the Creative Commons Attribution 4.0 License.
the Creative Commons Attribution 4.0 License.
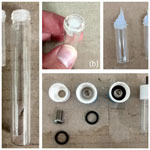
Technical note: Inexpensive modification of Exetainers for the reliable storage of trace-level hydrogen and carbon monoxide gas samples
Philipp A. Nauer
Eleonora Chiri
Thanavit Jirapanjawat
Chris Greening
Atmospheric trace gases such as dihydrogen (H2), carbon monoxide (CO) and methane (CH4) play important roles in microbial metabolism and biogeochemical cycles. Analysis of these gases at trace levels requires reliable storage of discrete samples of low volume. While commercial sampling vials such as Exetainers® have been tested for CH4 and other greenhouse gases, no information on reliable storage is available for H2 and CO. We show that vials sealed with butyl rubber stoppers are not suitable for storing H2 and CO due to release of these gases from rubber material. Treating butyl septa with NaOH reduced trace-gas release, but contamination was still substantial, with H2 and CO mixing ratios in air samples increasing by a factor of 3 and 10 after 30 d of storage in conventional 12 mL Exetainers. All tested materials showed a near-linear increase in H2 and CO mixing ratios, indicating a zero-order reaction and material degradation as the underlying cause. Among the rubber materials tested, silicone showed the lowest potential for H2 and CO release. We thus propose modifying Exetainers by closing them with a silicone plug to minimise contamination and sealing them with a stainless-steel bolt and O-ring as a secondary diffusion barrier for long-term storage. Such modified Exetainers exhibited stable mixing ratios of H2 and CH4 exceeding 60 d of storage at atmospheric and elevated (10 ppm) mixing ratios. The increase of CO was still measurable but was 9 times lower than in conventional Exetainers with treated septa; this can be corrected for due to its linearity by storing a standard gas alongside the samples. The proposed modification is inexpensive, scalable and robust, and thus it enables reliable storage of large numbers of low-volume gas samples from remote field locations.
- Article
(2764 KB) - Full-text XML
-
Supplement
(890 KB) - BibTeX
- EndNote
Dihydrogen (H2) and carbon monoxide (CO) are trace gases present in the atmosphere at 0.53 ppm and approximately 0.15 ppm (Ehhalt and Rohrer, 2009; Petrenko et al., 2013). They are important intermediates in numerous biogeochemical reactions, with environmental equilibrium concentrations kept at trace levels by tightly controlled production and consumption reactions (Hoehler et al., 1998; Khalil and Rasmussen, 1990). Atmospheric H2 and CO play an important role in microbial sustenance for soil bacteria, and soils are an important sink in the global atmospheric budget (Constant et al., 2009; Cordero et al., 2019; Greening et al., 2015; Liu et al., 2018). In addition, both gases can be produced and consumed abiotically in photochemical or naturally occurring redox reactions (Conrad and Seiler, 1985; Fraser et al., 2015; Hoehler, 2005; Lee et al., 2012).
Investigating the turnover of environmental H2 and CO at trace concentrations generally requires the collection of discrete samples for analysis with a sensitive gas chromatography system (GC). Ideally, the GC is field-deployable and measurements can be conducted in situ, which eliminates the problem of gas storage altogether (King and Weber, 2008; Meredith et al., 2017). Alternatively, a field laboratory or shipboard setup may allow for short-term (minutes to hours) storage of samples in syringes or gas-tight sampling bags (Conrad and Seiler, 1988). However, such arrangements are not always feasible (e.g. at remote or inaccessible field sites), and samples have to be stored for longer periods (days to months).
Glass flasks or bulbs of 0.5–1 L volume sealed with hoses or O-rings have been found to provide stable, long-term storage of a variety of atmospheric trace gases and their stable isotope ratios (Rothe et al., 2005; Thrun et al., 1979). They are routinely used in applications with strict stability requirements of less than a few parts per billion deviation after many months of storage, e.g. for cooperative atmospheric trace-gas monitoring (https://www.esrl.noaa.gov/gmd/ccgg/flask.html; last access: 18 November 2020) or sampling campaigns involving isotopic ratios of H2 (Chen et al., 2015; Schmitt et al., 2009). Other similar designs include stainless-steel flasks and cylinders (Khalil et al., 1990; Sulyok et al., 2001). Although these systems represent the “gold standard” for gas-sample storage, they have two major disadvantages: they require the collection of large sample volumes of > 1 L, and they are often prohibitively expensive for large-scale field campaigns with hundreds or thousands of samples.
In applications where signals are large and thus precision criteria less stringent, e.g. for measuring environmental gas fluxes, glass vials of a few millilitres volume closed with butyl rubber septa (either crimp-capped or screw-capped, e.g. Exetainers®) are widely used containers to store trace-gas samples. They are relatively inexpensive and fit many GC autosamplers, making them ideally suitable for large measurement campaigns. In particular, Exetainers have shown good stability (< 5 % deviation from a reference gas) of trace gases such as methane (CH4), nitrous oxide (N2O) and carbon dioxide (CO2) of up to 1 month, regardless of temperature (Faust and Liebig, 2018; Glatzel and Well, 2008; Rochette and Bertrand, 2003). Longer storage times induced deviations of up to 30 % from the reference gas but could be corrected for by storing a standard gas together with the samples (Faust and Liebig, 2018; Laughlin and Stevens, 2003).
To our knowledge, storage of H2 and CO at trace levels in Exetainers or crimp-cap vials have not been investigated. However, anecdotal reports of rapid sample contamination with H2 and CO from butyl stoppers has been an ongoing concern. Here we demonstrate that various butyl rubber septa commonly used for sealing glass vials release significant amounts of H2 and CO over short periods of time. We then propose a simple modification to seal Exetainers with silicone sealant and a stainless-steel bolt and confirm the long-term stability of H2, CH4 and to a lesser extent CO mixing ratios.
2.1 Demonstration of trace-gas release from rubber materials
A selection of typical rubber materials used for sealing gas-sampling containers were tested for release of H2, CO and CH4 by incubating them for several days in closed containers. Exetainers of 12 mL volume were chosen for containers as they fitted the autosampler of the GC, minimising variability due to manual sample injection. A total of 12 different treatments were prepared in quadruplicates; details of the treatments and rubber materials are summarised in Table 1. Some treatments involved pre-treating butyl rubber by (i) boiling for 2 h in 0.1 M NaOH and then twice in Milli-Q water (Lin et al., 2012) and (ii) washing it with a surfactant (Tween 20), followed by rinsing and autoclaving it twice (Ruth Henneberger, personal communication, 2012). After pre-treatments, rubber materials were weighed to 2 or 4 g depending on treatment and then cut to smaller pieces to fit into Exetainers. Five control Exetainers were prepared empty. All samples were closed with a NaOH pre-treated Exetainer septum (typical weight 0.6 g); thus, all treatments including controls were equally exposed to this septum in the lid. In addition, empty 3 mL control Exetainers were prepared in triplicates for each time point to investigate the effect of a smaller sample volume.
Table 1Treatments for testing rubber materials for H2 and CO release. The control treatments consisted of empty Exetainers; the other treatments contained the listed amount of material, excluding the approximately 0.6 g of pre-treated Exetainer septa used for sealing all treatments and controls.
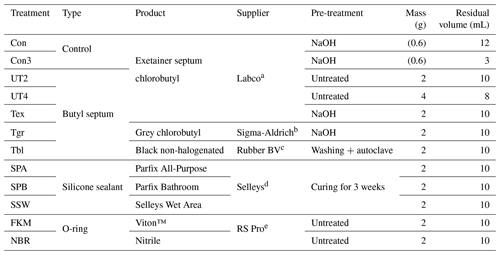
a Labco Limited, Lampeter, Ceredigion, SA48 7HH United Kingdom. b Rubber BV, 1211 JG Hilversum, the Netherlands. c Sigma-Aldrich Pty Ltd (a subsidiary of Merck), Macquarie Park, NSW 2113, Australia. d Selleys, a division of DuluxGroup (Australia) Pty Ltd, Clayton VIC 3168, Australia. e RS Components Pty Ltd, Smithfield, NSW 2164, Australia.
At the start of the experiment, all samples were flushed with a reference gas for at least 5 min at high flow (> 1 L min−1) with pressure regulated to 180 kPa at closure. The reference gas consisted of pre-calibrated industrial-grade pressurised air with mixing ratios of 0.568 ppm H2, 0.665 ppm CO and 1.96 ppm CH4. Samples were stored in the dark and measured five times over the course of 9 d; samples were measured repeatedly, except for the 3 mL control Exetainers, which were discarded after measurements due to insufficient volume for repeated sampling. Measurements of H2, CO and CH4 were performed on a VICI TGA 6k equipped with an autosampler and a sample-injection loop of 1 mL, using a pulse-discharge helium ionisation detector as already described by Islam et al. (2019). The detection limits for the three gases were 61 ppb for H2, 10 ppb for CO and 170 ppb for CH4, as determined by replicate samples (n = 7) of zero-grade air zero (BOC Australia, North Ryde, NSW, Australia).
2.2 Exetainer modifications
Based on the materials test, two simple modifications of Exetainers (silicone-sealed Exetainers, SEs) are proposed to minimise contamination of gas samples containing H2 and CO at trace levels. The modifications consist of (i) a small, permanent plug of silicone sealant that can be pierced with needles during short-term sample handling and minimises contamination during long-term storage and (ii) a stainless-steel bolt and Viton (FKM) O-ring instead of a butyl septum to provide a long-term seal blocking diffusive gas exchange. Selleys Wet Area silicone sealant was used to fill the inside top 5–10 mm of the Exetainer glass vials (Fig. 1a and b). The sealant was administered with a 10 mL plastic syringe for finer handling. First, a thin layer was applied to close the bottom end of the plug. Following this, the plug was filled upwards to avoid slow expulsion of sealant due to overpressure in the Exetainer. A surplus of about the same amount of sealant was administered at the top to account for potential contraction during curing (Fig. 1c). After curing for 1–2 weeks, the surplus was cut at the rim of the glass thread with a scalpel, while also scraping off any residual silicone from the rim. Newly prepared SEs were then flushed with high-purity dinitrogen (N2) at slight overpressure and placed in a vacuum chamber for 2 weeks to extract residual gases entrapped in the silicone. However, this vacuum step is not strictly necessary, as good results were also achieved with silicone Exetainers flushed with N2 and left in ambient air for several weeks.
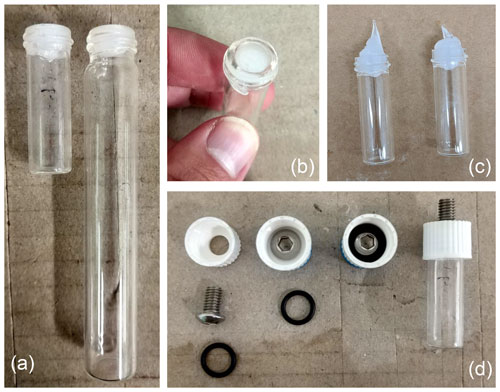
Figure 1Simple and inexpensive modification of Exetainers for minimising contamination of gas samples with dihydrogen (H2) and carbon monoxide (CO) at trace levels: (a, b) finished silicone-sealed Exetainers (SEs), (c) SE after curing and before cutting the excess, and (d) replacing the septum with a stainless-steel bolt and O-ring for long-term storage.
Well-prepared SEs should be gas-tight in the short term (minutes to hours) and hold overpressure for several days or weeks. However, gas diffusion through silicone is rapid compared to butyl rubber (Bhide and Stern, 1991; Van Amerongen, 1946), and thus SEs require a second seal for long-term storage. For this purpose, commercially available button-head stainless-steel bolts (M6 × 10 mm, grade 304) were inserted into empty Exetainer plastic lids, and a Viton O-ring (9.25 mm ID, 12.7 mm OD) was added to seal the bolt against the Exetainer glass rim (Fig. 1d). In principle, any disc of an impermeable and inert material fitting into the Exetainer cap may serve as a secondary diffusion barrier. In our case, using commercial M6 bolts was the most inexpensive and most readily available option, with the rounded button-head having the additional benefit of reducing the air cavity between bolt and silicone plug. For sample access or analysis, the bolt and O-ring can simply be removed, with the pierceable silicone plug containing the sample. For analysis times of several hours, e.g. when using an autosampler, SEs can be closed with a conventional treated septum to minimise diffusive exchange.
2.3 Long-term storage test of silicone-sealed Exetainers
Long-term storage tests were conducted with 3 and 12 mL SEs containing different reference gases, to test sample stability and tightness of SEs. Quadruplicate SEs of both volumes were prepared for each of the five time points by either flushing with pre-calibrated pressurised air (0.643 ppm H2 and 1.99 ppm CH4; CO not detected) to test for gas release from materials or flushing with a calibration gas (10.2 ppm H2, 9.90 ppm CO and 10.1 ppm CH4) to test for tightness of the seal. The SEs were prepared 8 months prior to flushing. The final pressure after flushing was set to 180 kPa. For comparison, a batch of 12 mL SEs was left uncapped and flushed with calibration gas, and a batch of 12 mL conventional Exetainers was capped with NaOH-treated septa and flushed with pressurised air. All samples were stored in the dark, and analysed alongside the reference gases after 3, 10, 30, 60, and 92 d of storage.
3.1 Hydrogen and carbon monoxide release from rubber materials
A short-term storage test using pre-calibrated pressurised air as reference gas was conducted with various sealing rubbers. All tested rubber materials released H2 and CO during short storage times of a few hours or days, with significant differences between materials (Figs. 2 and 3; Table S1 in the Supplement). Untreated Exetainer septa released the highest amounts of both H2 and CO; control-corrected mean mixing ratios in 12 mL Exetainers reached 16 ppm and 33 ppm from 2 g of material after 9 d (UT2, Fig. 2). This was 30 and 50 times higher than the initial reference gas and close to 20 times higher than empty control Exetainers. The increase was linear and roughly proportional to mass, with double the material (4 g) approximately doubling the mixing ratios of H2 and CO (UT4, Fig. 2). Rates of H2 and CO release were thus nearly identical for UT2 and UT4 treatments when normalised to mass (Fig. 3).
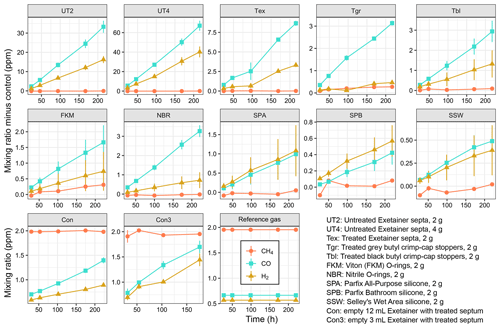
Figure 2Dihydrogen (H2), carbon monoxide (CO) and methane (CH4) released from various sealing rubbers and corrected for release in empty control vials. Replicate samples (n = 4) of 2 or 4 g of rubber material were prepared in 12 mL Exetainers sealed with a treated Exetainer septum and flushed with pressurised air as a reference gas. Controls consisted of empty Exetainers (n = 6) sealed with a treated Exetainer septum. Samples were measured repeatedly except for Con3 samples where n = 3 replicates were sacrificed for each measurement due to low gas volume. Error bars denote standard error of the mean. Please note the different scales of the y axes.
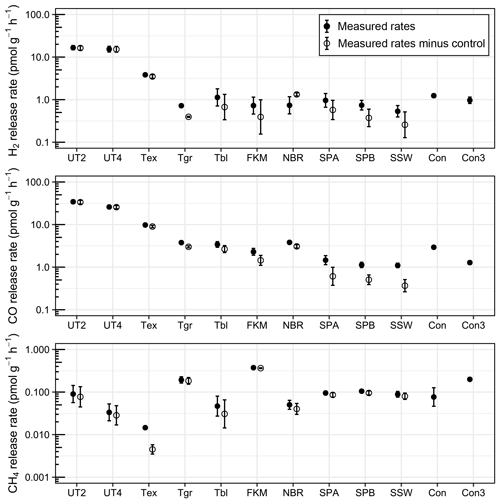
Figure 3Rates of trace-gas release from various rubber materials in 3 mL (Con3) and 12 mL (all other treatments) Exetainers (n=4), normalised to mass. Error bars denote standard error of the mean. Please refer to Fig. 2 for the treatment legend.
Treated materials performed significantly better. Boiling Exetainer septa with 0.1 M NaOH reduced H2 and CO release by a factor of 4 (Tex; Fig. 2); however, corrected mixing ratios still reached 3 and 8 ppm at the end of the experiment, with an increase of 3 pmol H2 and 10 pmol CO every hour per gram of material (Fig. 3). Empty control Exetainers of 12 mL volume closed with a treated septum showed mean H2 and CO mixing ratios of 0.89 and 1.4 ppm after 9 d, i.e. 1.6 and 2.1 times higher than initial mixing ratios in air, respectively. Smaller 3 mL Exetainers showed an even higher contamination with 1.4 ppm H2 and 1.7 ppm CO after 7 d.
Grey crimp-cap stoppers treated in the same way performed slightly better, with little H2 release and lower CO release than treated Exetainer septa (Tgr, Figs. 2 and 3). Washed and autoclaved black crimp-cap stoppers were comparable to grey stoppers for CO, but released slightly more H2 (Tbl; Figs. 2 and 3). Untreated O-ring materials performed better than all butyl rubbers; Viton O-rings showed lower H2 and CO release, and nitrile O-rings showed lower H2 release than butyl rubbers (FKM and NBR; Figs. 2 and 3). Silicone sealants showed the lowest potential for H2 and CO release (SPA, SPB and SSW; Fig. 2), with SSW performing the best. Interestingly, H2 and CO release rates were similar for all silicones, while they were nearly twice as high for CO compared to H2 for all butyl and O-ring rubbers (Figs. 2 and 3).
None of the tested rubber materials caused increasing CH4 mixing ratios (Fig. 2) except Viton O-rings, which had a small release of approximately 0.3 pmol g−1 h−1 (Fig. 3). Most treatments showed similar CH4 mixing ratios than controls, which occasionally led to negative mixing ratios when corrected (Fig. 2).
3.2 Stability of trace-gas samples in silicone-sealed Exetainers
Gas samples of two reference gases (pressurised air and a 10 ppm calibration mix) were stored up to 3 months in Exetainers with different seals and compared against fresh reference gas (Figs. 4 and S1). Conventional 12 mL Exetainers with treated septa showed a significant increase in H2 and CO mixing ratios during storage of pressurised air, with deviations from fresh gas larger than 5 % after 3 d of storage (Fig. 4); CH4 mixing ratios were within 5 % of fresh gas for at least 30 d and slightly higher afterwards. In contrast, pressurised air stored in 12 mL SEs sealed with a stainless-steel bolt remained stable (deviation < 5 %) for more than 60 d for both CH4 and H2. Mixing ratios of CO increased from below detection limit (∼ 70 ppb) to 0.18 ppm in 3 d and around 0.6 ppm in 92 d of storage; however, the rate of increase was approximately linear and nearly an order of magnitude lower compared to conventional Exetainers (Fig. S1). The 10 ppm calibration gas remained stable in bolt-sealed 12 mL SEs for all three gases for at least 60 d, and deviations were less than 10 % after 92 d of storage (Fig. 4). The smaller 3 mL SEs showed higher variability in both fresh and stored gases, with CH4 and H2 stable for up to 10 d (Fig. 4). Both CH4 and H2 mixing ratios increased with storage in pressurised air and decreased in the calibration mixture; for CO we observed a clear increase in both reference gases. We also tested the calibration mix in SEs with the silicone left open to laboratory air and observed the expected exponential decrease for all three gases as they equilibrated with air.
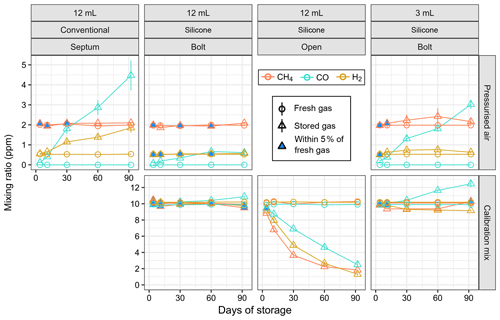
Figure 4Measured mixing ratio of reference gases (pressurised air and calibration mix) freshly flushed from cylinder versus stored for up to 3 months in Exetainers with different seals. Error bars indicate standard error of the mean. The blue-filled shapes indicate that the difference between freshly flushed and stored gas is within 5 % of fresh gas. Note that carbon monoxide (CO) was not detected in fresh pressurised air and that the mixing ratio is plotted as zero.
Our results clearly demonstrate that commercially available gas-sample vials sealed with butyl rubber are not suited for the storage of gas samples with trace levels of H2 and CO. Among tested rubber materials, butyl rubbers exhibited the highest rates of H2 and particularly CO release, regardless of any pre-treatment. Untreated Exetainer septa performed particularly poorly; measured rates indicate that mixing ratios of H2 and CO in air samples stored in conventional 12 mL Exetainers could essentially double and quadruple every 24 h, respectively. Treatment of septa with NaOH greatly reduced potential contamination, but mixing ratios in air samples could still double approximately every 2 weeks for H2 and every week for CO. The level of contamination was proportional to the volume of the vial and the mass of rubber (a proxy for the specific surface area in contact with the enclosed gas phase); therefore, form factor and volume of vials have to be considered. For example, serum vials with an increased volume of > 100 mL and thus lower exposed surface area relative to volume may still be suitable for gas storage or incubation experiments lasting a few weeks if treated stoppers are used (Islam et al., 2019, 2020; Kessler et al., 2019).
Viton and nitrile rubbers performed much better than butyl septa for H2, though CO release was still substantial. In principle, butyl septa could be replaced with Viton or nitrile; some commercial products are available for crimp-cap vials and may be an alternative for laboratories with an existing stock of such vials. We have not tested septa made from these materials, however, and tightness, possible treatments and resistance to multiple piercings will need to be evaluated alongside potential contamination. Particularly for CO, Viton and nitrile may not be much better than treated butyl rubber.
Silicone sealants showed the lowest potential for H2 and CO release. Assuming a second barrier to control diffusion (discussed below) and a typical septum weight of 0.5 g, contamination after 1 month of storage would theoretically accumulate to 0.1 ppm H2 and 0.2 ppm CO in vials sealed with silicone. For H2, this is close to the contamination introduced by piercing the septum with a needle, which inevitably introduces a small amount of ambient air (Lin et al., 2012). Such a rate can be acceptable for many projects, particularly when investigating relative changes. In addition, our selection of silicone sealants was based on local availability and thus rather limited. Although all three products performed reasonably well, there may be better or worse options among the multitude of commercially available silicone-based sealants. We thus strongly recommend testing some locally available products for gas release before preparing SEs.
Specific mechanisms for H2 and CO release from different rubbers have not been investigated here. We can, however, highlight some common patterns among the different rubbers tested. First, a near-linear increase in H2 and CO in all rubber materials, regardless of pre-treatment and mixing ratio, indicates a zero-order reaction and thus points towards degradation of material as underlying cause, rather than a release of entrapped or dissolved gas. Entrapped or dissolved gases would slowly equilibrate with the gas phase, and thus rates would show an inverse correlation with mixing ratios. Silicones showed slightly reduced rates towards the end of the release experiment (Fig. 2), but the trend was weak. We cannot exclude the possibility of entrapped or dissolved gases at higher concentrations in the materials, but this appears less realistic when considering negligible release of CH4. Chemical degradation of rubbers can occur via multiple and complex reactions and strongly depends on its composition and polymer structure (Dubey et al., 1995). It is likely that silicones degrade differently to butyl and O-ring rubbers, as indicated by the ratio of H2 to CO release of 1 : 1 for silicones and at least 1 : 2 for other rubbers. Temperature may also play an important role for any degradation reaction and thus merits further investigations for potentially reducing contamination. Regardless of the cause of H2 and CO release, among the rubbers tested here only silicones and possibly Viton seem to be suitable for storing small gas samples with H2 and CO at trace levels for longer than a few days.
Our modified SEs with a stainless-steel bolt and O-ring as secondary diffusion barrier provided stable storage for up to 2 months under ambient temperatures for gas samples with H2 and CH4 at ambient levels and all three gases at elevated levels. The SEs thus allow long-term storage of small volumes of trace-level H2 samples, and extend reliable storage times for CH4 samples by 1 month compared to conventional Exetainers (Faust and Liebig, 2018). There was still significant contamination of gas samples with CO at ambient levels, likely released from the silicone plug. However, the increase was 9 times lower than in conventional Exetainers with treated septa. Most importantly, the increase appeared to be linear, and thus it offers the potential to correct for this bias by storing a reference gas alongside the samples (Laughlin and Stevens, 2003). The 3 mL SEs were stable for 3 to 10 d for H2 and CH4 and showed deviations thereafter. However, for these gases mixing ratios stabilised within 10 to 30 d to a slightly higher level with pressurised air and a slightly lower level with calibration mix. We suspect this to be a result of equilibrium partitioning between silicone and gas phase, which is much more pronounced in the 3 mL vials with a higher silicone–gas ratio. This may lead to a “memory” effect when gases from previous samples can partition into silicone and then back into the gas phase in the new sample. In our case, 3 mL SEs were stored in lab air with slightly higher H2 and CH4 mixing ratios than pressurised air and lower mixing ratios than the calibration mix. Therefore, partitioning between gas and silicone could explain the observed pattern in 3 mL SEs but seemed largely negligible in 12 mL SEs. To minimise this effect, we suggest purging SEs with high-purity N2 after measurement and storing them in an atmosphere of known composition, ideally similar to future samples.
Surprisingly, mixing ratios of H2 were much less affected than CO during storage in SEs, unlike in emission experiments where silicones showed similar rates of H2 and CO release (Figs. 2 and 3). However, we also observed high variability in H2 emissions from silicones, with some replicates showing H2 increase comparable to CH4 and others that were comparable to CO. We suspect this was an effect of heterogeneous curing of the large silicone plugs we prepared for the emission experiment, which cured for 3 weeks before being cut into smaller pieces. In contrast, the SEs used in the long-term storage test were made 8 months prior to the test and were fully cured. We therefore speculate that silicones emit H2 only during curing, while CO appears to be released by a degradation reaction at a consistent rate.
We have now employed 12 mL SEs for several projects in terrestrial and marine environments with 400 to 600 samples and storage times of up to 1 month. Once prepared, SEs could be pierced numerous times without compromising tightness, while stainless-steel bolts, O-rings and Exetainer plastic caps have been repeatedly used without any signs of degradation. We have not observed any corrosion of bolts in marine applications, as SEs were kept dry and separate from water samples at all times. However, for certain applications where contact with seawater is inevitable, we recommend marine-grade stainless steel or using nylon bolts (after testing for H2 and CO release). Overall, the proposed system is simple to implement, inexpensive, adaptable, robust and reliable and can be integrated in many existing sampling and processing pipelines.
All data can be made available by the authors upon request.
The supplement related to this article is available online at: https://doi.org/10.5194/bg-18-729-2021-supplement.
PAN, EC and PLMC designed the experiments, and PAN, EC and TJ carried them out. CG and PLMC provided logistical and technical support. PAN prepared the manuscript with contributions from all co-authors.
The authors declare that they have no conflict of interest.
We thank Philip Eickenbusch for fruitful discussions and two anonymous reviewers for their expert assessment of our manuscript and constructive suggestions for improvements.
This research has been supported by the Australian Research Council (grant no. DP180101762; awarded to Perran L. M. Cook and Chris Greening), the Swiss National Science Foundation (grant no. P2EZP3_178421; awarded to Eleonora Chiri), and the Australian National Health and Medical Research Council (grant no. APP1178715; awarded to Chris Greening).
This paper was edited by Andreas Richter and reviewed by two anonymous referees.
Bhide, B. D. and Stern, S. A.: Permeability of silicone polymers to hydrogen, J. Appl. Polym. Sci., 42, 2397–2403, https://doi.org/10.1002/app.1991.070420904, 1991.
Chen, Q., Popa, M. E., Batenburg, A. M., and Röckmann, T.: Isotopic signatures of production and uptake of H2 by soil, Atmos. Chem. Phys., 15, 13003–13021, https://doi.org/10.5194/acp-15-13003-2015, 2015.
Conrad, R. and Seiler, W.: Influence of the Surface Microlayer on the Flux of Nonconservative Trace Gases (CO, H2, CH4, N2O) Across the Ocean-Atmosphere Interface, J. Atmos. Chem., 6, 83–94, https://doi.org/10.1007/BF00048333, 1988.
Conrad, R. and Seller, W.: Characteristics of Abiological Carbon Monoxide Formation from Soil Organic Matter, Humic Acids, and Phenolic Compounds, Environ. Sci. Technol., 19, 1165–1169, https://doi.org/10.1021/es00142a004, 1985.
Constant, P., Poissant, L., and Villemur, R.: Tropospheric H2 budget and the response of its soil uptake under the changing environment, Sci. Total Environ., 407, 1809–1823, https://doi.org/10.1016/j.scitotenv.2008.10.064, 2009.
Cordero, P. R. F., Bayly, K., Leung, P. M., Huang, C., Islam, Z. F., Schittenhelm, R. B., King, G. M., and Greening, C.: Atmospheric carbon monoxide oxidation is a widespread mechanism supporting microbial survival, ISME J., 13, 2868–2881, https://doi.org/10.1038/s41396-019-0479-8, 2019.
Dubey, V., Pandey, S. K., and Rao, N. B. S. N.: Research trends in the degradation of butyl rubber, J. Anal. Appl. Pyrolysis, 34, 111–125, https://doi.org/10.1016/0165-2370(95)00889-M, 1995.
Ehhalt, D. H. and Rohrer, F.: The tropospheric cycle of H2: A critical review, Tellus B, 61, 500–535, https://doi.org/10.1111/j.1600-0889.2009.00416.x, 2009.
Faust, D. R. and Liebig, M. A.: Effects of storage time and temperature on greenhouse gas samples in Exetainer vials with chlorobutyl septa caps, MethodsX, 5, 857–864, https://doi.org/10.1016/j.mex.2018.06.016, 2018.
Fraser, W. T., Blei, E., Fry, S. C., Newman, M. F., Reay, D. S., Smith, K. A., and McLeod, A. R.: Emission of methane, carbon monoxide, carbon dioxide and short-chain hydrocarbons from vegetation foliage under ultraviolet irradiation, Plant Cell Environ., 38, 980–989, https://doi.org/10.1111/pce.12489, 2015.
Glatzel, S. and Well, R.: Evaluation of septum-capped vials for storage of gas samples during air transport, Environ. Monit. Assess., 136, 307–311, https://doi.org/10.1007/s10661-007-9686-2, 2008.
Greening, C., Constant, P., Hards, K., Morales, S. E., Oakeshott, J. G., Russell, R. J., Taylor, M. C., Berney, M., Conrad, R., and Cook, G. M.: Atmospheric hydrogen scavenging: From enzymes to ecosystems, Appl. Environ. Microbiol., 81, 1190–1199, https://doi.org/10.1128/AEM.03364-14, 2015.
Hoehler, T. M.: Biogeochemistry of Dihydrogen (H2), in: Metal Ions in Biological Systems, 9–48, https://doi.org/10.1201/9780824751999, 2005.
Hoehler, T. M., Alperin, M. J., Albert, D. B., and Martens, C. S.: Thermodynamic control on hydrogen concentrations in anoxic sediments, Geochim. Cosmochim. Ac., 62, 1745–1756, https://doi.org/10.1016/S0016-7037(98)00106-9, 1998.
Islam, Z. F., Cordero, P. R. F., Feng, J., Chen, Y.-J., Bay, S. K., Jirapanjawat, T., Gleadow, R. M., Carere, C. R., Stott, M. B., Chiri, E., and Greening, C.: Two Chloroflexi classes independently evolved the ability to persist on atmospheric hydrogen and carbon monoxide, ISME J., 13, 1801–1813, https://doi.org/10.1038/s41396-019-0393-0, 2019.
Islam, Z. F., Welsh, C., Bayly, K., Grinter, R., Southam, G., Gagen, E. J., and Greening, C.: A widely distributed hydrogenase oxidises atmospheric H2 during bacterial growth, ISME J., 14, 2649–2658, https://doi.org/10.1038/s41396-020-0713-4, 2020.
Kessler, A. J., Chen, Y. J., Waite, D. W., Hutchinson, T., Koh, S., Popa, M. E., Beardall, J., Hugenholtz, P., Cook, P. L. M., and Greening, C.: Bacterial fermentation and respiration processes are uncoupled in anoxic permeable sediments, Nat. Microbiol., 4, 1014–1023, https://doi.org/10.1038/s41564-019-0391-z, 2019.
Khalil, M. A. K. and Rasmussen, R. A.: The global cycle of carbon monoxide: Trends and mass balance, Chemosphere, 20, 227–242, https://doi.org/10.1016/0045-6535(90)90098-E, 1990.
Khalil, M. A. K., Rasmussen, R. A., French, J. R., and Holt, J.: The influence of Termites on Atmospheric Trace Gases: CH4, CO2, N2O, CO, and Light Hydrocarbons, J. Geophys. Res., 95 3619–3634, https://doi.org/10.1029/JD095iD04p03619, 1990.
King, G. M. and Weber, C. F.: Interactions between bacterial carbon monoxide and hydrogen consumption and plant development on recent volcanic deposits, ISME J., 2, 195–203, https://doi.org/10.1038/ismej.2007.101, 2008.
Laughlin, R. J. and Stevens, R. J.: Changes in Composition of Nitrogen-15-Labeled Gases during Storage in Septum-Capped Vials, Soil Sci. Soc. Am. J., 67, 540–543, https://doi.org/10.2136/sssaj2003.5400, 2003.
Lee, H., Rahn, T., and Throop, H.: An accounting of C-based trace gas release during abiotic plant litter degradation, Glob. Change Biol., 18, 1185–1195, https://doi.org/10.1111/j.1365-2486.2011.02579.x, 2012.
Lin, Y. S., Heuer, V. B., Goldhammer, T., Kellermann, M. Y., Zabel, M., and Hinrichs, K. U.: Towards constraining H2 concentration in subseafloor sediment: A proposal for combined analysis by two distinct approaches, Geochim. Cosmochim. Ac., 77, 186–201, https://doi.org/10.1016/j.gca.2011.11.008, 2012.
Liu, L., Zhuang, Q., Zhu, Q., Liu, S., van Asperen, H., and Pihlatie, M.: Global soil consumption of atmospheric carbon monoxide: an analysis using a process-based biogeochemistry model, Atmos. Chem. Phys., 18, 7913–7931, https://doi.org/10.5194/acp-18-7913-2018, 2018.
Meredith, L. K., Commane, R., Keenan, T. F., Klosterman, S. T., Munger, J. W., Templer, P. H., Tang, J., Wofsy, S. C., and Prinn, R. G.: Ecosystem fluxes of hydrogen in a mid-latitude forest driven by soil microorganisms and plants, Glob. Change Biol., 23, 906–919, https://doi.org/10.1111/gcb.13463, 2017.
Petrenko, V. V., Martinerie, P., Novelli, P., Etheridge, D. M., Levin, I., Wang, Z., Blunier, T., Chappellaz, J., Kaiser, J., Lang, P., Steele, L. P., Hammer, S., Mak, J., Langenfelds, R. L., Schwander, J., Severinghaus, J. P., Witrant, E., Petron, G., Battle, M. O., Forster, G., Sturges, W. T., Lamarque, J.-F., Steffen, K., and White, J. W. C.: A 60 yr record of atmospheric carbon monoxide reconstructed from Greenland firn air, Atmos. Chem. Phys., 13, 7567–7585, https://doi.org/10.5194/acp-13-7567-2013, 2013.
Rochette, P. and Bertrand, N.: Soil air sample storage and handling using polypropylene syringes and glass vials, Can. J. Soil Sci., 83, 631–637, https://doi.org/10.4141/S03-015, 2003.
Rothe, M., Jordan, A., and Brand, W. A.: Trace gases, d13C and d18O of CO2-in-air samples: storage in glass flasks using PCTFE seals and other effects, Proc. 12th IAEA/WMO Meet. CO2 Expert., Toronto, Canada, September 2003, 64–70, available at: http://hdl.handle.net/11858/00-001M-0000-000E-D36E-0 (last access: 27 January 2021), 2005.
Schmitt, S., Hanselmann, A., Wollschläger, U., Hammer, S., and Levin, I.: Investigation of parameters controlling the soil sink of atmospheric molecular hydrogen, Tellus B, 61B, 416–423, https://doi.org/10.1111/j.1600-0889.2008.00402.x, 2009.
Sulyok, M., Haberhauer-Troyer, C., Rosenberg, E., and Grasserbauer, M.: Investigation of the stability of selected volatile sulfur compounds in different sampling containers, J. Chromatogr. A, 917, 367–374, https://doi.org/10.1016/S0021-9673(01)00654-9, 2001.
Thrun, K. E., Harris, J. C., and Beltis, K.: Gas Sample Storage, U.S. Environmental Protection Agency, Washington, D.C., USA, 1979.
Van Amerongen, G. J.: The permeability of different rubbers to gases and its relation to diffusivity and solubility, J. Appl. Phys., 17, 972–985, https://doi.org/10.1063/1.1707667, 1946.