the Creative Commons Attribution 4.0 License.
the Creative Commons Attribution 4.0 License.
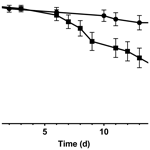
Anthropogenic CO2-mediated freshwater acidification limits survival, calcification, metabolism, and behaviour in stress-tolerant freshwater crustaceans
Alex R. Quijada-Rodriguez
Pou-Long Kuan
Po-Hsuan Sung
Mao-Ting Hsu
Garett J. P. Allen
Pung Pung Hwang
Yung-Che Tseng
Dirk Weihrauch
Dissolution of anthropogenic CO2 is chronically acidifying aquatic ecosystems. Studies indicate that ocean acidification will cause marine life, especially calcifying species, to suffer at the organism and ecosystem levels. In comparison, freshwater acidification has received less attention, rendering its consequences unclear. Here, juvenile Chinese mitten crabs, Eriocheir sinensis, were used as a crustacean model to investigate the impact of CO2-mediated freshwater acidification. Our integrative approach, investigating changes in the animal's acid–base homeostasis, metabolism, calcification, locomotory behaviour, and survival rate, indicates that this economically relevant crustacean will face energetic consequences from future freshwater acidification. These energetic trade-offs allow the animal to maintain its acid–base homeostasis at the cost of reduced metabolic activity, exoskeletal calcification, and locomotion, reducing the animal's overall fitness and increasing its mortality. Results indicate that present-day Chinese mitten crab could be heavily affected by freshwater acidification like their marine counterparts and emphasize the importance of understanding the long-term implications of freshwater acidification on species' fitness.
- Article
(595 KB) - Full-text XML
- BibTeX
- EndNote
Rising levels of atmospheric CO2 partially dissolve into marine systems, causing a decrease in oceanic pH referred to as ocean acidification. In marine species, ocean acidification has been demonstrated to impact development, metabolism, behaviour, and biomineralization, potentially leading to major ecosystem-level changes (Kroeker et al., 2013; Melzner et al., 2009; Tresguerres and Hamilton, 2017). It is generally believed that freshwater systems will also experience acidification (Hasler et al., 2016; Phillips et al., 2015; Weiss et al., 2018). However, the high variability in biogeochemistry between freshwater systems has been a limiting factor in modelling future freshwater scenarios (Hasler et al., 2016). Two recent case studies on different freshwater systems have suggested that the magnitude of CO2-mediated acidification could be similar to or even exceed predicted levels of ocean acidification (Phillips et al., 2015; Weiss et al., 2018). The potential that freshwater acidification may be of equal or greater severity than ocean acidification emphasizes the need to understand the biological responses and consequences to freshwater species.
Calcifying species are sensitive to acidification as dissolution of CO2 reduces carbonate availability in parallel to pH, potentially increasing dissolution of their calcified exoskeleton (Feely et al., 2004; Roleda et al., 2012). To date, no comprehensive studies have investigated the various physiological and behavioural effects of realistic future levels of CO2-mediated acidification on calcifying freshwater invertebrates. However, several studies have used high CO2 levels beyond those relevant for potential future freshwater acidification to investigate acid–base regulation and calcification in freshwater calcifying invertebrates (Cameron, 1978, 1985; David et al., 2020; Jeffrey et al., 2018a, b). Freshwater calcifying macro-organisms are largely limited to crustaceans and molluscs that comprise roughly 10 % and 4 % of freshwater species diversity, respectively (Balian et al., 2008). Crustaceans are arguably one of the most successful animal groups, occupying almost all ecological niches across the globe, including freshwater, marine, and terrestrial habitats, making them a suitable model to study global change consequences in a physiologically and ecologically robust group of species. Freshwater crustaceans occupy a key position in food webs where all crustacean life stages provide a vital food source for a wide range of juvenile and adult predators (Cumberlidge et al., 2009). Additionally, freshwater crustaceans provide vital ecological services as indicators of water quality, nutrient cycling of detritus, and bioturbation of sediment (Cumberlidge et al., 2009). From an economic standpoint, freshwater crustaceans account for ∼ 30 % (2.5×106 t) of aqua-cultured crustaceans worldwide, demonstrating that this group is an important human food source (Tacon, 2020). The ecological and economic importance of freshwater crustaceans, together with the apparent sensitivity of calcifying species to acidification based on marine studies, makes it imperative to determine whether freshwater crustaceans are sensitive to anthropogenic CO2-mediated freshwater acidification.
The Chinese mitten crab (Eriocheir sinensis) is one of the most important freshwater crustaceans, accounting for the third largest crustacean aquaculture globally (FAO, 2018). This highly invasive catadromous species spends most of its life cycle in freshwater systems but has the physiological plasticity to migrate into marine environments where it reproduces (Veilleux and de Lafontaine, 2007). The invasive status and aquacultural importance of E. sinensis have made it a well-studied freshwater crustacean model in biological research. Here, we used the juvenile life stage of E. sinensis as a freshwater crustacean model to investigate the effects of a potential future CO2-mediated freshwater acidification scenario on acid–base regulation, metabolism, calcification, behaviour, and survival rate. Native to China's Yangtze River system, the third largest river system in the world, juvenile E. sinensis in this habitat already experiences regular fluctuations in freshwater pCO2 from 681 to 3796 µatm (Ran et al., 2017), which may confer some pre-adaptation to elevated CO2 because of life history. Crustaceans are believed to be more CO2 tolerant than other calcifying organisms such as bivalves and coral because of their high metabolic activity and robust acid–base machinery, allowing for more efficient compensation for acid–base disturbances (Melzner et al., 2009). These combined predictors of CO2 tolerance make E. sinensis an interesting model to study the effects of future CO2-mediated freshwater acidification, as they may already possess the adaptations necessary to deal with future freshwater acidification conditions. Therefore, we hypothesized that E. sinensis would be well-adapted to counteract challenges associated with fluctuating pCO2 resulting from anthropogenic activity and not experience detrimental physiological or behavioural impairment.
2.1 Animal maintenance
Wild-caught male and female juvenile Chinese mitten crab (Eriocheir sinensis 10–20 g) were purchased from the Chinese mitten crab breeding association of Taiwan. Crabs were maintained at the Academia Sinica Institute of Cellular and Organismal Biology aquatics facility (Taipei, Taiwan) in three 120 L aquariums with flow through dechlorinated Taipei tap water (in µmol L−1, Na+ 237, K+ 16, Ca2+ 216, Mg2+ 213, Cl− 201; Yung-Che Tseng, personal communication, 2021; see ringer measurement methods below) on a 14 : 10 h light–dark cycle with temperature ranging from 23 to 25 ∘C. Water parameters for these holding tanks were the same as that of the control water used in the experimental acclimation. Juvenile crabs in non-experimental holding tanks were maintained at a density of roughly 100 individuals per tank with PVC pipes for shelter and a constant flow of freshwater to prevent the build-up of metabolic wastes. Crabs were fed ad libitum with oatmeal and mollusc meat three times per week and monitored for activity level and the presence of disease as general health indicators. Diet was selected to maintain an omnivorous diet as seen in the wild (Czerniejewski et al., 2010) and based on what is fed by our crab supplier (Yung-Che Tseng, personal communication, 2016). Crabs were fasted for a minimum of 48 h before sampling to minimize the effects of dietary intake on measured parameters.
2.2 Freshwater acidification
For experimental acclimation, crabs were sampled upon removal from the holding tanks (0 d time point) and transferred to flow through 10 L experimental tanks (six to seven crabs per tank, four tanks per treatment) containing either control or acidified freshwater (Table 1) with PVC pipes added for shelter. Acidified freshwater was achieved by injection of CO2 directly into the experimental tanks by air-stone to maintain environmental pH (pH controller, Aqua-MACRO). The pH controller system used in this study required that each tank had a pH probe, pH controller, CO2 tank, gas regulating solenoid, and air-stone, thus meaning each tank in this study was independently pH and CO2 regulated. CO2 bubbling rate and freshwater flow rate were adjusted to minimize overshooting the target pCO2 level. Following injection of CO2 to regulate water pCO2, we recorded a brief pCO2 overshoot to a maximum level of 5625 µatm resulting from direct CO2 injection into the experimental tanks by the pH controller. Water pH, total alkalinity, and temperature were regularly measured in the experimental tanks throughout the study. Water pH (NBS scale) and temperature were measured with a pH electrode (Accumet AP55 pH/ATC electrode, Ohio, USA) connected to a portable pH meter (Accumet AP71, Ohio, USA) calibrated with pH buffers (pH 4.00, 7.00, and 10.01) traceable to NIST standard reference material (Thermo Fisher Orion). Water alkalinity was measured by spectrophotometric assay on a Nanodrop 2000c (Thermo Scientific, Wilminton, DE, USA) according to previously established protocols (Sarazin et al., 1999). Water pCO2 was calculated with the CO2SYS Excel add-in (Lewis and Wallace, 1998) using measured water temperature, pH, and total alkalinity. Constants used for pCO2 calculations include freshwater carbonate dissociation constants (K1 and K2) from Millero (1979) and KHSO4 constants from Dickson (1990).
2.3 Hemolymph acid–base status
Hemolymph acid–base experiments were conducted over 7 d to determine if crabs could actively regulate acid–base status in the presence of future freshwater acidification conditions. Hemolymph samples (100 µL per crab) were taken at the base of a walking leg with a sterile syringe according to previous protocols for E. sinensis (Truchot, 1992). Samples from two to three crabs were pooled together (200–300 µL pooled hemolymph per n value) to get a sufficient volume for downstream analyses of ammonia, pH, and total carbon. Pooled hemolymph samples were gently mixed by slowly pipetting to avoid off-gassing of CO2 and disrupting hemolymph acid–base parameters. Measurements of pH and total carbon were performed immediately after hemolymph collection, and the remaining hemolymph was frozen at −20 ∘C for later analysis of ammonia. Hemolymph pH (200–300 µL samples) was measured on the NBS scale using an InLab micro pH electrode calibrated with pH buffers traceable to NIST standard reference material (Thermo Fisher Orion). Hemolymph total carbon was measured in duplicate (50 µL per measurement) using the Corning 965 carbon dioxide analyzer (±0.2 mmol L−1 precision) calibrated with NaHCO3 standards ranging from 0 to 20 mmol L−1 to produce a standard curve with a minimum R2 of 0.99. Hemolymph pCO2 and HCO were calculated using a rearrangement of the Henderson–Hasselbalch equation with pK1 and αCO2 values derived for E. sinensis hemolymph at 23 ∘C (pK1 = 6.079773, αCO2 = 0.00031263 mmol L−1 Pa−1 (Truchot, 1976, 1992). Hemolymph ammonium was measured in triplicate (25 µL hemolymph per measurement) with a microplate reader (Molecular Devices, SpectraMax, M5) using an orthophthaldialdehyde fluorometric assay which is insensitive to amino acids and proteins (Holmes et al., 1999). Ammonia standards were made from NH4Cl in E. sinensis ringer (pH 8.1) containing (in mmol L−1) 185 NaCl, 16 CaCl2, 6 MgCl2, 7 KCl, and 13 NaHCO3. The ion concentrations for the ringer were based on ion composition measurements done on four juvenile Chinese mitten crabs in this study (in mmol L−1, Na+ 191, K+ 7.2, Ca2+ 16.3, Mg2+ 5.9, Cl− 252). Concentrations of Na+, K+, Ca2+, and Mg2+ were measured by flame absorption spectrophotometry (polarized Zeeman atomic absorption spectrophotometer Z-5000, Hitachi High-Technologies, Tokyo, Japan), and Cl− was measured spectrophotometrically using the mercury (II) thiocyanate method (Florence and Farrar, 1971). HCO and pH values for the ringer were based on measurements taken from control crabs in this study and measured as described above.
2.4 Ammonia excretion and oxygen consumption
Ammonia excretion and oxygen consumption were measured over a 7 d acclimation to control and acidified freshwater. These two parameters were measured on individual crabs haphazardly selected from the four control and four acidified freshwater aquaria. Ammonia excretion and oxygen consumption measurements were performed in parallel to hemolymph sampling; however, crabs were first randomly selected and placed into respirometry chambers before selecting crabs for hemolymph sampling to avoid using crabs recently sampled for hemolymph. Ammonia excretion experiments were performed in plastic Tupperware filled with 200 mL of filtered control or acidified freshwater. Crabs were given 30 min to acclimate to the experimental chambers before initiation of water sampling, as ammonia excretion is elevated for a short time directly after handling (Hans et al., 2014). Water samples (1 mL) for ammonia analysis were collected directly after 30 and 90 min of being placed in the experimental chambers. Ammonia concentrations of the water at the 30 and 90 min time points were determined using the aforementioned orthophthaldialdehyde fluorometric assay (Holmes et al., 1999). Ammonia excretion rates were calculated according to the Eq. (1):
where Amm90 is the water ammonia concentration at 90 min, Amm30 is the water ammonia concentration at 30 min, V is the chamber volume during the flux period in litres, t is the flux time in hours, and m is the fresh weight of the crab in grams.
The oxygen consumption rate was measured by closed-system respirometry in custom-made 3 L glass respiration chambers containing filtered (0.2 µm) freshwater. To achieve the correct experimental CO2 tension, respirometry chambers were submerged in large 18 L buckets of filtered freshwater, and a pH controller (Aqua-MACRO) was used to regulate the injection of CO2 as described above for experimental tanks. Crabs were transferred to the submerged respirometry chambers and given 15 min to adjust to fully oxygenated respiration chambers before being sealed. Chambers were placed horizontally, allowing for lateral crab movement in the chamber, and oxygen saturation was measured continuously every 15 s for 30 min at 23 ∘C. The oxygen sensor (PreSens oxygen micro optode, type PSt1, PreSens Precision Sensing GmbH, Regensburg, Germany) was attached to the top of the chamber and connected to an OXY-4 mini multichannel fibre optic oxygen transmitter (PreSens Precision Sensing GmbH, Regensburg, Germany). Oxygen saturation was always maintained above 80 %. Respiration chambers without a crab were used to determine any potential background bacterial respiration for each trial. Preliminary trials demonstrated that crab movement and ventilation rate in the chamber were sufficient to mix the water within the chamber and prevent oxygen stratification, as indicated by a linear decline in oxygen availability. While this approach allows for the measurement of oxygen consumption, some limitations must be considered. Logistical constraints prevented the use of an intermittent flow respirometry approach where the animal could have been given a long amount of time to acclimate to the respirometry chamber. This technical limitation means that the reported measurements cannot be considered a resting metabolic rate as the handling stress, brief air exposure, and transfer to a novel environment may have influenced the animal's metabolic rate. However, we would like to point out that in previous trials from our lab using an intermittent flow respirometry setup on green crabs Carcinus maenas, crayfish Procambarus clarkii, and lobsters Homarus americanus crustaceans placed in respirometry chambers will stabilize oxygen consumption to a resting rate in under 30 min (Gwangseok R. Yoon, personal communication, 2021).
2.5 Carapace calcification
To assess carapace calcification, changes in the calcium content relative to carapace mass was measured at 1, 2, 3, and 6 weeks of high CO2 exposure according to previously established protocols (Spicer and Eriksson, 2003). In brief, a piece of carapace (ca. 2.5 cm2, 15.2±0.4 mg) was removed from the dorsal carapace. The weighed piece of carapace was digested in HNO3 (13.1 N) at 60 ∘C for 16 h. Digested samples were then diluted to a final HNO3 concentration of 2 % . The carapace Ca2+ content was measured by atomic absorption spectrophotometer (Z-8000; Hitachi). Standard solutions from Merck (Darmstadt, Germany) were used to make the Ca2+ standard curve.
2.6 Locomotory behaviour assay
A 24 cm × 24 cm, novel, opaque tank was used in the open field test to assess changes in movement of juvenile crabs after a 7 d exposure to control and freshwater acidified conditions. Acclimated crabs were transferred to the novel tank containing control or acidified freshwater and given 5 min to acclimate, as done in previous crustacean behavioural studies (Robertson et al., 2018). After acclimation, crab activity was recorded with a digital camera (UI-3240CP Rev.2, Ids, Germany) for 5 min (300 s), and videos of the movement were processed with the image analysis Ethovision XT motion tracking software (v. 7.0, Noldus, the Netherlands). In this study, four factors were measured: distance covered (cm), velocity (cm s−1), movement (time in movement, seconds), and mobility (time in mobile state, seconds). We defined movement as the duration for which the central body point (whole body) was changing location. Mobile state was defined as the duration in which crabs exhibited any movement, even if the center point of the animals remained in the same location, for example, appendage movement.
2.7 Statistical analysis
Statistical analyses were conducted using JMP Pro 16 (Cary, NC, USA) and GraphPad Prism 8.4.2 (San Diego, CA, USA). Data were analyzed for outliers by the ROUT test with a Q value of 1 %. For all data, heterogeneity of variance was tested by Levene's test and normal distribution of residuals by the Shapiro–Wilk test. Two transformations were done so that data could meet the assumptions of normal distribution and homogeneity of variance. A Johnson SB transformation was applied to hemolymph pCO2 data, and a square root transformation was applied to ammonia excretion rate data. In this study, hemolymph parameters, ammonia excretion, and oxygen consumption data were analyzed by a two-way ANOVA post hoc Dunnett test. For Dunnett's test, comparisons were made to the 0 d control with time and pCO2 values as fixed factors. Carapace calcification data were analyzed by two-way ANOVA post hoc Tukey HSD with time and pCO2 values as the fixed factors. Behavioural data displayed a high degree of co-linearity between dependent variables, violating the assumptions of the MANOVA test. Therefore, we analyzed behavioural data using a Student t test except for appendage movement time. Appendage movement time data violated assumptions of Student's t test, so they were analyzed by the Wilcoxon test. Survival curves were analyzed for significant differences by the Mantel–Cox test. The survival curve hazard ratio was determined by the Mantel–Haenszel test. For all data sets, p values ≤ 0.05 were considered significant. Data are presented as mean ± standard error (SEM). Statistical output results are written in text or summarized in Table 2.
Table 2Statistical results of two-way ANOVAs from hemolymph acid–base parameters, oxygen consumption, ammonia excretion, and carapace calcification experiments. For hemolymph acid–base measurements response variables were hemolymph pH, HCO, pCO2, or ammonia with time and CO2 as a fixed independent variable. For whole-animal experiments, response variables were oxygen consumption rate and ammonia excretion rate with time and CO2 as a fixed independent variable. For carapace calcification experiments, the response variable was carapace calcium content with time and CO2 as a fixed independent variable. p values below 0.05 are considered statistically significant and are bolded. df denotes degrees of freedom.
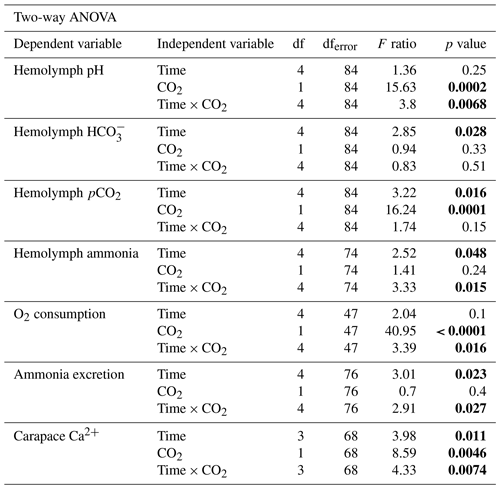
3.1 Probability of survival
The effect of freshwater acidification on survival was determined by generating survival curves for crabs in control and acidified freshwater (Fig. 1). There was a significant difference in the probability of survival between the control and acidified freshwater environments (Mantel–Cox log rank test, , p=0.0022, Fig. 1). Crabs in the acidified freshwater tanks had a 50 % mortality compared to 15 % mortality in control freshwater tanks. Calculation of the Mantel–Haenszel hazard ratio indicates that crabs in acidified freshwater have a 3.68 times greater probability of mortality than the crabs held under control conditions.
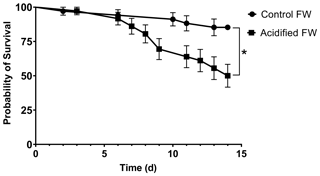
Figure 1Survivorship curves of juvenile Chinese mitten crab, Eriocheir sinensis, over 14 d of exposure to control (pH 7.4, 1364 µatm pCO2) or CO2-acidified (pH 6.8, 4633 µatm pCO2) freshwater. Data are presented as probability of survival ± SEM (N=34 for control freshwater and N=36 for acidified freshwater). Statistical significance was assessed by a Mantel–Cox test, with ∗ indicating significant difference between probability of survival between control and freshwater acidified crab populations.
3.2 Acid–base status
Chinese mitten crab maintained in control freshwater showed no changes in hemolymph pH, bicarbonate, pCO2, or ammonia throughout the experimental time course (Fig. 2; Table 2). In contrast, acidified freshwater had a significant effect on hemolymph pH, bicarbonate, pCO2, or ammonia (Fig. 2; Table 2). Exposure to acidified freshwater induced a respiratory acidosis indicated by a decline in hemolymph pH (pH 8.11±0.015 to 8.03±0.0019) and an increase in hemolymph pCO2 (404±23 to 486±26 Pa; 1 µatm = 0.101325 Pa) within the first 6 h of exposure (Fig. 2a, c). Hemolymph acidosis was maintained for 2 d. Recovery of hemolymph pH occurred by day seven, although hemolymph pCO2 remained elevated (499±20 Pa). Recovery of hemolymph pH coincided with increases in hemolymph HCO (16.7±0.78 mmol L−1) and ammonia (136±2.9 µmol L−1; Fig. 2b, d). No significant changes in hemolymph HCO and ammonia were observed until 7 and 2 d of exposure, respectively, suggesting a delayed extracellular pH regulatory response.
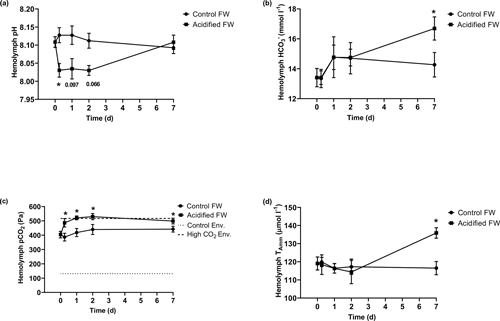
Figure 2Changes in extracellular (a) pH, (b) HCO, (c) pCO2, and (d) ammonia of juvenile Chinese mitten crabs, Eriocheir sinensis, during a 7 d time course of exposure to control (pH 7.41, 1299 µatm pCO2) or CO2-acidified (pH 6.73, 5109 µatm pCO2) freshwater. Data are presented as mean ± SEM. (N = 6–14, 2–3 crabs pooled per N value). Statistical significance was assessed by two-way ANOVA followed by a post hoc Dunnett test with ∗ indicating significant difference from day zero measurements. p values near but not <0.05 are written above the corresponding data point.
3.3 Metabolism
Metabolic changes were quantified through ammonia excretion rate and oxygen consumption rate. Ammonia excretion rate was used as an indicator of potential shifts in protein catabolism. Oxygen consumption rate was used as an indicator of changes in aerobic metabolism. Control crabs exhibited steady oxygen consumption rates and ammonia excretion rates throughout the measured time course (Fig. 3; Table 2). Crabs exposed to freshwater acidification experienced a significant reduction in oxygen consumption rate within 6 h that was maintained throughout the rest of the time course (Fig. 3a; Table 2). Ammonia excretion rates were also significantly affected by freshwater acidification (Fig. 3b; Table 2). Initially, ammonia excretion rates were unchanged until the second day of exposure (Fig. 3b). On the second day of exposure, ammonia excretion rates doubled and remained elevated for the duration of the 7 d time course (Fig. 3b).
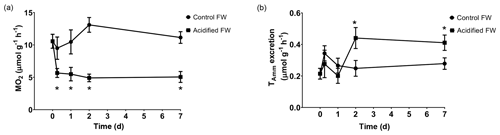
Figure 3Changes in whole-animal (a) oxygen consumption rate (MO2) and (b) ammonia excretion rate of juvenile Chinese mitten crabs, Eriocheir sinensis, during a 7 d time course of exposure to control (pH 7.41, 1299 µatm pCO2) or CO2-acidified (pH 6.73, 5109 µatm pCO2) freshwater. Data are presented as mean ± SEM. (N = 5–6 for oxygen consumption and N = 7–12 for ammonia excretion). Statistical significance was assessed by two-way ANOVA followed by a post hoc Dunnett test. Significant differences from day zero measurements are indicated by ∗. p values near but not <0.05 are written above the corresponding data point.
3.4 Carapace calcification
Changes in calcification were quantified as the change in the crab's exoskeletal calcium content following exposure to freshwater acidification conditions. Calcification was measured several times over a 6-week acclimation, as several studies on marine crustaceans report changes in calcification after 20+ d of acclimation (Long et al., 2013; Ries et al., 2009; Taylor et al., 2015). Overall, there was a significant time, pCO2, and interactive time and pCO2 effect on calcification (Table 2). Post hoc analysis suggests there were no significant changes in carapace calcification in the first 2 weeks of exposure to freshwater acidification (Fig. 4). However, after 3 and 6 weeks of exposure, a significant decline in carapace calcium content to 84.1±2.9 % and 85.2±3.3 % of control crab levels was observed (Fig. 4).
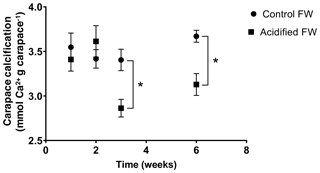
Figure 4Changes in carapace calcium content of juvenile Chinese mitten crabs, Eriocheir sinensis, over a 6-week exposure to control (pH 7.4, 1389 µatm pCO2) or CO2-acidified (pH 6.8, 4634 µatm pCO2) freshwater. Data are presented as mean ± SEM (N = 6–12). Statistical significance was assessed by two-way ANOVA followed by a post hoc Tukey HSD test, with ∗ indicating significant difference between control and acidified FW crabs for each respective week.
3.5 Locomotory behaviour assay
An open field test was used to quantify locomotory behavioural changes over a 5 min recording period in a novel arena (Table 3). Crabs exposed to acidified freshwater covered less distance than crabs in control freshwater (Student's t test, , p=0.017, Table 3). Crabs in acidified freshwater also had a lower velocity than crabs in control freshwater after the 7 d of exposure (Student's t test, , p=0.024, Table 3). Movement and mobility were also quantified. Movement was defined as the crab changing its relative location in the arena. Mobility was defined as the movement of body appendages even if the crab's location did not change. There was a significant decrease in movement (Student's t test, , p=0.015, Table 3) and mobility (Wilcoxon test, Z=2.08, p=0.037, Table 3) following the 7 d exposure to acidified freshwater.
Table 3Changes in locomotory behaviour of juvenile Chinese mitten crabs, Eriocheir sinensis, after a 7 d exposure to control (pH 7.41, 1299 µatm pCO2) or CO2-acidified (pH 6.73, 5109 µatm pCO2) freshwater. Data are presented as mean ± SEM. (N = 18–19). Statistical significance was assessed by Student's t test or the Wilcoxon test for mobility time, with * indicating significant difference between control and acidified FW treatments.

Anthropogenically driven aquatic acidification has the potential to negatively impact both freshwater and marine life. Meta-analyses of biological responses to ocean acidification suggest that marine crustaceans generally experience minimal consequences of pCO2 tensions (∼ 1000 µatm) predicted to occur by the year 2100 (Kroeker et al., 2013; Melzner et al., 2009; Wittmann and Pörtner, 2013). Acidification to levels expected for the year 2300 (∼ 2000 µatm) negatively impacts about half of the studied marine crustaceans (Wittmann and Pörtner, 2013). In contrast, the biological responses of any freshwater invertebrate to realistic future CO2-mediated freshwater acidification remain unknown. In the present study, we aimed to demonstrate the physiological and behavioural consequences of a future CO2-mediated freshwater acidification scenario on a juvenile freshwater crustacean, the Chinese mitten crab, Eriocheir sinensis. Our results suggest that freshwater juvenile Chinese mitten crabs experience significant impairment of metabolism, calcification, locomotory behaviour, and survival when exposed to freshwater acidification (4633–5109 µatm pCO2). The high energetic demands to sustain essential physiological processes such as acid–base regulation may cause energetic reallocation that impairs several physiological processes and alters animal fitness.
4.1 Plausibility of freshwater acidification conditions
Modelling of future CO2-mediated freshwater acidification for the year 2100 is nearly non-existent, making the plausibility of the pCO2 levels used in this study difficult to assess. The control pCO2 levels used in this study reflect the average pCO2 measured in 13 stations along the mainstem of the Yangtze River system (excluding Nanjing station which is at the mouth of the river and influenced by coastal upwelling) (Ran et al., 2017). The future freshwater acidification conditions used in this study represent a 3500+ µatm increase in pCO2 from control levels. This increase is roughly 1000+ µatm higher than the highest average level recorded by the 13 stations along the mainstem of the Yangtze River (Ran et al., 2017). While future CO2-mediated acidification models are not available for the Yangtze River, the relationship between changes in freshwater pCO2 in other freshwater systems as a response to changes in atmospheric pCO2 may provide indications of plausible future increases in pCO2. Weiss et al. (2018) tracked changes in pCO2 of four freshwater bodies in Germany between 1981–2015 and reported that freshwater pCO2 increased by an average of 561 µatm over this time period while atmospheric pCO2 increased by ∼ 60 µatm from 340 to 399 µatm (National Oceanic and Atmospheric Administration; https://www.esrl.noaa.gov/gmd/dv/iadv, last access: February 2021). This relationship suggests that for every 1 µatm increase in atmospheric pCO2, these freshwater bodies increased by 9.35 µatm. Since atmospheric pCO2 is projected to rise to approximately 985 µatm by the year 2100 (IPCC, 2013), this would mean that freshwater pCO2 in these systems could rise by as much as 5469 µatm. Assuming this relationship is accurate, the pCO2 levels used in this study would be within a range that could feasibly occur in the Chinese mitten crab's native environment by the year 2100. Further, it should be noted that while freshwater systems average pCO2 levels of 3100 µatm (streams and rivers) and 1410 µatm (lakes), the pCO2 levels used for acidified freshwater in this study are within ranges that can already be seen in freshwater systems globally (Raymond et al., 2013). For example, the Mackenzie, Mississippi, Ohio, and Elbe rivers, suggesting that the acidification scenario used in this study is conceivable for freshwater (Cole and Caraco, 2001; Raymond et al., 2013).
4.2 Probability of survival
Sensitivity to aquatic acidification is quite variable in marine crustaceans. In mid-intertidal to high intertidal and burrowing species including porcelain crabs (Petrolisthes cinctipes, Petrolisthes manimaculus, and Porcellana platycheles), burrowing shrimp (Upogebia deltaura), and barnacles (Semibalanus balanoides and Elminius modestus), minimal changes in survival probability are reported at pCO2 tensions ranging from 1395 to 2707 µatm (Donohue et al., 2012; Findlay et al., 2010; Page et al., 2017). Presumably, the variability in CO2 levels experienced in burrows and intertidal zones has driven the evolution of adaptation for greater CO2 tolerance in these groups of crustaceans. We predicted that juvenile Chinese mitten crabs would also have an elevated CO2 tolerance and face minimal changes in survival probability because the Yangtze River normally fluctuates by as much as 3000 µatm (Ran et al., 2017). Despite being a freshwater organism with strong ionoregulatory capabilities, our results show a sharp decrease in survival rate of Chinese mitten crabs over 14 d of exposure to 4633 µatm pCO2 (Fig. 1). Such rapid decreases in survival have also been observed in non-burrowing crustaceans or crustaceans that do not inhabit high-intertidal regions including brine shrimp (Artemia sinica), red king crab (Paralithodes camtschaticus), and low-intertidal long-clawed porcelain crab (Pisidia longicornis), exposed to 1500, 1637, and 5821 µatm pCO2, respectively (Long et al., 2013; Page et al., 2017; Zheng et al., 2015). It might be tempting to conclude that low survival in Chinese mitten crabs compared to tolerant mid-intertidal to high-intertidal and burrowing marine crustaceans is simply due to the greater pCO2 tensions used in the present study (4633 µatm). However, in the intertidal broad-clawed porcelain crab (Porcellana platycheles) pCO2 levels of 5821 µatm have been shown to not affect the probability of survival after 24 d of exposure (Page et al., 2017). It should also be mentioned that for all mortalities in this experiment there were no obvious signs of disease and intact bodies of deceased crabs were collected, suggesting that the elevated CO2 treatment and not disease or cannibalism was the reason for increased mortality. Therefore, the low survival rates in the present study suggest a high susceptibility to acidification and contradict our hypothesis that inhabiting a highly fluctuating CO2 environment would confer tolerance to future freshwater acidification.
4.3 Physiological responses
Juvenile Chinese mitten crabs effectively recovered extracellular pH following respiratory acidosis resulting from freshwater acidification by the accumulation of extracellular HCO as a buffer (Fig. 2). Compensation for acid–base homeostasis under freshwater acidification was not surprising given that strong acid–base regulatory capabilities are typically seen in highly active organisms such as fish, cephalopods, and crustaceans (Melzner et al., 2009). Similar recovery of extracellular pH to elevated environmental CO2 has also been observed in Dungeness crab (Metacarcinus magister) and velvet crab (Necora puber) exposed to even higher pCO2 tensions (10 000+ µatm; Pane and Barry, 2007; Spicer et al., 2007). In contrast, green crab (Carcinus maenas) and blue crab (Callinectes sapidus) have been shown to not fully compensate extracellular pH at 10 000+ µatm CO2 levels (Cameron, 1978; Fehsenfeld and Weihrauch, 2016). However, measurements in these species were only done over 48 h, and more time may have been required for the animals to recover, as seen in our study, where recovery was only observed after 7 d. The compensatory responses to acidosis in crustaceans generally include respiratory CO2 excretion, H+ excretion typically through Na+/H+ or NH exchange, and accumulation of extracellular HCO as a buffer, where HCO is derived through either branchial Cl− or HCO exchange and, to a lesser degree, from calcified structures (e.g. exoskeleton) (Wheatly and Henry, 1992). In freshwater crustaceans, acid–base regulation occurs mainly within the gills (Henry et al., 2012), where the Na+/K+-ATPase and H+-ATPase generate the electrochemical gradients that drive ion exchange (Leone et al., 2017). The Na+/K+-ATPase alone may already account for over 20 % of an animal's energetic budget (Milligan and McBride, 1985). The increase in ion transport that must occur to re-establish and maintain acid–base homeostasis in the face of freshwater acidification could pose an increased energetic demand. In fact, in sea urchin larvae pCO2 tensions of 800 µatm have been shown to double ion transport ATP demands (Pan et al., 2015). It is therefore conceivable that the energetic cost for long-term maintenance of acid–base homeostasis under freshwater acidification may come at substantial energetic cost, which could have negative implications on other physiological parameters and thereby animal fitness.
Heightened energetic demands to maintain crucial physiological processes during exposure to environmental CO2 acidification can occur through reallocation of energy budgets or through modification of metabolism to increase energy supplies. In fact, in the marine brittle star, Amphiura filiformis, exposure to CO2 tensions ranging from 1000 to 8000 µatm for 40 d caused an increase in metabolic rate (increased energy budget) (Wood et al., 2008). This metabolic change was postulated to fuel increased calcification observed in this species (Wood et al., 2008). In contrast, the metabolic rate of juvenile European lobster (Homarus gammarus) remained unchanged when exposed to 1100 and 8000 µatm CO2 (Small et al., 2020). However, in H. gammarus, branchial Na+/K+ ATPase activity was increased, demonstrating a reallocation of energy supplies despite maintaining an unchanged energy budget (Small et al., 2020). Unlike juvenile European lobster and brittle star, juvenile Chinese mitten crabs experienced a decrease in oxygen consumption (potentially decreased energy budget). Despite reductions in oxygen consumption, crabs could still re-establish extracellular pH through HCO accumulation, suggesting a potential reallocation of energy supplies to essential ionoregulatory processes.
Typically, a reduction in oxygen consumption, as seen in the present study, is observed when an organism cannot compensate for a reduction in extracellular pH (Pörtner et al., 2004). While in juvenile Chinese mitten crabs, this could be the case at the initial 2 d of the time course, by day 7 extracellular pH was fully compensated for, yet oxygen consumption rates were reduced. It is known that high environmental pCO2 levels can trigger an accumulation of compounds such as adenosine that can lead to reduced oxygen consumption as observed in the peanut worm, Sipunculus nudus (Reipschläger et al., 1997). A similar mechanism could conceivably be in place that led to reduced oxygen consumption in the Chinese mitten crab as a strategy to conserve energy supplies to promote survival upon exposure to short-term stressors like high environmental pCO2 levels. Such an adaptation may be present in Chinese mitten crab as these crabs would regularly experience short-term fluctuations in environmental CO2 of their natural habitat. In fact, in the Mediterranean mussel (Mytilus galloprovincialis) chronically reduced oxygen consumption rates lasting up to 90 d have been observed to allow survival following exposure to ocean acidification (5026 µatm pCO2; Michaelidis et al., 2005). Reducing oxygen consumption is a viable strategy used by many organisms to survive short-term periods of environmental stress (Guppy and Withers, 1999). However, it is a less viable long-term strategy as reduction in metabolic rate reduces energy availability for costly physiological processes such as calcification and protein synthesis which would ultimately affect growth and reproductive success as reported in freshwater pink salmon (Oncorhynchus gorbuscha) and marine amphipod (Gammarus locusta) (Borges et al., 2018; Ou et al., 2015).
Besides reduced oxygen consumption, freshwater acidification led to an increase in extracellular concentrations and excretion of ammonia, a metabolic product of protein catabolism. Elevated excretion of ammonia may function as an excretable acid equivalent to assist the maintenance of pH homeostasis, a mechanism suggested for the brackish water green crab (Carcinus maenas) and hydrothermal vent crab (Xenograpsus testudinatus) (Allen et al., 2020; Fehsenfeld and Weihrauch, 2013). Furthermore, the previously mentioned reduction in oxygen consumption and increased ammonia excretion (decrease in O : N ratio) indicate that juvenile Chinese mitten crabs have a greater reliance on protein catabolism as an energy source under elevated environmental CO2. Similar decreases in oxygen consumption and increases in ammonia excretion have been observed in the Mediterranean mussel (M. galloprovincialis, 5026 µatm pCO2, 15–90 d) and brittle star (A. filiformis, 6643 µatm pCO2, 28 d), where catabolism of amino acid such as glutamine may provide metabolic bicarbonate to further help sustain pH homeostasis (Hu et al., 2014; Michaelidis et al., 2005). While potentially beneficial for sustaining acid–base status, elevated protein catabolism requires a consistent source of protein through either a high-protein diet or increased food consumption, which if not met could cause muscle wastage, an effect seen in brittle stars during heightened energetic demands of ocean acidification (Wood et al., 2008). Interestingly, feeding rate has been shown in juvenile European lobster (H. gammarus) and green crab (C. maenas) to decline as a result of elevated environmental CO2, making a greater reliance on protein catabolism during energetically constricted times a potentially precarious situation for juvenile Chinese mitten crabs (Appelhans et al., 2012; Small et al., 2020).
Carapace calcification is an energetically costly process related to growth and predation defence in crustaceans that freshwater acidification and the associated metabolic changes could impair. Decapod crustaceans are believed to be the least susceptible of calcifying organisms to aquatic acidification as their exoskeletal CaCO3 exists in the more stable calcite form, providing greater resilience to dissolution in contrast to bivalves and corals (Ries et al., 2009). Indeed, the marine crustacean carapace is well protected from aquatic acidification-mediated dissolution with reports of either no change or an increase in calcification being typically observed (Kroeker et al., 2013; Ries et al., 2009; Whiteley, 2011). However, in the present study, juvenile Chinese mitten crabs had reduced levels of carapace calcification as reflected by a lower carapace calcium content after 3 and 6 weeks of exposure (Fig. 4). While not as common, examples of reductions in carapace calcification have been observed in marine crustaceans, including several porcelain crabs and the tanner crab, Chionoecetes bairdi (Long et al., 2013; Page et al., 2017). In crustaceans, carapace dissolution may occur to support extracellular pH buffering that normally occurs through branchial HCO uptake by providing an alternative source of HCO (Cameron, 1985; Defur et al., 1980). In the present study, extracellular pH was recovered long before carapace dissolution was apparent; therefore it is less likely that the carapace is mobilized as a source of HCO. Instead, reductions in carapace calcium content most likely reflect an alteration in the rate of calcification or acid-mediated dissolution of the carapace. As carapace formation and maintenance are energetically expensive processes requiring careful ion regulation by numerous organs, the aforementioned changes in whole-animal energetics due to freshwater acidification could have negative implications on animal fitness either by weakening the exoskeleton or impairing post-moult calcification, which can hamper growth and leave animals vulnerable to predation.
4.4 Behavioural responses
Elevated freshwater pCO2 altered locomotory behaviour in juvenile Chinese mitten crabs. Crabs in acidified freshwater covered less total distance during movement and did so at a lower velocity. No studies have previously examined changes in crustacean distance covered in the presence of elevated environmental CO2. However, reduced speed of movement has also been reported in Shiba shrimp (Metapenaeus joyneri) exposed to CO2 levels of 9079 µatm; however, unlike in Chinese mitten crabs, this shrimp did not experience a reduction in oxygen consumption rate correlated with locomotory impairment (Dissanayake and Ishimatsu, 2011). While not measured in our study, in Shiba shrimp there was a reduction in aerobic scope, which would likely lead to reduced aerobic performance and reduced movement (Dissanayake and Ishimatsu, 2011). Similar alterations in aerobic scope could partially be behind the reductions in velocity seen in juvenile Chinese mitten crabs; however, this is entirely speculative and there are many cases where elevated CO2 does not alter aerobic scope (Lefevre, 2016). In addition to moving slower, Chinese mitten crab spent less time moving their entire body throughout the novel arena and less time moving only their appendages while staying at a fixed location. Reduced movement time and appendage movement were also seen in the hermit crab (Pagurus bernhardus) exposed to 12 000 µatm CO2 (de la Haye et al., 2011). In contrast, the isopod (Paradella dianae) experienced no change in swim time or crawling time when exposed to 2085 µatm CO2 despite a measured metabolic depression (Alenius and Munguia, 2012). Differences in the effect of CO2 on movement time may result from the CO2 levels employed, but further studies on a greater variety of species are required to determine potential patterns for crustaceans. It is plausible that overall locomotory behaviour is reduced in this study due to alterations in neurological function resulting from ionic imbalances or other CO2-mediated effects that may occur from elevated environmental CO2 (for a review of neural effects of aquatic acidification, see Tresguerres and Hamilton, 2017). With a potential reduction in overall energy availability, crabs may reduce energy expenditure through locomotion to conserve energy stores for physiological processes more crucial to surviving the physiological distress caused by freshwater acidification. The overall reductions in locomotion observed in juvenile Chinese mitten crabs could have negative consequences for their survival, as reduced movement would make these crabs more vulnerable to predation, reduce migratory capabilities, and reduce foraging ability.
In conclusion, we found impairment of survival, metabolism, calcification, and locomotion with exposure to a potential future CO2-mediated freshwater acidification scenario. Energy availability was reduced despite heightened ionoregulatory energetic demands. Changes in the animals' energy budgets likely result in a greater dependency on protein catabolism as an energy source to allow for extracellular pH recovery at the cost of reducing their exoskeletal calcification and locomotion. We found that despite successful acid–base compensation, survival rates declined, with a 3.8 times greater probability of mortality under acidified freshwater conditions. While our study suggests negative impacts of freshwater acidification, these results should be assessed with caution as the assumed acidification levels are based on a relationship between changes in atmospheric CO2 and freshwater CO2 which must still be more effectively modelled. Nevertheless, this study shows that despite inhabiting an environment that experiences regular fluctuations in pCO2, the Chinese mitten crab may be at risk under future freshwater acidification. This emphasizes the importance of modelling acidification in freshwater systems to accurately assess biological consequences of global change. Based on our findings that a physiologically robust species displays sensitivity to future freshwater acidification, further research investigating the effect of freshwater acidification on a wide range of freshwater species from all phyla is required to better identify the effects of anthropogenic CO2 accumulation on freshwater ecosystems.
Data are available at the following link: https://doi.org/10.6084/m9.figshare.13888034 (Quijada-Rodriguez et al., 2021).
ARQR designed the study, performed experiments, analyzed data, and wrote the manuscript. PLK, PHS, and MTH performed experiments. GJPA analyzed data and assisted with writing. PPH provided financial support and analytical tools. YCT assisted in designing the study and writing the manuscript and provided financial support and analytical tools. DW assisted in designing the study and writing the manuscript and provided financial support and analytical tools.
The contact author has declared that neither they nor their co-authors have any competing interests.
Publisher’s note: Copernicus Publications remains neutral with regard to jurisdictional claims in published maps and institutional affiliations.
The authors would like to thank the technicians and students of Pung Pung Hwang's lab for assistance with animal care.
Research was supported by the National Science and Engineering Research Council of Canada (NSERC DG; Dirk Weihrauch, grant no. RGPIN-2018-05013), the Ministry of Science and Technology, Taiwan, Republic of China (MOST 108=2621-M-001-003; Yung-Che Tseng), and NSERC's postgraduate scholarship doctorate and University of Manitoba Graduate Fellowship (Alex R. Quijada-Rodriguez and Garett J. P. Allen).
This paper was edited by Kenneth Rose and reviewed by three anonymous referees.
Alenius, B. and Munguia, P.: Effects of pH variability on the intertidal isopod, Paradella dianae, Mar. Freshw. Behav. Phy., 45, 245–259, https://doi.org/10.1080/10236244.2012.727235, 2012.
Allen, G. J. P., Kuan, P. L., Tseng, Y. C., Hwang, P. P., Quijada-Rodriguez, A. R., and Weihrauch, D.: Specialized adaptations allow vent-endemic crabs (Xenograpsus testudinatus) to thrive under extreme environmental hypercapnia, Sci. Rep.-UK, 10, 1–13, https://doi.org/10.1038/s41598-020-68656-1, 2020.
Appelhans, Y. S., Thomsen, J., Pansch, C., Melzner, F., and Wahl, M.: Sour times: Seawater acidification effects on growth, feeding behaviour and acid-base status of Asterias rubens and Carcinus maenas, Mar. Ecol.-Prog. Ser., 459, 85–97, https://doi.org/10.3354/meps09697, 2012.
Balian, E. V., Segers, H., Lévèque, C., and Martens, K.: The Freshwater Animal Diversity Assessment: an overview of the results, Hydrobiologia, 595, 627–637, https://doi.org/10.1007/s10750-007-9246-3, 2008.
Borges, F. O., Figueiredo, C., Sampaio, E., Rosa, R., and Grilo, T. F.: Transgenerational deleterious effects of ocean acidification on the reproductive success of a keystone crustacean (Gammarus locusta), Mar. Environ. Res., 138, 55–64, https://doi.org/10.1016/j.marenvres.2018.04.006, 2018.
Cameron, J. N.: Effects of hypercapnia on blood acid-base status, NaCl fluxes, and trans-gill potential in freshwater blue crabs, Callinectes sapidus, J. Comp. Physiol. B, 123, 137–141, https://doi.org/10.1007/BF00687841, 1978.
Cameron, J. N.: Compensation of hypercapnic acidosis in the aquatic blue crab, Callinectes Sapidus: The predominance of external sea water over carapace carbonate as the proton sink, J. Exp. Biol., 114, 197–206, 1985.
Cole, J. J. and Caraco, N. F.: Carbon in catchments: connecting terrestrial carbon losses with aquatic metabolism, Marine Freshwater Res., 52, 101–110, https://doi.org/10.1071/MF00084, 2001.
Cumberlidge, N., Ng, P. K. L., Yeo, D. C. J., Magalhães, C., and Campos, M. R.: Freshwater crabs and the biodiversity crisis: Importance, threats, status, and conservation challenges, Biol. Conserv., 142, 1665–1673, https://doi.org/10.4324/9780203114261, 2009.
Czerniejewski, P., Rybczyk, A., and Wawrzyniak, W.: Diet of the Chinese mitten crab, Eriocheir sinensis H. Milne Edwards, 1853, and potential effects of the crab on the aquatic community in the River Odra/Oder estuary (N.-W. Poland), Crustaceana, 83, 195–205, https://doi.org/10.1163/001121609X12591347509202, 2010.
David, A. A., Pettit, L., and Edmund, M.: Resilience of a highly invasive freshwater gastropod, viviparus georgianus (Caenogastropoda: Viviparidae), to CO2-induced acidification, J. Molluscan Stud., 86, 259–262, https://doi.org/10.1093/MOLLUS/EYAA008, 2020.
Defur, P. L., Wilkes, P. R. H., and McMahon, B. R.: Non-equilibrium acid-base status in C. productus: Role of exoskeletal carbonate buffers, Resp. Physiol., 42, 247–261, https://doi.org/10.1016/0034-5687(80)90118-8, 1980.
de la Haye, K. L., Spicer, J. I., Widdicombe, S., and Briffa, M.: Reduced sea water pH disrupts resource assessment and decision making in the hermit crab Pagurus bernhardus, Anim. Behav., 82, 495–501, https://doi.org/10.1016/j.anbehav.2011.05.030, 2011.
Dickson, A. G.: Standard potential of the reaction: –AgCl(s)+1/2HAg(s)+HCl(aq) and the standard acidity constant of the ion HSO in synthetic sea water from 273.15 to 318.15 K, J. Chem. Thermodynam., 22, 113–127, https://doi.org/10.1016/0021-9614(90)90074-Z, 1990.
Dissanayake, A. and Ishimatsu, A.: Synergistic effects of elevated CO2 and temperature on the metabolic scope and activity in a shallow-water coastal decapod (Metapenaeus joyneri; Crustacea: Penaeidae), ICES J. Mar. Sci., 68, 1147–1154, https://doi.org/10.1093/icesjms/fsq188, 2011.
Donohue, P. J. C., Calosi, P., Bates, A. H., Laverock, B., Rastrick, S., Mark, F. C., Strobel, A., and Widdicombe, S.: Impact of exposure to elevated pCO2 on the physiology and Behaviour of an important ecosystem engineer, the burrowing shrimp Upogebia deltaura, Aquat. Biol., 15, 73–86, https://doi.org/10.3354/ab00408, 2012.
FAO: The State of World Fisheries and Aquaculture 2018 – Meeting the sustainable development goals, FAO, Rome, 2018.
Feely, R. A., Sabine, C. L., Lee, K., Berelson, W., Kleypas, J., Fabry, V. J., and Millero, F. J.: Impact of anthropogenic CO2 on the CaCO3 system in the oceans, Science, 305, 362–366, https://doi.org/10.1126/science.1097329, 2004.
Fehsenfeld, S. and Weihrauch, D.: Differential acid-base regulation in various gills of the green crab Carcinus maenas: Effects of elevated environmental pCO2, Comp. Biochem. Phys. A, 164, 54–65, https://doi.org/10.1016/j.cbpa.2012.09.016, 2013.
Fehsenfeld, S. and Weihrauch, D.: Mechanisms of acid – base regulation in seawater-acclimated green crabs (Carcinus maenas), Can. J. Zool., 94, 95–107, 2016.
Findlay, H. S., Kendall, M. A., Spicer, J. I., and Widdicombe, S.: Post-larval development of two intertidal barnacles at elevated CO2 and temperature, Mar. Biol., 157, 725–735, https://doi.org/10.1007/s00227-009-1356-1, 2010.
Florence, T. M. and Farrar, Y. J.: Spectrophotometric determination of chloride at the parts-per-billion level by the mercury(II) thiocyanate method, Anal. Chim. Acta, 54, 373–377, https://doi.org/10.1016/S0003-2670(01)82142-5, 1971.
Guppy, M. and Withers, P.: Metabolic depression in animals: Physiological perspectives and biochemical generalizations, Biol. Rev., 74, 1–40, https://doi.org/10.1111/j.1469-185X.1999.tb00180.x, 1999.
Hans, S., Fehsenfeld, S., Treberg, J. R., and Weihrauch, D.: Acid–base regulation in the Dungeness crab (Metacarcinus magister), Mar. Biol., 161, 1179–1193, https://doi.org/10.1007/s00227-014-2409-7, 2014.
Hasler, C. T., Butman, D., Jeffrey, J. D., and Suski, C. D.: Freshwater biota and rising pCO2?, Ecol. Lett., 19, 98–108, https://doi.org/10.1111/ele.12549, 2016.
Henry, R. P., Lucu, C., Onken, H., and Weihrauch, D.: Multiple functions of the crustacean gill: osmotic/ionic regulation, acid-base balance, ammonia excretion, and bioaccumulation of toxic metals, Front. Physiol., 3, 431, https://doi.org/10.3389/fphys.2012.00431, 2012.
Holmes, R. M., Aminot, A., Kérouel, R., Hooker, B. A., and Peterson, B. J.: A simple and precise method for measuring ammonium in marine and freshwater ecosystems, Can. J. Fish. Aquat. Sci., 56, 1801–1808, https://doi.org/10.1139/f99-128, 1999.
Hu, M. Y., Casties, I., Stumpp, M., Ortega-Martinez, O., and Dupont, S.: Energy metabolism and regeneration are impaired by seawater acidification in the infaunal brittlestar Amphiura filiformis, J. Exp. Biol., 217, 2411–2421, https://doi.org/10.1242/jeb.100024, 2014.
IPCC: Climate Change 2013: The Physical Science Basis. Contribution of Working Group I to the Fifth Assessment Report of the Intergovernmental Panel on Climate Change, edited by: Stocker, T. F., Qin, D., Plattner, G.-K., Tignor, M., Allen, S. K., Boschung, J., Nauels, A., Xia, Y., Bex, V., and Midgley, P. M., Cambridge University Press, Cambridge, United Kingdom and New York, NY, USA, 1535 pp., 2013.
Jeffrey, J. D., Hannan, K. D., Hasler, C. T., and Suski, C. D.: Chronic exposure of a freshwater mussel to elevated pCO2: Effects on the control of biomineralization and ion-regulatory responses, Environ. Toxicol. Chem., 37, 538–550, https://doi.org/10.1002/etc.3991, 2018a.
Jeffrey, J. D., Hannan, K. D., Hasler, C. T., and Suski, C. D.: Hot and bothered: Effects of elevated pCO2 and temperature on juvenile freshwater mussels, Am. J. Phys.-Reg. I., 315, R115–R127, https://doi.org/10.1152/ajpregu.00238.2017, 2018b.
Kroeker, K. J., Kordas, R. L., Crim, R., Hendriks, I. E., Ramajo, L., Singh, G. S., Duarte, C. M., and Gattuso, J.-P.: Impacts of ocean acidification on marine organisms: quantifying sensitivities and interaction with warming, Glob. Change Biol., 19, 1884–1896, https://doi.org/10.1111/gcb.12179, 2013.
Lefevre, S.: Are global warming and ocean acidification conspiring against marine ectotherms? A meta-analysis of the respiratory effects of elevated temperature, high CO2 and their interaction, Conserv. Physiol., 4, 1–31, https://doi.org/10.1093/conphys/cow009, 2016.
Leone, F. A., Lucena, M. N., Garçon, D. P., Pinto, M. R., and McNamara, J. C.: Gill Ion Transport ATPases and Ammonia Excretion in Aquatic Crustaceans, in: Acid-Base Balance and Nitrogen Excretion in Invertebrates, edited by: Weihrauch, D. and O'Donnell, M., Springer, Cham., https://doi.org/10.1007/978-3-319-39617-0_3, 2017.
Lewis, E. and Wallace, D. W. R.: Program Developed for CO2 System Calculations, Oak Ridge National Laboratory, Oak Ridge, TN, ORNL/CDIAC-105, 1998.
Long, W. C., Swiney, K. M., Harris, C., Page, H. N., and Foy, R. J.: Effects of Ocean Acidification on Juvenile Red King Crab (Paralithodes camtschaticus) and Tanner Crab (Chionoecetes bairdi) Growth, Condition, Calcification, and Survival, PLoS One, 8, e60959, https://doi.org/10.1371/journal.pone.0060959, 2013.
Melzner, F., Gutowska, M. A., Langenbuch, M., Dupont, S., Lucassen, M., Thorndyke, M. C., Bleich, M., and Pörtner, H.-O.: Physiological basis for high CO2 tolerance in marine ectothermic animals: pre-adaptation through lifestyle and ontogeny?, Biogeosciences, 6, 2313–2331, https://doi.org/10.5194/bg-6-2313-2009, 2009.
Michaelidis, B., Ouzounis, C., Paleras, A., and Pörtner, H.-O.: Effects of long-term moderate hypercapnia on acid-base balance and growth rate in marine mussels Mytilus galloprovincialis, Mar. Ecol.-Prog. Ser., 293, 109–118, 2005.
Millero, F. J.: The thermodynamics of the carbonate system in seawater, Geochim. Cosmochim. Ac., 43, 1651–1661, https://doi.org/10.1016/0016-7037(79)90184-4, 1979.
Milligan, L. P. and McBride, B. W.: Shifts in Animal Energy Requirements across Physiological and Alimentational States: Energy Costs of Ion Pumping by Animal Tissues, J. Nutr., 115, 1374–1382, 1985.
Ou, M., Hamilton, T. J., Eom, J., Lyall, E. M., Gallup, J., Jiang, A., Lee, J., Close, D. A., Yun, S.-S., and Brauner, C. J.: Responses of pink salmon to CO2-induced aquatic acidification, Nat. Clim. Change, 5, 950–955, https://doi.org/10.1038/nclimate2694, 2015.
Page, T. M., Worthington, S., Calosi, P., and Stillman, J. H.: Effects of elevated pCO2 on crab survival and exoskeleton composition depend on shell function and species distribution: A comparative analysis of carapace and claw mineralogy across four porcelain crab species from different habitats, ICES J. Mar. Sci., 74, 1021–1032, https://doi.org/10.1093/icesjms/fsw196, 2017.
Pan, T. C. F., Applebaum, S. L., and Manahan, D. T.: Experimental ocean acidification alters the allocation of metabolic energy, P. Natl. Acad. Sci. USA, 112, 4696–4701, https://doi.org/10.1073/pnas.1416967112, 2015.
Pane, E. F. and Barry, J. P.: Extracellular acid-base regulation during short-term hypercapnia is effective in a shallow-water crab, but ineffective in a deep-sea crab, Mar. Ecol.-Prog. Ser., 334, 1–9, https://doi.org/10.3354/meps334001, 2007.
Phillips, J. C., Mckinley, G. A., Bennington, V., Bootsma, H. A., Pilcher, D. J., Sterner, R., and Urban, N. R.: The Potential for CO2-Induced Acidification in Freshwater: A Great Lakes Case Study, Oceanography, 28, 136–145, 2015.
Pörtner, H. O., Langenbuch, M., and Reipschläger, A.: Biological impact of elevated ocean CO2 concentrations: Lessons from animal physiology and earth history, J. Oceanogr., 60, 705–718, https://doi.org/10.1007/s10872-004-5763-0, 2004.
Quijada-Rodriguez, A., Kuan, P.-L., Sung, P.-H., Hsu, M.-T., Allen, G., Hwang, P. P., Tseng, Y.-C., and Weihrauch, D.: Anthropogenic CO2-mediated freshwater acidification limits survival, calcification, metabolism, and behaviour in stress-tolerant freshwater crustaceans, figshare [data set], https://doi.org/10.6084/m9.figshare.13888034.v1, 2021.
Ran, L., Lu, X. X., and Liu, S.: Dynamics of riverine CO2 in the Yangtze River fluvial network and their implications for carbon evasion, Biogeosciences, 14, 2183–2198, https://doi.org/10.5194/bg-14-2183-2017, 2017
Raymond, P. A., Hartmann, J., Lauerwald, R., Sobek, S., McDonald, C., Hoover, M., Butman, D., Striegl, R., Mayorga, E., Humborg, C., Kortelainen, P., Dürr, H., Meybeck, M., Ciais, P., and Guth, P.: Global carbon dioxide emissions from inland waters, Nature, 503, 355–359, https://doi.org/10.1038/nature12760, 2013.
Reipschläger, A., Nilsson, G. E. and Pörtner, H. O.: A role for adenosine in metabolic depression in the marine invertebrate Sipunculus nudus, Am. J. Phys.-Reg. I., 272, R350–R356, https://doi.org/10.1152/ajpregu.1997.272.1.r350, 1997.
Ries, J. B., Cohen, A. L., and McCorkle, D. C.: Marine calcifiers exhibit mixed responses to CO2-induced ocean acidification, Geology, 37, 1131–1134, https://doi.org/10.1130/G30210A.1, 2009.
Robertson, M. D., Hernandez, M. F., Midway, S. R., Hasler, C. T., and Suski, C. D.: Shelter-seeking behavior of crayfish, Procambarus clarkii, in elevated carbon dioxide, Aquat. Ecol., 52, 225–233, https://doi.org/10.1007/s10452-018-9657-4, 2018.
Roleda, M. Y., Boyd, P. W., and Hurd, C. L.: Before ocean acidification: Calcifier chemistry lessons, J. Phycol., 48, 840–843, https://doi.org/10.1111/j.1529-8817.2012.01195.x, 2012.
Sarazin, G., Michard, G., and Prevot, F.: A rapid and accurate spectroscopic method for alkalinity measurements in sea water samples, Water Res., 33, 290–294, https://doi.org/10.1016/S0043-1354(98)00168-7, 1999.
Small, D. P., Calosi, P., Rastrick, S. P. S., Turner, L. M., Widdicombe, S. and Spicer, J. I.: The effects of elevated temperature and pCO2 on the energetics and haemolymph pH homeostasis of juveniles of the European lobster, Homarus gammarus, J. Exp. Biol., 223, 1–10, https://doi.org/10.1242/jeb.209221, 2020.
Spicer, J. I. and Eriksson, S. P.: Does the development of respiratory regulation always accompany the transition from pelagic larvae to benthic fossorial postlarvae in the Norway lobster Nephrops norvegicus (L.)?, J. Exp. Mar. Biol. Ecol., 295, 219–243, https://doi.org/10.1016/S0022-0981(03)00296-X, 2003.
Spicer, J. I., Raffo, A., and Widdicombe, S.: Influence of CO2-related seawater acidification on extracellular acid-base balance in the velvet swimming crab Necora puber, Mar. Biol., 151, 1117–1125, https://doi.org/10.1007/s00227-006-0551-6, 2007.
Tacon, A. G. J.: Trends in Global Aquaculture and Aquafeed Production: 2000–2017, Rev. Fish. Sci. Aquac., 28, 43–56, https://doi.org/10.1080/23308249.2019.1649634, 2020.
Taylor, J. R. A., Gilleard, J. M., Allen, M. C., and Deheyn, D. D.: Effects of CO2-induced pH reduction on the exoskeleton structure and biophotonic properties of the shrimp Lysmata californica, Sci. Rep.-UK, 5, 1–12, https://doi.org/10.1038/srep10608, 2015.
Tresguerres, M. and Hamilton, T. J.: Acid-base physiology, neurobiology and behaviour in relation to CO2-induced ocean acidification, J. Exp. Biol., 220, 2136–2148, https://doi.org/10.1242/jeb.144113, 2017.
Truchot, J. P.: Carbon dioxide combing properties of the blood of the shore crab Carcinus maenas (L.): Carbon dioxide solubility coefficieny and carbonic acid dissociation constants, J. Exp. Biol., 64, 45–57, 1976.
Truchot, J. P.: Acid-base changes on transfer between sea- and freshwater in the Chinese crab, Eriocheir sinensis, Resp. Physiol., 87, 419–427, 1992.
Veilleux, E. and de Lafontaine, Y.: Biological synopsis of the Chinese mitten crab (Eriocheir sinensis), Can. Manusc. Rep. Fish. Aquat. Sci., 2812, vi+45p. ii, 2007.
Weiss, L. C., Pötter, L., Steiger, A., Kruppert, S., Frost, U., and Tollrian, R.: Rising pCO2 in Freshwater Ecosystems Has the Potential to Negatively Affect Predator-Induced Defenses in Daphnia, Curr. Biol., 28, 327–332.e3, https://doi.org/10.1016/j.cub.2017.12.022, 2018.
Wheatly, M. G. and Henry, R. P.: Extracellular and intracellular acid-base regulation in crustaceans, J. Exp. Zool., 263, 127–142, https://doi.org/10.1002/jez.1402630204, 1992.
Whiteley, N. M.: Physiological and ecological responses of crustaceans to ocean acidification, Mar. Ecol.-Prog. Ser., 430, 257–271, https://doi.org/10.3354/meps09185, 2011.
Wittmann, A. C. and Pörtner, H. O.: Sensitivities of extant animal taxa to ocean acidification, Nat. Clim. Change, 3, 995–1001, https://doi.org/10.1038/nclimate1982, 2013.
Wood, H. L., Spicer, J. I., and Widdicombe, S.: Ocean acidification may increase calcification rates, but at a cost, P. Biol. Sci., 275, 1767–73, https://doi.org/10.1098/rspb.2008.0343, 2008.
Zheng, C., Jeswin, J., Shen, K., Lablche, M., Wang, K., and Liu, H.: Detrimental effect of CO2-driven seawater acidification on a crustacean brine shrimp, Artemia sinica, Fish Shellfish Immun., 43, 181–190, https://doi.org/10.1016/j.fsi.2014.12.027, 2015.