the Creative Commons Attribution 4.0 License.
the Creative Commons Attribution 4.0 License.
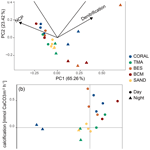
Quantifying functional consequences of habitat degradation on a Caribbean coral reef
Alice E. Webb
Didier M. de Bakker
Karline Soetaert
Tamara da Costa
Steven M. A. C. van Heuven
Fleur C. van Duyl
Gert-Jan Reichart
Lennart J. de Nooijer
Coral reefs are declining worldwide. The abundance of corals has decreased alongside a rise of filter feeders, turf, and algae in response to intensifying human pressures. This shift in prevalence of functional groups alters the biogeochemical processes in tropical water ecosystems, thereby influencing reef functioning. An urgent challenge is to understand the functional consequences of these shifts to develop suitable management strategies that aim at preserving the biological functions of reefs.
Here, we quantify biogeochemical processes supporting key reef functions (i.e. net community calcification (NCC) and production (NCP) and nutrient recycling) in situ for five different benthic assemblages currently dominating shallow degraded Caribbean reef habitats. To this end, a transparent custom-made enclosure was placed over communities dominated by either one of five functional groups – coral, turf and macroalgae, bioeroding sponges, cyanobacterial mats, or sand – to determine chemical fluxes between these communities and the overlying water, during both day and night. To account for the simultaneous influence that distinct biogeochemical processes have on measured variables, the rates were then derived by solving a model consisting of differential equations describing the contribution of each process to the measured chemical fluxes.
Inferred rates were low compared to those known for reef flats worldwide. Reduced accretion potential was recorded, with negative or very modest net community calcification rates for all communities. Net production during the day was also low, suggesting limited accumulation of biomass through photosynthesis and remineralisation of organic matter at night was relatively high in comparison, resulting in net heterotrophy over the survey period for most communities. Estimated recycling processes (i.e. nitrification and denitrification) were high but did not fully counterbalance nutrient release from aerobic mineralisation, rendering all substrates sources of nitrogen. Results suggest similar directions and magnitudes of key biogeochemical processes of distinct communities on this shallow Curaçaoan reef. We infer that the amount and type of organic matter released by abundant algal turfs and cyanobacterial mats on this reef likely enhances heterotroph activity and stimulates the proliferation of less diverse copiotrophic microbial populations, rendering the studied reef net heterotrophic and drawing the biogeochemical “behaviour” of distinct communities closer to each other.
- Article
(4551 KB) -
Supplement
(607 KB) - BibTeX
- EndNote
Community composition and biodiversity across all kinds of ecosystems are responding to escalating anthropogenic activities (McGill et al., 2015). In both terrestrial and aquatic systems, climate change, pollution and habitat fragmentation have promoted the expansion of opportunistic and tolerant species and the elimination of more sensitive yet key specialists (Clavel et al., 2011). Communities within ecosystems and across spatial scales have become more biologically homogeneous (Burman et al., 2012; Cramer et al., 2021), which may lead to a decrease in functional diversity, therefore limiting services provided by biological communities (Matsuzaki et al., 2013; White et al., 2018). Additionally, this may cause synchronisation of the biological response to new or intensified anthropogenic pressures across local communities, thus reducing resilience of metacommunities (Tobias and Monika, 2012; Sonnier et al., 2014; Petsch et al., 2020).
Coral reefs support immense biodiversity and provide important ecosystem services to millions of people (Moberg and Folke, 1999). They are, however, in global decline as they are experiencing major loss in coral abundance and shifts in species composition in response to increasing human pressures and accelerating rates of environmental and climate change (Koop et al., 2001; Langdon and Atkinson, 2005; Andersson and Gledhill, 2013; De'ath et al., 2012; Chen et al., 2015). Returning degraded reefs to their original state is, in many cases, no longer an option (Hughes et al., 2017). Instead, today's challenge is to guide coral reefs through this transition while identifying and securing the ecosystem functions that underpin resilience and services of modern reef assemblages (Oliver et al., 2015).
This is particularly relevant for the depauperate reef systems in the Caribbean, which have, since the early 1970s, undergone considerable reorganisation with regards to community composition and structural appearance (Gardner et al., 2003; Jackson et al., 2014). The communities encountered on these reefs bear little resemblance to the systems once dominated by reef-building Acropora spp. and Orbicella spp. (van Duyl, 1985; Alvarez-Filip et al., 2009). Major declines in the abundance of these species severely compromise reef function as they were the main drivers of critical processes including carbonate accretion, productivity, and structural complexity (Wolfe et al., 2020). This has led to low functional redundancy on Caribbean reefs, i.e. a reduced capacity of one or more species to functionally compensate for the loss of another, which makes them particularly vulnerable (Bellwood et al., 2003; McWilliam et al., 2018). On many reefs, areas covered by turf assemblages and macroalgae, excavating sponges, cyanobacteria, rubble, and sand have increased alongside the decrease in stony corals (Aronson et al., 2005; Burman et al., 2012; Cramer et al., 2021). Although changes in community composition are well documented as they can be followed by monitoring the coverage of the various benthic taxa over time (Barott et al., 2012; de Bakker et al., 2016, 2017), assessing the impact of these shifts on the community ecophysiology in situ has proven more challenging.
The keystones of coral reef functioning include provision of a structural habitat through carbonate deposition, production and assimilation of biomass produced through photosynthesis, and efficient cycling of nutrients within the ecosystem (Brandl et al., 2019). The biogeochemical processes that underlie these key functions are primary production, aerobic mineralisation, calcification, bioerosion, and nutrient release/uptake. Complementary to conventional monitoring efforts, quantification of the net budgets of these processes will provide insight into how reef degradation and community reorganisation affect reef functioning (Brandl et al., 2019, Bellwood et al., 2019). However, obtaining accurate in situ measurements while accounting for the complexity of interactions between processes can render their quantification rather complicated.
In environments where the flow of water over the reef is relatively linear, the upstream/downstream method can be performed (Shaw et al., 2014; Koweek et al., 2015; Albright et al., 2016, 2018). For flow regimes that are not unidirectional, factors such as water residence time and biochemical and hydrological offshore conditions need to be considered (Courtney et al., 2016). When conditions allow build-up of considerable chemical vertical gradients, net fluxes (of, for example, nutrients) can be measured (McGillis et al., 2011; Takeshita et al., 2016). On fully exposed reefs where virtually no detectable accumulation occurs over the reef flat – even within the boundary layer – incubating communities allow for the quantification of the fluxes into and out of the overlying water. Presently, efforts to quantify community processes have focused on individual functional groups (Brocke et al., 2015, 2018; den Haan et al., 2016; Webb et al., 2017; de Bakker et al., 2018) or on reconstructed communities ex situ (e.g. Dove et al., 2013, 2020). Moreover, the few studies that incubated whole communities in situ have so far not accounted for the complexity of interactions between biogeochemical processes (Yates and Halley, 2003; Kline et al., 2012; van Heuven et al., 2018; Roth et al., 2020, 2021).
Here, biogeochemical processes underlying key reef functions were quantified in situ across five different benthic assemblages found on the fringing reef of Curaçao, consisting of functional groups that currently characterise many degraded shallow reef habitats throughout the wider Caribbean. To this end, a custom-made tent was placed over substrates dominated by either (1) coral, (2) turf and macroalgae, (3) bioeroding sponges, (4) benthic cyanobacteria mats, or (5) sand. Chemical fluxes between water column and reef were then determined by monitoring nutrients, inorganic carbon chemistry, and oxygen. This was done both during the day and at night to estimate overall net metabolism of these communities. To account for the simultaneous convoluted influence that various processes have on measured variables, the change in their concentrations is related to the responsible metabolic processes by solving a model consisting of ordinary differential equations describing the contribution of each process to the measured chemical fluxes. With this approach, model parameters (i.e. rates of biogeochemical processes) are derived from concurrent changes in all measured variables, the aim being to provide accurate estimates of the rates of the biogeochemical processes that underlie functions of the newly configured shallow Caribbean reefs.
2.1 Study site
Reef incubations were carried out on the leeward side of Curaçao (Piscadera Bay; N, W) between 12 February and 22 March 2018 at depths ranging from 5 to 7 m. The water at the study site is characterised by episodes of high turbidity and is periodically eutrophied due to terrestrial runoff and ineffective waste-water treatment. Sediment plumes transporting high concentrations of nitrate, ammonium, and phosphate into the shore's fringing reef are commonly encountered after a period of heavy rainfall (den Haan et al., 2016). The shallow reef flat near the entrance of the bay in which we conducted our incubations is characterised by rubble and patchy distribution of small coral heads, making this location particularly suitable for the deployment of tent incubations.
2.2 Tent incubations
The incubation enclosure consists of a custom made, tetrahedron-shaped “tent” (Fig. 1). It has transparent, vinyl and butanyl walls with rigid pole edges of 1 m in length, resembling the cBIT described by Haas et al. (2013). It also includes 0.5 m long flaps extending outward from each of the tent's three sides, allowing for the better sealing of the tent to the substrate by placing weights (metal chains) on these flaps. The enclosure covers a 0.43 m2 planar surface and encloses a 118 L volume. All three sides of the tent contained an opening to allow flushing of the enclosed volume between incubations: during incubations these openings were sealed by zippers. Water enclosed in the incubation tent was homogenised during the experiment by means of a continuously running brushless submergible water pump (BLDC Pump Co., Ltd.). This pump was attached to one of the tent poles, at half the height of the tent, generating a vertical circulating turbulence while minimising the upsurge of sediment. Effectiveness of the stirring was demonstrated by rapid and even dispersal of a small dose of injected fluorescein prior to the incubation. Surge movement was retained due to the non-rigid texture of the tent walls. Incubated communities included five different types of substrate dominated by either turf and macroalgae, sand, bioeroding sponges, benthic cyanobacteria mats, or coral (Fig. 2), equalling a total of 15 studied communities (three of each type). Each community was incubated during the day (n=15), and due to practical reasons, only two of each type were incubated during the night (n=10) (i.e. for each type of community, three daytime and two night-time incubations were carried out).
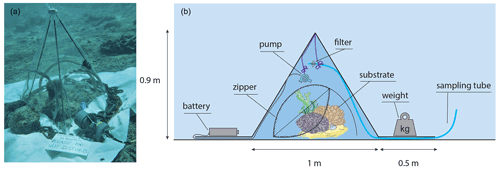
Figure 1The tent incubation set-up used. (a) Photograph depicting the tent incubation during the experiments. (b) Schematic cross section of the employed set-up for enclosing a small patch of reef. A battery powered mixing propeller for maintaining water circulation and analysers for salinity (S), temperature (T), oxygen (O2), and light (PAR) are located inside the tent. Outside the enclosure other S, T, and PAR analysers were placed, as well as the battery for the pump. Sampling of exterior and interior water (though sampling tube) was performed by divers using large volume syringes. Zippers allow for opening of tent windows for re-equilibrating the interior to the exterior conditions between incubations.
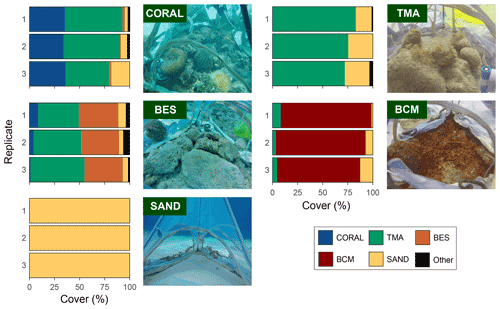
Figure 2Benthic cover of the 15 incubated reef communities dominated either by coral, turf and macroalgae (TMA), bioeroding sponges (BESs), cyanobacterial mats (BCMs), or sand with exemplary photograph.
The incubations were carried out one at a time over the study period and lasted 4 h each. Prior to each incubation, the tent was placed with flaps open over the substrate and left for a minimum of 3 h before the incubation was started. When day incubations were terminated, the tent was left in place with flaps open so that night incubation could be carried out on the same substrate. Daytime incubations were started at 10:00 LT, and night-time incubations started at 18:30 LT.
2.3 Substrate compositions
Substrates dominated by either coral, turf and macroalgae (TMA), bioeroding sponges (BESs), cyanobacteria mats (BCMs), or sand were incubated (Fig. 2). Three reef patches of each reef assemblage type were chosen depending on their cover of the dominant benthic component (see Table S1 in the Supplement for detailed species composition and cover). In some cases, to fit adequate incubation location and the tent capacity, pieces of rubble infested with sponge or covered in turf were added or retrieved from the community. In these cases, the community was left to stabilise 2 or 3 d before starting incubations. Incubated substrate included colonised hard substrate surrounded by bare hard substrate covered in a fine layer of sand for better enclosure deployment (except for sand incubations). The incubated coral species are characteristic of degraded Caribbean reefs and include some of the most prominent tolerant and opportunistic species found on modern reefs (Darling et al., 2012; de Bakker et al., 2016; Cramer et al., 2021) (see Table S1). Turf here refers to the epilithic algal matrix, defined by Clements et al. (2016) as “a conglomeration of short, turf-forming filamentous algae (<1 cm high), macroalgal spores, microalgae, sediment, detritus and associated fauna”. The benthic cyanobacterial mats in all three tent replicates were thick brown/reddish in colour and in line with the description in Brocke et al. (2018) for mats found between 3 and 7 m dominated by the species Oscillatoria bonnemaisonii. Percentage cover was measured in situ after removal of the tent. For substrates dominated by coral, its cover ranged from 34 % to 36 %. Turf and macroalgae cover ranged between 72 % to 83 %, bioeroding sponge cover varied from 38 % to 40 %, and cyanobacterial mat cover ranged from 83 % to 91 % in their respective incubations (Fig. 2).
2.4 In situ measurements
Measurements of salinity (S), temperature (T), dissolved oxygen (O2), and photosynthetically active radiation (PAR) within the tent were recorded at 1 min intervals throughout the duration of the incubations. S and T were measured using a Star-Oddi DST CTD, O2 was recorded using a HOBO U26 dissolved oxygen sensor and data logger, and PAR was assessed by an Odyssey light logger (Dataflow Systems Pty Ltd., Christchurch, NZ), calibrated in air against a Walz instrument (Walz ULM-500, Walz GmbH, Effeltrich, Germany). In addition, S, T, and PAR were measured for the duration of the incubations outside the tent using the same sampling frequency. All instruments within the tent were attached to the ridges except the Odyssey logger which was placed on the substrate facing upwards (covering approximately 150 cm2 of the substrate).
2.5 Discrete sampling
During each incubation, discrete samples were collected both inside and outside the tent at T0, after 2 h (T2), and after 4 h (T4) by scuba diving for the analyses of total alkalinity (AT), dissolved inorganic carbon (DIC), and nutrients (NO2+NO3, NO2 and NH4). The pH was calculated from the former two parameters using the package seacarb in the R software environment (Gattuso et al., 2018; R Core Team, 2020). Sampling of the tent interior was carried out from the outside by drawing seawater through 150 mL plastic syringes connected to a 1.5 m gas-impermeable tube (Tygon; Fig. 1). Syringes were flushed three times with the sampling water before collecting an actual sample. The tubing was fixed around a rigid pole of the tent in such way that the seawater was sampled from the centre of the tent incubation. The tube end located inside the tent was equipped with a Whatman® filter (G/F 0.47 µm) which was replaced daily to avoid the collection of particulate matter.
Analyses for AT were performed within 2 h upon sampling using spectrophotometrically guided single-step acid titration (Liu et al., 2015), and samples for DIC were run on an autoanalyser Traacs 800 spectrophotometric system (Stoll et al., 2001) at the NIOZ (Royal Netherlands Institute for Sea Research). Accuracy of both instruments was set using certified reference material supplied by Scripps Institute of Oceanography (Dickson et al., 2007). Precision of replicates was 2.7 µmol kg−1 for DIC and 0.9 µmol kg−1 for AT. Samples for dissolved inorganic macronutrients were prepared by dispensing sampled water through 0.80.2 µm Acrodisc filters into 5 mL pony vials and were subsequently stored at −20 ∘C until analysis at the NIOZ on a QuAAtro continuous flow analyser (SEAL Analytical, GmbH, Norderstedt, Germany) following the GO-SHIP protocol (Hydes et al., 2010).
2.6 Rates of water exchange
After sampling water at T0 for AT, DIC, and nutrients in each incubation, 450 mL of salt-saturated water was injected into the tent. The rate at which the elevated interior salinity equilibrated with ambient salinity during the incubation was used to estimate the rate of water exchange with the surrounding seawater for each incubation.
The rate of change in salinity within the incubation can be solved by the differential equation below:
where is the rate at which salinity changes within the tent, Sout is the exterior salinity, S is the interior salinity, and K is the water exchange rate.
The equation is solved using the function “ode”, within the package deSolve (Soetaert et al., 2010), which is the R routine that solves the differential equations. The function “modfit” from the package FME (Soetaert and Petzoldt, 2010) was used to perform iterative minimisation (based on least squares) on residuals to find the best fit within lower and upper bounds.
2.7 Inverse modelling and model–data comparison
The use of inverse modelling is advantageous as it enables us to derive unknown parameters (here rates of biogeochemical processes) simultaneously from all measured data. The mathematical “state” of the incubation's dynamic system can be described based on the mass balance between AT, DIC, O2, NH4, and NO3 which is influenced by various biogeochemical processes. The rate of these processes are the unknown parameters that need to be quantified by fitting against an incomplete data set (only three time points for AT, DIC, NH4, and NO3).
The model consists of the five differential equations depicted below that relate the change in measured variable concentrations over time to the responsible processes, which are here assumed to have remained constant over time.
Since the involved processes affect the different chemical components simultaneously, the combination of these differential equations can be used to solve the contribution (in terms of rates) of the processes to the observed changes. The processes in question include aerobic mineralisation (O2 consumption related to mineralisation), primary production (PP), calcification, dissolution, nitrification, and denitrification.
Mineralisation describes the degradation of an organic compound to its mineral components, i.e. carbon dioxide and inorganic nutrients. PP is the primary production, and calcification is the deposition of calcium carbonate. Pnh4 is the part of N uptake as NH4 for primary production. Dissolution results in an increase in calcium and carbonate ions by degradation of calcium carbonate shells and/or skeletons, and K is the water exchange rate. Nitrification is the process by which ammonium () is converted into nitrate (); 2 mol of oxygen are needed to oxidise 1 mol of ammonium during nitrification. The fraction of mineralisation that respires nitrate (i.e. denitrification) is pDeni. The OCratio is the ratio between the concentrations of oxygen and DIC. The NCratio is the ratio between N and DIC. The 0.8 constant refers to the denitrification redox reaction (Soetaert et al., 2007).
We start by determining the parameters that can be fitted, based on parameter collinearity. After producing a best fit set of the selected parameters, we quantify parameter uncertainty and produce sensitivity ranges around the modelled variables.
The OCratio, NCratio, and K parameters are always fixed and estimated from data prior to running the model. Others vary between fixed and free (to be fitted) depending on collinearity and light. For instance, primary production is fixed at 0 during night incubation; however, during the day, only the dominant process can be estimated. Some parameters are highly correlated with each other such as primary production and remineralisation or calcification and dissolution and therefore cannot be estimated simultaneously. In general, when the collinearity index exceeds 20, the linear dependence is assumed to be critical (i.e. it will be impossible or difficult to estimate all the parameters in the combination together).
Collinearity of the parameter sets is measured using the function “collin” within the FME package (Soetaert and Petzold, 2010).
The model equations are specified in a function that calculates the rate of change in the state variables (dDIC, dNH4, etc). Inputs to the function are the model time (t), the values of the state variables (DIC, NH4, NO3, AT, and O2), and the parameters (remineralisation, calcification, etc.). The differential equation model is solved using the function “ode”, within the package deSolve, which is the R routine that solves the differential equations.
The discrepancy of the model solution with observed changes within the tents is calculated using the function “modCost”, still in the FME package, which estimates the residuals and the variable and model costs (sum of squared residuals).
The function “modfit” was then used to perform iterative minimisation (based on least squares) on residuals to find the parameter giving the best fit within lower and upper bounds. Estimated parameters are the unknown fluxes (mineralisation, PP, calcification, etc.).
2.8 Conversion to fluxes
The best-fit parameters, i.e. the input rates R (in ), in the tent are converted to fluxes from the water–substrate interface (), assuming an enclosed mass of water of 108±10 kg (tent encloses approximately 118 L of volume, of which substrate volume is ∼10 L and seawater density ∼1022 kg m−3) and an incubated planar surface of 0.43 m2.
Net community calcification (NCC) fluxes were determined from the predicted calcification and dissolution. The model captures the dominant net flux and does not distinguish the relative contributions of gross calcification and dissolution to the integrated NCC rate. Net community production (NCP) is the difference between remineralisation and primary production. Denitrification is estimated from the pDeni fraction and the mineralisation parameters.
2.9 Comparing biogeochemical signatures between incubations
To evaluate if water exchange rate had an impact on estimated processes, the non-parametric Kendall rank correlation test was performed. All inferred biogeochemical process rates (mineralisation, primary production, NCP, NCC, nitrification, and denitrification) were tested against incubation water exchange rates.
Principal component analysis (PCA) was used to identify groupings among the 23 tent incubations (day: n=13; night: n=10) in relation to their biogeochemical signature (i.e. NCC, NCP, nitrification, and denitrification). The oxygen logger malfunctioned during two of the day incubations consisting of one BCM- and one TMA-dominated community incubations. The model was therefore not run through these tents. PCA was conducted on a centred multivariate data set consisting of the four main biogeochemical processes (i.e. NCC, NCP, nitrification, and denitrification). Additionally, NCC was plotted against NCP to evaluate how the balance between both processes varied among distinct communities and by which process communities were dominated.
3.1 Ambient conditions
In-tent light and temperature were only slightly impacted by the tent enclosure compared to the exterior (Fig. 3). Light was on average 17 % lower inside the tent, and changes in temperature were dampened within the tent. In-tent temperatures were on average 0.2 ∘C higher than those outside the tent. Average ambient AT, DIC, pH, NH4, and NO3 were 2386.8±13.9 µmol kg−1, 2125.5±20.0 µmol kg−1, 7.9±0.003, 0.31±0.15 µmol kg−1, and 0.32±0.14 µmol kg−1, respectively. Measured data for each incubation and inside and outside the tent for all three time points, as well as the differences between T0 and T4, are presented in Table S2 in the Supplement.
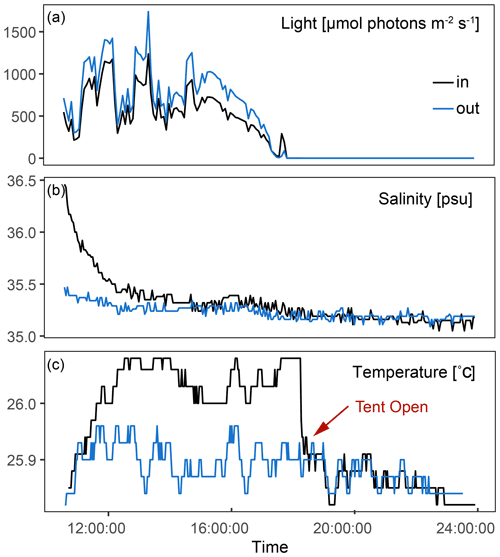
Figure 3Exterior and interior measurements for three representative incubations performed throughout this study. (a) Difference in light between the inside of the tent and the ambient environment during an incubation. (b) Injection of salt within the tent at the start of the incubation and its gradual return to ambient salinity. (c) Temperature within the tent compared to exterior conditions; when the tent is opened at the end of the incubation, temperature immediately returns to ambient conditions.
3.2 Water exchange quantification
Application of Eq. (1) to salinity data collected during all incubations yielded dilution rate K ranging between 0.004 and 0.044 min−1. This indicates that 2.1 to 4.8 kg of seawater (i.e. K×108 kg) was exchanged every minute between the incubation enclosure and the environment. These rates correspond to the intensity of the water movement observed and were recorded visually at the time of each incubation. Figure 4 shows the data used to estimate the rate of water exchange of an incubation with relatively minor leakage (A) and one in which leakage is more severe (B). In these examples, in-tent salinity returns to ambient concentrations after ∼1 and ∼2 h, respectively.
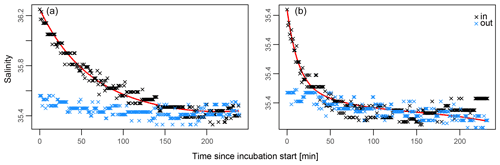
Figure 4(a, b) Best fit explaining in-tent salinity at any given time (Eq. 1, red line). In-tent salinity measurements are in black, and ambient salinity measurements are in blue. Panel (a) illustrates an incubation that leaked relatively slowly with K=0.0192 ( L min), while (b) depicts a more rapidly leaking tent with K=0.044 ( L min).
3.3 Model output
Figure 5 illustrates the output of our approach for all incubations. Using a minimisation routine, best fit parameters (mineralisation, PP, calcification, etc.) were predicted to best fit the model to the measured data. Individual graphic outputs for two incubations (including one carried out during the day on substrate dominated by BESs and one performed at night-time on BCM-dominated substrate) with respective fixed and fitted parameters are presented in Fig. S1 in the Supplement. Details for parameter prediction and best fit can be found in Table S3 in the Supplement. The model output shows a relatively good fit to the measured observations (Fig. 5), indicating that the interactions between processes and their effects on chemical fluxes were considered correctly. Overall fit is usually better on night data, which is mostly due to the inability of the model to predict irregular oxygen evolution caused by light variability during the daytime (Table S3).
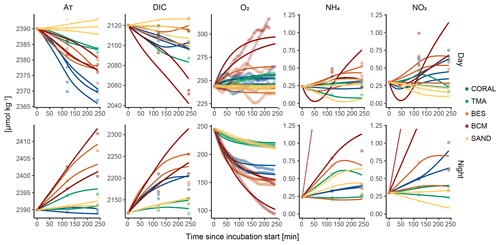
Figure 5Illustrative results of the model (coloured lines) employed to infer the process rates from measured data. The top graphs depict the model output of incubations performed during the day, while the bottom ones show the output for night-time incubations. Points represent measured values of the state variables inside and outside the tent. The measured data were centred for graphic visualisation purposes (non-centred data for all incubations can be found in Table S2). The blue, green, orange, red, and yellow colours represent communities dominated by coral, turf and macroalgae, bioeroding sponges, benthic cyanobacterial mats, and sand, respectively. Note that NH4 and NO3 measurements in BCM incubations at night-time were much higher than the rest. The y axes of the graphs depicting NH4 and NO3 results were therefore truncated in order to better visualise model output of all other incubations (model output for the off-chart BCM incubation can be found in Fig. S1).
As the process estimates are limited to net increase or decrease, fluxes for PP and mineralisation are presented as net community production (NCP), and calcification and dissolution are combined into net community calcification (NCC; Fig. 6).
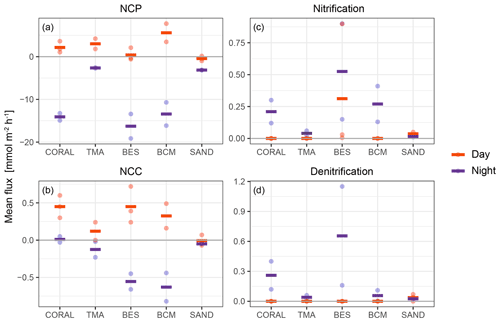
Figure 6Process rates for all tent replicates (points) and respective averages (line) estimated from observed concentration changes and model output in the tent enclosure on each substrate type. Processes occurring during the day are depicted in red, and processes at night are represented in purple.
3.4 Estimated biogeochemical processes
NCP showed a clear diurnal pattern (Fig. 6). While all NCP values were modestly skewed towards net autotrophy during the day (except for sand), the strongest signal was found for substrates dominated by BCM with an average daily NCP of 5.6 . Night values indicated net respiration ranging from an average of −2.64 on substrates dominated by TMA to −16.28 on substrates dominated by bioeroding sponges.
A clear diurnal signal also resided in NCC fluxes for all substrates involved (Fig. 6). Most NCC fluxes recorded during daytime (except for sand incubations) indicated net CaCO3 precipitation. At night, most NCC fluxes indicated net CaCO3 dissolution, especially on substrates dominated by BESs and BCMs. The absence of change in AT for coral-dominated substrates during the night indicated that dissolution equalled calcification during these incubations, and hence, the average NCC at night was close to 0. Substrates dominated by coral generated the strongest decrease in AT (net precipitation) during daytime, yielding an average NCC rates of 0.45 . Highest net dissolution was found at night-time for incubations of substrates dominated by bioeroding sponges, and cyanobacterial mats, with a comparable average of 0.56 and 0.63 , respectively.
Nitrification was found to occur predominantly at night with higher fluxes in incubations of substrates dominated by bioeroding sponges, cyanobacterial mats, and corals. Denitrification also occurred mostly at night except on sand where daytime and night-time fluxes were small but relatively similar (Fig. 6).
The Kendall rank correlation test did not reveal a significant correlation between water exchange rates and rates of mineralisation (p=0.79, τ=0.04), primary production (p=0.47, τ=0.12), NCP (p=0.75, τ=0.05), NCC (p=0.17, τ=0.21), nitrification (p=0.81, τ=0.04), and denitrification (p=0.27, τ=0.18). The Kendall correlation coefficient τ is closer to zero than to one in all cases, implying there is no significant association between the two tested variables.
3.5 Incubation comparison
The PCA based on the four main biogeochemical processes revealed different groups for night incubations and day incubations (Fig. 7a) except for sand incubations in which night and day incubations grouped relatively close to each other. The first two principal component axes (PC1 and PC2) explained 88.68 % of the total variability within the data. PC1 described a gradient in NCP and NCC from high (negative PCA scores) to low (positive PCA scores) and an opposite pattern for nitrification and denitrification. PC2 further explains the variability in NCC and nitrification and to a lesser extent NCP and denitrification.
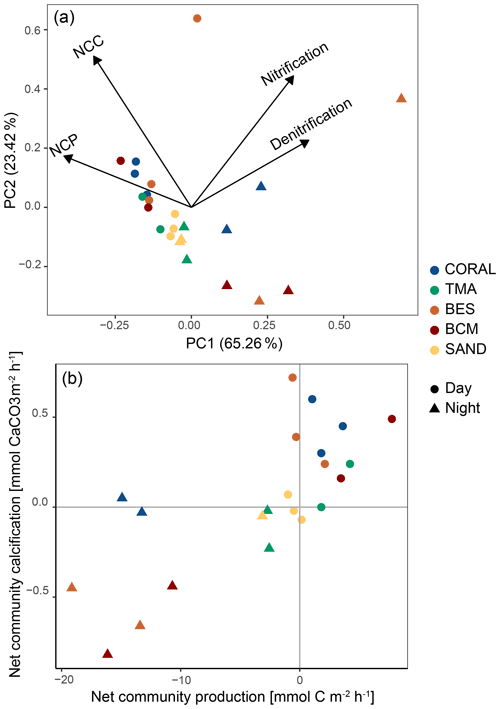
Figure 7(a) Principal component analysis (PCA) diagram displaying the spatial variation of all incubations along the first two principal components. Processes are plotted as vectors, and dots represent daytime incubations, while triangles depict night-time incubations. Colour refers to the type of substrate incubated. (b) NCP vs. NCC rates for every incubation. Each dot/triangle represents an individual incubation, and colour indicates community composition.
One of the communities dominated by bioeroding sponges (replicate 1) is separate from other communities both during the day and during the night due to considerably higher rates of nitrification and denitrification compared to other communities.
Figure 7b showed the position of the 15 communities in the different quadrants of the NCC vs. NCP diagram. A clear separation is observed between day incubations, characterised by net photosynthesis and calcification, and night incubations, indicating net respiration and dissolution. Daytime sand incubations are centred due to the low magnitude of the processes occurring on these substrates, and they are the only incubations exhibiting net dissolution during the daytime. For night-time incubations, only coral-dominated communities displayed modest net calcification.
The biogeochemical flux assessment has enabled us to identify and quantify the biological functions that are currently at play on this degraded Curaçaoan reef flat. Although the present study only investigated the shallow part of one single reef, it provides insight into the effects that shifts in coral species and functional groups have had on the overall functioning of these communities. Comparison with coral reefs in a broader biogeographical context is needed to establish whether the rates obtained here are site-specific or representative of degrading Caribbean coral reefs in general.
The shallow reef communities investigated at this site barely support reef functions that are usually ascribed to a healthy coral reef. Overall, net community calcification and production on these substrates were low compared to reef flats worldwide (Atkinson, 2011). Very low or negative NCC rates were recorded on all substrates, suggesting reduced net accretion potential. Net production was also low, likely indicating limited accumulation of biomass, while heterotrophic processes were prominent. Recycling processes (i.e. nitrification and denitrification) were high but did not prevent net nutrient release from aerobic mineralisation, rendering all substrates sources of nitrogen. Although processes recorded on substrates dominated by coral, bioeroding sponges and cyanobacterial mats showed some variation between types of substrates, the overall behaviour of complementary processes for each of these assemblages was relatively comparable in terms of direction and magnitude.
4.1 Net dissolving reef
Although net calcification was recorded during the day on all substrate types (except sand), it did not compensate for higher dissolution rates at night except on substrates dominated by coral. Diel shifts between net calcification and net dissolution are usual for coral reefs and have been recorded in healthier systems than the one studied here (Yates and Halley, 2003; Albright et al., 2013, 2015; Koweek et al., 2015), with instances of net dissolution mainly taking place at night, coinciding with net respiration (and most likely with low gross calcification) (Cyronak et al., 2018). However, the community calcification budget over 24 h resulting from this diurnal variability in the present study resulted in a modest average net calcification rate of 5.7 on coral-dominated substrates. This is low compared to rates reported for reef flats worldwide (with an average around 130 and ranging from 20 to 250 ; Atkinson, 2011). Overall, the limited number of in situ flux-based experiments carried out in the wider Caribbean (Yates and Halley 2003; Muehllehner et al., 2016; van Heuven et al., 2018) suggest they are among the lowest NCC rates recorded worldwide (Albright et al., 2015; Shaw et al., 2015; Silverman, 2007) and particularly low compared to those in the Indo-Pacific region (Koweek et al., 2015; Takeshita et al., 2016). Surveys using a census-based approach (Perry et al., 2013; de Bakker et al., 2019) also showed that some Caribbean reefs are net eroding. The recorded low rates of net carbonate production in the wider Caribbean may be expected simply due to the region-wide decrease in coral coverage since the 1970s. However, the decrease in net calcification relative to historical values is likely related to more than just coral cover loss. Indeed, the latter has subsequently left surfaces available for colonisation by turf, macroalgae (Hughes, 1994), and more recently cyanobacterial mats (de Bakker et al., 2017). Shallow reefs around Curaçao (<10 m) are covered by filamentous algal turf canopies that presently represent the most dominant benthic component on these reefs (Vermeij et al., 2010). Given their abundance and high release rates of dissolved organic carbon (Mueller et al., 2016), heterotrophic activity is likely to be stimulated. Furthermore, cyanobacterial mats release part of their photosynthetically fixed carbon as dissolved organic carbon (DOC) into the water column at a higher rate than turf and macroalgae (Mueller et al., 2014; Brocke et al., 2015). They have been shown to be responsible for 79 % of the total DOC released over a 24 h diel cycle at this same study site (Brocke et al., 2015). Considering their proliferation around the islands of Curaçao and Bonaire since ∼2003 (de Bakker et al., 2017) and the prevalence of turf algae in the area, an accumulation of organic matter may have resulted in a reduction in pH due to oxidation of organic matter, i.e. stimulated heterotrophic activity, resulting in reduced calcification (Bates et al., 2010). Muehllehner et al. (2016) suggested that the seasonal character of reef dissolution they recorded on the Florida Reef Tract coincided with an accumulation of organic matter following the die-off of annual sea grasses in the area.
4.2 Net heterotrophic reef
Low net community production rates in the current study indicate that autotrophic processes dominate modestly during the day. Integrating the NCP values over 24 h (day and night) yielded rates skewed towards net respiration, indicating heterotrophy in all incubations. Although net community production of reef flats has been reported to vary notably over the course of the day (Koweek et al., 2015), with values ranging from −220 to +310 (Atkinson, 2011), large amplitude shifts between net autotrophy and net heterotrophy are usually recorded between day and night (Yates and Halley, 2003; Albright et al., 2013, 2015; Koweek et al., 2015). Here, the amplitude of this shift between day and night was modest. It should be noted, however, that the reduction in light intensity by 17 % on average may have resulted in a slight underestimation of NCP rates during the daytime. This would hold especially true for BCM incubations. Reductions in light would intensify down the steep vertical physiochemical gradients present in these microbial mats and could interfere with light-controlled circadian regulation of photosynthesis and respiration in these cooperative communities (Hörnlein et al., 2018), favouring respiration and decreasing net community productivity.
The reduction in the amplitude of the diel shift in net production and calcification recorded in the present study may have severe implications. For instance, metabolic fluctuations from reef biota cause strong temporal fluxes in compounds which affect the oscillatory behaviour of reef seawater microbial communities (Kelly et al., 2019; Weber et al., 2020), leading to less distinct populations and more redundancy in microbial specialists' functions, i.e. a shift to a dominance in catabolic pathways. Organic material supplied to the ecosystem by benthic primary producers as exudates is also thought to play a pivotal role in microbial growth (Haas et al., 2011) and diversity (Nelson et al., 2013; Haas et al., 2016) depending on its origin. Studies on the effect of exudates of macroalgae and turf on microbial metabolism demonstrated that the composition of exudates stimulated rapid growth of less diverse microbe communities compared to coral-derived exudates. Consequently, reef microbial communities shift towards copiotrophic populations that have the potential to remineralise available organic nutrients at a high rate and encode greater numbers of potential virulence factor genes, ultimately harming corals and maintaining algal dominance (Nelson et al., 2013; Dinsdale and Rohwer, 2011). We infer that the amount and type of organic matter provided by abundant algal turf mats on this reef likely enhance heterotroph activity and stimulate the proliferation of less diverse copiotrophic microbial populations, rendering the studied reef net heterotrophic regardless of substrate type.
4.3 Nitrogen cycling
Nitrogen pathways support high primary productivity in oligotrophic environments by supplying nutrients while simultaneously preventing the build-up of excess nutrients that may favour opportunistic primary producers such as algal turfs (e.g. O'Neil and Capone, 2008; Karcher et al., 2020). The abundance of non-coral primary producers on these reefs suggest that nitrogen is not a limiting factor for growth. Results showed that all substrate types acted as and sources during the day and the night, apart from sand and turf substrates which acted as sinks for . This is to be expected from overall net heterotrophic communities; however, even in instances of net autotrophy during the day, substrates still acted as DIN sources. This is comparable to recent results from in situ incubations carried out in the central Red Sea on net autotrophic coral and algae-dominated communities (Roth et al., 2020, Roth et al., 2021). It is likely that other community-wide processes, such as the consumption and transformation of organic matter by microbial populations (e.g. Pfister and Altabet, 2019), masked the assimilation of DIN by primary producers.
Nitrification and denitrification rates measured in the present study generally fall within the published range of in situ measurements in tidal pools dominated by algae and corals (Webb and Wieber, 1975), in cavities covered in encrusting sponges (Scheffers et al., 2004), on cyanobacterial mats (Bonin and Michotey, 2006), and on carbonate sand (Capone et al., 1992; Eyre et al., 2013b). However, there was no nitrification during the day (except for one community dominated by sponges), which may be explained by light causing a reduced activity of nitrifiers (Kwon et al., 2020). Owing to the rather shallow depths of our experiment, nitrifiers may have been negatively affected by the light. As mentioned above, microbial communities are impacted by organic matter composition and temporal fluctuations in biochemicals. Shifts in diversity and abundance of the microbial communities inhabiting the reef substrate may also lead to diel shifts in nitrogen-cycling capacity (Rädecker et al., 2015). Further research investigating how alterations in diversity and abundance of these microbial functional groups relate to changes in the nitrogen-cycling capacity of reef assemblages is needed at this point.
4.4 Similar biogeochemical processes by reef communities dominated by corals, sponges, and cyanobacteria
Although processes recorded on distinct community assemblages showed some variation between substrate types, present results suggest that the various communities of this degraded reef of Curaçao exhibit similar directions and magnitudes of key net biogeochemical processes. Results indicate that even on substrates with coral cover ranging from 34 % to 36 %, which is high for Curaçaoan reefs and relative to the wider Caribbean region (Jackson et al., 2014; de Bakker et al., 2016, 2019), net community calcification is very low. In fact, daily rates are in a similar range to those for substrates dominated by bioeroding sponges where coral cover ranged from 1 % to 9 % and to substrates covered by cyanobacterial mats where no live coral was recorded (coral: 0.45; BES: 0.45; and BCM: 0.33 ). Recent work by Romanó de Orte et al. (2021) found comparable results showing similar daytime calcification rates for live coral and dead coral substrate. Although hard coral is generally assumed to dominate the calcification signal on tropical reefs, these results suggest that coral might not be the sole key player in coral reef calcification dynamics on such impacted sites. Cementation/lithification processes carried out by coralline calcifying algae, micro-calcifiers (e.g. foraminifera and juvenile shells), and benthic microbial communities, resulting in the trapping and binding of rubble and sediment in cryptic habitats and within/on the rubble, may play a comparably important role, counteracting some of the dissolution occurring in these communities.
The main differences between coral-dominated substrates and others, in terms of NCC, are that they were the only substrate able to balance out night-time dissolution. Primary production is barely compensating for heterotrophic processes during the day on all substrates. Although substrates incubated in the present study are distinct in taxon dominance, they do share some similarities that may be drawing biogeochemical process differences closer to each other regardless of substrate type. For instance, turf covers any part of hard substrate available, the sand and rubble potentially harbour a variety of comparable cryptic organisms, and the microbial community within and above each of these substrates may be shifting similarly towards generalist copiotrophic populations.
Shifts in community composition have resulted in the impairment of key reef processes, and the present results may suggest that some degree of functional homogenisation (Clavel et al., 2011) exists among substrates with different community assemblages. It is noteworthy that seasonality may explain biogeochemical process similarity between major biogeochemical processes on reefs dominated by distinct functional groups. Roth et al. (2020) recently found that summer temperatures amplified functional differences between coral- and algae-dominated communities in the central Red Sea. Higher temperatures benefit algae-dominated communities in terms of primary production and growth, while coral-dominated communities shifted towards a more heterotrophic state with depressed net community calcification rates. The fact that coral-dominated substrates studied here are already in a heterotrophic state with very low NCC values in winter temperatures attests to the differences in the studied systems and provides an opportunity for comparison between a relatively healthy system and a degraded one (Roff and Mumby, 2012). Additionally, average ambient pH at the current study site was 7.9, which is lower than average “summer” pH, usually between 8.1 and 8.2 (den Haan et al., 2016). This may suggest that depressed calcification rates in the Piscadera Bay are indeed linked to seasonality. However, further research and additional incubations are needed to better understand the effect of seasonal variation on the functional states of these degraded reefs.
4.5 Method considerations
The combination of in situ incubations and inverse modelling, which incorporates the complexity of interactions between processes, has proven to be an effective tool to provide quantitative data on the functional state of coral reef patches. Quantification of the exchange of substances between reef communities and the overlaying water was achieved despite the presence of swell-induced seawater exchange because this approach allowed for a volume exchange between the environment and the incubation. The incubations can thereby be replenished to some extent, keeping saturated O2 levels within the tent and thus minimising unrepresentative reef community metabolism. Fluxes within the incubations can be treated as if acquired by an in situ flow through system.
For the interpretation of the measured concentration differences, the multifaceted influence of metabolic processes on chemical fluxes was accounted for. The model shows a good fit of the observations around the fitted curve, indicating that the interactions between processes and their effects on chemical fluxes were considered correctly.
Nonetheless, due the limited number of incubations that were carried out for this study, we interpret results with caution. Additionally, incubations were only deployed on the reef flat of one degraded reef site, and the future application of this or similar incubation methods should consider multiple sites. Lastly, methods would be further improved by continuous monitoring of the exchange rate rather than assuming it to be constant throughout the incubation.
Results acquired on this shallow Curaçaoan reef provide insight into the impact of habitat degradation and benthic composition shifts on reef functions. Currently prevalent corals, although more resilient, calcify at a slower pace than previously abundant species (such as Acropora spp.) and cannot balance out heterotrophic processes from other functional groups. Coral presence does, however, contribute to counteracting dissolution processes at night, therefore acting as a buffer, albeit marginal, to reef deconstruction. In the context of ongoing global change, the environmental resilience of generalist species could be a determining factor of ecosystem stability (Clavel et al., 2011). For instance, on some reef terraces of the fringing reefs of Curaçao and Bonaire (southern Caribbean), certain stretches appear to harbour a considerable cover of steadily growing little boulder-constructing tolerant corals (including mainly (Pseudo-)Diploria spp., Porites astreoides, and Siderastrea sidereal) (de Bakker et al., 2019). These are often found near areas which have locally suffered chronic stress from terrestrial sources (i.e. inflow, intense coastal development, factory outflow) but are often limited to areas providing hard substrate and relatively little sand. Data on the processes underlying such developments, however, are virtually absent, but this may indicate that even the most severely degraded reefs could slowly regain essential functions when a critical adaptive capacity is reached.
While these sites may provide a spark of hope with regards to recovery potential, most of the reefs in the Caribbean presently reside in ecological states that closely resemble the reef site studied here (or are expected to do so in the near future). Ultimately, Caribbean reefs will benefit most notably from adequate mitigation strategies to give these systems a chance to adapt and restore key functions in the face of exacerbating environmental conditions.
Data and R code will be made available on request.
The supplement related to this article is available online at: https://doi.org/10.5194/bg-18-6501-2021-supplement.
AEW, DMdB, SMACvH, and LJdN conceived the ideas and designed methodology. AEW, DMdB, and TdC collected the data. AEW and KS analysed the data. AEW and LJdN led the writing of the manuscript in consultation with DMdB, SMACvH, FCvD, KS, and GR. All authors contributed critically to the drafts and gave final approval for publication.
The contact author has declared that neither they nor their co-authors have any competing interests.
Publisher's note: Copernicus Publications remains neutral with regard to jurisdictional claims in published maps and institutional affiliations.
The authors are particularly grateful to the Carmabi research station as a whole and especially Mark Vermeij for his support and facilitating the fieldwork on Curaçao. We would also like to thank Jasper de Goeij for lending us equipment. We extent our sincere gratitude to Karel Baker and Sharyn Ossebaar for analysing nutrient and DIC samples.
This paper was edited by Tyler Cyronak and reviewed by Jennifer Mallon and Kennedy Wolfe.
Atkinson, M. J.: Biogeochemistry of nutrients. In Coral reefs: An ecosystem in transition, 199–206, Springer, Dordrecht, 2011.
Albright, R., Langdon, C., and Anthony, K. R. N.: Dynamics of seawater carbonate chemistry, production, and calcification of a coral reef flat, central Great Barrier Reef, Biogeosciences, 10, 6747–6758, https://doi.org/10.5194/bg-10-6747-2013, 2013.
Albright, R., Benthuysen, J., Cantin, N., Caldeira, K., and Anthony, K.: Coral reef metabolism and carbon chemistry dynamics of a coral reef flat, Geophys. Res. Lett, 42, 3980–3988, https://doi.org/10.1002/2015GL063488, 2015.
Albright, R., Caldeira, L., Hosfelt, J., Kwiatkowski, L., Maclaren, J. K., Mason, B. M., Nebuchina, Y., Ninokawa, A., Pongratz, J., Ricke, K. L., and Rivlin, T.: Reversal of ocean acidification enhances net coral reef calcification, Nature, 531, 362–365, 2016.
Albright, R., Takeshita, Y., Koweek, D. A., Ninokawa, A., Wolfe, K., Rivlin, T., Nebuchina, Y., Young, J., and Caldeira, K.: Carbon dioxide addition to coral reef waters suppresses net community calcification, Nature, 555, 516–519, 2018.
Alvarez-Filip, L., Dulvy, N. K., Gill, J. A., Côté, I. M., and Watkinson, A. R.: Flattening of Caribbean coral reefs: Region-wide declines in architectural complexity, P. R. Soc. B., 276, 3019–3025, https://doi.org/10.1098/rspb.2009.0339, 2009.
Andersson, A. J. and Gledhill, D.: Ocean Acidification and Coral Reefs: Effects on breakdown, dissolution, and net ecosystem calcification, Annu. Rev. Mar. Sci., 5, 321–348, https://doi.org/10.1146/annurev-marine-121211-172241, 2013.
Aronson, R. B., Macintyre, I. G., Lewis, S. A., and Hilbun, N. L.: Emergent zonation and geographic convergence of coral reefs, Ecology, 86, 2586–2600, 2005.
Bates, N. R., Amat, A., and Andersson, A. J.: Feedbacks and responses of coral calcification on the Bermuda reef system to seasonal changes in biological processes and ocean acidification, Biogeosciences, 7, 2509–2530, https://doi.org/10.5194/bg-7-2509-2010, 2010.
Barott, K. L., Williams, G. J., Vermeij, M. J., Harris, J., Smith, J. E., Rohwer, F. L., and Sandin, S. A.: Natural history of coral–algae competition across a gradient of human activity in the Line Islands, Mar. Ecol. Prog. Ser., 460, 1–12, 2012.
Bonin, P. C. and Michotey, V. D.: Nitrogen budget in a microbial mat in the Camargue (southern France), Mar. Ecol. Prog. Ser., 322, 75–84, 2006.
Bellwood, D. R., Hoey, A. S., and Choat, J. H.: Limited functional redundancy in high diversity systems: resilience and ecosystem function on coral reefs, Ecol. Lett., 6, 281–285, 2003.
Bellwood, D. R., Streit, R. P., Brandl, S. J., and Tebbett, S. B.: The meaning of the term “function” in ecology: a coral reef perspective, edited by: Wolfe, K., Anthony, K., and Babcock, R., Funct. Ecol., 33, 948–961, 2019.
Brandl, S. J., Rasher, D. B., Côté, I. M., Casey, J. M., Darling, E. S., Lefcheck, J. S., and Duffy, J. E.: Coral reef ecosystem functioning: eight core processes and the role of biodiversity, Front. Ecol. Environ., 17, 445–454, 2019.
Brocke, H. J., Wenzhoefer, F., de Beer, D., Mueller, B., van Duyl, F. C., and Nugues, M. M.: High dissolved organic carbon release by benthic cyanobacterial mats in a Caribbean reef ecosystem, Sci. Rep., 5, 8852, https://doi.org/10.1038/srep08852, 2015.
Brocke, H. J., Piltz, B., Herz, N., Abed, R. M., Palinska, K. A., John, U., den Haan, J., de Beer, D., and Nugues, M. M.: Nitrogen fixation and diversity of benthic cyanobacterial mats on coral reefs in Curaçao, Coral Reefs, 37, 861–874, 2018.
Burman, S. G., Aronson, R. B., and van Woesik, R.: Biotic homogenization of coral assemblages along the Florida reef tract, Mar. Ecol. Prog. Ser., 467, 89–96, 2012.
Capone, D. G., Dunham, S. E., Horrigan, S. G., and Duguay, L. E.: Microbial nitrogen transformations in unconsolidated coral reef sediments, Mar. Ecol. Prog. Ser., 18, 75–88, 1992.
Chen, P. Y., Chen, C. C., Chu, L. F., and McCarl, B.: Evaluating the economic damage of climate change on global coral reefs, Global Environ. Chang., 30, 12–20, https://doi.org/10.1016/j.gloenvcha.2014.10.011, 2015.
Clavel, J., Julliard, R., and Devictor, V.: Worldwide decline of specialist species: toward a global functional homogenization?, Front. Ecol. Environ., 9, 222–228, 2011.
Clements, K. D., German, D. P., Piché, J., Tribollet, A., and Choat, J. H.: Integrating ecological roles and trophic diversification on coral reefs: multiple lines of evidence identify parrotfishes as microphages, Biol. J. Linn. Soc., 120, 729–751, 2016.
Courtney, T. A., Andersson, A. J., Bates, N. R., Collins, A., Cyronak, T., de Putron, S. J., Eyre, B. D., Garley, R., Hochberg, E. J., Johnson, R., and Musielewicz, S.: Comparing chemistry and census-based estimates of net ecosystem calcification on a rim reef in Bermuda, Front. Mar. Sci., 3, 181, https://doi.org/10.3389/fmars.2016.00181, 2016.
Cramer, K., Donovan, M., Jackson, J., Greenstein, B., Korpanty, C., Cook, G., and Pandolfi, J.: The transformation of Caribbean coral communities since humans, Authorea Preprints, https://doi.org/10.22541/au.161048962.21724991/v1, 2021.
Cyronak, T., Andersson, A. J., Langdon, C., Albright, R., Bates, R., Caldeira, K., Carlton, R., Corredor, J. E., Dunbar, R. B., Enochs, I., Erez, J., Eyre, B. D., Gattuso, J., Lantz, C., Lazar, B., Manzello, D., Mcmahon, A., Mele, M., Page, H. N., Santos, I. R., Schulz, K. G., Shaw, E., and Silverman, J.: Taking the metabolic pulse of the world's coral reefs, PloS one, 13, 1–17, 2018.
Darling, E. S., Alvarez-Filip, L., Oliver, T. A., McClanahan, T. R., and Côté, I. M.: Evaluating life-history strategies of reef corals from species traits, Ecol. Lett., 15, 1378–1386, 2012.
De'ath, G., Fabricius, K. E., Sweatman, H., and Puotinen, M.: The 27 year decline of coral cover on the Great Barrier Reef and its causes, P. Natl. Acad. Sci. USA, ., 109, 17995–17999, https://doi.org/10.1073/pnas.1208909109, 2012.
de Bakker, D. M., Meesters, E. H., Bak, R. P. M., Nieuwland, G., and Van Duyl, F. C.: Long-term Shifts in Coral Communities On Shallow to Deep Reef Slopes of Curaçao and Bonaire: Are There Any Winners?, Front. Mar. Sci., 3, 1–14, https://doi.org/10.3389/fmars.2016.00247, 2016.
de Bakker, D. M., van Duyl, F. C., Bak, R. P. M., Nugues, M. M., Nieuwland, G., and Meesters, E. H.: 40 Years of benthic community change on the Caribbean reefs of Curaçao and Bonaire: the rise of slimy cyanobacterial mats, Coral Reefs, Springer, Berlin, Heidelberg, 36, 355–367, https://doi.org/10.1007/s00338-016-1534-9, 2017.
de Bakker, D. M., Webb, A. E., van den Bogaart, L. A., van Heuven, S. M., Meesters, E. H., and van Duyl, F. C.: Quantification of chemical and mechanical bioerosion rates of six Caribbean excavating sponge species found on the coral reefs of Curaçao, PloS one, 13, e0197824, https://doi.org/10.1371/journal.pone.0197824, 2018.
de Bakker, D. M., van Duyl, F. C., Perry, C. T., and Meesters, E. H.: Extreme spatial heterogeneity in carbonate accretion potential on a Caribbean fringing reef linked to local human disturbance gradients, Glob. Change Biol., 25, 4092–4104, 2019.
den Haan, J., Huisman, J., Brocke, H. J., Goehlich, H., Latijnhouwers, K. R., Van Heeringen, S., Honcoop, S. A., Bleyenberg, T. E., Schouten, S., Cerli, C., and Hoitinga, L.: Nitrogen and phosphorus uptake rates of different species from a coral reef community after a nutrient pulse, Sci. Rep., 6, 1–13, 2016.
Dickson, A. G., Sabine, C. L., and Christian, J. R.: Guide to best practices for ocean CO2 measurements, North Pacific Marine Science Organization, PICES Special Publication 3, Sydney, Canada, 2007.
Dinsdale, E. A. and Rohwer, F.: Fish or germs? Microbial dynamics associated with changing trophic structures on coral reefs, in: Coral reefs: an ecosystem in transition, 231–240, Springer, Dordrecht, 2011.
Dove, S. G., Kline, D. I., Pantos, O., Angly, F. E., Tyson, G. W., and Hoegh-Guldberg, O.: Future reef decalcification under a business-as-usual CO2 emission scenario, PNAS, 110, 15342–15347, 2013.
Dove, S. G., Brown, K. T., Van Den Heuvel, A., Chai, A., and Hoegh-Guldberg, O.: Ocean warming and acidification uncouple calcification from calcifier biomass which accelerates coral reef decline, Communications Earth & Environment, 1, 1–9, 2020.
Eyre, B. D., Santos, I. R., and Maher, D. T.: Seasonal, daily and diel N2 effluxes in permeable carbonate sediments, Biogeosciences, 10, 2601–2615, 2013.
Gardner, T. A., Côté, I. M., Gill, J. A., Grant, A., and Watkinson, A. R.: Long-term Regional-wide declining in Caribbean corals, Science, 301, 958–960, https://doi.org/10.1126/science.1086050, 2003.
Gattuso, J.-P., Epitalon, J.-M. Lavigne, H., and Orr, J.: seacarb: seawater carbonate chemistry, R package version 3.3.0, https://doi.org/10.5281/zenedo.4600014, 2018.
Haas, A. F., Nelson, C. E., Kelly, L. W., Carlson, C. A., Rohwer, F., Leichter, J. J., Wyatt, A., and Smith, J. E.: Effects of coral reef benthic primary producers on dissolved organic carbon and microbial activity, PloS one, 6, e27973, https://doi.org/10.1371/journal.pone.0027973, 2011.
Haas, A. F., Nelson, C. E., Rohwer, F., Wegley-Kelly, L., Quistad, S. D., Carlson, C. A., Leichter, J. J., Hatay, M., and Smith, J. E.: Influence of coral and algal exudates on microbially mediated reef metabolism, PeerJ, 1, e108, https://doi.org/10.7717/peerj.108, 2013.
Haas, A. F., Fairoz, M. F., Kelly, L. W., Nelson, C. E., Dinsdale, E. A., Edwards, R. A., Giles, S., Hatay, M., Hisakawa, N., Knowles, B., and Lim, Y. W.: Global microbialization of coral reefs, Nat. Microbiol., 1, 1–7, 2016.
Hörnlein, C., Confurius-Guns, V., Stal, L. J., and Bolhuis, H.: Daily rhythmicity in coastal microbial mats, NPJ Biofilms Microbiomes, 4, 1–11, 2018.
Hughes, T. P.: Catastrophes, phase shifts, and large-scale degradation of a Caribbean coral reef, Science, 265, 1547–1551, 1994.
Hughes, T. P., Barnes, M. L., Bellwood, D. R., Cinner, J. E., Cumming, G. S., Jackson, J. B., Kleypas, J., Van De Leemput, I. A., Lough, J. M., Morrison, T. H. and Palumbi, S. R., Coral reefs in the Anthropocene, Nature, 546, 82–90, 2017.
Hydes, D. J., Aoyama, M., Aminot, A., Bakker, K., Becker, S., Coverly, S., Daniel, A., Dickson, A. G., Grosso, O., Kerouel, R., Van Ooijen, J., Sato, K., Tanhua, T., Woodward, M., and Zhang, J. Z.: Determination of dissolved nutrients (N, P, Si) in seawater with high precision and inter-comparability using gas-segmented continuous flow analysers, The GO-SHIP Repeart Hydrography Manual: A Collection of Expert Reports and Guidelines, 1–87, https://doi.org/10.25607/OBP-555, 2010.
Jackson, J. B. C., Donovan, M. K., Cramer, K. L., and Lam, V.: Status and Trends of Caribbean Coral Reefs: 1970–2012, GCRMN, IUCN, Gland, Switzerland, 306, https://doi.org/10.1242/jeb.061267, 2014.
Karcher, D. B., Roth, F., Carvalho, S., El-Khaled, Y. C., Tilstra, A., Kürten, B., Struck, U., Jones, B. H., and Wild, C.: Nitrogen eutrophication particularly promotes turf algae in coral reefs of the central Red Sea, PeerJ, 8, e8737, https://doi.org/10.7717/peerj.8737, 2020.
Kelly, L. W., Nelson, C. E., Haas, A. F., Naliboff, D. S., Calhoun, S., Carlson, C. A., Edwards, R. A., Fox, M. D., Hatay, M., Johnson, M. D., and Kelly, E. L.: Diel population and functional synchrony of microbial communities on coral reefs, Nat. Commun., 10, 1–9, 2019.
Kline, D. I., Teneva, L., Schneider, K., Miard, T., Chai, A., Marker, M., Headley, K., Opdyke, B., Nash, M., Valetich, M., and Caves, J. K.: A short-term in situ CO2 enrichment experiment on Heron Island (GBR), Sci. Rep., 2, 1–9, 2012.
Koop, K., Booth, D., Broadbent, A., Brodie, J., Bucher, D., Capone, D., Coll, J., Dennison, W., Erdmann, M., Harrison, P., Hoegh-Guldberg, O., Hutchings, P., Jones, G. B., Larkum, A. W. D., O'Neil, J., Steven, A., Tentori, E., Ward, S., Williamson, J., and Yellowlees, D.: ENCORE: The effect of nutrient enrichment on coral reefs. Synthesis of results and conclusions, Mar. Pollut. Bull., 42, 91–120, https://doi.org/10.1016/S0025-326X(00)00181-8, 2001.
Koweek, D., Dunbar, R. B., Price, N., Mucciarone, D., and Teneva, L.: Environmental and ecological controls of coral community metabolism on Palmyra Atoll, Coral Reefs, 34, 339–351, https://doi.org/10.1007/s00338-014-1217-3, 2015.
Kwon, G., Le, L. T., Jeon, J., Noh, J., Jang, Y., Kang, D., and Jahng, D.: Effects of light and mass ratio of microalgae and nitrifiers on the rates of ammonia oxidation and nitrate production, Biochem. Eng. J., 161, 107656, https://doi.org/10.1016/j.bej.2020.107656, 2020.
Langdon, C. and Atkinson, M. J.: Effect of elevated pCO2 on photosynthesis and calcification of corals and interactions with seasonal change in temperature/ irradiance and nutrient enrichment, J. Geophys. Res.-Oceans, 110, 1–16, https://doi.org/10.1029/2004JC002576, 2005.
Liu, X., Byrne, R. H., Lindemuth, M., Easley, R., and Mathis, J. T.: An automated procedure for laboratory and shipboard spectrophotometric measurements of seawater alkalinity: Continuously monitored single-step acid additions, Mar. Chem., 174, 141–146, https://doi.org/10.1016/j.marchem.2015.06.008, 2015.
Matsuzaki, S. I. S., Sasaki, T., and Akasaka, M.: Consequences of the introduction of exotic and translocated species and future extirpations on the functional diversity of freshwater fish assemblages, Glob. Ecol. Biogeogr., 22, 1071–1082, 2013.
McGill, B. J., Dornelas, M., Gotelli, N. J., and Magurran, A. E.: Fifteen forms of biodiversity trend in the Anthropocene, Trends Ecol. Evol., 30, 104–113, 2015.
McGillis, W. R., Langdon, C., Loose, B., Yates, K. K., and Corredor, J.: Productivity of a coral reef using boundary layer and enclosure methods, Geophys. Res. Lett, 38, 1–5, 2011.
McWilliam, M., Hoogenboom, M. O., Baird, A. H., Kuo, C. Y., Madin, J. S., and Hughes, T. P.: Biogeographical disparity in the functional diversity and redundancy of corals, P. Natl. Acad. Sci. USA, 115, 3084–3089, 2018.
Moberg, F. and Folke, C.: Ecological goods and services of coral reef ecosystems, Ecol. Econ., 29, 215–233, 1999.
Muehllehner, N., Langdon, C., Venti, A., and Kadko, D.: Dynamics of carbonate chemistry, production, and calcification of the Florida Reef Tract (2009–2010): Evidence for seasonal dissolution, Global Biogeochem. Cy., 30, 661–688, https://doi.org/10.1002/2015GB005327, 2016.
Mueller, B., Goeij, J. M. De, Vermeij, M. J. A., Mulders, Y., Ent, E. Van Der, Ribes, M., and van Duyl, F. C.: Natural Diet of Coral-Excavating Sponges Consists Mainly of Dissolved Organic Carbon (DOC), PloS one, 9, e90152, https://doi.org/10.1371/journal.pone.0090152, 2014.
Mueller, B., Den Haan, J., Visser, P. M., Vermeij, M. J., and Van Duyl, F. C.: Effect of light and nutrient availability on the release of dissolved organic carbon (DOC) by Caribbean turf algae, Sci. Rep., 6, 1–9, 2016.
Nelson, C. E., Goldberg, S. J., Kelly, L. W., Haas, A. F., Smith, J. E., Rohwer, F., and Carlson, C. A.: Coral and macroalgal exudates vary in neutral sugar composition and differentially enrich reef bacterioplankton lineages, ISME J., 7, 962–979, 2013.
Oliver, T. H., Heard, M. S., Isaac, N. J., Roy, D. B., Procter, D., Eigenbrod, F., Freckleton, R., Hector, A., Orme, C. D. L., Petchey, O. L., and Proença, V.: Biodiversity and resilience of ecosystem functions, Trends Ecol. Evol., 30, 673–684, 2015.
O'Neil, J. M. and Capone, D. G.: Nitrogen cycling in coral reef environments, Nitrogen in the marine environment, edited by: Capone, D. G., Bronk, D. A., Mulholland, M. R., and Carpenter, E. J., 949–989, https://doi.org/10.1016/B978-0-12-372522-6.00021-9, 2008.
Perry, C. T., Murphy, G. N., Kench, P. S., Smithers, S. G., Edinger, E. N., Steneck, R. S., and Mumby, P. J.: Caribbean-wide decline in carbonate production threatens coral reef growth, Nat. Commun., 4, 1402, https://doi.org/10.1038/ncomms2409, 2013.
Petsch, D. K., Saito, V. S., Landeiro, V. L., Silva, T. S., Bini, L. M., Heino, J., Soininen, J., Tolonen, K. T., Jyrkänkallio-Mikkola, J., Pajunen, V., and Siqueira, T.: Beta diversity of stream insects differs between boreal and subtropical regions, but land use does not generally cause biotic homogenization, Freshw. Sci., 40, 53–64, https://doi.org/10.1086/712565, 2020.
Pfister, C. A. and Altabet, M. A.: Enhanced microbial nitrogen transformations in association with macrobiota from the rocky intertidal, Biogeosciences, 16, 193–206, 2019.
R Core Team: R: A language and environment for statistical computing. R Foundation for Statistical Computing, Vienna, Austria, available at: https://www.R-project.org/, last access: 26 August 2020.
Rädecker, N., Pogoreutz, C., Voolstra, C. R., Wiedenmann, J., and Wild, C.: Nitrogen cycling in corals: the key to understanding holobiont functioning?, Trends Microbiol., 23, 490–497, 2015.
Roff, G. and Mumby, P. J.: Global disparity in the resilience of coral reefs, Elsevier Ltd., Trends Ecol. Evol., 27, 404–413, https://doi.org/10.1016/j.tree.2012.04.007, 2012.
Romanó de Orte, M., Koweek, D. A., Cyronak, T., Takeshita, Y., Griffin, A., Wolfe, K., Szmant, A., Whitehead, R., Albright, R., and Caldeira, K.: Unexpected role of communities colonizing dead coral substrate in the calcification of coral reefs, Limnol. Oceanogr., 66, 1793–1803, 2021.
Roth, F., Rädecker, N., Carvalho, S., Duarte, C. M., Saderne, V., Anton, A., Silva, L., Calleja, M. L., Morán, X. A. G., Voolstra, C. R., and Kürten, B.: High summer temperatures amplify functional differences between coral-and algae-dominated reef communities, Ecology, 102, e03226, https://doi.org/10.1002/ecy.3226, 2020.
Roth, F., El-Khaled, Y. C., Karcher, D. B., Rädecker, N., Carvalho, S., Duarte, C. M., Silva, L., Calleja, M. L., Morán, X. A. G., Jones, B. H., and Voolstra, C. R.: Nutrient pollution enhances productivity and framework dissolution in algae-but not in coral-dominated reef communities, Mar. Pollut. Bull., 168, 112444, https://doi.org/10.1016/j.marpolbul.2021.112444, 2021.
Scheffers, S. R., Nieuwland, G., Bak, R. P. M., and Van Duyl, F. C.: Removal of bacteria and nutrient dynamics within the coral reef framework of Curaçao (Netherlands Antilles), Coral Reefs, 23, 413–422, 2004.
Shaw, E. C., Phinn, S. R, Tilbrook, B., and Steven, A.: Comparability of slack water and Lagrangian flow respirometry methods for community metabolic measurements, PloS one, 9, e112161, https://doi.org/10.1371/journal.pone.0112161, 2014.
Shaw, E. C., Phinn, S. R., Tilbrook, B., and Steven, A.: Natural in situ relationships suggest coral reef calcium carbonate production will decline with ocean acidification. Limnol. Oceanogr., 60, 777–788, https://doi.org/10.1002/lno.10048, 2015.
Silverman, J., Lazar, B., and Erez, J.: Effect of aragonite saturation, temperature, and nutrients on the community calcification rate of a coral reef, J. Geophys. Res.-Oceans, 112, 1–14, https://doi.org/10.1029/2006JC003770, 2007.
Soetaert, K., Hofmann, A. F., Middelburg, J. J., Meysman, F. J., and Greenwood, J.: The effect of biogeochemical processes on pH, Mar. Chem., 106, 380–401, 2007.
Soetaert, K. and Petzoldt, T.: Inverse modelling, sensitivity and Monte Carlo analysis in R using package FME, J. Stat. Softw, 33, 1–28, 2010.
Soetaert, K. E., Petzoldt, T., and Setzer, R. W.: Solving differential equations in R: package deSolve, J. Stat. Softw, 33, 1–25, https://doi.org/10.18637/jss, 2010.
Sonnier, G., Johnson, S. E., Amatangelo, K. L., Rogers, D. A., and Waller, D. M.: Is taxonomic homogenization linked to functional homogenization in temperate forests?, Glob. Ecol. Biogeogr., 23, 894–902, 2014.
Stoll, M. H. C., Bakker, K., Nobbe, G. H., and Haese, R. R.: Continuous-flow analysis of dissolved inorganic carbon content in seawater, Anal. Chem., 73, 4111–4116, https://doi.org/10.1021/ac010303r, 2001.
Takeshita, Y., McGillis, W., Briggs, E. M., Carter, A. L., Donham, E. M., Martz, T. R., Price, N. N., and Smith, J. E.: Assessment of net community production and calcification of a coral reef using a boundary layer approach, J. Geophys. Res.-Oceans, 121, 5655–5671, 2016.
Tobias, N. and Monika, W.: Does taxonomic homogenization imply functional homogenization in temperate forest herb layer communities?, Plant Ecol., 213, 431–443, 2012.
van Duyl, F. C.: Atlas of the living reefs of Curaçao and Bonaire (Netherlands Antilles), 117, Netherlands Foundation for Scientific Research in Surinam and the Netherlands Antilles, Utrecht, 1985.
van Heuven, S. M. A. C., Webb, A. E., Bakker, D. M. De, Meesters, E., Duyl, F. C. Van, Reichart, G., and Lennart, J.: In-situ incubation of a coral patch for community-scale assessment of metabolic and chemical processes on a reef slope, PeerJ, 6, 1–24, https://doi.org/10.7717/peerj.5966, 2018.
Vermeij, M. J., Van Moorselaar, I., Engelhard, S., Hörnlein, C., Vonk, S. M., and Visser, P. M.: The effects of nutrient enrichment and herbivore abundance on the ability of turf algae to overgrow coral in the Caribbean, PloS one, 5, e14312, https://doi.org/10.1371/journal.pone.0014312, 2010.
Webb, A. E., van Heuven, S. M., de Bakker, D. M., van Duyl, F. C., Reichart, G. J., and de Nooijer, L. J.: Combined effects of experimental acidification and eutrophication on reef sponge bioerosion rates, Front. Mar. Sci., 4, 311, https://doi.org/10.3389/fmars.2017.00311, 2017.
Webb, K. L. and Wiebe, W. J.: Nitrification on a coral reef, Can. J. Microbiol., 21, 1427–1431, 1975.
Weber, L. and Apprill, A.: Diel, daily, and spatial variation of coral reef seawater microbial communities, PloS one, 15, e0229442, https://doi.org/10.1371/journal.pone.0229442, 2020.
White, H. J., Montgomery, W. I., Storchová, L., Hořák, D., and Lennon, J. J.: Does functional homogenization accompany taxonomic homogenization of British birds and how do biotic factors and climate affect these processes?, Ecol. Evol., 8, 7365–7377, 2018.
Wolfe, K., Anthony, K., Babcock, R. C., Bay, L., Bourne, D. G., Burrows, D., Byrne, M., Deaker, D. J., Diaz-Pulido, G., Frade, P. R., and Gonzalez-Rivero, M.: Priority species to support the functional integrity of coral reefs. Oceanogr. Mar. Biol., 58, 179–318, https://doi.org/10.1201/9780429351495-5, 2020.
Yates, K. K. and Halley, R. B.: Measuring coral reef community metabolism using new benthic chamber technology, Coral Reefs, 22, 247–255, https://doi.org/10.1007/s00338-003-0314-5, 2003.