulation estimates that the presence of SPPs in the atmosphere could suppress precipitation in clean continental clouds by about 30 % (Wozniak et al., 2018). However, estimations of the amount of such material in the atmosphere is based on rough assumptions since data are missing. One reason for the lack of data is that little is known about the nature and composition of INMs, and, as a consequence, direct measurement methods that can identify INMs in the atmosphere are not available. To date only a few studies investigated the chemical nature of INMs and found that INMs contained in birch pollen washing water are water soluble and are likely composed of polysaccharides (Dreischmeier et al., 2017; Pummer et al., 2012, 2015). However, there is also evidence that the ice nucleating activity stems from proteinaceous substances; e.g. Tong and colleagues show that the ice activity of birch washing water diminishes if proteinaceous components are extracted (Tong et al., 2015).
One major difficulty in identifying INMs is related to the complexity of a pollen grain. In fact, a pollen grain is a highly complex particle composed of different parts and materials (Fægri and Iversen, 1992). Figure 1 illustrates a birch pollen grain and its main parts. The actual cell is protected by a robust outer shell made of two layers: the exine (outer layer) and the intine (inner layer). The exine is composed of the mechanically resistant and chemically inert biopolymer sporopollenin, while the intine, composed of cellulose and pectin, is a more fragile membrane. The cell is filled with cytoplasmic material including proteins, lipids, and polysaccharides such as starch. Many pollen types, such as birch pollen grains, also contain pores. Pores are spots where the exine is missing and thus give access to the cell membrane (intine). Under humid conditions most fresh pollen grains are likely to expel cytoplasmic material, a process often referred to as pollen rupture. In the literature two mechanisms are documented by which pollen grains can release cytoplasmic content including starch granules from their interior. First, entire pollen grains can rupture by osmotic shock during hydration (D'Amato et al., 2007; Suphioglu et al., 1992; Taylor and Jonsson, 2004). Second, pollen grains can be stimulated to germinate and grow pollen tubes (Grote et al., 2003; Schäppi et al., 1997). The pollen tubes rupture at their tips just as they would during fertilization on a female stigma and expel genetic and cytoplasmic material. This process is referred to as abortive germination (Grote et al., 2003). In a more recent laboratory study Sénéchal and co-workers showed that SPPs are also released from birch and cypress pollen after the grains were exposed to humidity or to NO2 (Sénéchal et al., 2015). Mechanical rupture caused by wind-induced impaction might also generate SPPs (Visez et al., 2015).
These processes have since been examined in several studies from the medical fields, e.g. Grote et al. (2003), Schäppi et al. (1997), Staff et al. (1999), Suphioglu et al. (1992), and Taylor et al. (2004). Pollen rupture is hypothesized to occur in the atmosphere and offers an explanation of the presence of allergens in the fine aerosol fraction (< 5 µm), i.e. the presence of allergens detached from pollen grains. For example, birch pollen grains were shown to germinate on leaves after light rain and release starch granules (Schäppi et al., 1999). Simultaneously a rise in the allergen concentration in the air was measured without the presence of pollen grains (Schäppi et al., 1999, 1997). SPPs are also hypothesized to cause the phenomenon of thunderstorm asthma (Taylor et al., 2007; Taylor and Jonsson, 2004). Thunderstorm asthma is the observed coincidence of severe asthma epidemics and thunderstorms (e.g. Thien, 2018). Due to their large size, pollen grains are efficiently trapped in the upper airways when inhaled. In contrast, SPPs can penetrate deep into the lungs and trigger asthma. Cases of thunderstorm asthma have been documented worldwide (D'Amato et al., 2019). An increase in submicron particles of biological origin possibly connected to pollen grains during rain events or thunderstorms has also been observed in a few recent studies not related to allergens that use chemical tracers and measurements of fluorescence particles as an indication for biological particles possibly originating from pollen grains (Hughes et al., 2020; Rathnayake et al., 2017; Huffman et al., 2013).
In this study, we investigate SPPs of birch pollen grains. The aim of our study is to shed light on the differences between INMs and SPPs. As discussed above, in atmospheric literature the term SPP is usually used in a rather unspecific sense to simply describe the remaining materials when aqueous extracts of pollen grains are generated. For the purposes of our study, we follow the usage of SPPs as done in medical literature. Purified SPPs are composed of starch and are contained in the pollen grains as energy storage units. Starch is a polysaccharide made of amylose and amylopectin (Hancock and Bryon, 2000; Buléon et al., 1998) inside the amyloplast membrane (Matsushima et al., 2016). Accordingly, we define SPPs as the starch granules contained in the cytoplasm. With the extraction methods commonly used and predominantly applied to commercial pollen grains, SPPs are not obtained. We emphasize that commercial pollen grains do not rupture and do not expel SPPs. We therefore develop a method to extract SPPs and separate those from other cytoplasmic materials. We thereby aim to answer the question of whether SPPs are ice nucleation active and if INMs are linked to SPPs. To our knowledge this is the first study to investigate thoroughly the ice nucleating activity of SPPs extracted from pollen grains. Additionally, we use fluorescence spectroscopy to gain chemical information about the ice active substances and the Bradford assay to quantify protein concentrations. To clarify the role of proteins in heterogeneous freezing we conducted a specific enzymatic digestion and protein unfolding experiment.
2.1 Pollen samples
We used commercially purchased birch pollen samples. The samples were purchased from ThermoFisher Scientific Allergon and are specified by the company as pollen from Betula pendula (common name: silver birch). Pollen are collected from trees in southern Sweden and are carefully purified from other plant materials after sampling. The pollen sample is reported to contain less than 2 % of plant parts other than pollen grains. At the time of purchase the birch pollen grains were already 1 year old. Freshly harvested pollen samples were collected from a birch tree at the Donau Kanal (location birch tree: 48.237480, 16.362990) in Vienna. Collection took place on 9 April 2021, when birch trees were reported to be ready to bloom by the Austrian pollen forecast service (https://www.pollenwarndienst.at, last access: 9 April 2021). Additionally, phenological observations at the sampling site were performed (i.e. check for clearly visible anthers on the catkins) to confirm the maturity of the pollen grains.
2.2 Extraction of SPPs
In order to extract SPPs of birch pollen grains we develop an extraction process that starts with cracking the pollen grains so that the cytoplasmic content is available even without the process of pollen rupture. Fresh birch pollen grains naturally rupture when soaked with water and release their cytoplasmic content including starch granules. With commercially purchased pollen this ability is almost lost due to ageing and desiccation of pollen and the loss of viability. Therefore, we use a mixer mill (MM400, Retsch GmbH, Haan, Germany) to crush the grains. The extraction process is illustrated in Fig. 2. Crushing the pollen grains with the mixer mill is the first step of the extraction method (Fig. 2a, step 1). In order to crush the grains with the mixer mill we blend 0.5 g pollen grains with 2 mL ultrapure water (Milli-Q, 18.2 MΩ) and pour the water pollen suspension into the grinding jar including one single ball as a grinder. The mixer mill is operated for 1 min at 25 Hz (step 1). As a result, 30 %–40 % of the pollen grains are cracked, and cytoplasmic material and SPPs are gained (see Fig. 2b). It should be noted that the pollen wall cracks but does not fragment into very small pieces (below ∼ 10 µm). In fact, we find very few small wall fragments after grinding (analysed in electron microscopic pictures). Apart from the SPPs, the cytoplasmic content appears soluble, forming amorphous structures when dried.
In a second step, we fill up the suspension to a total volume of 50 mL and use filter paper with 10 µm pore size to filter pollen wall fragments and the remaining undamaged pollen grains. Particulate material larger than 10 µm is retained by the filter, while all other materials pass through. The resulting extraction product is referred to as sample A. Sample A contains SPPs, other cytoplasmic materials (mostly soluble), and a few small wall fragments.
In step 3, sample A is filtrated with a syringe filter (0.2 µm pore size, Nylon membrane, sterile); i.e. particles larger than 0.2 µm are retained by the filter. Sample B refers to the solution without SPPs. In step 4, the filter with the remaining material is rinsed with ultrapure water until most of the soluble material is washed off. The rinsing is done stepwise with 1 to 70 mL ultrapure water. After passing through the filter the filtrate is called sample C. The ice nucleation activity of each sample C fraction was tested at each rinsing step (note that the rinsing water was not pooled). As the purpose of this extraction was to test if INMs can be separated from SPPs, rinsing was continued as long as sample C was ice nucleation active. Rinsing was stopped after 70 mL when the ice nucleation activity of sample C had disappeared.
After rinsing, the filter is reversed and flushed with ultrapure water (step 5). A suspension of SPPs in ultrapure water is obtained. This sample is referred to as sample D (Fig. 2c) and was used to test the ice nucleation activity of SPPs.
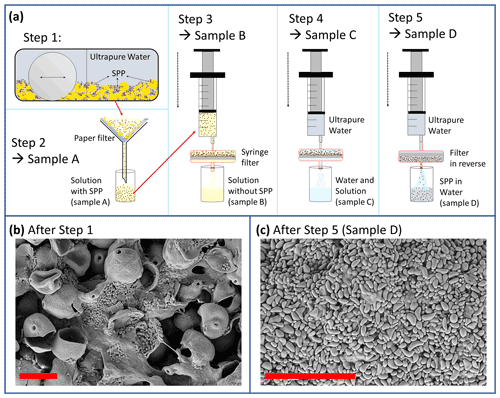
Figure 2(a) Extraction process of SPPs. Step 1: entire pollen grains mixed with ultrapure water are crushed in a mixer mill (MM400, Retsch GmbH, Haan, Germany). Step 2: the sample is filtered so that entire pollen grains and large outer shells are excluded. Step 3: filtration with a 0.2 µm pore sized filter. Step 4: the filter including the SPPs is rinsed with ultrapure water to wash off soluble material. Step 5: the filter is reversed and the SPPs are extracted with ultrapure water. (b) Crushed pollen grains, cytoplasmic material, and SPPs (starch granules) after step 1. (c) Extracted SPPs after step 5. Red scale bar is 20 µm.
2.3 Determination of the geometric size distribution
The geometric size of the SPPs was determined from images taken with a field emission gun scanning electron microscope (FEG-SEM; Zeiss Supra 55 VP). The shape of the SPPs was approximated by a cylinder capped with hemispheres at each side with the diameters of the hemispheres being equal to the width of the cylinder (Fig. 3). The width s and length l of the cylinder were measured manually with the help of image analysis software (SmartTIFF V1.0.1.2), and the volume was calculated. This was done for 326 particles in total. The volume was then used to determine the volume equivalent diameter of the SPPs, i.e. the diameter of a sphere having roughly the same volume as the irregular-shaped SPPs. Based on the approximated shape the volume equivalent diameter dv was calculated.
Additionally, the aspect ratio was used to describe the sphericity of the particle. The aspect ratio is defined as width/length and is 1 for spherical particles and smaller than 1 for elongated particles.
2.4 Ice nucleation measurements
INM content from birch pollen was quantified in immersion freezing mode by using the Vienna Optical Droplet Crystallization Analyzer (VODCA) set-up. A detailed description of the experiment is given in Felgitsch et al. (2018), Zolles et al. (2015), and Pummer et al. (2012): an emulsion of 2 µL sample solution and 4 µL inert oil mixture (10 wt % lanolin, anhydrous, VWR Int., Radnor, PA, USA; 90 wt % paraffin, light, pure grade, AppliChem GmbH, Darmstadt, Germany) is prepared by mixing with a pipette tip on a microscopic glass slide and transferred into a cryo-cell. Sample emulsions are cooled at a rate of 10 ∘C min−1. The freezing process was monitored by videos at four different spots of each sample glass slide via a microscope camera (MDC320, Hengtech, Germany). On each spot about 20 droplets in the corresponding size range are observed. The fraction of frozen droplets () was plotted as a function of the temperature. Furthermore, the cumulative nucleus concentration (CNC) was determined using Eq. (2) (Vali, 1971):
where nfrozen is the number of frozen droplets at the given temperature, ntotal the total number of droplets, V the droplet volume, and d the dilution factor. CNC(Ti) indicates the number of INMs per unit volume actively present above the temperature Ti. Note that one ice nucleus can also be an aggregate of more than a single molecule (Qiu et al., 2019). To compare the values of INMs from different samples we chose Ti at −25 ∘C, since most biogenic INMs are active above that temperature (Kanji et al., 2017; Murray et al., 2012; Pummer et al., 2015) and −34 ∘C since homogeneous ice nucleation starts at −35 ∘C with the VODCA set-up. Thus, the CNC(−34 ∘C) value includes all heterogeneous freezing events. Highly concentrated samples were diluted in the experiment to prevent underestimation of INM contents, and the dilution factor (d) was included in Eq. (2) (see Vali, 1971). Only droplets with a diameter between 15 and 40 µm (droplet volume: 1.8–34 pL) are counted in the evaluation, and the average volume of 8.2 pL was used to calculate CNC values. The selected size range is relevant for atmospheric cloud droplets. The temperature uncertainty of VODCA is 0.5 ∘C (Zolles et al., 2015). Furthermore, we calculated the counting error and performed a Gauss error propagation as described in Kunert et al. (2018). Accordingly, data points with errors larger than 100 % were excluded in the graphs.
2.5 Fluorescence spectroscopy
Autofluorescence active materials such as SPPs and respective extracts (see Fig. 2) were characterized by fluorescence spectroscopy using a FSP920 spectrometer (Edinburgh Instruments, UK), equipped with a Xe900 xenon arc lamp (450 W) and a S900 single photon photomultiplier. Sample solutions were measured in a quartz glass cuvette (500 µL, Hellma™ Suprasil™ quartz, Germany) using a designated cuvette optic. The excitation light beam is arranged in a 90∘ angle relative to the detector. The software F900 allowed excitation–emission maps to be recorded (excitation from 220 to 400 nm, emission from 320 to 500 nm). The excited state was held for 0.25 s dwell time, and step width of the monochromators was set to 2 nm. To avoid first- and second-order excitation (Fig. 7, grey area), we used a 295 nm low-pass filter (Stablife Technology®, Newport, USA) and an offset of 10 nm. Two samples (sample A and B; see Fig. 2) were highly fluorescent active and were thus diluted during the measurements to minimize the influence of quenching agents. The excitation–emission maps obtained were normalized to 5.0 × 104 counts.
2.6 Quantitative protein analysis
The protein concentration of the sample solutions was determined by the common protein assay first described by Marion M. Bradford (Bradford, 1976). The method is based on a protein–dye binding reaction. The dye reagent Coomassie brilliant blue forms a complex with proteins in solutions and shifts the absorption maximum from 465 to 595 nm. A standard curve ranging from 2.5 to 25 µg mL−1 was prepared by diluting an albumin standard (Thermo Scientific, Germany) with ultrapure water. Samples A and B (see Sect. 2.2) were diluted 1:5 since their concentration was out of test specifications. We pipetted 150 µL of each sample, standard and blank (ultrapure water), directly onto a microtiter microplate (MaxiSorp, Nunc-Immuno, Thermo Scientific, Germany). By using a stepper pipette, 150 µL of Coomassie reagent (Thermo Scientific, Germany) was added to each cavity. The plate was shaken with a microplate shaker (PMS-1000i, Grant Instruments, UK). After incubating for 10 min at room temperature, we measured the absorbance at 600 nm with a photometer (Sunrise, Tecan, Switzerland). For evaluation, the average blank value was subtracted from the absorption values. For the standard curve a linear regression was determined, and the total protein content of the sample was calculated.
2.7 Enzymatic and chemical treatment
Sample B was treated with (1) subtilisin A, (2) urea, and (3) a mixture of subtilisin A and urea. The enzyme subtilisin A is a protease, which belongs to the Serine S8 Endoproteinase family (Hedstrom, 2002). It denatures proteins with a broad specificity by hydrolyzing peptide bonds (Rawlings et al., 2010). Highly concentrated urea, in contrast, is a chaotropic reagent which does not cut covalent bonds but unfolds proteins by weakening the hydrogen bond system.
First, a 100 mM Tris buffer (Sigma Aldrich, 252859, St. Louis, MO, USA) with a pH of 8.4 (adjusted with 2 M HCl) was prepared. Subtilisin A was dissolved in the Tris buffer with a resulting concentration of 2 mg mL−1. Further, we prepared a 8 mol L−1 urea solution (Sigma Aldrich, St. Louis, MO, USA) using the buffer as a solvent. For the incubation, we prepared four samples: 50 µL of sample B was mixed with (1) 210 µL Tris buffer (serving as a control sample to monitor whether temperature changes the ice nucleation activity, INA), (2) 10 µL subtilisin A and 200 µL Tris, (3) 10 µL Tris and 200 µL urea, and (4) 10 µL subtilisin A and 200 µL urea. All samples had a total volume of 260 µL resulting in a Tris buffer concentration of 81 mmol L−1 and 6.2 mol L−1 urea. The samples were incubated at 37 ∘C (Heiz-Thermoshaker, Thermo Scientific, Waltham, WA, USA); after 1 and 6 h, 2 µL sample aliquots were taken and diluted 1:10 with ultra pure water prior to ice nucleation measurements to decrease the freezing point depression of the buffer and urea. Chemicals used in the treatment did not show heterogeneous INA.
3.1 Extraction process and size distribution of SPPs
The extraction process in this study differs from the usual approach in other studies (e.g. Gute and Abbatt, 2020; Pummer et al., 2012; Steiner et al., 2015) especially in one aspect: commercial or stored pollen grains do not germinate or rupture and therefore do not release insoluble SPPs (starch granules) contained in the cytoplasm. In contrast, we observe that fresh pollen grains directly shed from catkins germinate and rupture when immersed in water or exposed to high relative humidity (> 95 %) for several hours. This process has also been documented in the literature (Grote et al., 2003). To ensure that insoluble SPPs and material from inside the pollen grains are obtained we first crack the exine of the pollen grains. This is done with a mixer mill. As seen in Fig. 2b the exine cracks and gives access to the pollen grain's interior including the starch granules. This step was necessary to mimic the natural behaviour of fresh pollen grains. Figure 4 shows fresh pollen grains that had been exposed to high relative humidity (> 95 %) for 8 h. The behaviour of fresh pollen grains immersed in water is also illustrated in the linked video (https://doi.org/10.5281/zenodo.5550102). In both cases particulate, insoluble material can be clearly observed. These insoluble SPPs are likely to spread in the atmosphere due to pollen rupture or abortive germination (Schäppi et al., 1999; Grote et al., 2003; Taylor et al., 2004; Hughes et al, 2020). With fresh birch pollen we find that both processes take place: pollen rupture (video) and abortive germination (Fig. 4). Note that most of the insoluble SPPs in Fig. 4 are coated with amorphous material that is expelled by the pollen grain at the same time as the SPPs. Only a few SPPs seem “pure” (the interpretation, however, is limited by the resolution of the electron microscope). Most of the amorphous material probably originates from the cytoplasm, but we cannot exclude that some of the material is also washed off the exine, i.e. the pollen grain surface. We find that the ability to rupture is almost entirely lost when freshly harvested birch pollen grains were stored in the lab for a few days to weeks. The highest germination activity (i.e. most pollen grains germinated) was observed when fresh pollen grains were exposed to water on the very same day they were harvested. With commercially purchased pollen grains we did not find any germination activity and also no SPPs. In addition, we also treated pollen grains mixed with water up to 1 h in the ultrasonic bath to see if pollen rupture could be induced this way. However, even after 1 h of ultrasonic treatment we did not find any ruptured pollen grains or SPPs (Fig. S1 in the Supplement). We emphasize that the usually applied extraction methods, in which pollen grains are only left in water and are then filtrated, do not actually yield SPPs unless very fresh pollen grains are used. The usual extraction method likely yields only the most soluble components. For example, it is known that some highly soluble proteins (mostly allergenic ones) migrate to the pollen surface within seconds to minutes upon hydration even without pollen rupture (e.g. Vrtala et al., 1993). The exine of birch pollen contains microchannels that enable such an exchange (Diethart et al., 2007). Another study also documents the passage of proteins contained in the cytoplasm through the intact cell membrane (Hoidn et al., 2005). While insect-pollinated plants produce pollen with a thick lipid-containing pollenkitt (coating of the exine) that functions as a barrier to water soluble components, this pollenkitt is almost entirely absent with pollen from wind-pollinated plants such as birch pollen (Diethart et al., 2007). Our extraction method is unique as it guarantees access to less soluble substances and might be a closer approximation to the processes in the atmosphere than the usual applied extraction method. Our method also offers the possibility to study the ice nucleation activity of isolated SPPs for the first time and to investigate whether INMs are connected to SPPs.
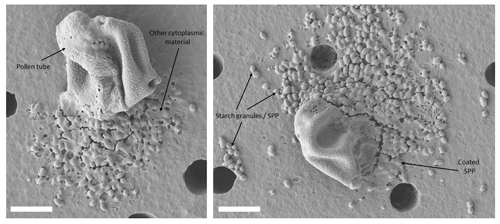
Figure 4Germinated and ruptured pollen grains. Freshly harvested birch pollen grains were exposed to high relative humidity. Pollen grains were deposited on a polycarbonate nuclepore filter (pore size: 8 µm) within an inline filter holder, and humid air (95 % relative humidity) was sucked through for 8 h. This process can only be observed with fresh pollen grains. Commercial pollen grains remained intact after the same treatment. White scale bar is 10 µm.
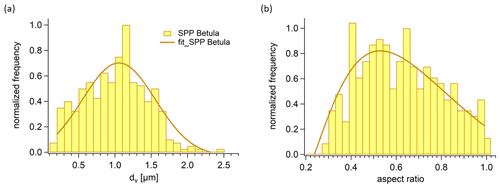
Figure 5(a) Size distribution of SPPs. The volume equivalent diameter dv was calculated by Eq. (1), which is based on the approximated shape of an SPP. The dv of 326 SPPs was binned into 24 equidistant intervals between 0.2 and 2.5 µm and normalized to the interval with the maximum number of SPPs. (b) Aspect ratio of SPPs.
We also analysed the geometric size of the SPPs gained by our extraction method and calculated volume equivalent diameters (Fig. 3, Eq. 1). The volume equivalent diameters of SPPs range from 0.2 to 2.5 µm and are roughly normally distributed (Fig. 5a). The mean value of the distribution, as well as the maximum of the gauss fit, is about 1.1 µm. To describe the sphericity of the SPPs the aspect ratio was calculated. It ranges from 0.27 to 1 in which 1 describes a perfect sphere. The aspect ratio (Fig. 5b) is roughly log-normal distributed with a maximum at about 0.5, confirming that most SPPs have a rather elongated form as can be seen in Fig. 2c. In fact, the length of the largest SPPs reaches up to 4 µm. Considering a density of 1.6 g m−3 for SPPs (Dengate et al., 1978) and neglecting the non-spherical shape, the aerodynamic diameter would be a factor of 1.26 larger than the volume equivalent diameter. The aerodynamic diameter is essential to describe the behaviour of particles in the atmosphere. With this rough estimation we can conclude that the aerodynamic diameter of the SPPs ranges from 0.25 to 3.2 µm. It should be noted that we used a filter with 0.2 µm pore size and that smaller particles might have been lost in the extraction process.
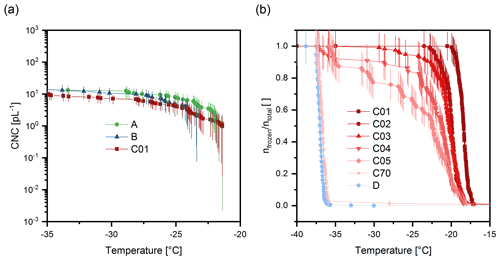
Figure 6Ice nucleation spectra of extracted samples. (a) Cumulative ice nucleus spectra of samples A, B, and C01. Error bars correspond to the Gauss error propagation. (b) Freezing spectra of SPP washing solutions (C01, 1 mL water used to wash SPPs, C02, second 1 mL fraction, etc.) and of the purified SPPs (D). Vertical lines correspond to the counting error. The temperature uncertainty of VODCA is 0.5 ∘C.
3.2 Ice nucleation activity
We analysed the ice nucleation activity of all samples obtained during the SPP extraction process (sample A, B, C, and D; see Fig. 2). The goals of these measurements were (1) to gain information about the location of the INMs within the pollen grain and (2) to investigate whether SPPs are ice nucleation active.
In step 4, the syringe filter was rinsed with up to 70 mL of ultrapure water. Rinsing was continued until the ice nucleation activity of the rinsed material (sample C) was completely lost; i.e. only homogeneous freezing occurred at temperatures below −35 ∘C. Here, the rinsing steps are labelled C01 to C70. The number indicates the water volume in millilitres used to rinse the sample up to this step; i.e. C01 and C70 mean that the sample had been rinsed in total with 1 mL and 70 mL of ultrapure water, respectively. We analysed the ice nucleation activity of all C01 to C70 samples obtained during the washing process. Freezing curves of all samples are shown in the Supplement (Fig. S2). Fig 6 shows selected samples only to avoid an overcrowded figure. The freezing curves follow the typical pattern (inclining curves and formation of a plateau) that results from diluting an ice active substance (Felgitsch et al., 2018). Samples A, B, and C01 clearly exhibit heterogeneous ice nucleation. The concentration of INMs active above −34 ∘C was 13.2 pL−1 for sample A, 13.1 pL−1 for sample B, and 8.7 pL−1 for sample C01 (Fig. 6a). After sample C10 the ice nucleation activity rapidly diminishes, but only after 70 mL of washing was homogeneous freezing the dominant process in the experiment (99 % of the observed droplets froze homogeneously). After the last washing step, purified SPPs (sample D) were obtained by reversing the syringe filter and flushing the filter with ultrapure water. Sample D did not show heterogeneous ice nucleation activity. As losses of SPPs in the syringe filter are expected during the washing process we also conducted a control experiment, in which we generated a sample with highly concentrated SPPs. In the control experiment we stopped the washing process after 30 mL and further separated SPPs from solutes via centrifugation (1000 rcf, 3 min). A picture of the concentrated SPP precipitation is shown in the Supplement (Fig. S3). After several steps of centrifugation and cleaning the precipitate with ultrapure water, even the accumulated SPPs did not exhibit ice nucleation activity (see Supplement, Fig. S4). The result indicates that purified SPPs are not ice nucleation active. The results thereby show that INMs can be separated from SPPs and are not tightly attached to the SPPs. However, based on our experiments we cannot make a definite statement on the type of bonding.
3.3 Chemical analytics
In order to gain information about the chemical nature of the INMs we analysed the extracted samples using fluorescence spectroscopy. Since we found that the starch contained in pollen grains is not ice active, we focused this analysis on the excitation–emission wavelength range where proteins are expected. Biological ice nucleation is often linked to the presence of proteins acting as INPs (Maki et al., 1974; Wilson et al., 2006). The amino acids tryptophan, tyrosine, and phenylalanine, which are present in natural proteins, contain excitable π electrons. Thus, proteins show auto-fluorescence when excited at around 280 nm with a Stokes shift of about 50 nm (Pöhlker et al., 2012). In Fig. 7a–c fluorescence excitation–emission maps of our samples show high intensity at λex 280 nm and λem 330 nm. This maximum clearly indicates the presence of proteins. The fluorescence intensity at the maximum decreases with decreasing CNC (Table 1). Furthermore, we did not see any signal in this range for purified SPPs (Fig. 7d), leading us to the conclusion that no proteins are detectable in that final fraction anymore. The thin line still visible in Fig. 7d is the Raman signal of water. We conclude that the ice nucleation of our samples might be linked to proteins, whereas the SPPs mainly composed of starch are not ice nucleation active.
Table 1Protein concentration and cumulative nuclei concentration (CNC). LOQ = limit of quantification.
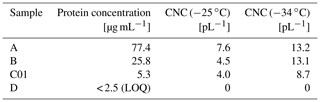
Even though proteins can show strong fluorescence signals, light absorbing substances in the extract might lead to quenching effects (Papadopoulou et al., 2005) that decrease the protein signal. More specific measurements of protein content can be carried out using UV–visible spectroscopy after staining the proteins with Coomassie brilliant blue (Bradford assay; Bradford, 1976). Quantification via Bradford assay gave protein concentration of 77.4 µg mL−1 for sample A, 25.8 µg mL−1 for sample B, and 5.3 µg mL−1 for C01. Values for sample D were lower than the limit of quantification (2.5 µg mL−1). A summary of the determined values for samples analysed is given in Table 1. Comparing the protein concentration to CNC(−25 ∘C), representing the biological region (Kanji et al., 2017; Murray et al., 2012; Pummer et al., 2015), shows a general trend: a higher protein concentration coincides with higher CNC(−25 ∘C) values. For lower temperatures (i.e. CNC(−34 ∘C)) the dependency on the protein concentration is less pronounced since non-proteinaceous materials become ice nucleation active in the temperature window between −25 and −34 ∘C.
To further test the hypothesis whether proteins play a role in the ice nucleation activity we conducted a protein unfolding and enzyme digestion experiment using subtilisin A and urea. Treating ice nucleation active samples with enzymes (e.g. Kozloff et al., 1991; Pummer et al., 2012; Felgitsch et al., 2019), chaotropic reagents (e.g. Pummer et al., 2012; Fröhlich-Nowoisky et al., 2015), or a strong oxidizer (e.g. H2O2; Gute et al., 2020) to investigate the nature of ice nuclei has been performed in the past. The experiment conducted in this paper is inspired by a publication from Felgitsch et al. (2019) in which they investigated the role of proteins in ice nucleation active extracts from perennial plants.
Results show that after incubating sample B for 1 h at 37 ∘C, the ice nucleation activity of samples containing urea decreased. For urea alone, 65 % of droplets remained to freeze heterogeneously (see Fig. 8a). However, treating the sample with the combination of subtilisin A and urea led to an even stronger decrease in INA due to the unfolding activity of urea (50 % of droplets froze heterogeneously). Sample B in Tris (control sample) and the digestion with subtilisin A alone did not show a significant decrease in heterogeneous freezing activity after 1 h treatment. However, after 6 h incubation time, subtilisin A seems to slightly influence the INA, and the freezing curve is dropping at around −22 ∘C (see Fig 8b). Nevertheless, the urea treatment decreased the ice nucleation ability even more. Further, the strongest influence of INA is clearly derived from the mixture of urea and subtilisin A. Again, this can be explained by the unfolding effect of urea in combination with the cleavage of peptide bonds by subtilisin A. In addition, the freezing onset temperatures of samples containing urea are shifted approx. 2 ∘C towards colder temperatures. This phenomenon is attributed to the freezing point depression induced by urea, which is also visible in the homogenous freezing regime. The sensitivity of the sample to urea treatment indicates that proteins play a role in the INA. Unfolding using urea as a reagent and further cutting peptide bonds decreases the INA. This suggests that the secondary and primary structure is important for the proteins to act as INMs.
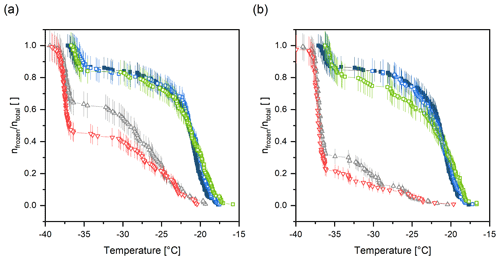
Figure 8Results of the unfolding experiment and enzymatic digestion of extracted INMs (sample B) at 37 ∘C using subtilisin A from Bacillus licheniformis and urea after (a) 1 h and (b) 6 h incubation times. Filled, blue squares correspond to sample B in Tris buffer at room temperature prior to treatment and hollow, blue squares to the control sample. Green circles show the treatment with subtilisin A and grey triangles (cone up) with urea, and red triangles (cone down) represent the treatment using a mixture of subtilisin A and urea. Vertical lines represent the counting error.
However, in the literature only Tong et al. (2015) deal with proteins as INMs in birch pollen. Still, the exact chemical composition of the INMs from primary biological aerosol particles (PBAPs) is a matter of controversial discussion. When Pummer et al. (2012) discovered that ice nuclei are water soluble and can be extracted from pollen, they proposed that polysaccharides are the responsible moieties. This was supported by infrared and Raman spectra and by size filtration experiments pointing to INMs larger than 100 kDa bearing carboxylate groups (Pummer et al., 2013; Dreischmeier, 2017). INMs with similar physical and chemical properties were also extracted from other PBAPs such as fungi (Haga et al., 2013, 2014; Kunert et al., 2019). This led Hiranuma et al. (2015,2019) to the conclusion that cellulose, which is a polysaccharide and part of PBAP cell walls, might be responsible for the INA. But also for other polymers such as lignin and nanogels, which can take up water in their structures as well, a similar ice nucleation activity as for cellulose was found (Bogler and Borduas-Dedekind, 2020; Xi et al., 2021). Structure and size are also crucial for bacterial ice nucleation proteins, in which repeat numbers (similar sequence patterns) and oligomerization contribute in a seemingly independent manner to the nucleation mechanism (Ling et al., 2018; Šantl-Temkiv, 2015). In general, INMs in the atmosphere are manifold and found in different sources such as forests, deserts, and marine environments (Lloyd et al., 2020).
In summary, our experiments come to a similar conclusion as Tong et al. (2015) pointing in the direction of proteins but are not a priori in contradiction to other recent literature. The missing piece of the puzzle could be that a glycoprotein, which exhibits carboxylate functionalities, can bind water in tertiary structures and display degeneration and unfolding of its secondary structure due to heat treatment or reaction with enzymes. Also glycoproteins are known to be part of the metabolism of cells regulating freezing stress tolerance (Zachariassen and Christensen, 2000). We suggest that further investigations should aim in this direction.
In this study, we develop an extraction method that gives access to the cytoplasmic material of pollen grains even after the grains have lost the ability to germinate and rupture. We emphasize that birch pollen contains soluble and insoluble cytoplasmic materials. The soluble INMs are easily extracted with water. In nature, INMs from the surface of birches are washed down by rain (Seifried et al., 2020), possibly ending up in soil and/or rivers (Borduas-Dedekind et al., 2019; Knackstedt et al., 2018). The insoluble material is mostly starch granules that we refer to as SPPs in accordance with the usage of the term SPP in the medical sciences (e.g. Bacsi et al., 2006). INMs are exclusively found within the aqueous solution, but it took several times of washing and dilution for a sample to lose the ice nucleation activity, suggesting that INMs are present in high concentrations. In contrast, we find that purified SPPs are not ice nucleation active. Fluorescence spectroscopy reveals a strong protein signal in the remaining ice active solution that is not found with the highly concentrated SPPs. Generally, we see a quantitative link between the ice nucleation activity CNC(−25 ∘C) and the protein concentration during the washing procedure. Therefore, the INMs, as well as the proteins of the suspension, must be soluble and extractable during the filtration process in the same manner. We highlight that the ice nucleation activity of Betula pendula pollen is linked not only to polysaccharides (Pummer et al., 2015) but also to proteinaceous INMs. The abundance of INMs suggests that INMs and SPPs might not naturally separate in the atmosphere. SPPs could act as carriers of INMs. Similarly, starch granules are known to act as carriers of allergens of different pollen grains (Bacsi et al., 2006; Schäppi et al., 1999; Staff et al., 1999). Pollen rupture generating airborne SPPs could therefore be a possible mechanism of how INMs from pollen grains could disperse in the atmosphere without the presence of the grains.
All data are available from the corresponding author upon request.
The video shows birch (Betula pendula) pollen grains immersed in water. It can be seen that the pollen grains rupture and expel cytoplasmic material including starch granules. These materials are also referred to as subpollen particles (https://doi.org/10.5281/zenodo.5550102, last access: 6 October 2021) (Burkart and Gratzl, 2021).
The supplement related to this article is available online at: https://doi.org/10.5194/bg-18-5751-2021-supplement.
JB wrote the manuscript with contributions from all co-authors. JB and HG developed the concept of the study. JB and HG acquired financial support for the project. JB worked out the details and guided experiments. JB conducted pollen rupture experiments, took SEM images, and produced the video material with the help of JG. JG and JB developed the extraction method. JG analysed subpollen particles and created the graphical representation. TMS and PB conducted the ice nucleation measurements and respective data analysis including graphical representation. TMS and PB performed the Bradford assay, the fluorescence spectroscopy, and the control experiment, as well as the digestion and unfolding experiments.
The authors declare that they have no conflict of interest.
Publisher’s note: Copernicus Publications remains neutral with regard to jurisdictional claims in published maps and institutional affiliations.
The authors would like to thank Robin Hoheneder at the University of Natural Resources and Life Sciences, Vienna, for his help with the Bradford assay.
The authors gratefully acknowledge the Hochschuljubiläumsstiftung der Stadt Wien (grant nos. H-318313/2018 and H-2075/2010) and the Austrian Science Fund (FWF; grant no. P26040) for financial support. Open-access funding has been provided by the University of Vienna.
This paper was edited by Dan Yakir and reviewed by two anonymous referees.
Bacsi, A., Choudhury, B. K., Dharajiya, N., Sur, S., and Boldogh, I.: Subpollen particles: Carriers of allergenic proteins and oxidases, J. Allergy Clin. Immun., 118, 844–850, https://doi.org/10.1016/j.jaci.2006.07.006, 2006.
Baker, H. G. and Baker, I.: Starch in angiosperm pollen grains and its anthecological significance, New. Zeal. J. Bot., 17, 535–535, https://doi.org/10.1080/0028825X.1979.10432569, 1979.
Bogler, S. and Borduas-Dedekind, N.: Lignin's ability to nucleate ice via immersion freezing and its stability towards physicochemical treatments and atmospheric processing, Atmos. Chem. Phys., 20, 14509–14522, https://doi.org/10.5194/acp-20-14509-2020, 2020.
Borduas-Dedekind, N., Ossola, R., David, R. O., Boynton, L. S., Weichlinger, V., Kanji, Z. A., and McNeill, K.: Photomineralization mechanism changes the ability of dissolved organic matter to activate cloud droplets and to nucleate ice crystals, Atmos. Chem. Phys., 19, 12397–12412, https://doi.org/10.5194/acp-19-12397-2019, 2019.
Boucher, O., Randall, D., Artaxo, P., Bretherton, C., Feingold, G., Forster, P., Kerminen, V.-M., Kondo, Y., Liao, H., Lohmann, U., Rasch, P., Satheesh, S. K., Sherwood, S., Stevens, B., and Zhang, X. Y.: Clouds and Aerosols, in: T. F. Intergovernmental Panel on Climate Change, (edn. Climate Change 2013: The Physical Science Basis) Contribution of Working Group I to the Fifth Assessment Report of the Intergovernmental Panel on Climate Change, edited by: Stocker, T. F., Qin, D., Plattner, G.-K., Tignor, M., Allen, S. K., Boschung, J., Nauels, A., Xia, Y., Bex, V., and Midgley, P. M., Cambridge University Press, 571–658, https://doi.org/10.1017/CBO9781107415324.016, 2013.
Bradford, M. M.: A rapid and sensitive method for the quantitation of microgram quantities of protein utilizing the principle of protein-dye binding, Anal Biochem., 72, 248–254, https://doi.org/10.1006/abio.1976.9999, 1976.
Buléon, A., Colonna, P., Planchot, V., and Ball, S.: Starch granules: structure and biosynthesis, Int. J. Biol. Macromol., 23, 85–112, https://doi.org/10.1016/S0141-8130(98)00040-3, 1998.
Burkart, J. and Gratzl, J.: Birch pollen rupture, Zenodo, https://doi.org/10.5281/zenodo.5550102, 2021.
D'Amato, G., Liccardi, G., and Frenguelli, G.: Thunderstorm-asthma and pollen allergy, Allergy, 62, 11–16, https://doi.org/10.1111/j.1398-9995.2006.01271.x, 2007.
D'Amato, G., Tedeschini, E., Frenguelli, G., and D'Amato, M.: Allergens as trigger factors for allergic respiratory diseases and severe asthma during thunderstorms in pollen season, Aerobiologia, 35, 379–382, https://doi.org/10.1007/s10453-019-09560-8, 2019.
Damialis, A., Kaimakamis, E., Konoglou, M., Akritidis, I., Traidl-Hoffmann, C., and Gioulekas, D.: Estimating the abundance of airborne pollen and fungal spores at variable elevations using an aircraft: how high can they fly?, Sci. Rep.-UK, 7, 44535, https://doi.org/10.1038/srep44535, 2017.
Dengate, H. N., Baruch, D. W., and Meredith, P.: The Density of Wheat Starch Granules: A Tracer Dilution Procedure for Determining the Density of an Immiscible Dispersed Phase, Starch – Stärke, 30, 80–84, https://doi.org/10.1002/star.19780300304, 1978.
Diehl, K., Matthias-Maser, S., Jaenicke, R., and Mitra, S. K.: The ice nucleating ability of pollen:: Part II. Laboratory studies in immersion and contact freezing modes, Atmos. Res., 61, 125–133, https://doi.org/10.1016/S0169-8095(01)00132-6, 2002.
Diehl, K., Quick, C., Matthias-Maser, S., Mitra, S. K., and Jaenicke, R.: The ice nucleating ability of pollen: Part I: Laboratory studies in deposition and condensation freezing modes, Atmos. Res., 58, 75–87, https://doi.org/10.1016/S0169-8095(01)00091-6, 2001.
Diethart, B., Sam, S., and Weber, M.: Walls of allergenic pollen: Special reference to the endexine, Grana, 46, 164–175, https://doi.org/10.1080/00173130701472181, 2007.
Dreischmeier, K., Budke, C., Wiehemeier, L., Kottke, T., and Koop, T.: Boreal pollen contain ice-nucleating as well as ice-binding “antifreeze” polysaccharides, Sci. Rep.-UK, 7, 41890, https://doi.org/10.1038/srep41890, 2017.
Fægri, K. and Iversen, J.: Textbook of pollen analysis, 4 edn., reprint, edited by: Fægri, K., Emil Kaland, P., and Krzywinski, K., Wiley, Chichester, https://ubdata.univie.ac.at/AC07661753, 328 pp., ISBN: 0471937193, 1992.
Felgitsch, L., Baloh, P., Burkart, J., Mayr, M., Momken, M. E., Seifried, T. M., Winkler, P., Schmale III, D. G., and Grothe, H.: Birch leaves and branches as a source of ice-nucleating macromolecules, Atmos. Chem. Phys., 18, 16063–16079, https://doi.org/10.5194/acp-18-16063-2018, 2018.
Felgitsch, L., Bichler, M., Burkart, J., Fiala, B., Häusler, T., Hitzenberger, R., and Grothe, H.: Heterogeneous Freezing of Liquid Suspensions Including Juices and Extracts from Berries and Leaves from Perennial Plants, Atmosphere-Basel, 10, 37, https://doi.org/10.3390/atmos10010037, 2019.
Fröhlich-Nowoisky, J., Hill, T. C. J., Pummer, B. G., Yordanova, P., Franc, G. D., and Pöschl, U.: Ice nucleation activity in the widespread soil fungus Mortierella alpina, Biogeosciences, 12, 1057–1071, https://doi.org/10.5194/bg-12-1057-2015, 2015.
Fröhlich-Nowoisky, J., Kampf, C. J., Weber, B., Huffman, J. A., Pöhlker, C., Andreae, M. O., Lang-Yona, N., Burrows, S. M., Gunthe, S. S., Elbert, W., Su, H., Hoor, P., Thines, E., Hoffmann, T., Després, V. R., and Pöschl, U.: Bioaerosols in the Earth system: Climate, health, and ecosystem interactions, Atmos. Res., 182, 346–376, https://doi.org/10.1016/j.atmosres.2016.07.018, 2016.
Grote, M., Valenta, R., and Reichelt, R.: Abortive pollen germination: A mechanism of allergen release in birch, alder, and hazel revealed by immunogold electron microscopy, J. Allergy Clin. Immun., 111, 1017–1023, https://doi.org/10.1067/mai.2003.1452, 2003.
Gute, E. and Abbatt, J. P.: Ice nucleating behavior of different tree pollen in the immersion mode, Atmos. Environ., 231, 117488, https://doi.org/10.1016/j.atmosenv.2020.117488, 2020.
Gute, E., David, R. O., Kanji, Z. A., and Abbatt, J. P.: Ice Nucleation Ability of Tree Pollen Altered by Atmospheric Processing, ACS Earth Space Chem., 4, 12, 2312–2319, 2020.
Haga, D. I., Iannone, R., Wheeler, M. J., Mason, R., Polishchuk, E. A., Fetch, T., Kamp, B. J. van der, McKendry, I. G., and Bertram, A. K.: Ice nucleation properties of rust and bunt fungal spores and their transport to high altitudes, where they can cause heterogeneous freezing, J. Geophys. Res.-Atmos., 118, 7260–7272, https://doi.org/10.1002/jgrd.50556, 2013.
Haga, D. I., Burrows, S. M., Iannone, R., Wheeler, M. J., Mason, R. H., Chen, J., Polishchuk, E. A., Pöschl, U., and Bertram, A. K.: Ice nucleation by fungal spores from the classes Agaricomycetes, Ustilaginomycetes, and Eurotiomycetes, and the effect on the atmospheric transport of these spores, Atmos. Chem. Phys., 14, 8611–8630, https://doi.org/10.5194/acp-14-8611-2014, 2014.
Hancock, R. D. and Tarbet, B. J.: The Other Double Helix – The Fascinating Chemistry of Starch, J. Chem. Educ., 77, 988, https://doi.org/10.1021/ed077p988, 2000.
Hedstrom, L.: Serine protease mechanism and specificity, Chem. Rev., 102, 4501–4524, https://doi.org/10.1021/cr000033x, 2002.
Hiranuma, N., Möhler, O., Yamashita, K., Tajiri, T., Saito, A., Kiselev, A., Hoffmann, N., Hoose, C., Jantsch, E., Koop, T., and Murakami, M.: Ice nucleation by cellulose and its potential contribution to ice formation in clouds, Nat. Geosci., 8, 273–277, https://doi.org/10.1038/ngeo2374, 2015.
Hiranuma, N., Adachi, K., Bell, D. M., Belosi, F., Beydoun, H., Bhaduri, B., Bingemer, H., Budke, C., Clemen, H.-C., Conen, F., Cory, K. M., Curtius, J., DeMott, P. J., Eppers, O., Grawe, S., Hartmann, S., Hoffmann, N., Höhler, K., Jantsch, E., Kiselev, A., Koop, T., Kulkarni, G., Mayer, A., Murakami, M., Murray, B. J., Nicosia, A., Petters, M. D., Piazza, M., Polen, M., Reicher, N., Rudich, Y., Saito, A., Santachiara, G., Schiebel, T., Schill, G. P., Schneider, J., Segev, L., Stopelli, E., Sullivan, R. C., Suski, K., Szakáll, M., Tajiri, T., Taylor, H., Tobo, Y., Ullrich, R., Weber, D., Wex, H., Whale, T. F., Whiteside, C. L., Yamashita, K., Zelenyuk, A., and Möhler, O.: A comprehensive characterization of ice nucleation by three different types of cellulose particles immersed in water, Atmos. Chem. Phys., 19, 4823–4849, https://doi.org/10.5194/acp-19-4823-2019, 2019.
Hoidn, C., Puchner, E., Pertl, H., Holztrattner, E., and Obermeyer, G.: Non diffusional release of allergens from pollen grains of Artemisia vulgaris and Lilium longiflorum depends mainly on the type of the allergen, Int. Arch. Allergy. Imm., 137, 27–36, https://doi.org/10.1159/000084610, 2005.
Hoose, C., Kristjánsson, J. E., and Burrows, S. M.: How important is biological ice nucleation in clouds on a global scale?, Environ. Res. Lett., 5, 024009, https://doi.org/10.1088/1748-9326/5/2/024009, 2010a.
Hoose, C., Kristjánsson, J. n. E., Chen, J.-P., and Hazra, A.: A Classical-Theory-Based Parameterization of Heterogeneous Ice Nucleation by Mineral Dust, Soot, and Biological Particles in a Global Climate Model, J. Atmos. Sci., 67, 2483–2503, https://doi.org/10.1175/2010jas3425.1, 2010b.
Huffman, J. A., Prenni, A. J., DeMott, P. J., Pöhlker, C., Mason, R. H., Robinson, N. H., Fröhlich-Nowoisky, J., Tobo, Y., Després, V. R., Garcia, E., Gochis, D. J., Harris, E., Müller-Germann, I., Ruzene, C., Schmer, B., Sinha, B., Day, D. A., Andreae, M. O., Jimenez, J. L., Gallagher, M., Kreidenweis, S. M., Bertram, A. K., and Pöschl, U.: High concentrations of biological aerosol particles and ice nuclei during and after rain, Atmos. Chem. Phys., 13, 6151–6164, https://doi.org/10.5194/acp-13-6151-2013, 2013.
Hughes, D. D., Mampage, C. B. A., Jones, L. M., Liu, Z., and Stone, E. A.: Characterization of Atmospheric Pollen Fragments during Springtime Thunderstorms, Environ. Sci. Tech. Lett., 7, 409–414, https://doi.org/10.1021/acs.estlett.0c00213, 2020.
Jochner, S., Lüpke, M., Laube, J., Weichenmeier, I., Pusch, G., Traidl-Hoffmann, C., Schmidt-Weber, C., Buters, J. T. M., and Menzel, A.: Seasonal variation of birch and grass pollen loads and allergen release at two sites in the German Alps, Atmos. Environ., 122, 83–93, https://doi.org/10.1016/j.atmosenv.2015.08.031, 2015.
Kanji, Z. A., Ladino, L. A., Wex, H., Boose, Y., Burkert-Kohn, M., Cziczo, D. J., and Krämer, M.: Overview of Ice Nucleating Particles, Meteor. Mon., 58, 1.1–1.33. https://doi.org/10.1175/amsmonographs-d-16-0006.1, 2017.
Knackstedt, K., Moffett, B. F., Hartmann, S., Wex, H., Hill, T. C. J., Glasgo, E., Reitz, L., Augustin-Bauditz, S., Beall, B., Bullerjahn, G. S., Fröhlich-Nowoisky, J., Grawe, S., Lubitz, J., Stratmann, F., and McKay, R. M.: A terrestrial origin for abundant riverine nanoscale ice-nucleating particles, Environ. Sci. Technol., 52, 12358–12367, https://doi.org/10.1021/acs.est.8b03881, 2018.
Kozloff, L. M., Turner, M. A., and Arellano, F.: Formation of bacterial membrane ice-nucleating lipoglycoprotein complexes, J. Bacteriol., 173, 6528–6536, https://doi.org/10.1128/jb.173.20.6528-6536.1991, 1991.
Kunert, A. T., Lamneck, M., Helleis, F., Pöschl, U., Pöhlker, M. L., and Fröhlich-Nowoisky, J.: Twin-plate Ice Nucleation Assay (TINA) with infrared detection for high-throughput droplet freezing experiments with biological ice nuclei in laboratory and field samples, Atmos. Meas. Tech., 11, 6327–6337, https://doi.org/10.5194/amt-11-6327-2018, 2018.
Kunert, A. T., Pöhlker, M. L., Tang, K., Krevert, C. S., Wieder, C., Speth, K. R., Hanson, L. E., Morris, C. E., Schmale III, D. G., Pöschl, U., and Fröhlich-Nowoisky, J.: Macromolecular fungal ice nuclei in Fusarium: effects of physical and chemical processing, Biogeosciences, 16, 4647–4659, https://doi.org/10.5194/bg-16-4647-2019, 2019.
Ling, M. L., Wex, H., Grawe, S., Jakobsson, J., Löndahl, J., Hartmann, S., Finster, K., Boesen, T., and Šantlâ Temkiv, T.: Effects of Ice Nucleation Protein Repeat Number and Oligomerization Level on Ice Nucleation Activity, J. Geophys. Res.-Atmos., 123, 1802–1810, https://doi.org/10.1002/2017JD027307, 2018.
Lloyd, G., Choularton, T., Bower, K., Crosier, J., Gallagher, M., Flynn, M., Dorsey, J., Liu, D., Taylor, J. W., Schlenczek, O., Fugal, J., Borrmann, S., Cotton, R., Field, P., and Blyth, A.: Small ice particles at slightly supercooled temperatures in tropical maritime convection, Atmos. Chem. Phys., 20, 3895–3904, https://doi.org/10.5194/acp-20-3895-2020, 2020.
Maki, L. R., Galyan, E. L., Chang-Chien, M.-M., and Caldwell, D. R.: Ice nucleation induced by Pseudomonas syringae, Appl. Microbiol., 28, 456–459, https://doi.org/10.1128/am.28.3.456-459.1974, 1974.
Matsushima, R., Maekawa, M., Kusano, M., Tomita, K., Kondo, H., Nishimura, H., Crofts, N., Fujita, N., and Sakamoto, W.: Amyloplast Membrane Protein SUBSTANDARD STARCH GRAIN Controls Starch Grain Size in Rice Endosperm, Plant. Physiol., 170, 1445–1459, https://doi.org/10.1104/pp.15.01811, 2016.
Mikhailov, E., Ivanova, O., Nebosko, E. Y., Vlasenko, S., and Ryshkevich, T.: Subpollen particles as atmospheric cloud condensation nuclei. Izvestiya, Atmos. Ocean. Phys., 55, 357–364, https://doi.org/10.1134/S000143381904008X, 2019.
Möhler, O., DeMott, P. J., Vali, G., and Levin, Z.: Microbiology and atmospheric processes: the role of biological particles in cloud physics, Biogeosciences, 4, 1059–1071, https://doi.org/10.5194/bg-4-1059-2007, 2007.
Murray, B. J., O'Sullivan, D., Atkinson, J. D., and Webb, M. E.: Ice nucleation by particles immersed in supercooled cloud droplets, Chem. Soc. Rev., 41, 6519–6554, https://doi.org/10.1039/C2CS35200A, 2012.
O'Sullivan, D., Murray, B. J., Ross, J. F., Whale, T. F., Price, H. C., Atkinson, J. D., Umo, N. S., and Webb, M. E.: The relevance of nanoscale biological fragments for ice nucleation in clouds, Sci. Rep.-UK., 5, 8082, https://doi.org/10.1038/srep08082, 2015.
Papadopoulou, A., Green, R. J., and Frazier, R. A.: Interaction of flavonoids with bovine serum albumin: a fluorescence quenching study, J. Agr. Food. Chem., 53, 158–163, https://doi.org/10.1021/jf048693g, 2005.
Pöhlker, C., Huffman, J. A., and Pöschl, U.: Autofluorescence of atmospheric bioaerosols – fluorescent biomolecules and potential interferences, Atmos. Meas. Tech., 5, 37–71, https://doi.org/10.5194/amt-5-37-2012, 2012.
Pope, F. D.: Pollen grains are efficient cloud condensation nuclei, Environ. Res. Lett., 5, 044015, https://doi.org/10.1088/1748-9326/5/4/044015, 2010.
Pummer, B. G., Bauer, H., Bernardi, J., Bleicher, S., and Grothe, H.: Suspendable macromolecules are responsible for ice nucleation activity of birch and conifer pollen, Atmos. Chem. Phys., 12, 2541–2550, https://doi.org/10.5194/acp-12-2541-2012, 2012.
Pummer, B. G., Bauer, H., Bernardi, J., Chazallon, B., Facq, S., Lendl, B., Whitmore, K., and Grothe, H.: Chemistry and morphology of dried-up pollen suspension residues, J. Raman. Spectrosc., 44, 1654–1658, https://doi.org/10.1002/jrs.4395, 2013.
Pummer, B. G., Budke, C., Augustin-Bauditz, S., Niedermeier, D., Felgitsch, L., Kampf, C. J., Huber, R. G., Liedl, K. R., Loerting, T., Moschen, T., Schauperl, M., Tollinger, M., Morris, C. E., Wex, H., Grothe, H., Pöschl, U., Koop, T., and Fröhlich-Nowoisky, J.: Ice nucleation by water-soluble macromolecules, Atmos. Chem. Phys., 15, 4077–4091, https://doi.org/10.5194/acp-15-4077-2015, 2015.
Qiu, Y., Hudait, A., and Molinero, V.: How size and aggregation of ice-binding proteins control their ice nucleation efficiency, J. Am. Chem. Soc., 141, 7439–7452, https://doi.org/10.1021/jacs.9b01854, 2019.
Rawlings, N. D., Barrett, A. J., and Bateman, A.: MEROPS: the peptidase database, Nucleic Acids Res., 38(suppl_1), D227–D233, https://doi.org/10.1093/nar/gkp971, 2010.
Rathnayake, C. M., Metwali, N., Jayarathne, T., Kettler, J., Huang, Y., Thorne, P. S., O'Shaughnessy, P. T., and Stone, E. A.: Influence of rain on the abundance of bioaerosols in fine and coarse particles, Atmos. Chem. Phys., 17, 2459–2475, https://doi.org/10.5194/acp-17-2459-2017, 2017.
Šantl-Temkiv, T., Sahyoun, M., Finster, K., Hartmann, S., Augustin-Bauditz, S., Stratmann, F., Wex, H., Clauss, T., Nielsen, N. W., Sørensen, J. H., Korsholm, U. S., Wick, L. Y., and Karlson, U. G.: Characterization of airborne ice-nucleation-active bacteria and bacterial fragments, Atmo. Environ., 109, 105–117, https:/doi.org/10.1016/j.atmosenv.2015.02.060, 2015.
Schäppi, G. F., Taylor, P. E., Staff, I. A., Suphioglu, C., and Knox, R. B.: Source of Bet v 1 loaded inhalable particles from birch revealed, Sex. Plant. Reprod., 10, 315–323, https://doi.org/10.1007/s004970050105, 1997.
Schäppi, G., Taylor, P., Staff, I., Rolland, J., and Suphioglu, C.: Immunologic significance of respirable atmospheric starch granules containing major birch allergen Bet v 1, Allergy, 54, 478–483, https://doi.org/10.1034/j.1398-9995.1999.00838.x, 1999.
Seifried, T. M., Bieber, P., Felgitsch, L., Vlasich, J., Reyzek, F., Schmale III, D. G., and Grothe, H.: Surfaces of silver birch (Betula pendula) are sources of biological ice nuclei: in vivo and in situ investigations, Biogeosciences, 17, 5655–5667, https://doi.org/10.5194/bg-17-5655-2020, 2020.
Sénéchal, H., Visez, N., Charpin, D., Shahali, Y., Peltre, G., Biolley, J.-P., Lhuissier, F., Couderc, R., Yamada, O., Malrat-Domenge, A., Pham-Thi, N., Poncet, P., and Sutra, J.-P.: A Review of the Effects of Major Atmospheric Pollutants on Pollen Grains, Pollen Content, and Allergenicity, The Scientific World Journal, 2015, 940243, https://doi.org/10.1155/2015/940243, 2015.
Šikoparija, B., Mimić, G., Panić, M., Marko, O., Radišić, P., Pejak-Šikoparija, T., and Pauling, A.: High temporal resolution of airborne Ambrosia pollen measurements above the source reveals emission characteristics, Atmos. Environ., 192, 13–23, https://doi.org/10.1016/j.atmosenv.2018.08.040, 2018.
Skjøth, C. A., Sommer, J., Stach, A., Smith, M., and Brandt, J.: The long-range transport of birch (Betula) pollen from Poland and Germany causes significant pre-season concentrations in Denmark, Clin. Exp. Allergy., 37, 1204–1212. https://doi.org/10.1111/j.1365-2222.2007.02771.x, 2007.
Sofiev, M., Belmonte, J., Gehrig, R., Izquierdo, R., Smith, M., Dahl, Å., and Siljamo, P.: Airborne pollen transport, in: Allergenic Pollen, edited by: Sofiev, M. and Bergmann, K. C., Springer, Dordrecht, 127–159, https://doi.org/10.1007/978-94-007-4881-1_5, 2012.
Staff, I., Schäppi, G., and Taylor, P.: Localisation of allergens in ryegrass pollen and in airborne micronic particles, Protoplasma, 208, 47–57, https://doi.org/10.1007/BF01279074, 1999.
Steiner, A. L., Brooks, S. D., Deng, C., Thornton, D. C. O., Pendleton, M. W., and Bryant, V.: Pollen as atmospheric cloud condensation nuclei, Geophys. Res. Lett., 42, 3596–3602. https://doi.org/10.1002/2015GL064060, 2015.
Suphioglu, C., Singh, M. B., Taylor, P., Knox, R., Bellomo, R., Holmes, P., and Puy, R.: Mechanism of grass-pollen-induced asthma, Lancet, 339, 569–572, https://doi.org/10.1016/0140-6736(92)90864-Y, 1992.
Taylor, P. E. and Jonsson, H.: Thunderstorm asthma, Curr. Allergy Asthm. Reports, 4, 409–413, https://doi.org/10.1007/s11882-004-0092-3, 2004.
Taylor, P. E., Flagan, R., Miguel, A. G., Valenta, R., and Glovsky, M.: Birch pollen rupture and the release of aerosols of respirable allergens, Clin. Exp. Allergy, 34, 1591–1596, https://doi.org/10.1111/j.1365-2222.2004.02078.x, 2004.
Taylor, P. E., Jacobson, K. W., House, J. M., and Glovsky, M. M.: Links between pollen, atopy and the asthma epidemic, Int. Arch. Allergy. Imm., 144, 162–170, https://doi.org/10.1159/000103230, 2007.
Thien, F.: Melbourne epidemic thunderstorm asthma event 2016: Lessons learnt from the perfect storm, Respirology, 23, 976–977, https://doi.org/10.1111/resp.13410, 2018.
Tong, H.-J., Ouyang, B., Nikolovski, N., Lienhard, D. M., Pope, F. D., and Kalberer, M.: A new electrodynamic balance (EDB) design for low-temperature studies: application to immersion freezing of pollen extract bioaerosols, Atmos. Meas. Tech., 8, 1183–1195, https://doi.org/10.5194/amt-8-1183-2015, 2015.
Vali, G.: Quantitative Evaluation of Experimental Results and the Heterogeneous Freezing Nucleation of Supercooled Liquids, J. Atmos. Sci., 28, 402–409, https://doi.org/10.1175/1520-0469(1971)028<0402:QEOERA>2.0.CO;2, 1971.
Visez, N., Chassard, G., Azarkan, N., Naas, O., Sénéchal, H., Sutra, J.-P., Poncet, P., and Choël, M.: Wind-induced mechanical rupture of birch pollen: Potential implications for allergen dispersal, J. Aerosol. Sci., 89, 77–84, https://doi.org/10.1016/j.jaerosci.2015.07.005, 2015.
von Blohn, N., Mitra, S. K., Diehl, K., and Borrmann, S.: The ice nucleating ability of pollen: Part III: New laboratory studies in immersion and contact freezing modes including more pollen types, Atmos. Res., 78, 182–189, https://doi.org/10.1016/j.atmosres.2005.03.008, 2005.
Vrtala, S., Grote, M., Duchêne, M., Kraft, D., Scheiner, O., and Valenta, R.: Properties of tree and grass pollen allergens: reinvestigation of the linkage between solubility and allergenicity, Int. Arch. Allergy Imm., 102, 160–169, https://doi.org/10.1159/000236567, 1993.
Williams, C. G. and Després, V.: Northern Hemisphere forests at temperate and boreal latitudes are substantial pollen contributors to atmospheric bioaerosols, Forest. Ecol. Manag., 401, 187–191, https://doi.org/10.1016/j.foreco.2017.06.040, 2017.
Wilson, S. L., Kelley, D. L., and Walker, V. K.: Ice-active characteristics of soil bacteria selected by ice-affinity, Environ. Microbiol., 8, 1816–1824, https://doi.org/10.1111/j.1462-2920.2006.01066.x, 2006.
Wozniak, M. C., Solmon, F., and Steiner, A. L.: Pollen Rupture and Its Impact on Precipitation in Clean Continental Conditions, Geophys. Res. Lett., 45, 7156–7164, https://doi.org/10.1029/2018GL077692, 2018.
Xi, Y., Mercier, A., Kuang, C., Yun, J., Christy, A., Melo, L., Maldonado, M. T., Raymond, J. A., and Bertram, A. K.: Concentrations and properties of ice nucleating substances in exudates from Antarctic sea-ice diatoms, Environ. Sci. Process. Impacts, 23, 323–334, https://doi.org/10.1039/D0EM00398K, 2021.
Zachariassen, K. E. and Kristiansen, E.: Ice nucleation and antinucleation in nature, Cryobiology, 41, 257–279, https://doi.org/10.1006/cryo.2000.2289, 2000.
Zolles, T., Burkart, J., Häusler, T., Pummer, B., Hitzenberger, R., and Grothe, H.: Identification of ice nucleation active sites on feldspar dust particles, The Journal of Physical Chemistry A, 119, 2692-2700, https://doi.org/10.1021/jp509839x, 2015.