the Creative Commons Attribution 4.0 License.
the Creative Commons Attribution 4.0 License.
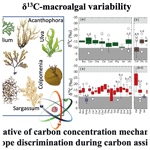
An analysis of the variability in δ13C in macroalgae from the Gulf of California: indicative of carbon concentration mechanisms and isotope discrimination during carbon assimilation
Roberto Velázquez-Ochoa
María Julia Ochoa-Izaguirre
Martín Federico Soto-Jiménez
The isotopic composition of carbon in macroalgae (δ13C) is highly variable, and its prediction is complex concerning terrestrial plants. The determinants of δ13C macroalgal variations were analyzed in a large stock of specimens that vary in taxa and morphology and were collected in shallow marine habitats in the Gulf of California (GC) with distinctive environmental conditions. A large δ13C variability (−34.6 ‰ to −2.2 ‰) was observed. Life-forms (taxonomy 57 %, morphology and structural organization 34 %) explain the variability related to carbon use physiology. Environmental conditions influenced the δ13C macroalgal values but did not change the physiology, which is most likely inherently species-specific. Values of δ13C were used as indicators of the presence or absence of carbon concentrating mechanisms (CCMs) and as integrative values of the isotope discrimination during carbon assimilation in the life cycle macroalgae. Based on δ13C signals, macroalgae were classified in three strategies relative to the capacity of CCM: (1) HCO uptake (δ13C > −10 ‰), (2) using a mix of CO2 and HCO uptake (C > −30 ‰), and (3) CO2 diffusive entry (δ13C < −30 ‰). Most species showed a δ13C that indicates a CCM using a mix of CO2 and HCO uptake. HCO uptake is also widespread among GC macroalgae, with many Ochrophyta species. Few species belonging to Rhodophyta relied on CO2 diffusive entry exclusively, while calcifying macroalgae species using HCO included only Amphiroa and Jania. The isotopic signature evidenced the activity of CCM, but it was inconclusive about the preferential uptake of HCO and CO2 in photosynthesis and the CCM type expressed in macroalgae. In the study of carbon use strategies, diverse, species-specific, and complementary techniques to the isotopic tools are required.
- Article
(5023 KB) - Full-text XML
-
Supplement
(675 KB) - BibTeX
- EndNote
Macroalgae show a wide diversity of thallus morphologies (e.g., filamentous, articulated, flattened), structural organization (e.g., surface area : volume ratio), and various photosynthetic pigments (e.g., Chlorophyll a, b, phycocyanin) (Lobban and Harrison, 1994). According to the predominant pigment contents in the thallus, macroalgae are classified into three phyla. The interaction of morphologies and photosynthetic pigments is classified into dozens of groups (Balata et al., 2011; Littler and Littler, 1980; Littler and Arnold, 1982). For example, the mixture of chlorophyll (a, b) and carotenoids is dominant in Chlorophyta, and chlorophyll (a, c) and fucoxanthin carotenoid are dominant in Ochrophyta, while Rhodophyta contains chlorophyll (a, d), carotenoid, and a mixture of phycobilin (e.g., phycocyanin, phycoerythrin, allophycocyanin) (Bold and Wynne, 1978; Gateau et al., 2017; Masojidek et al., 2004). Both traits work as an excellent approximation to explain the fundamentals of metabolism, growth, zonation, and colonization (Littler and Littler, 1980; Littler and Arnold, 1982; Nielsen and Sand-Jensen, 1990; Vásquez-Elizondo and Enríquez, 2017).
In marine environments, where pH ∼ 8.1±1, the diffusion rate of CO2 in seawater is low. Thus, HCO accounts for 98 % of the total dissolved inorganic carbon (DIC), resulting in a high HCO : CO2 ratio (150 : 1) (Sand-Jensen and Gordon, 1984). Low CO2 concentrations in seawater, which limit macroalgae growth, are compensated for by carbon concentrating mechanisms (CCMs) that increase the internal inorganic carbon concentration near the site of RuBisCo activity (Giordano et al., 2005). Therefore, the absorption of HCO by most macroalgae is the primary source of inorganic carbon for photosynthesis, but some species depend exclusively on the use of dissolved CO2 that enters cells by diffusion (Beardall and Giordano, 2002; Giordano et al., 2005; Maberly et al., 1992; Raven et al., 2002a, b). Hence, macroalgal species with productivity limited by lacking CCMs (having low plasticity for inorganic carbon uptake) seems to be restricted to subtidal habitats and composed mainly of red macroalgae (but without a morphological patron apparent) (Cornwall et al., 2015; Kübler and Dudgeon, 2015). The rest of the macroalgae with CCM occupies the intertidal to the deep subtidal zone.
The habitat features and environmental conditions in marine ecosystems modify the main macroalgae photosynthesis drivers, such as light (Anthony et al., 2004; Johansson and Snoeijs, 2002), DIC (Brodeur et al., 2019; Zeebe and Wolf-Gladrow, 2001), and inorganic nutrients (Ochoa-Izaguirre and Soto-Jiménez, 2015; Teichberg et al., 2010). These factors could generate negative consequences for their productivity, principally when they cause resource limitation. Each factor varies from habitat to habitat (e.g., local scale: from intertidal to subtidal zone; and global scale: from temperate to tropical regions), and in response to these environmental changes, macroalgae can modulate their photosynthetic mechanism (Dudgeon et al., 1990; Kübler and Davison, 1993; Lapointe and Duke, 1984; Young and Beardall, 2005). The modulation, to increase their photosynthetic activity (up-and-down regulation processes), implies a physiological acclimation enhancing the transport of DIC (CO2, HCO) into the cell and its fixation rates (Enríquez and Rodríguez-Román, 2006; Giordano et al., 2005; Klenell et al., 2004; Madsen and Maberly, 2003; Rautenberger et al., 2015; Zou et al., 2004).
The δ13C macroalgal values indicate the carbon source used (CO2 or HCO) in photosynthesis and allow the presence or absence of CCMs to be inferred (Giordano et al., 2005; Maberly et al., 1992; Raven et al., 2002a). However, the isotopic signature may be inconclusive for determining the carbon source's preference (Roleda and Hurd, 2012). Also, the δ13C signal in the algal thallus can be used to indicate the physiological state of photosynthetic metabolism (Kim et al., 2014; Kübler and Dungeon, 2015). For example, δ13C variability depends, in part, on the life-forms' taxonomy, morphology, and structural organization (Lovelock et al., 2020; Marconi et al., 2011; Mercado et al., 2009; Roleda and Hurd, 2012). δ13C is also modulated by the interaction with environmental conditions (e.g., light, DIC, and nutrients) (Carvalho et al., 2010a, b; Cornelisen et al., 2007; Dudley et al., 2010; Mackey et al., 2015; Rautenberger et al., 2015; Roleda and Hurd, 2012). In this study, our objective was to investigate the contributions of life-forms, the changes in the habitat features, and environmental conditions to the δ13C macroalgal variability in communities in the Gulf of California (GC). We collected a large stock of macroalgae specimens of a diversity of species characterized by various morphological and physiological properties to reach our objective. Besides high diversity, in terms of life-forms, we selected various shallow marine habitats along a latitudinal gradient in the GC or the sample collection, characterized by unique and changing environmental factors. The GC features abundant and diverse macroalgae populations, acclimated and adapted to diverse habitats with environmental conditions determining the light, DIC, and nutrient availability. The δ13C signal from the thallus of macroalgae was used as indicative of the presence or absence of CCMs and as integrative values of the isotope discrimination during carbon assimilation and respiration along the life cycle of macroalgae in macroalgal communities in the GC as a function of taxa and environmental factors (Díaz-Pulido et al., 2016; Hepburn et al., 2011; Maberly et al., 1992; Raven et al., 2002a). Because the GC is a subtropical zone with high irradiance and specimens were collected in the intertidal and shallow subtidal zone, we expect to find a high proportion of species with active uptake HCO (δ13C > −10 ‰). A third objective was to explore any geographical pattern in the δ13C macroalgae along and between the GC bioregions. Previous studies have indicated changes in the δ13C signal with latitude, mainly related to the light and temperature (Hofmann and Heesch, 2018; Lovelock et al., 2020; Marconi et al., 2011; Mercado et al., 2009; Stepien, 2015). Macroalgae as biomonitors constitute an efficient tool in monitoring programs in large geographical regions (Balata et al., 2011) and for environmental impact assessments (Ochoa-Izaguirre and Soto-Jiménez, 2015).
2.1 Gulf of California description
The Gulf of California is a subtropical, semi-enclosed sea of the Pacific coast of Mexico, with exceptionally high productivity making it the most important fishing region for Mexico and one of the most biologically diverse worldwide marine areas (Espinosa-Carreón and Valdez-Holguín, 2007; Lluch-Cota et al., 2007; Páez-Osuna et al., 2017; Zeitzschel, 1969). The Gulf of California represents only 0.008 % of the area covered by the seas of the planet (265 894 km2, 150 km wide, and 1000 km long covering >9 ∘ latitude). However, the GC has a high physiographic diversity and is biologically mega-diverse with many endemic species, including ∼ 766 macrofauna species and/or subspecies in which the major number belong to Arthropoda (118 species) and Mollusca (460 species) taxa (Brusca et al., 2005; Espinosa-Carreón and Escobedo-Urías, 2017; Wilkinson et al., 2009) and 116 to macroalgae species (Espinoza-Avalos, 1993; Norris, 1975, 1985).
Regionalization criteria of the GC include phytoplankton distribution (Gilbert and Allen, 1943), topography (Rusnak et al., 1964) and depth (Álvarez-Borrego, 1983), oceanographic characteristics (Álvarez-Borrego, 1983; Marinone and Lavín, 2003; Roden and Emilson, 1979), biogeography (Santamaría-del-Ángel et al., 1994), and bio-optical characteristics (Bastidas-Salamanca et al., 2014). The topography is variable along the GC and includes submarine canyons, basins, and variable continental platforms. Besides, the GC presents complex hydrodynamic processes, including internal waves, fronts, upwelling, vortices, and mixing of tides. The gulf's coastline is divided into three shores: extensive rocky shores, long sandy beaches, numerous scattered estuaries, coastal lagoons, open muddy bays, tidal flats, and coastal wetlands (Lluch-Cota et al., 2007).
The Gulf of California is different in the north and the south, related to a wide range of physicochemical factors. The surface currents seasonally change direction and flow to the southeast with maximum intensity during the winter and to the northwest in summer (Roden, 1958). The northern part is very shallow (<200 m deep on average), divided into the upper gulf, northern gulf, and Midriff Islands regions (Roden, 1958; Roden and Groves, 1959). The surrounding deserts largely influence this region (Norris, 2010), which shows marked seasonal changes in coastal surface seawater temperatures (Marinone, 2007; Martínez-Díaz-de-León et al., 2006). Tidal currents induce a significant cyclonic circulation through June to September and anticyclonic from November to April (Bray, 1988; Carrillo and Palacios-Hernández, 2002; Martínez-Díaz-de-León, 2001; Velasco-Fuentes and Marinone, 1999). The southern part consists of a series of basins whose depths increase southwards (Fig. 1). The intertidal macroalgae in the southern region are subject to desiccation, mostly during summer. The water column's physicochemical characteristics are highly influenced by the contrasting climatic seasons in the GC: the dry season (nominally from November to May) and the rainy season (from June to October). Annual precipitation (1080 mm yr−1) and evaporation (56 mm yr−1) rates registered during the past 40 years were 881±365 mm yr−1 and 53±7 mm yr−1, respectively (CNA, 2012).
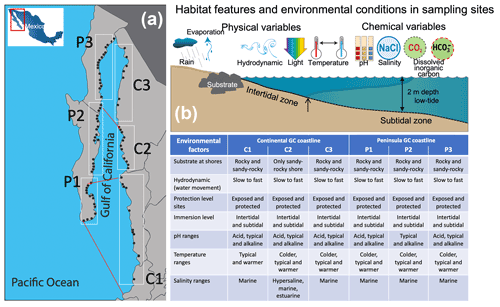
Figure 1Site collection along the continental (C1–C3) and peninsular (P1–P3) Gulf of California coastlines (a), range of environmental factors supporting or limiting the life processes for the macroalgal communities within a habitat (b), and inserted table with the features and environmental conditions in the diverse habitats in the GC bioregions that delimit the macroalgal community's zonation.
In the GC around 669 macroalgae species exist, including 116 endemic species (Espinoza-Avalos, 1993; Norris, 1975; Pedroche and Sentíes, 2003). Many endemic species currently have a wide distribution along the Pacific Ocean coast but with GC origin (Aguilar-Rosas et al., 2014; Dreckman, 2002). Based on oceanographic characteristics (Roden and Groves, 1959) and in the endemic species distribution (Aguilar-Rosas and Aguilar-Rosas, 1993; Avalos, 1993), the GC can be classified into three phycofloristic zones: (1) the first zone located from the imaginary line connecting San Francisquito Bay, B.C. (Baja California), to Guaymas, Sonora, with 51 endemic species; (2) the second zone with an imaginary line from La Paz Bay (B.C.S.; Baja California Sur) to Topolobampo (Sinaloa) with 41 endemic species; (3) the third zone is located with an imaginary line from Cabo San Lucas (B.C.S.) to Cabo Corrientes (Jalisco) with 10 endemic species. Besides, 14 endemic species are distributed throughout the GC (Espinoza-Ávalos, 1993). The macroalgal communities are subject to the changing environmental conditions in the diverse habitats in the GC that delimit their zonation, which tolerates a series of anatomical and physiological adaptations to water movement, temperature, sun exposure, light intensities, low pCO2, and desiccation (Espinoza-Avalos, 1993).
2.2 Macroalgae sampling
In this study, the GC coastline (21–30∘ N latitude) was divided into six coastal sectors based on the three phycofloristic zones along peninsular and continental GC coastlines (Fig. 1a). In each coastal sector, selected ecosystems and representative habitats were sampled based on macroalgae communities' presence and habitat characterization. Habitats were classified by substrate type (e.g., sandy-rock, rocky shore), hydrodynamic (slow to faster water flows), protection level (exposed or protected sites), and immersion level (intertidal or subtidal) (Fig. 1b).
Based on the local environmental factors, four to five macroalgae specimens of the most representative species were gathered by hand (free diving) during low tide. A total of 809 composite samples were collected from marine habitats along both GC coastlines. The percentages of specimens collected for the substrate type were 28 % sandy-rock and 72 % rocky shores based on the habitat features. In the hydrodynamic, 30 % of the specimens were collected in habitats with slow to moderate and 70 % with moderate to fast water movement. Regarding the protection level, 57 % were exposed specimens, and 43 % were protected. Finally, 56 % were intertidal and 44 % subtidal macroalgae organisms concerning the emersion level. About half of the protected specimens were collected in isolated rock pools, which was noted.
In four to five sites of each habitat, we measured in situ the salinity, temperature, and pH by using a calibrated multiparameter sonde (Y.S.I. 6600V) and the habitat characteristics mentioned above noted. Besides, composite water samples were collected for a complementary analysis of nutrients, alkalinity (and their chemical components), and δ13C DIC (data not included). Briefly, the representative habitats were classified by pH levels of >9.0 “alkalinized”, 7.9–8.2 “typical”, and <7.9 “acidified”. Based on colder (<20 ∘C), typical (20–25 ∘C), and warmer (>25 ∘C) temperatures, 72 % of the specimens were collected at typical, 22 % at alkalinized, and 6 % at acidified pH values. Regarding the temperature, about 55 % of the specimens were collected at typical, 31 % at warmer, and 14 % at colder seawaters. Regarding salinity, most of the ecosystems showed typical values for seawater (35.4 ± 0.91 PSU, from 34.5 to 36.1 PSU). In this study, the collection surveys were conducted during spring (March–April) and dry season (nominally from November to May) from 2008 to 2014. Only in a few selected ecosystems located at C1, C2, and C3 sectors was one sampling survey conducted at the end of the rainy season (nominally from June to October in 2014). Thus, these ecosystems were possible to include habitat with a salinity range varying from estuarine (23.5 ± 3.0 PSU) to hypersaline (42.7 ± 7.0 PSU) values. These habitats were mainly isolated rock pools, and only a few were sites near tidal channels receiving freshwater discharges. About 95 % of the specimens were collected at typical seawater salinity (34–36 PSU) and only 1.5 % and 3.5 % in estuarine (<30 PSU) and hypersaline (>37 PSU) environments, respectively. Detailed information on the selected shallow marine ecosystems, habitat characterization, and environmental conditions is summarized in the inserted table in Fig. 1.
2.3 Macroalgae processing and analysis of the isotopic composition of carbon
The collected material was washed in situ with surface seawater to remove the visible epiphytic organisms, sediments, sand, and debris and then thoroughly rinsed with Milli-Q water. The composite samples were double-packed in a plastic bag, labeled with the locality's name and collection date, placed in an ice cooler to be kept to 4 ∘C, and immediately transported to the laboratory UAS-Facimar in Mazatlán. In the field, sample aliquots were also preserved in 4 % formaldehyde solution for taxonomic identification to the genus or species level (when possible). The following GC macroalgal flora identification manuals were consulted (Abbot and Hollenberg, 1976; Dawson, 1944, 1954, 1956, 1961, 1962, 1963; Norris, 2010; Ochoa-Izaguirre et al., 2007; Setchell and Gardner, 1920, 1924).
In the laboratory, macroalgae samples were immediately frozen at −30 ∘C until analysis. Then, samples were freeze-dried at −38 ∘C and 40 mm Hg for 3 d, upon which they were ground to a fine powder and exposed to HCl vapor for 4 h (acid-fuming) to remove carbonates and dried at 60 ∘C for 6 h (Harris et al., 2001). Aliquots of ∼ 5 mg were encapsulated in tin cups (5 × 9 mm) and stored in sample trays until analysis. Macroalgae samples were sent to the Stable Isotope Facility (SIF) at the University of California at Davis, CA, USA. Natural 13C relative abundance relative to 12C in samples was determined with mass spectrometry, using a Carlo Erba elemental analyzer attached to a Finnigan Delta S mass spectrometer equipped with a Europa Scientific stable isotope analyzer (ANCA-NT 20-20) and a liquid–solid preparation unit (PDZ, Europa, Crewz, UK). Isotope ratios of the samples were calculated using the equation δ (‰) = , where R = . The Rstandard is relative to the international V-PDB (Vienna PeeDee Belemnite) standard. During the isotopic analysis, the SIF lab used different certified reference materials (e.g., IAEA-600, USGS-40, USGS-41, USGS-42, USGS-43, USGS-61, USGS-64, and USGS-65) for the analytical control quality. The analytical uncertainties reported for the SIF lab were 0.2 ‰ for δ13C (https://stableisotopefacility.ucdavis.edu/carbon-and-nitrogen-solids, last access: 18 January 2021). We also included triplicate aliquots of several specimens of the same species and condition, collected from one patch or attached to the same substrate, to assess the method error by sampling and processing procedures. The methodological uncertainties were <0.4 ‰.
2.4 Analysis of δ13C macroalgal variability
The variability in δ13C values in macroalgae was analyzed as a function of the taxonomy (phylum, genus, and species) and morphofunctional groups (e.g., thallus structure, growth form, branching pattern, and taxonomic affinities; Balata et al., 2011; Ochoa-Izaguirre and Soto-Jiménez, 2015). The carbon fixation strategies in the macroalgae communities of the GC were identified by δ13C (Díaz-Pulido et al., 2016; Hepburn et al., 2011) in agreement with the Maberly et al. (1992) and Raven et al. (2002a) thresholds. So, macroalgae were classified into three strategies for DIC uptake: (1) CCM only by active uptake HCO (δ13C > −10 ‰), (2) CCM active uptake HCO and diffusive uptake CO2 (δ13C < −11 to −30 ‰), and (3) non-CCM CO2 by diffusion only (δ13C < −30 ‰). The measured δ13C macroalgal signals are integrative of the discrimination by photosynthesis (Δ13Cp) on the carbon source (δ13C DIC in seawater), respiration (Δ13Cr), and probable CO2 leak out inside the cell during the CCM process (Carvalho et al., 2009a, b; Raven et al., 2005; Sharkey and Berry, 1985).
Macroalgae were grouped according to their morphofunctional characteristics proposed initially by Littler and Littler (1980) and modified by Balata et al. (2011). Most of the macroalgae species showed a limited distribution along the GC coastlines. Few cosmopolites' species included Colpomenia tuberculata, Sargassum sinicola, Padina durvillei, and Ulva lactuca. Also, not all morphofunctional groups and taxa were present in every site during each sampling survey, and the sample size in each group varied for taxa, location, and time.
A basic statistical analysis of δ13C values in different macroalgae groups was applied to distribute and calculate the arithmetic mean, standard deviation, and minimum and maximum. Because not all macroalgal species were present in sufficient numbers at different collection habitats, several macroalgal groups were not considered for statistical analysis. We compared taxa and morphofunctional groups collected in the same habitat (within-subjects factor) by multivariate analysis of variance. When differences were noted, a Tukey–Kramer HSD (honestly significant difference) test was performed. Besides, variations in δ13C macroalgae in specimens of the same morphofunction and taxon collected in different habitats were also investigated with a Kruskal–Wallis test.
The relationships between δ13C with the inherent macroalgae properties (taxon and morphology), biogeographical collection zone (GC coastline and coastal sector), habitat features (substrate, hydrodynamic, protection, and emersion level), and environmental conditions (temperature, pH, and salinity) were examined through simple and multiple linear regression analyses. Excepting temperature, pH, and salinity, most of the independent variables are categorical independent variables. Simple linear regression analyses were performed to establish the relationships between δ13C macroalgae with each environmental parameter analyzed as possible driving factors (e.g., temperature, salinity, and pH). Multiple linear regression analyses were conducted to evaluate the combined effects of those independent variables (macroalgae properties, biogeographical collection zone, habitat features, and environmental conditions) on the δ13C macroalgae. In the multivariable regression model, the dependent variable, δ13C macroalgal values, is described as a linear function of the independent variables Xi, as follows:
where a is regression constant (it is the value of intercept, and its value is zero), b1, b2, and bn are regression coefficients for each independent variable Xi. From each one of the fitted regression models, we extracted the estimated regression coefficients for each of the predictor variables: e.g., Bayesian information criterion (BIC), Akaike information criterion (AIC), root-mean-square error (RMSE), Mallow's Cp criterion, F ratio test, the p value for the test (prob > F), coefficients of determination (R2), and the adjusted R2 statistics (Stroup et al., 2018). All regression coefficients were used as indicators of the quality of the regression (Burnham and Anderson, 2002; Draper and Smith, 1998). The Kolmogorov–Smirnov normality test was applied for all variables, and all were normally distributed. Most of the δ13C values in each group showed a normal distribution. For all statistical tests, a probability P<0.05 was used to determine statistical significance. The statistical analysis of the results was using JMP 14.0 software (SAS Institute Inc.).
3.1 Taxonomy and morphofunctional groups
Sampled specimens belong to 3 phyla, 63 genera, and 170 species. The phyla were identified as Chlorophyta (25 %), Ochrophyta (22 %), and Rhodophyta (53 %). The most representative genera (and their species) were Ulva (U. lactuca, U. lobata, U. flexuosa, and U. intestinalis), Codium (C. amplivesiculatum and C. simulans), Chaetomorpha (C. antennina), Padina (P. durvillei), Dictyota (D. dichotoma), Colpomenia (C. tuberculata and C. sinuosa), Sargassum (S. sinicola and S. horridum), Amphiroa (Amphiroa spp.), Spyridia spp., Polysiphonia spp., Gymnogongrus spp., Gracilaria (G. vermiculophylla, G. pacifica, and G. crispata), Hypnea (H. pannosa and H. johnstonii), Grateloupia (G. filicina and G. versicolor), and Laurencia (L. papillosa and L. pacifica). The endemic species included Chlorophyta Codium amplivesiculatum, Rhodophyta Laurencia papillosa, Chondracanthus squarrulosa, Gracilaria spinigera, and G. subsecundata, and Ochrophyta Cutleria hancockii, Sargassum herphorizum, and S. johnstonii.
An analysis of the biogeographical diversity among sectors evidenced that P3 (43 genera of 63, 68 %) and C3 (63 %) in the north recorded the highest number of the genus, followed by C1 (38 %) and P1 (29 %) in the south, and P2 (27 %) and C2 (22 %). The same pattern was observed in the species diversity: zones P3 (94 of 167 species, 56 %) and C3 (52 %) in the north, C1 (34 %) and P1 (25 %) in the south, and C2 and P2 (19 %–20 %) in the center.
The morphofunctional groups identified were 21. The most common were C-Tubular (6 species, n=69); C-Blade-like (6 species, n=55); C-Filamentous uniseriate (17 species, n=49); C-Erect thallus (5 species, n=33); O-Compressed with branched or divided thallus (19 species, n=92); O-Thick leathery macrophytes (12 species, n=104); O-Hollow with spherical or subspherical shape (4 species, n=87); R-Larger-sized corticated (57 species, n=225); R-Filamentous uniseriate and pluriseriate with erect thallus (9 species, n=48); and R-Larger-sized articulated corallines (6 species, n=17). The diversity, in terms of presence/absence of the morphofunctional groups, varied among coastline sectors and was higher in C3 (16 of 21, 76 %) and P3 (71 %) in the north, followed by C1 (57 %) and P1 (48 %) in the south, and C2 and P2 and (42 %–48 %) in the center of both GC coastlines.
3.2 The δ13C macroalgal variability as a function of taxonomic and morphofunctional groups
The variability in δ13C values in macroalgae was analyzed by taxon (phylum, genus, species) and morphofunctional groups classified by habitat, coastal sector, and collection season. A complete list of the results of δ13C in 170 macroalgae species is provided in Supplement (Table S1). Firstly, δ13C values analyzed by phylum showed a unimodal distribution with a peak at ‰ (Fig. 2). Ochrophyta (−21.5 ‰ to −2.2 ‰, ‰), displayed significantly higher values than Chlorophyta (−25.9 ‰ to −5.5 ‰, ‰) and Rhodophyta (−34.6 ‰ to −4.5 ‰, ‰). The δ13C macroalgal values (average ± SD) for the genera of Chlorophyta, Ochrophyta, and Rhodophyta (Fig. 3) varied from ‰ for Schizymenia to ‰ for Amphiroa. Based on the highest values, specimens of three phyla showed δ13C values > −10 ‰, which evidenced the presence of CCMs by active uptake of HCO (strategy 1) (Fig. 3). For example, Caulerpa, Cladophora, Codium, and Ulva for Chlorophyta, Colpomenia, Dictyota, Padina, and Sargassum for Ochrophyta, and Hypnea and Polysiphonia for Rhodophyta showed δ13C values ‰. Likewise, high δ13C values were observed in the calcifying macroalgal genera Amphiroa and Jania under strategy 1 (Fig. 3c). The δ13C values lower than −30 ‰ that denote uptake of CO2 by diffusion (strategy 3) were observed only in Rhodophyta Schizymenia, Halymenia, and Gigartina. However, most species showed large δ13C variabilities, which is evidence of a mechanism that uses a mix of HCO and CO2 for photosynthesis (strategy 2).
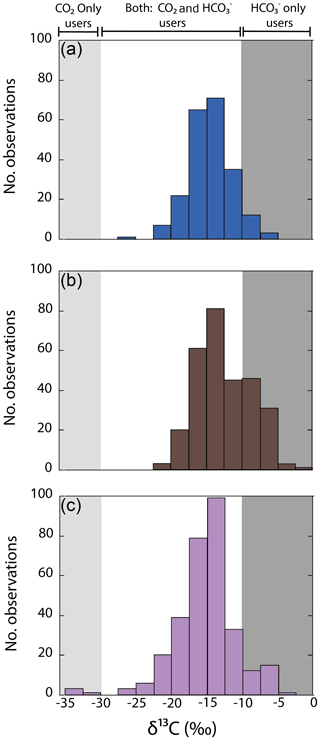
Figure 2Variability in δ13C values for specimens of different macroalgae species collected along GC coastlines classified by taxon: (a) Chlorophyta, (b) Ochrophyta, and (c) Rhodophyta. Shaded background represents the cutoff limits for using CO2 only users and HCO only users, according to Raven et al. (2002a).
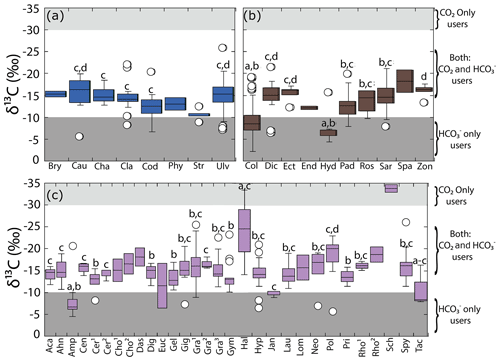
Figure 3Variability in δ13C values for the genera collected along the coastline of the Gulf of California according to their taxon: (a) Chlorophyta, (b) Ochrophyta, and (c) Rhodophyta. Genera with n=1 are not shown, and genera with n=2 were not considered in the statistical comparison. Different letters indicate significant differences (P<0.05): . Shaded background represents the cutoff limits for using CO2 only users and HCO only users, according to Raven et al. (2002a). For Chlorophyta: Bry = Bryopsis, Cau = Caulerpa, Cha = Chaetomorpha, Cla = Cladophora, Cod = Codium, Phy = Phyllodictyon, Str = Struveopsis, Ulv = Ulva. Ochrophyta: Col = Colpomenia, Dic = Dictyota, Ect = Ectocarpus, End = Endarachne, Hyd = Hydroclathrus, Pad = Padina, Ros = Rosenvingea, Sar = Sargassum, Spa = Spatoglossum, Zon = Zonaria. Rhodophyta: Aca = Acantophora, Ahn = Ahnfeltiopsis, Amp = Amphiroa, Cen = Centroceras, Cer1 = Ceramium, Cer2 = Ceratodictyon, Cho1 = Chondracanthus, Cho2 = Chondria, Das = Dasya, Dig = Digenia, Euc = Eucheuma, Gel = Gelidium, Gig = Gigartina, Gra1= Gracilaria, Gra2= Grateloupia, Gra3 = Gracilariopsis, Gym = Gymnogongrus, Hal = Halymenia, Hyp = Hypnea, Jan = Jania, Lau = Laurencia, Lom = Lomentaria, Neo = Neosiphonia, Pol = Polysiphonia, Pri = Prionitis, Rho1 = Rhodoglossum, Rho2 = Rhodymenia, Sch = Schizymenia, Spy = Spyridia, Tac = Tacanoosca.
Multiple comparison analyses revealed significant differences in the δ13C macroalgal values among genera, ordered as Schizymenia < Polysiphonia < Ulva, Gracilaria and Spyridia ( ‰ to ‰) < Gymnogongrus, Laurencia, Hypnea, Cladophora, Dictyota, Sargasum, Chaetomorpha, and Grateloupia (from ‰ to ‰) < Codium and Padina ( ‰ to ‰) < Colpomenia and Amphiroa ( ‰ to ‰) (F=16.81, p<0.001).
Aggrupation of δ13C values based on morphofunctional features is displayed in Fig. 4. The most representative groups in the phylum Chlorophyta varied from ‰ for C-Tubular to ‰ for C-Thallus erect. The phylum Ochrophyta includes O-Thick leathery with the lowest mean ( ‰) and O-Hollow with a spherical or subspherical shape with the highest values ( ‰). The lowest and highest δ13C values for Rhodophyta were observed for R-flattened macrophytes ( ‰) and R-Larger-sized articulated coralline ( ‰), respectively. Significant differences were observed among groups, which were ordered as follows: R-Flattened macrophytes < R-Blade-like < C-Tubular < O-Tick leathery and R-Larger-sized corticated < C-Blade-like and C-Filamentous uniseriate < C-Thallus erect and O-Compressed with branch < O-Hollow with spherical < R-Larger-sized articulated coralline.
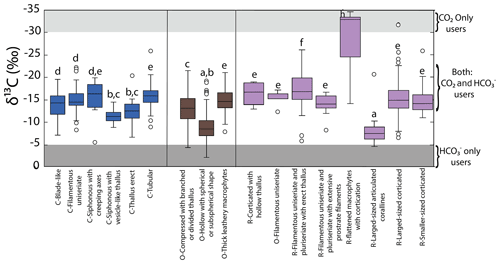
Figure 4Variability in δ13C values for morphofunctional groups by taxa along the coastline of the Gulf of California.
High intraspecific variability in δ13C signal for the more representative genera of each taxon is showed in Tables 1–3. For Codium, C. brandegeei (11.8 ± 1.2 ‰) and C. simulans ( ‰) showed higher δ13C values than C. amplivesculatum ( ‰). Colpomenia species had higher δ13C values than the other genera, with higher values for C. tuberculata ( ‰) than Colpomenia sp. ( ‰) and C. sinuosa ( ‰). Gracilaria showed comparable δ13C values in the four species (from ‰ for G. pacifica to ‰ for Gracilaria sp.). Hypnea showed non-significant δ13C differences in three representative species ( ‰ for H. spinella to ‰ for Hypnea sp.). Laurencia sp. ( ‰) was higher than L. pacifica ( ‰), while Padina sp. ( ‰) higher than P. durvillei ( ‰). Sargassum was one of the most diverse genera studied with six representative species, with δ13C values ordered as follow: S. horridum = S. sinicola = S. johnstonii ( to ‰) < S. lapazeanum ( ‰) = Sargassum sp. ( ‰) < S. herphorizum ( ‰). Spyridia sp. ( ‰) and S. filamentosa ( ‰) showed non-significant differences. The six representative species of Ulva were divided into two morphological groups, filamentous and laminates. Filamentous species averaged ‰ for U. clathrata, ‰ for U. flexuosa, ‰ for U. acanthophora, and ‰ for U. intestinalis, and Ulva laminates included U. linza ( ‰) and U. lactuca ( ‰). Non-significant differences were observed between morphological groups and among species. A high intra-specific variability, 11 %–28 %, explains average overlapping.
Table 1Carbon isotopic composition (‰) in species of phylum Chlorophyta collected along the Gulf of California coastlines.
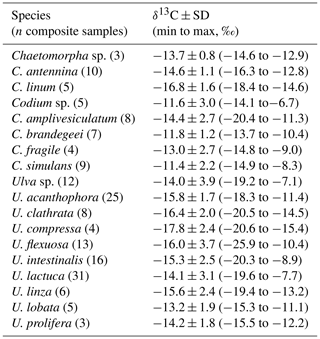
Table 2Carbon isotopic composition (‰) in species of phylum Ochrophyta collected along the Gulf of California coastlines.
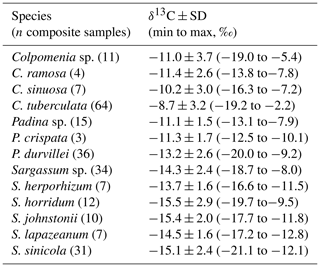
3.3 δ13C macroalgal variability in coastal sectors
A diversity of macroalgal assemblages were documented along the GC coastlines, with differences in the taxonomic composition according to their fico-floristic region. Multiple comparison analyses of δ13C signals evidenced significant differences between the most common genera and species of macroalgae between and within assemblages grouped by coastal sector, season, and collecting year (Tables S2–S3). For example, genera Padina (e.g., P. durvillei) and Ulva (e.g., U. lactuca), collected in C1 sector during the rainy season, showed lower δ13C values than in other sectors. Differences in the δ13C signal are mainly related to the carbon uptake strategies of the macroalgae (Fig. 5). Even though most species inhabiting the GC coastal sectors dominated strategies based on active CCMs, the tendencies differed between taxa and coastal regions. Strategy 2 with mixing DIC sources is dominant in all regions and taxa (60 %–90 %). Exceptions were observed in the P1 (68 %) and C1 (37 %) regions for Ochrophyta, in which the specialized strategy 1 (the HCO user) was significant. Strategy 3 based on the use of CO2 was observed in the peninsular coast in P2 and P3 for Rhodophyta with 2 %–3.3 %. Overall, more negative δ13C values were observed at continental (C2) compared to the peninsular coastline (P1–P3) and southward than northward.
3.4 The δ13C macroalgal variability as a function of taxonomy, habitat features, and environmental conditions
Variability in δ13C values for the most representative genera was evaluated by multiple comparative analyses as a function of habitat features, including the substrate, hydrodynamic, and emersion level. Large δ13C variability observed between specimens of the same genus collected in the different habits do not show any significant pattern, and non-significant differences were observed. An exception was observed with the emersion level (shown in Fig. 6), in which intertidal specimens recorded less negative values than subtidal in most macroalgae genera, for example, for Hydroclathrus (intertidal ‰, subtidal ‰), Amphiroa (intertidal , subtidal ‰), Hypnea (intertidal ‰, subtidal ‰), and Laurencia (intertidal ‰, subtidal ‰). Exceptions were observed for Polysiphonia (intertidal ‰, subtidal ‰), Spyridia (intertidal ‰, subtidal ‰), and Colpomenia (intertidal ‰, subtidal ‰).
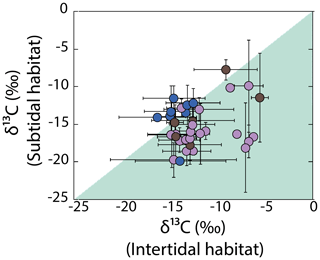
Figure 6Variability in δ13C values in macroalgae specimens for the most representative genera as a function of habitat features (emersion level). Blue circles represent the genus Chlorophyta, brown circles represent the genus Ochrophyta, and purple circles represent the genus Rhodophyta.
Non-significant differences were observed for the same genera at different temperature ranges except for Grateloupia (cold, ‰, typical ‰, warm ‰) and Polysiphonia (cold, ‰, typical ‰, warm ‰) with more negative values in colder than warmer waters (F=6.42, p<0.001). Neither significant difference was observed in δ13C values in macroalgae specimens from the different genera in the same temperature range (Fig. 7a).
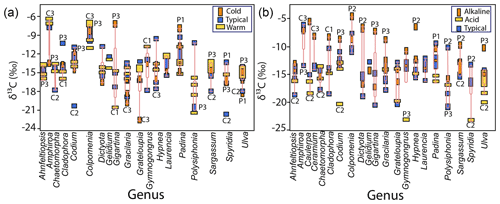
Figure 7Variability in δ13C values in macroalgae specimens for the most representative genera as a function of temperature (a) and pH (b) ranges in samples collected along the Gulf of California coastline.
Significant differences were observed among the genera related to the pH level in seawater (Fig. 7b). Under typical pH seawater, Amphiroa and Colpomenia were 1 ‰–2 ‰ more negatives than in alkaline waters, while Ulva and Spyridia were 3 ‰–5 ‰ less negative than in acidic waters. Amphiroa and Colpomenia were not collected in acidic water, and neither was Spyridia in alkaline waters to compare. Another genus also showed extremes values between alkaline (Tacanoosca ‰) and acidic waters (Schizymenia ‰). The following order was observed in the genera collected at the three pH ranges: alkaline > typical > acidic. Significant differences were observed for genera Ahnfeltiopsis, Caulerpa, Gymnogongrus, Padina, and Ulva, with higher values in alkaline than in acidic waters. Values of δ13C for specimens of the same genus collected in typical pH waters are mostly overlapped between alkaline and acidic seawaters. Non-significant differences in δ13C values were observed for Grateloupia, Hypnea, and Polysiphonia concerning pH-type waters.
We analyzed the carbon uptake strategies on macroalgal assemblages as a function of environmental factors like temperature, pH, and salinity (Fig. 8). The temperature and salinity non-significantly explained the δ13C macroalgal variability. A poor but significant correlation was observed between δ13C and pH (R2=0.04) (Table 4). The proportion of specimens with a strategy of only HCO use was different between environmental factors and taxa (previously described). For example, Ochrophyta showed the highest proportion (35 %) in colder temperatures, in pH alkaline (31 %), and in a typical salinity regimen (27 %). Chlorophyta was enhanced to 30 % in acid pH, and Rhodophyta recorded 21 % in normal seawater. The opposite strategy (only use of dissolved CO2) was observed only in Rhodophyta. The highest percentage was observed in the estuarine salinity regimen (10 %).
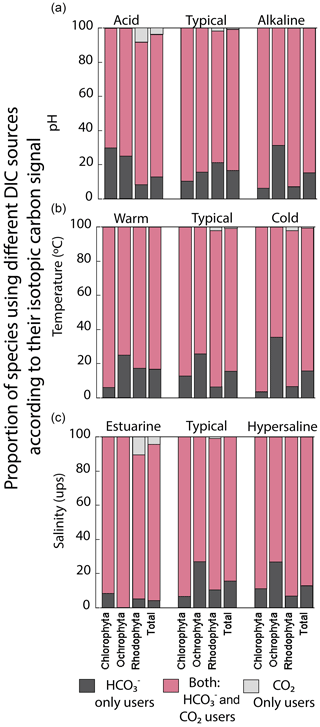
Figure 8Proportion of species using different DIC sources according to their carbon assimilation strategies: HCO only users (CO2 concentrating mechanism active), users of both sources (HCO and CO2), and CO2 only users (non-CO2 concentrating mechanism active) as a function of (a) pH ranges, (b) temperature ranges, and (c) salinity ranges.
Table 4Summary of the estimated regression coefficients for each simple linear regression analysis and of the constant of fitted regression models. Estimated regression coefficients include degree of freedom for the error (DFE), root-mean-square error (RMSE), coefficient of determination (R2) and the adjusted R2 statistics, Mallow's Cp criterion (Cp), Akaike information criterion (AIC), Bayesian information criterion (BIC) minimum, F ratio test, and p value for the test (prob > F). Model information includes value of the constant a (δ13C, ‰), standard error (SE), t ratio, and prob > (values * are significant).
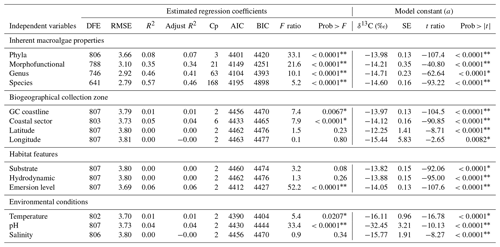
* p<0.05, p<0.0001.
3.5 Variation latitudinal of δ13C macroalgae
The δ13C macroalgal variation in the GC biogeography was evaluated by linear regression analysis between δ13C values along the 9 ∘ latitude of both GC coastlines. A non-significant latitudinal trend was observed for datasets, but for the three phyla's most representative genera, δ13C values correlated with latitude (Fig. 9). In Chlorophyta, with the higher genera number, δ13C values increased with latitude, with low but significant correlation. Contrarily, in Ochrophyta and Rhodophyta specimens, the δ13C values decreased non-significantly with latitude.
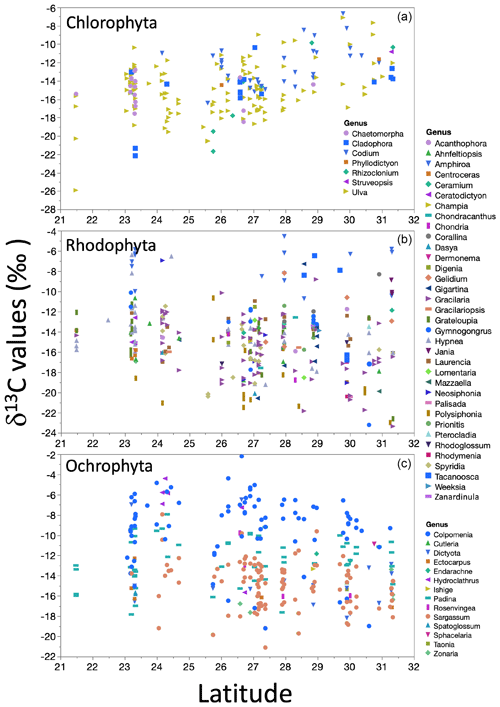
Figure 9Trends in the δ13C macroalgae in specimens for genera by taxa along the coastline of the Gulf of California as a function of latitudinal gradient.
In the most representative morphofunctional groups, significant correlations (p<0.001) were observed for δ13C macroalgae versus latitude (Fig. 10). Representative morphofunctional groups of Chlorophyta (e.g., C-Tubular, C-Filamentous uniseriate) showed a positive correlation, while those belonging to Ochrophyta (e.g., O-Thick leathery) and Rhodophyta (e.g., R-Larger-sized corticated) showed a negative trend with latitude.
3.6 Analyses of δ13C macroalgal variability
The δ13C macroalgal variability was analyzed as a function of the life-form and environmental factors. Firstly, simple linear regression analyses were performed to evaluate the dependent variable's prediction power (δ13C macroalgal variable) as a function of several independent variables controlling the main macroalgae photosynthesis drivers (light, DIC, and inorganic nutrients). Regression coefficients were estimated for each fitted regression model, which are used as indicators of the quality of the regression (Burnham and Anderson, 2002; Draper and Smith, 1998) as was described in Methods; however, the description of our results focused on the coefficients of determination (R2 and adjusted R2). The coefficient R2 describes the relationship between the independent variables Xi with the dependent variable Y (δ13C macroalgal values). R2 is interpreted as the percent of contribution to the δ13C variability. In comparison, the adjusted R2 statistics compensate for possible confounding effects between variables.
Results of the analysis of the relationships between δ13C with each independent variable are summarized in Table 4. Phyla explain only 8 % variability regarding the inherent macroalgae properties, the morphofunctional properties 35 %, genera 46 %, and species 57 %.
The biogeographical collection zone, featured by coastline (continental versus peninsular) and coastal sectors (C1–C3 and P1–P3), explained a maximum of 5 % variability. Only the emersion level (6 %) contributed to the δ13C variability related to the habitat features. The contribution of the seawater's environmental conditions was marginal for pH (4 %) and negligible for temperature and salinity. A marginal reduction in the percentage of contribution was observed for phyla (1 %) and morphofunctional properties (1 %), but it was significant for genera (5 %) and species (10 %).
Multiple regression analyses were also performed to interpret the complex relationships among δ13C macroalgae, considering the life-forms (morphofunctional properties and taxa by genus) and their responses to environmental parameters. Results for the fitted regression models performed for morphofunctional groups (Table 5) and genera (Table 6) evidenced that the effect of the coastal sector and pH ranges on the δ13C macroalgae increased the contribution by 9 %–10 % for each one. The emersion level increased by 5 %–6 %, the contribution with respect to the individual effect of morphofunctional group and genus, and the temperature and pH by 1 % and 3 %, respectively, while salinity decreased by 1 %–2 %. The combined effect of the biogeographical collection zone (e.g., coastline sector) and morphofunctional group (Table 5) and genus (Table 7) increased in 11 %–12 %.
Table 5Summary of the estimated regression coefficients for each multivariate linear regression analysis and of their constant of fitted regression models performed in individuals binned by genus. Estimated regression coefficients include degree of freedom for the error (DFE), root-mean-square error (RMSE), coefficient of determination (R2) and the adjusted R2 statistics, Mallow's Cp criterion (Cp), Akaike information criterion (AIC), Bayesian information criterion (BIC) minimum, F ratio test, and p value for the test (prob > F). Model information includes value of the constant a (δ13C, ‰), standard error (SE), t ratio, and prob > (values * are significant).
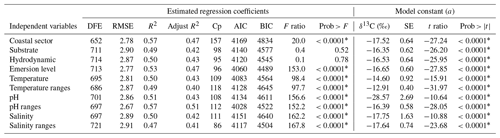
Table 6Summary of the estimated regression coefficients for each multivariate linear regression analysis and of their constant of fitted regression models performed in individuals binned by coastline sector and genus. Estimated regression coefficients include degree of freedom for the error (DFE), root-mean-square error (RMSE), coefficient of determination (R2) and the adjusted R2 statistics, Mallow's Cp criterion (Cp), Akaike information criterion (AIC), Bayesian information criterion (BIC) minimum, F ratio test, and p value for the test (prob > F). Model information includes value of the constant a (δ13C, ‰), standard error (SE), t ratio, and prob > (values * are significant).
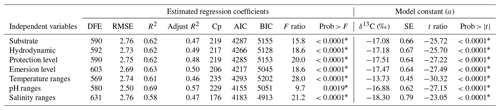
Table 7Summary of the estimated regression coefficients for each multivariate linear regression analysis and of their constant of fitted regression models performed in individuals binned in coastline sector, habitat features, environmental conditions, and physiological state separately by morphofunctional group and genus. Estimated regression coefficients include degree of freedom for the error (DFE), root-mean-square error (RMSE), coefficient of determination (R2) and the adjusted R2 statistics, Mallow's Cp criterion (Cp), Akaike information criterion (AIC), Bayesian information criterion (BIC) minimum, F ratio test, and p value for the test (prob > F). Model information includes value of the constant a (δ13C, ‰), standard error (SE), t ratio, and prob > (values * are significant).
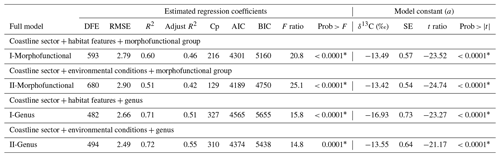
Considering the combined effect of the coastline sector + habitat features for morphofunctional group or genus (Table 7), the full model showed R2 values of 0.60 and 0.71. In contrast, coastline sector + environmental conditions + morphofunctional group or genus the R2 increased to 0.62 and 0.72, respectively. The interactive explanations of environmental factors increased the explanation percentage of δ13C variability; however, these contributions were significantly lower than those explained by life-forms, such as the morphofunctional properties and taxa by genus and species.
The combined effect of environmental conditions on the δ13C variability was tested for the best-represented genera and morphological groups. Results evidenced that 9 of 21 morphological groups showed significant effects on the δ13C variability (Table 8), five increasing and four decreasing the model constant of δ13C = −14.2 ‰. For example, for the O-Hollow with spherical or subspherical shape (+4.9 ‰) and R-Larger-sized articulated corallines (+6.3 ‰), the predicted values are ‰ and ‰. For R-Filamentous uniseriate and pluriseriate with erect thallus (−2.1 ‰) and C-Tubular (−1.6 ‰), the predicted values are ‰ and ‰, respectively. Regarding taxon, a significant effect was observed only in 13 genera, including Colpomenia (+5.4 ‰), Amphiroa (+6.8 ‰), and Padina (+2.2 ‰) increasing the signal and Polysiphonia (−3.7 ‰), Gracilaria (−0.9 ‰), and Spyridia (−1.4 ‰) decreasing the signal of the model constant (Table 9). In 33 species a significant effect on the δ13C variability was observed, including C. tuberculate (+5.9 ‰), C. sinuosa (+4.4 ‰), H. pannosa (+4.4 ‰), H. johnstonii (+4.4 ‰), and Amphiroa spp. (+4.4 ‰ to 8.2 ‰) increasing the model constant δ13C = −14.6 ‰, and Spyridia sp. (−2.5 ‰), G. filicina (−2.3 ‰), P. mollis (−5.2 ‰), and S. pacifica (−19.2 ‰) decreasing the model constant (Table 10).
Table 8Constant of fitted regression model explaining the δ13C variability by morphofunctional groups. Model information includes value of the constant a (δ13C, ‰), standard error (SE), t ratio, and prob > . Only morphofunctional groups with significant effects are listed.
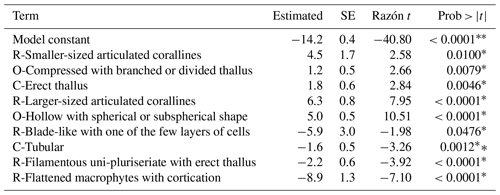
* p<0.05, p<0.0001.
Table 9Constant of fitted regression model explaining the δ13C variability by genus. Model information includes value of the constant a (δ13C, ‰), standard error (SE), t ratio and prob > . Only genera with significant effects are enlisted.
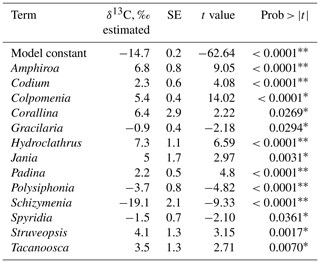
* p<0.05, p<0.001.
3.7 Preliminary estimations of Δ13C macroalgae
Concurrent analysis of surface seawater for alkalinity, proportions of the chemical species of DIC (CO2, HCO, and CO, and δ13C DIC evidenced that δ13C DIC in GC seawater averages 1.4±0.4 ‰ (−1 ‰ to 4.9 ‰) (Fig. S1). In our preliminary data, the δ13C-DIC seawater slightly (in 0.5 ‰) decreased during the rainy season in those zones influenced by river discharges along the continental coastline. Non-significant differences were observed among coastal sectors. The δ13C-DIC values in GC seawater are comparable to the averages 1.4 ‰–1.6 ‰ reported for the surface seawaters in the eastern North Pacific in the 1970s–2000s (Hinger et al., 2010; Quay et al., 2003; Santos et al., 2011).
Based on the subtraction of δ13C macroalgae to δ13C-DIC seawater, the integrative discrimination factor against 13C averaged 16.0±3.1 ‰, 16.8±4.3 ‰, and 14.0±3.8 ‰ for phyla Chlorophyta, Rhodophyta, and Ochrophyta, respectively. Five groups were identified as a function of the Δ13C values: one for Chlorophyta (Δ13C = 16.0±3.1 ‰), two for Rhodophyta (16.6±3.8 ‰ and 34.6±1 ‰), and two for Ochrophyta (9.1±1.7 ‰ and 15.7±2.7 ‰) (Fig. S2). Values of Δ13C were comparable to δ13C of the thallus of macroalgae. Thus, δ13C macroalgae reflect mainly the discrimination during carbon assimilation. Like δ13C macroalgae, the Δ13C values were subject to considerable variation.
4.1 Explaining the δ13C macroalgal variability
A high variability in the δ13C values was revealed in the large inventory of macroalgae collected along the GC coastline. A linear regression analysis of the effects of life-forms revealed that the δ13C variability in the macroalgal community is mainly explained by taxonomic (genus 46 %, species 57 %) and morphofunctional group (35 %). This result is consistent with the report of Lovelock et al. (2020), which found that 66 % of δ13C variability was explained by taxonomy. Even so, the variability associated with each genus is not the same and can be classified in three groups: (1) high variability (e.g., Schizymenia = ±19.1 ‰), moderate variability (e.g., Hydroclathrus = ±7.3 ‰; Amphiroa = ±6.8 ‰), and low variability (e.g., Gracilaria = ±0.89; Spyridia = ±1.46 ‰). The observed δ13C variability in this study is comparable with those reported in the literature, compiled in Table S4.
Most authors studying the isotopic composition of C in macroalgae have reported the high isotopic variability, which has been attributable to the taxon-specific photosynthetic DIC acquisition properties (Díaz-Pulido et al., 2016; Lovelock et al., 2020; Marconi et al., 2011; Mercado et al., 2009; Raven et al., 2002a; Stepien, 2015). Our study observed that the intrinsic characteristics of each morphofunctional group of macroalgae (e.g., thallus structure, growth form, branching pattern, and taxonomic affinities) also influence the δ13C macroalgal signals. The thallus thickness influences the diffusion boundary layer on the surface of the macroalgae, where they carry out the absorption of essential ions and dissolved gases (Hurd, 2000; Sanford and Crawford, 2000). Thus, morphology can modulate the photosynthesis rates. However, a non-biological or ecological explanation of the δ13C variability, and therefore carbon use physiology, can be given in terms of morphology.
The δ13C macroalgae depend on the carbon source (δ13C DIC in seawater), the isotope discrimination during carbon assimilation in the photosynthesis (Δ13Cp < 29 ‰ in a variable degree), and the plant respiration (Δ13Cr average ±2.3 ‰) (Carvalho et al., 2009a, b, 2010a; Carvalho and Eyre, 2011; Rautenberger et al., 2015). Comparatively, the Δ13Cr value is relatively small regarding Δ13Cp. Thus, δ13C macroalgal value is an integrative value of the isotope discrimination during DIC seawater assimilation (Δ13C = (δ13C-DIC seawater − δ13C macroalgae)) (Carvalho et al., 2009a). Based on the Δ13C values, five groups were identified in our study: one for Chlorophyta (Δ13C = 16.0 ± 3.1 ‰), two for Rhodophyta (16.6 ± 3.8 ‰ and 34.6 ± 1 ‰), and two for Ochrophyta (9.1 ± 1.7 ‰ and 15.7 ± 2.7 ‰). Values of Δ13C were comparable to δ13C of the thallus of macroalgae. The δ13C macroalgal values reflect the discrimination during carbon assimilation attributable to the taxon-specific photosynthetic DIC acquisition properties. Δ13C macroalgal variability, captured in the δ13C macroalgal signals, is related to the thickness of the boundary layer around the thallus (Raven et al., 1982), the leakage during carbon uptake (Maberly et al., 1992; Sharkey and Berry, 1985), photosynthetic intensity (Kübler and Raven, 1995, 1996; Wiencke and Fischer, 1990), and respiration rates (Carvalho et al., 2010a; Carvalho and Eyre, 2011; Rautenberger et al., 2015). All intrinsic properties are related to the life-form.
Many species that recorded high δ13C values (and low Δ13C values) were fleshy macroalgae that are characterized to be bloom-forming macroalgae belonging to genera Ulva, Gracilaria, Cladophora, Spyridia, and Sargassum (Páez-Osuna et al., 2013; Valiela et al., 2018). It is not surprising that species with high photosynthetic activity and high relative growth rates (Hiraoka et al., 2020) have high carbon demand that results in lower isotopic discrimination against 13C (Carvalho et al., 2010a, b; Cornelisen, et al., 2007; Kübler and Dungeon, 2015; Rautenberger et al., 2015). Bloom-forming macroalgae (e.g., Ulva, Gracilaria, Sargassum) have been remarked as facultative species capable of switching from C3 to C4 pathway (Valiela et al., 2018). C4 pathway reduces photorespiration, the antagonist process of RuBisCo, enhancing the DIC assimilation in 25 %–40 % and increasing the δ13C values (Bauwe et al., 2010; Ehleringer et al., 1991; Zabaleta et al., 2012). C4 pathway has more energy investment in CCMs than in RuBisCo protein content than C3 pathway (Young et al., 2016). Also, the reports of C4 or C4-like pathway features in algae have increased in the last years (Doubnerová and Ryšslavá, 2011; Roberts et al., 2007; Xu et al., 2012, 2013). For example, high activity of key enzymes of C4 metabolisms, such as pyruvate orthophosphate dikinase (PPDK), phosphoenolpyruvate carboxylase (PEPC), and phosphoenolpyruvate carboxykinase (PCK), has been described in many algae species. But the establishment of a true C4 pathway in marine algae is not clear since the massive changes in gene expression patterns seem to be incomplete, and it is suggested that many marine algae have high plasticity to use a combination of CCM to overcome DIC limitations (Doubnerová and Ryšlavá, 2011; Roberts et al., 2007; Xu et al., 2012, 2013). A stepwise model of the path from C3 to C4 photosynthesis is explained by Gowik and Westhoff (2011). More research is required on this topic considering the increasing frequency, intensity, and extension of bloom-forming macroalgae events worldwide (Teichberg et al., 2010; Valiela et al., 2018) and in México (Ochoa-Izaguirre et al., 2007; Ochoa-Izaguirre and Soto-Jiménez, 2015; Páez-Osuna et al., 2017).
Changes in the habitat features and environmental conditions, such as light intensity and DIC availability, influencing the growth rate and photosynthetic intensity, have a strong influence on δ13C signal (Carvalho et al., 2007, 2009a; Carvalho and Eyre, 2011; Mackey et al., 2015; Rautenberger et al., 2015; Stepien, 2015). The light intensity is the external factor with more influence on the Δ13C macroalgae due to the regulation of carbon assimilation intensity (Carvalho et al., 2009a, b; Cooper and DeNiro, 1989; Grice et al., 1996). Experimental studies found the light levels to be a critical factor affecting the δ13C values. For example, under saturating light conditions, Ulva switched from a carbon uptake of HCO and CO2 to increased HCO use (Rautenberger et al., 2015). Furthermore, field studies have shown that species growing in low-light habitats like deep subtidal zones tend to have more negative δ13C values than those in higher-light environments (Cornwall et al., 2015; Díaz-Pulido et al., 2016; Hepburn et al., 2011; Marconi et al., 2011; Mercado et al., 2009; Stepien, 2015). In this study, intertidal specimens recorded less negative values than subtidal in most macroalgae genera. However, our study did not record the vertical effect in the δ13C signal related to the light limitation because only shallow habitats (non-light limited) were studied.
The δ13C-DIC seawater is reasonably uniform in surface seawater (−4.8 ‰ to 3.6 ‰, median 1.5 ‰), with δ13C values for CO2, HCO, and CO nearly −10 ‰, −0.5 ‰, and 2 ‰, respectively (Kroopnick, 1985; Mook et al., 1974). Exceptions can be expected where variations in the salinity, alkalinity, and proportions of the chemical species of DIC (CO2, HCO, or CO occur (e.g., in coastal environments influenced by river and groundwater discharges) (Carvalho et al., 2015; Chanton and Lewis, 1999; Hinger et al., 2010; Mook et al., 1974). Regarding DIC sources for macroalgae in the GC surface seawater, the availability, chemical proportions, and δ13C DIC were also relatively constant and uniform. Thus, the influence of the δ13C-DIC variations on the δ13C macroalgal variability is negligible in the GC.
The effect of other environmental factors, such as salinity and pH, on δ13C macroalgal signals was evaluated. Regarding salinity, the influence of freshwater discharge by rivers and groundwater decreases the δ13C signal, which could be explained by the reduction in the salinity regimen that follows a decrease in δ13C DIC in water (Hinger et al., 2010; Santos et al., 2011). In our study, a non-significant correlation between δ13C macroalgae and salinity was observed.
Based on pH, differences in δ13C were found only for a few genera (e.g., Amphiroa, Colpomenia, Ulva, Spyridia), with an increasing trend in the δ13C values with pH increase, such as was reported by Maberly et al. (1992) and Raven et al. (2002b). Similar results were reported for Cornwall et al. (2017) in the field study, with the differential response of the δ13C signals to pH among 19 species, in which only four species were sensitive to pH changes. A very weak but significant positive linear regression was observed between δ13C and pH. Also, a decreasing trend in the δ13C was recorded in the following order: alkaline > typical > acidic. According to Stepien (2015), the result of meta-analyses between pH drift experiments and δ13C thresholds was positive only for Rhodophyta and Ochrophyta but not for Chlorophyta. About 86% of the Stepien metadata met the theoretical CCM assignation based on both parameters, with exceptions for species with δ13C < −30 ‰ that have been capable of raising pH to > 9. A strong association between pH compensation point and δ13C was reported by Iñiguez et al. (2019) in three taxa of polar macroalgae. Environmental conditions may influence the δ13C macroalgal values but not change the carbon use physiology in the macroalgae, which is most likely inherently species-specific.
4.2 Using δ13C macroalgae to indicate the presence of an active CCM
In our study, the δ13C macroalgal signals were used to evidence the presence of an active CCM. This tool was first used in macroalgal shallow communities of the GC. Most macroalgae species displayed δ13C values that exhibit active CCMs. Then, macroalgae were classified into three strategies for DIC uptake, in agreement with the Maberly et al. (1992) and Raven et al. (2002a) thresholds: (1) CCM-only by active uptake HCO (δ13C > −10 ‰), (2) CCM active uptake HCO and diffusive uptake CO2 (δ13C < −11 ‰ to −30 ‰), and (3) non-CCM CO2 by diffusion only (δ13C < −30 ‰). About 84 % of the analyzed specimens showed the facultative uptake of HCO and CO2, the most common strategy identified in macroalgal shallow communities (Cornwall et al., 2015; Díaz-Pulido et al., 2016; Hepburn et al., 2011; Stepien, 2015). Based on the carbon uptake strategies, the most abundant macroalgae were those able to use both HCO and CO2 using active uptake plus passive diffusion (strategy 2).
Macroalgae collected in GC also involved only HCO users (strategy 1) and those relying on diffusive CO2 uptake (strategy 3). Photosynthesis that relies on CO2 uptake (lack of CCM), the most primitive mechanism (Cerling et al., 1993), has fewer energy costs than HCO uptake, which requires complex machinery with a high operational cost (Giordano et al., 2005; Hopkinson et al., 2011, 2014; Raven and Beardall, 2016). The energy for macroalgae to uptake HCO, cross the plasma membrane, and convert to CO2 for photosynthesis is obtained through irradiance (Cornelisen et al., 2007). Based on our sampling effort, focused on intertidal and shallow subtidal habitats featured by high light intensities, we expected high proportions of species with the carbon uptake strategy that use only HCO. Results evidenced that strategy 1 was recorded in specimens belonging to 58 species of 170 total species. The higher proportions of CCM species (HCO users) with high energetic requirements are explained by those elevated irradiances (Cornwall et al., 2015; Hepburn et al., 2011). Ochrophyta showed the highest proportion of species that depend only on HCO uptake on both coastlines in the southern region of GC (P1, C1). The low solubility of CO2 is related to high temperatures in subtropical waters (Zeebe and Wolf-Gladrow, 2001) that impulse the development of CCM (Raven et al., 2002b) and by the high affinity to DIC by Ochrophyta, such as has been described before by Díaz-Pulido et al. (2016).
Only three non-calcifying species (Schizymenia pacifica, Halymenia sp., Gigartina sp.) belonging to Rhodophyta were CO2-exclusive users (δ13C = ‰). Based on measurements of pH drift, Murru and Sandgren (2004) reported Schizymenia pacifica and two species of Halymenia (e.g., H. schizymenioides and H. gardner) as restricted CO2 users. Measurements of δ13C in Halymenia dilatata confirmed the CO2-restricted photosynthesis in specimens collected offshore in deep reefs of the Great Barrier Reef (Díaz-Pulido et al., 2016). Red macroalgae that lack CCM tend to inhabit low-light habitats like subtidal or low intertidal zones and are abundant in cold waters (Cornwall et al., 2015; Raven et al., 2002a). According to these authors, approximately 35 % of the total red algae tested globally are strictly CO2 dependents. The percentage of macroalgae species representative of Arctic and Antarctic ecosystems that lack CCM is 42 %–60 % (Iñiguez et al., 2019; Raven et al., 2002b), 50 % for temperate waters of New Zealand (Hepburn et al., 2011), and up to 90 % found for a single site of Tasmania, Australia (Cornwall et al., 2015). Our study sampled 91 red macroalgae species (of 453 red macroalgae species reported in the GC; Pedroche and Sentíes, 2003), of which <3 % were CO2 dependents. This low percentage could be related to the fact that deep habitats (>2 m depth low tide) were not explored in our surveys.
Few calcifying macroalgae species using HCO were also collected, including the genera Amphiroa ( ‰) and Jania ( ‰), both Rhodophyta with articulated form. Padina, a genus with less capacity to precipitate CaCO3 (Iluz et al., 2017), displayed relatively high δ13C values ( ‰), suggesting the presence of CCM using HCO. Some species of Padina can use HCO, but their efficiency may differ from species to species (Enríquez and Rodríguez-Román, 2006; Raven et al., 2002a). Stepien (2015) reported a global mean of ‰ for calcifying species compared to ‰ for non-calcifying species. Calcifying macroalgae species showed a δ13C signal indicative of HCO use, the same source described as the substrate for calcification (Digby, 1977; Roleda et al., 2012), and other sources like respiratory CO2 for the calcifying process (Borowitzka and Larkum, 1976). Also, the boundary layers acidified by an excess of H+ released as residual products of the calcification benefit the HCO uptake (Comeau et al., 2012; McConnaughey et al., 1997). Another possibility to explain high δ13C values can also be related to the highly efficient light properties enhanced by the carbonate skeleton, resulting in an optimization of photosynthetic activity (Vásquez-Elizondo et al., 2017). Hofmann and Heesch (2018) reported high δ13C values in eight rhodolith species (calcifying species) for the organic matter thallus and for thallus, including CaCO3 structure collected in deep habitats (25–40 m) where light availability is limited. Because of the ocean acidification in progress, negative impacts are expected on calcifying organisms, and more attention as ecological sentinels is warranted in the GC.
Measurements of δ13C signal are evidence of the presence or absence of CCMs in macroalgae and indicate carbon use physiology (Giordano et al., 2005). However, the isotopic signature may be inconclusive in determining the efficient use of one or more DIC species (CO2 and HCO (Roleda and Hurd, 2012). The preferential DIC uptake of macroalgae is assessed by pH drift experiments (Fernández et al., 2014, 2015; Hepburn et al., 2011; Narvarte et al., 2020; Roleda and Hurd, 2012). Also, it can be determined by simultaneously measuring the CO2 uptake and O2 production rates using membrane-inlet mass spectroscopy (MIMS) (Burlacot et al., 2020; Douchi et al., 2019). Macroalgae that are unable to raise the seawater pH to > 9.0 are primarily CO2 users, while those that can raise the seawater pH > 9.0 (absence of CO2) are HCO users (Roleda and Hurd, 2012). Those differences in the carbon uptake strategies can be easily deduced by pH drift experiments, which were not done in our study but are reported in the literature (Table S4). Also, the change in δ13C signature within the range specific to a carbon use strategy (e.g., mixed HCO3 and user) can be complemented by simultaneous measurements of O2 and CO2 produced and consumed, respectively, using MIMS. For example, photosynthetic O2 production in a certain macroalgal species with an active CCM preference (e.g., CO2) is about 10 times higher than a non-active CCM (Burlacot et al., 2020).
Based on the δ13C values, it is possible to assume that at least one basal CCM is active. However, it is not possible to discern what type of CCM is expressed in the organisms (e.g., direct HCO uptake by the anion-exchange protein – AE; Drechsler and Beer, 1991; Drechsler et al., 1993) or types of mitochondrial carbonic anhydrase (e.g., internal and external) that enhance the fixation of DIC by recycling mitochondrial CO2 (Bowes, 1969; Sand-Jensen et al., 2020; Zabaleta et al., 2012). Also, the co-existence of different CCMs has been described for the same species (Axelsson et al., 1999; Xu et al., 2012), and it has even been described that different CCMs can operate simultaneously, generating different DIC contributions to RuBisCo internal pool (Rautenberger et al., 2015). The variety of CCMs and their combinations could contribute to the high δ13C variability for the same species. In our field study, it is impossible to explain the variations in δ13C or Δ13C macroalgae relative to CCM or CA activity types. Controlled experiments, like those conducted by Carvalho and collaborators (e.g., Carvalho et al., 2009a, b, 2010a), are required to obtain this knowledge.
4.3 Variability in δ13C macroalgae between the GC bioregions
Changes in the δ13C signal with latitude, mainly related to the light and temperature, have been reported in the literature (Hofmann and Heesch, 2018; Lovelock et al., 2020; Marconi et al., 2011; Mercado et al., 2009; Stepien, 2015). For example, a negative correlation between latitude and δ13C macroalgae was described by Stepien (2015). The authors concluded that the δ13C signal increased by 0.09 ‰ for each latitude degree from the Equator. Hofmann and Heesch (2018) showed a robust decreasing latitudinal effect in δ13C signals (R2=0.43δ13Ctotal and 0.13, for δ13Corganic-tissue, p=0.001) for rhodolite and macroalgae from coral reefs in Australia. In both cases, the latitude range is higher than what we tested (30 to 80∘ and from 10 to 45∘, respectively). These differences on a large scale tend to be associated with a temperature effect (Stepien, 2015) and their effect on CO2 solubility in seawater (Zeebe and Wolf-Gladrow, 2001). However, in our study, no geographical pattern in the δ13C macroalgae was observed. Our linear regression analyses for latitudes showed a low but significant correlation for the dataset classified by morphofunctional group and genus – negative in the cases of Rhodophyta and Ochrophyta groups and positive for Chlorophyta.
Light is not limited along the GC latitudes. Most of the shallow habitats occupied by macroalgal communities in the GC were high-light environments. In agreement with the literature, the surface seawater temperature across the GC varies by only 1 ∘C annual mean (Escalante et al., 2013; Robles-Tamayo, 2018). However, larger temperature variations of 5–10 ∘C were recorded in the coastal waters across the GC bioregions in both climatic seasons. The combined effect of the coastline sector, habitat feature, and environmental condition for morphofunctional group or genus explained 60 %–62 % and 71 %–72 % of the δ13C variability, respectively. Our analysis of variability for the best-represented morphological groups (e.g., R-Filamentous uniseriate and pluriseriate with erect thallus and C-Tubular) and genera (e.g., Colpomenia, Padina, Polysiphonia, and Gracilaria) revealed that certain life-forms are better monitors explaining the variability in δ13C macroalgae (and Δ13C values) than others. The δ13C variability in morphological groups refers to change within a specific carbon use strategy but not change in the carbon use physiology that is inherently species-specific. The biological or ecological relevance of the δ13C variability as a function of the morphology, in terms of the efficiency in the use of DIC and the isotope discrimination during carbon assimilation and respiration, must be investigated in species of the same genus but which are morphologically different or between the same morphological structures belonging to a different taxon.
The proportion of specimens with different carbon uptake strategies also showed regional variations. For example, the facultative uptake of HCO and CO2 was dominant in the macroalgal shallow communities in the GC (60 % to 90 % of specimens). Exceptions were observed for Ochrophyta in the P1 (68 %) and C1 (37 %) regions, where the strategy using only HCO dominated, while the strategy based on the use of only CO2 was observed in the peninsular coast in P2 and P3 for Rhodophyta with 2 %–3.3 %. Finally, the coastal sector C2 showed more negative δ13C values in macroalgae specimens of the same genus compared to the peninsular coastline (P1–P3). Small but detectable changes were observed in the phylum distribution based on environmental conditions. For example, Ochrophyta showed the highest proportion (35 %) in colder temperature, in pH alkaline (31 %), and in typical salinity regimen (27 %), while Chlorophyta enhanced to 30 % in acid pH, and Rhodophyta recorded 21 % in normal seawater. The opposite strategy (only use of dissolved CO2) was observed only in Rhodophyta. The highest percentage was observed in the estuarine salinity regimen (10 %). Again, more research is required to obtain valuable information on the physiological and environmental status of macroalgae.
In conclusion, we observed high δ13C macroalgal variability in macroalgae communities in the Gulf of California, such as reported in other worldwide marine ecosystems. The life-form is the principal cause of δ13C macroalgal variability, which explains up to 57 %. Changes in habitat characteristics and environmental conditions also influence the δ13C macroalgal variability within a specific carbon use strategy. Considering the combined effect of the life-form, coastline sector, and environmental conditions, the full model explains up to 72 % (genus) of the variability. The effects of the coastal sector, pH ranges, and emersion level were significant, while for salinity and temperature they were negligible.
Most macroalgae inhabiting in GC displayed the presence of CO2 concentrating mechanisms to uptake HCO for photosynthesis, and 84 % of the total analyzed specimens were able to use both HCO and/or CO2 employing active uptake plus passive diffusion (strategy 2: −10 < δ13C > −30 ‰). Specimens belonging to 58 species of 170 total species showed carbon uptake strategy 1 that uses only HCO. A higher proportion of CCM species (HCO users) was expected because we focused on intertidal and shallow subtidal habitats featured by high light intensities. Only three non-calcifying species (Schizymenia pacifica, Halymenia sp., Gigartina sp.) belonging to Rhodophyta (3 %) were CO2-exclusive users (strategy 3: δ13C < −30 ‰). The low percentage of CO2 dependents versus 40 %–90 % reported for temperate regions could be related to the shallow habitat sampled in our surveys (<2 m depth low tide). The calcifying macroalgae genera Amphiroa and Jania using HCO (high δ13C values) were present in the macroalgal communities in the GC. Because of the ongoing ocean acidification, these calcifying organisms constitute excellent ecological sentinels in the GC.
Finally, diverse authors have reported significant correlations between δ13C signal and latitude, mainly related to the light and temperature. However, in our study's latitude range (21–31∘ N), the linear regression analyses showed a low correlation for the δ13C macroalgal dataset classified by morphofunctional group and genus, which was negative for Rhodophyta and Ochrophyta and positive for Chlorophyta. Non-clear δ13C macroalgal patterns occur along the GC latitudes. However, detectable changes were observed in the δ13C macroalgae and the proportion of specimens with different carbon uptake strategies among coastal sectors. For example, the facultative uptake of HCO and CO2 was dominant in the macroalgal shallow communities in the GC (60 % to 90 % of specimens), but in the P1 (68 %) and C1 (37 %) the use of only HCO was the dominant strategy.
Our research is the first approximation to understand the δ13C macroalgal variability in one of the most diverse marine ecosystems in the world, the Gulf of California. We did not pretend to resolve the intricate processes controlling the variations in δ13C or Δ13C macroalgae during carbon assimilation and respiration and determine the isolated influence of each environmental factor. Despite the large dataset and corresponding statistical analyses, our study faces limitations due to research design and because no research on δ13C macroalgal analysis was developed previously in the GC. The primary deficiency is the lack of pH drift experiments to discriminate δ13C signal variations in the carbon uptake strategies to determine preferential DIC uptake of macroalgae (CO2 or HCO). The second limitation concerns the lack of controlled experiments to discern what type of CCM is expressed in macroalgae (e.g., direct HCO uptake by the anion-exchange protein AE, types of mitochondrial AC, or the co-existence of different CCMs). Also, more research is required to assess the biological or ecological relevance of the δ13C variability as a function of the morphology (e.g., DIC uptake efficiency and isotope discrimination during carbon assimilation and respiration). Future studies assessing the ability of macroalgae to use CO2 and/or HCO can be assessed by pH drift experiments and MIMS in the cosmopolites' species and within genus with differences in the δ13C values between species (e.g., Ulva and Sargassum). Finally, controlled experiments in laboratory and mesocosm type combined with field studies are required to elucidate what type of CCM is expressed in macroalgae. Even so, the δ13C macroalgae were a good indicator to infer the presence or absence of CCMs, to identify the macroalgae lineages that could be in a competitive advantage based on their carbon uptake strategy, and to identify their geographical distribution along with GC. Under the current climate change conditions and their effects as ocean acidification progresses and the bloom-forming macroalgae events increase in Mexico and worldwide, the analysis of δ13C macroalgae constitutes an excellent tool to help to predict the prevalence and shift of species in macroalgal communities which are focused on carbon metabolism. However, to obtain the maximum benefit from isotopic tools in the carbon-use strategy study, diverse and species-specific, it is necessary to use them in combination with other techniques referred to herein.
Datasets are each permanently deposited. Further information can be found at https://www.proquest.com/openview/2060de58b217ca47495469b53ae2f347/1?pq-origsite=gscholar&cbl=4882998 (Soto-Jimenez et al., 2020).
The supplement related to this article is available online at: https://doi.org/10.5194/bg-19-1-2022-supplement.
RVO participated in the collection, processing, and analysis of the samples as a part of his master's degree thesis. MJOI also participated in sample collections and identified macroalgae specimens. MFSJ coordinated the research, was the graduate thesis director, and prepared the manuscript with contributions from all co-authors.
The contact author has declared that neither they nor their co-authors have any competing interests.
Publisher's note: Copernicus Publications remains neutral with regard to jurisdictional claims in published maps and institutional affiliations.
The authors would like to thank Humberto Bojórquez-Leyva, Yovani Montaño-Ley, and Arcelia Cruz-López for their invaluable field and laboratory work assistance. Thanks to Sarahí Soto-Morales for the English revision. UNAM-PAPIIT IN206409 and IN208613 provided financial support, and UNAM-PASPA supported MF Soto-Jimenez for a sabbatical year. Thanks to CONACYT for a graduate fellowship to Roberto Velázquez-Ochoa.
This research has been supported by the Dirección General de Asuntos del Personal Académico, Universidad Nacional Autónoma de México (grant nos. PAPIIT IN206409 and PAPIIT IN208613).
This paper was edited by Aninda Mazumdar and reviewed by Matheus C. Carvalho, Michael Roleda, and one anonymous referee.
Abbot, I. A. and Hollenberg, G.: Marine algae of California, Standford University Press, California, 827 pp., 1976.
Aguilar-Rosas, L. E. and Aguilar-Rosas, R.: Ficogeografía de las algas pardas (Phaeophyta) de la península de Baja California, in: Biodiversidad Marina y Costera de México, Comisión Nacional Biodiversidad y Centro de Investigaciones de Quintana Roo, México, edited by: Salazar-Vallejo, S. I. and González, N. E., 197–206, 1993.
Aguilar-Rosas, L. E., Pedroche, F. F., and Zertuche-González, J. A.: Algas Marinas no nativas en la costa del Pacífico Mexicano. Especies acuáticas invasoras en México, Comisión Nacional para el Conocimiento y Uso de la Biodiversidad, México, 211–222, 2014.
Álvarez-Borrego, S.: Gulf of California, in: Ecosystems of the World, edited by: Ketchum, B. H., Estuaries and Enclosed Seas, Elsevier, Amsterdam, 427–449, 1983.
Anthony, K. R., Ridd, P. V., Orpin, A. R., Larcombe, P., and Lough, J.: Temporal variation of light availability in coastal benthic habitats: Effects of clouds, turbidity, and tides, Limnol. Oceanogr., 49, 2201–2211, https://doi.org/10.4319/lo.2004.49.6.2201, 2004.
Axelsson, L., Larsson, C., and Ryberg, H.: Affinity, capacity and oxygen sensitivity of two different mechanisms for bicarbonate utilization in Ulva lactuca L. (Chlorophyta), Plant Cell Environ., 22, 969–978, https://doi.org/10.1046/j.1365-3040.1999.00470.x, 1999.
Balata, D., Piazzi, L., and Rindi, F.: Testing a new classification of morphological functional groups of marine macroalgae for the detection of responses to stress, Mar. Biol., 158, 2459–2469, https://doi.org/10.1007/s00227-011-1747-y, 2011.
Bastidas-Salamanca, M., Gonzalez-Silvera, A., Millán-Núñez, R., Santamaria-del-Angel, E., and Frouin, R.: Bio-optical characteristics of the Northern Gulf of California during June 2008, Int. J. Oceanogr., 2014, 384618, https://doi.org/10.1155/2014/384618, 2014.
Bauwe, H., Hagemann, M., and Fernie, A. R.: Photorespiration: players, partners and origin, Trends Plant Sci., 15, 330–336, https://doi.org/10.1016/j.tplants.2010.03.006, 2010.
Beardall, J. and Giordano, M.: Ecological implications of microalgal and cyanobacterial CO2 concentrating mechanisms, and their regulation, Funct. Plant Biol., 29, 335–347, https://doi.org/10.1071/PP01195, 2002.
Bold, C. H. and Wynne, J. M.: Introduction to the Algae: Structure and reproduction, Prentice-Hall, Incorporated, New Jersey, USA, 706 pp., 1978.
Borowitzka, M. A. and Larkum, A. W. D.: Calcification in green alga Halimeda. III. Sources of inorganic carbon for photosynthesis and calcification and a model of mechanism of calcification, J. Exp. Bot., 27, 879–893, 1976.
Bowes, G. W.: Carbonic anhydrase in marine algae, Plant Physiol., 44, 726–732, https://doi.org/10.1104/pp.44.5.726, 1969.
Bray, N. A.: Thermohaline circulation in the Gulf of California, J. Geophys. Res.-Oceans., 93, 4993–5020, https://doi.org/10.1029/JC093iC05p04993, 1988.
Brodeur, J. R., Chen, B., Su, J., Xu, Y. Y., Hussain, N., Scaboo, K. M., Zhang, Y., Testa, J. M., and Cai, W. J.: Chesapeake Bay inorganic carbon: Spatial distribution and seasonal variability, Front. Mar. Sci., 6, 1–17, https://doi.org/10.3389/fmars.2019.00099, 2019.
Brusca, R. C., Findley, L. T., Hastings, P. A., Hendrickx, M. E., Cosio, J. T., and van der Heiden, A. M.: Macrofaunal diversity in the Gulf of California, in: Biodiversity, ecosystems, and conservation in Northern Mexico, 2005.
Burlacot, A., Burlacot, F., Li-Beisson, Y., and Peltier, G.: Membrane inlet mass spectrometry: a powerful tool for algal research, Front. Plant Sci., 11, 1302, https://doi.org/10.3389/fmicb.2019.01356, 2020.
Burnham, K. P. and Anderson, D. R.: A practical information-theoretic approach, Model selection and multimodel inference, 2nd Edn., Springer, New York, 2002.
Carrillo, L. and Palacios-Hernández, E.: Seasonal evolution of the geostrophic circulation in the northern Gulf of California, Estuar. Coast. Shelf S., 54, 157–173, https://doi.org/10.1006/ecss.2001.0845, 2002.
Carvalho, M. C. and Eyre, B. D.: Carbon stable isotope discrimination during respiration in three seaweed species, Mar. Ecol.-Prog. Ser., 437, 41–49, https://doi.org/10.3354/meps09300, 2011.
Carvalho, M. C., Hayashizaki, K., Ogawa, H., and Kado, R.: Preliminary evidence of growth influence on carbon stable isotope composition of Undaria pinnatifida, Mar. Res. Indones., 32, 185–188, 2007.
Carvalho, M. C., Hayashizaki, K., and Ogawa, H.: Carbon stable isotope discrimination: a possible growth index for the kelp Undaria pinnatifida, Mar. Ecol.-Prog. Ser., 381, 71–82, https://doi.org/10.3354/meps07948, 2009a.
Carvalho, M. C., Hayashizaki, K. I., and Ogawa, H.: Short-term measurement of carbon stable isotope discrimination in photosynthesis and respiration by aquatic macrophytes, with marine macroalgal examples, J. Phycol., 45, 761–770, 2009b.
Carvalho, M. C., Hayashizaki, K., and Ogawa, H.: Effect of pH on the carbon stable isotope fractionation in photosynthesis by the kelp Undaria pinnatifida, Coast. Mar. Sci., 34, 135–139, 2010a.
Carvalho, M. C., Hayashizaki, K., and Ogawa, H.: Temperature effect on carbon isotopic discrimination by Undaria pinnatifida (Phaeophyta) in a closed experimental system, J. Phycol., 46, 1180–1186, https://doi.org/10.1111/j.1529-8817.2010.00895.x, 2010b.
Carvalho, M. C., Santos, I. R., Maher, D. T., Cyronak, T., McMahon, A., Schulz, K. G., and Eyre, B. D.: Drivers of carbon isotopic fractionation in a coral reef lagoon: Predominance of demand over supply, Geochim. Cosmochim. Ac., 153, 105–115, https://doi.org/10.1016/j.gca.2015.01.012, 2015.
Cerling, T. E., Wang, Y., and Quade, J.: Expansion of C4 ecosystems as an indicator of global ecological change in the late Miocene, Nature, 361, 344–345, https://doi.org/10.1038/361344a0, 1993.
Chanton, J. P. and Lewis, F. G.: Plankton and dissolved inorganic carbon isotopic composition in a river-dominated estuary: Apalachicola Bay, Florida, Estuaries, 22, 575–583, https://doi.org/10.2307/1353045, 1999.
CNA (Comisión Nacional del Agua): Atlas del agua en México, available at: https://www.gob.mx/cms/uploads/attachment/file/259372/_2012_EAM2012.pdf, (last access: 5 November 2019) 2012.
Comeau, S., Carpenter, R. C., and Edmunds, P. J.: Coral reef calcifiers buffer their response to ocean acidification using both bicarbonate and carbonate, Proc. Bio. Sci., 280, 20122374, https://doi.org/10.1098/rspb.2012.2374, 2012.
Cooper, L. W. and DeNiro, M. J.: Stable carbon isotope variability in the seagrass Posidonia oceanica: Evidence for light intensity effects, Mar. Ecol.-Prog. Ser., 50, 225–229, 1989.
Cornelisen, C. D., Wing, S. R., Clark, K. L., Hamish Bowman, M., Frew, R. D., and Hurd, C. L.: Patterns in the δ13C and δ15N signature of Ulva pertusa: interaction between physical gradients and nutrient source pools, Limnol. Oceanogr., 52, 820–832, 2007.
Cornwall, C. E., Revill, A. T., and Hurd, C. L.: High prevalence of diffusive uptake of CO2 by macroalgae in a temperate subtidal ecosystem, Photosynth. Res., 124, 181–190, https://doi.org/10.1007/s11120-015-0114-0, 2015.
Cornwall, C. E., Comeau, S., and McCulloch, M. T.: Coralline algae elevate pH at the site of calcification under ocean acidification, Glob. Change Biol., 23, 4245–4256, 2017.
Dawson, E. Y.: The marine algae of the Gulf of California, Allan Hancock Pac. Exped., 3, 189–453, 1944.
Dawson, E. Y.: Marine red algae of Pacific México. Part 2. Cryptonemiales (cont.), Allan Hancock Pac. Exped., 17, 241–397, 1954.
Dawson, E. Y.: How to know the seaweeds, W.M.C. Brown Co. Publishers, Dubuque, Iowa, USA, 197 pp., 1956.
Dawson, E. Y.: The marine red algae of Pacific Mexico, Part 4, Gigartinales, Allan Hancock Pac. Exped., 2, 191–343, 1961.
Dawson, E. Y.: Marine red algae of Pacific México. Part 7. Ceramiales: Ceramiaceae, Delesseriaceae, Allan Hancock Pac. Exped., 26, 1–207, 1962.
Dawson, E. Y.: Marine red algae of Pacific México. Part 8. Ceramiales: Dasyaceae, Rhodomelaceae, Nova Hedwigia, 6, 437–476, 1963.
Díaz-Pulido, G., Cornwall, C., Gartrell, P., Hurd, C., and Tran, D. V.: Strategies of dissolved inorganic carbon use in macroalgae across a gradient of terrestrial influence: implications for the Great Barrier Reef in the context of ocean acidification, Coral Reefs, 35, 1327–1341, https://doi.org/10.1007/s00338-016-1481-5, 2016.
Digby, P. S. B.: Growth and calcification in coralline algae, Clathromorphum circumscriptum and Corallina officinalis, and significance of pH in relation to precipitation, J. Mar. Biol. Assoc. UK, 57, 1095–109, https://doi.org/10.1017/S0025315400026151, 1977.
Doubnerová, V. and Ryšlavá, H.: What can enzymes of C4 photosynthesis do for C3 plants under stress?, Plant Sci., 180, 575–583, https://doi.org/10.1016/j.plantsci.2010.12.005, 2011.
Douchi, D., Liang, F., Cano, M., Xiong, W., Wang, B., Maness, P. C., Lindblad, P., and Yu, J.: Membrane-Inlet Mass Spectrometry enables a quantitative understanding of inorganic carbon uptake flux and carbon concentrating mechanisms in metabolically engineered cyanobacteria, Front. Microbiol., 10, 1356–1356, https://https://doi.org/10.3389/fmicb.2019.01356, 2019.
Draper, N. R. and Smith, H.: Applied regression analysis, Vol. 326, John Wiley and Sons, New Jersey, USA, 1–715, ISBN 0-471-17082-8, 1998.
Drechsler, Z. and Beer, S.: Utilization of inorganic carbon by Ulva lactuca, Plant Physiol., 97, 1439–1444, https://doi.org/10.1104/pp.97.4.1439, 1991.
Drechsler, Z., Sharkia, R., Cabantchik, Z. I., and Beer, S.: Bicarbonate uptake in the marine macroalga Ulva sp. is inhibited by classical probes of anion exchange by red blood cells, Planta, 191, 34–40, https://doi.org/10.1007/BF00240893, 1993.
Dreckmann, K. M.: El género Gracilaria (Gracilariaceae, Rhodophyta) en el Pacífico centro-sur mexicano, Monografías ficológicas, 1, 77–118, 2002.
Dudgeon, S. R., Davison, I. R., and Vadas, R. L.: Freezing tolerance in the intertidal red algae Chondrus crispus and Mastocarpus stellatus: Relative importance of acclimation and adaptation, Mar Biol., 106, 427–436, https://doi.org/10.1007/BF01344323, 1990.
Dudley, B. D., Barr, N. G., and Shima, J. S.: Influence of light intensity and nutrient source on δ13C and δ15N signatures in Ulva pertusa, Aquat. Biol., 9, 85–93, https://doi.org/10.3354/AB00241, 2010.
Ehleringer, J. R., Sage, R. F., Flanagan, L. B., and Pearcy, R. W.: Climate change and the evolution of C4 photosynthesis, Trends Ecol. Evol., 6, 95–99, https://doi.org/10.1073/pnas.1718988115, 1991.
Enríquez, S. and Rodríguez-Román, A.: Effect of water flow on the photosynthesis of three marine macrophytes from a fringing-reef lagoon, Mar. Ecol.-Prog. Ser., 323, 119–132, https://doi.org/10.3354/meps323119, 2006.
Escalante, F., Valdez-Holguín, J. E., Álvarez-Borrego, S., and Lara-Lara, J. R.: Temporal and spatial variation of sea surface temperature, chlorophyll a, and primary productivity in the Gulf of California, Cienc. Mar., 39, 203–215, 2013.
Espinoza-Avalos, J.: Macroalgas marinas del Golfo de California, Biodiversidad marina y costera de México, Comisión Nacional Biodiversidad, Centro de Investigaciones de Quintana Roo, México, edited by: Salazar-Vallejo, S. I. and González, N. E., 328–357, 1993.
Espinosa-Carreón, T. L. and Escobedo-Urías, D.: South region of the Gulf of California large marine ecosystem upwelling, fluxes of CO2 and nutrients, Environ. Dev., 22, 42–51, https://doi.org/10.1016/j.envdev.2017.03.005, 2017.
Espinosa-Carreón, T. L. and Valdez-Holguín, E.: Variabilidad interanual de clorofila en el Golfo de California, Ecol. Appl., 6, 83–92, 2007.
Fernández, P. A., Hurd, C. L., and Roleda, M. Y.: Bicarbonate uptake via an anion exchange protein is the main mechanism of inorganic carbon acquisition by the giant kelp Macrocystis pyrifera (L aminariales, Phaeophyceae) under variable pH, J. Phycol., 50, 998–1008, https://https://doi.org/10.1111/jpy.12247, 2014.
Fernández, P. A., Roleda, M. Y., and Hurd, C. L.: Effects of ocean acidification on the photosynthetic performance, carbonic anhydrase activity and growth of the giant kelp Macrocystis pyrifera, Photosynth. Res., 124, 293–304, 2015.
Gateau, H., Solymosi, K., Marchand, J., and Schoefs, B.: Carotenoids of microalgae used in food industry and medicine, Mini-Rev. Med. Chem., 17, 1140–1172, https://doi.org/10.2174/1389557516666160808123841, 2017.
Gilbert, J. Y. and Allen, W. E.: The phytoplankton of the Gulf of California obtained by the “E.W. Scripps” in 1939 and 1940, J. Mar. Res., 5, 89–110, https://doi.org/10.1016/0022-0981(67)90008-1, 1943.
Giordano, M., Beardall, J., and Raven, J. A.: CO2 concentrating mechanisms in algae: mechanisms, environmental modulation and evolution, Annu. Rev. Plant Biol., 66, 99–131, https://doi.org/10.1146/annurev.arplant.56.032604.144052, 2005.
Grice, A. M., Loneragan, N. R., and Dennison, W. C.: Light intensity and the interactions between physiology, morphology and stable isotope ratios in five species of seagrass, J. Exp. Mar. Biol. Ecol., 195, 91–110, https://doi.org/10.1016/0022-0981(95)00096-8, 1996.
Gowik, U. and Westhoff, P.: The path from C3 to C4 photosynthesis, Plant Physiol., 155, 56–63, https://doi.org/10.1104/pp.110.165308, 2011.
Harris, D., Horwáth, W. R., and Van Kessel, C.: Acid fumigation of soils to remove carbonates prior to total organic carbon or carbon-13 isotopic analysis, Soil Sci. Soc. Am. J., 65, 1853–1856, https://doi.org/10.2136/sssaj2001.1853, 2001.
Hepburn, C. D., Pritchard, D. W., Cornwall, C. E., McLeod, R. J., Beardall, J., Raven, J. A., and Hurd, C. L.: Diversity of carbon use strategies in a kelp forest community: implications for a high CO2 ocean, Glob. Change Biol., 17, 2488–2497, https://doi.org/10.1111/j.1365-2486.2011.02411.x, 2011.
Hinger, E. N., Santos, G. M., Druffel, E. R. M., and Griffin, S.: Carbon isotope measurements of surface seawater from a time-series site off Southern California, Radiocarbon, 52, 69–89, 2010.
Hiraoka, M., Kinoshita, Y., Higa, M., Tsubaki, S., Monotilla, A. P., Onda, A., and Dan, A.: Fourfold daily growth rate in multicellular marine alga Ulva meridionalis, Sci. Rep.-UK, 10, 1–7, 2020.
Hofmann, L. C. and Heesch, S.: Latitudinal trends in stable isotope signatures and carbon-concentrating mechanisms of northeast Atlantic rhodoliths, Biogeosciences, 15, 6139–6149, https://doi.org/10.5194/bg-15-6139-2018, 2018.
Hopkinson, B. M., Dupont, C. L., Allen, A. E., and Morel, F. M. M.: Efficiency of the CO2-concentrating mechanism of diatoms, P. Natl. Acad. Sci. USA, 108, 3830–3837, https://doi.org/10.1073/pnas.1018062108, 2011.
Hopkinson, B. M., Young, J. N., Tansik, A. L., and Binder, B. J.: The minimal CO2 concentrating mechanism of Prochlorococcus MED4 is effective and efficient, Plant Physiol., 166, 2205–2217, https://doi.org/10.1104/pp.114.247049, 2014.
Hurd, C. L.: Water motion, marine macroalgal physiology and production, J. Phycol., 36, 453–472, https://doi.org/10.1046/j.1529-8817.2000.99139.x, 2000.
Iluz, D., Fermani, S., Ramot, M., Reggi, M., Caroselli, E., Prada, F., Dubinsky, Z., Goffredo, S., and Falin, G.: Calcifying response and recovery potential of the brown alga Padina pavonica under ocean acidification, ACS Earth Space Chem., 1, 316–323, https://doi.org/10.1021/acsearthspacechem.7b00051, 2017.
Iñiguez, C., Galmés, J., and Gordillo, F. J.: Rubisco carboxylation kinetics and inorganic carbon utilization in polar versus cold-temperate seaweeds, J. Exp. Bot., 70, 1283–1297, https://doi.org/10.1093/jxb/ery443, 2019.
Sand-Jensen, E. L., Maberly, S. C., and Gontero, B.: Insights on the functions and ecophysiological relevance of the diverse carbonic anhydrases in microalgae, Int. J. Mol. Sci., 21, 2922, https://doi.org/10.3390/ijms21082922, 2020.
Johansson, G. and Snoeijs, P.: Macroalgal photosynthetic responses to light in relation to thallus morphology and depth zonation, Mar. Ecol.-Prog. Ser., 244, 63–72, https://https://doi.org/10.3354/meps244063, 2002.
Kim, M. S., Lee, S. M., Kim, H. J., Lee, S. Y., Yoon, S. H., and Shin, K. H.: Carbon stable isotope ratios of new leaves of Zostera marina in the mid-latitude region: implications of seasonal variation in productivity, J. Exp. Mar. Biol. Ecol., 461, 286–296, https://doi.org/10.1016/j.jembe.2014.08.015, 2014.
Klenell, M., Snoeijs, P., and Pedersen, M.: Active carbon uptake in Laminaria digitata and L. saccharina (Phaeophyta) is driven by a proton pump in the plasma membrane, Hydrobiologia, 514, 41–53, https://doi.org/10.1023/B:hydr.0000018205.80186.3e, 2004.
Kroopnick, P. M.: The distribution of 13C of ΣCO2 in the world oceans, Deep-Sea Res. Pt. I, 32, 57–84, https://doi.org/10.1016/0198-0149(85)90017-2, 1985.
Kübler, J. E. and Davison, I. R.: High-temperature tolerance of photosynthesis in the red alga Chondrus crispus, Mar. Biol., 117, 327–335, https://doi.org/10.1007/BF00345678, 1993.
Kübler, J. E. and Dudgeon, S. R.: Predicting effects of ocean acidification and warming on algae lacking carbon concentrating mechanisms, PLoS One, 10, e0132806, https://doi.org/10.1371/journal.pone.0132806, 2015.
Kübler, J. E. and Raven, J. A.: The interaction between inorganic carbon acquisition and light supply in Palmaria palmata (Rhodophyta), J. Phycol., 31, 369–375, https://doi.org/10.1111/j.0022-3646.1995.00369.x, 1995.
Kübler, J. E. and Raven, J. A.: Inorganic carbon acquisition by red seaweeds grown under dynamic light regimes, Hydrobiologia, 326, 401–406, 1996.
Lapointe, B. E. and Duke, C. S.: Biochemical strategies for growth of Gracilaria tikvahiae (Rhodophyta) in relation to light intensity and nitrogen availability, J. Phycol., 20, 488–495, https://doi.org/10.1111/j.0022-3646.1984.00488.x, 1984.
Littler, M. M. and Arnold, K. E.: Primary productivity of marine macroalgal functional-form groups from south-western North America, J. Phycol., 18, 307–311, https://doi.org/10.1111/j.1529-8817.1982.tb03188.x, 1982.
Littler, M. M. and Littler, D. S.: The evolution of thallus form and survival strategies in benthic marine macroalgae: field and laboratory tests of a functional form model, Am. Nat., 116, 25–44, 1980.
Lobban, C. S. and Harrison, P. J.: Seaweed ecology and physiology, Cambridge University Press, New York, USA, ISBN 9780511626210, 1–366, 1994.
Lovelock, C. E., Reef, R., Raven, J. A., and Pandolfi, J. M.: Regional variation in δ13C of coral reef macroalgae, Limnol. Oceanogr., 65, 2291–2302, https://doi.org/10.1002/lno.11453, 2020.
Lluch-Cota, S. E., Aragón-Noriega, E. A., Arreguín-Sánchez, F., Aurioles-Gamboa, D., Bautista-Romero, J. J., Brusca, R. C., Cervantes-Duarte, R., Cortes-Altamirano, R., Del-MonteLuna, P., Esquivel-Herrera, A., Fernández, G., Hendrickx, M. E., Hernandez-Vazquez, S., Herrera-Cervantes, H., Kahru, M., Lavin, M., Lluch-Belda, D., Lluch-Cota, D. B., López-Martínez, J., Marinone, S. G., Nevarez-Martinez, M. O., Ortega-García, S., Palacios-Castro, E., Pares-Sierra, A., Ponce-Díaz, G., Ramirez-Rodríguez, M., Salinas-Zavala, C. A., Schwartzlose, R. A., and Sierra-Beltrán, A. P.: The Gulf of California: Review of ecosystem status and sustainability challenges, Prog. Oceanogr., 73, 1–26, https://doi.org/10.1016/j.pocean.2007.01.013, 2007.
Maberly, S. C., Raven, J. A., and Johnston, A. M.: Discrimination between 12C and 13C by marine plants, Oecologia, 91, 481–492, https://doi.org/10.1007/BF00650320, 1992.
Mackey, A. P., Hyndes, G. A., Carvalho, M. C., and Eyre, B. D.: Physical and biogeochemical correlates of spatio-temporal variation in the δ13C of marine macroalgae, Estuar. Coast. Shelf S., 157, 7–18, https://doi.org/10.1016/j.ecss.2014.12.040, 2015.
Madsen, T. V. and Maberly, S. C.: High internal resistance to CO2 uptake by submerged macrophytes that use HCO: measurements in air, nitrogen and helium, Photosynth. Res., 77, 183–190, https://doi.org/10.1023/A:1025813515956, 2003.
Marinone, S. G.: A note on “Why does the Ballenas Channel have the coldest SST in the Gulf of California?”, Geophys. Res. Lett., 34, L02607, https://doi.org/10.1029/2006GL028589, 2007.
Marinone, S. G. and Lavín, M. F.: Residual flow and mixing in the large islands' region of the central Gulf of California: Nonlinear processes in geophysical fluid dynamics, Springer, Dordrecht, https://doi.org/10.1007/978-94-010-0074-1_13, 2003.
Marconi, M., Giordano, M., and Raven, J. A.: Impact of taxonomy, geography and depth on the δ13C and δ15N variation in a large collection of macroalgae, J. Phycol., 47, 1023–1035, https://doi.org/10.1111/j.1529-8817.2011.01045.x, 2011.
Martínez-Díaz-de-León, A.: Upper-ocean circulation patterns in the Northern Gulf of California, expressed in Ers-2 synthetic aperture radar imagery, Cienc. Mar., 27, 209–221, https://doi.org/10.7773/cm.v27i2.465, 2001.
Martínez-Díaz-de-León, A., Pacheco-Ruíz, I., Delgadillo-Hinojosa, F., Zertuche-González, J. A., Chee-Barragán, A., Blanco-Betancourt, R., Guzmán-Calderón, J. M., and Gálvez-Telles, A.: Spatial and temporal variability of the sea surface temperature in the Ballenas-Salsipuedes Channel (central Gulf of California), J. Geophys. Res.-Oceans, 111, C02008, https://doi.org/10.1029/2005JC002940, 2006.
Masojidek, J., Kopecká, J., Koblížek, M., and Torzillo, G.: The xanthophyll cycle in green algae (Chlorophyta): its role in the photosynthetic apparatus, Plant Biol., 6, 342–349, https://doi.org/10.1055/s-2004-820884, 2004.
McConnaughey, T. A., Burdett, J., Whelan, J. F., and Paull, C. K.: Carbon isotopes in biological carbonates: respiration and photosynthesis, Geochim. Cosmochim. Ac., 61, 611–622, https://doi.org/10.1016/S0016-7037(96)00361-4, 1997.
Mercado, J. M., De los Santos, C. B., Pérez-Lloréns, J. L., and Vergara, J. J.: Carbon isotopic fractionation in macroalgae from Cadiz Bay (Southern Spain): comparison with other bio-geographic regions, Estuar. Coast. Shelf S., 85, 449–458, https://doi.org/10.1016/j.ecss.2009.09.005, 2009.
Mook, W. G., Bommerson, J. C., and Staverman, W. H.: Carbon isotope fractionation between dissolved bicarbonate and gaseous carbon dioxide, Earth Planet. Sc. Lett., 22, 169–176, https://doi.org/10.1016/0012-821X(74)90078-8, 1974.
Murru, M. and Sandgren, C. D.: Habitat matters for inorganic carbon acquisition in 38 species of red macroalgae (Rhodophyta) from Puget Sound, Washington, USA, J. Phycol., 40, 837–845, https://doi.org/10.1111/j.1529-8817.2004.03182.x, 2004.
Narvarte, B. C. V., Nelson, W. A., and Roleda, M. Y.: Inorganic carbon utilization of tropical calcifying macroalgae and the impacts of intensive mariculture-derived coastal acidification on the physiological performance of the rhodolith Sporolithon sp., Environ. Pollut., 266, 115344, https://doi.org/10.1016/j.envpol.2020.115344 , 2020.
Nielsen, S. L. and Sand-Jensen, K.: Allometric settling of maximal photosynthetic growth rate to surface/volume ratio, Limnol. Oceanogr., 35, 177–180, https://doi.org/10.4319/lo.1990.35.1.0177, 1990.
Norris, J. N.: The marine algae of the northern Gulf of California, PhD dissertation, University of California, Santa Barbara, 575 pp., 1975.
Norris, J. N.: Studies on Gracilaria Grev.(Gracilariaceae, Rhodophyta) from the Gulf of California, Mexico, Taxonomy of Economic Seaweeds, California Sea Grant College Program, California, Vol. I, 123–135, 1985.
Norris, J. N.: Marine algae of the northern Gulf of California: Chlorophyta and Phaoephyceae, Smithsonian Institution Scholarly Press, Washington DC, USA, no. 94, https://doi.org/10.5479/si.19382812.96, 2010.
Ochoa-Izaguirre, M. J. and Soto-Jiménez, M. F.: Variability in nitrogen stable isotope ratios of macroalgae: consequences for the identification of nitrogen sources, J. Phycol., 51, 46–65, https://doi.org/10.1111/jpy.12250, 2015.
Ochoa-Izaguirre, M. J., Aguilar-Rosas, R., and Aguilar-Rosas, L. E.: Catálogo de Macroalgas de las lagunas costeras de Sinaloa, Serie Lagunas Costeras, edited by: Páez-Osuna, F., UNAM, ICMyL, México, 117 pp., 2007.
Páez-Osuna, F., Piñón-Gimate, A., Ochoa-Izaguirre, M. J., Ruiz-Fernández, A. C., Ramírez-Reséndiz, G., and Alonso-Rodríguez, R.: Dominance patterns in macroalgal and phytoplankton biomass under different nutrient loads in subtropical coastal lagoons of the SE Gulf of California, Mar. Pollut. Bull., 77, 274–281, https://doi.org/10.1016/j.marpolbul.2013.09.048, 2013.
Páez-Osuna, F., Álvarez-Borrego, S., Ruíz-Fernández, A. C., García-Hernández, J., Jara-Marini, E., Bergés-Tiznado, M. E., Piñón-Gimate, A., Alonso-Rodríguez, R., Soto-Jiménez, M. F., Frías-Espericueta, M. G., Ruelas-Inzunza, J. R., Green-Ruíz, C. R., Osuna-Martínez, C. C., and Sánchez-Cabeza, J. A.: Environmental status of the Gulf of California: a pollution review, Earth-Sci. Rev., 166, 181–205, https://doi.org/10.1016/j.earscirev.2016.09.015, 2017.
Pedroche, F. F. and Sentíes, A.: Ficología marina mexicana: Diversidad y Problemática actual, Hidrobiológica, 13, 23–32, 2003.
Quay, P., Sonnerup, R., Westby, T., Stutsman, J., and McNichol, A.: Changes in the 13C/12C of dissolved inorganic carbon in the ocean as a tracer of anthropogenic CO2 uptake, Global Biogeochem. Cy., 17, 1–20, https://doi.org/10.1029/2001GB001817, 2003.
Rautenberger, R., Fernández, P. A., Strittmatter, M., Heesch, S., Cornwall, C. E., Hurd, C. L., and Roleda, M. Y.: Saturating light and not increased carbon dioxide under ocean acidification drive photosynthesis and growth in Ulva rigida (Chlorophyta), Ecol. Evol., 5, 874–888, https://doi.org/10.1002/ece3.1382, 2015.
Raven, J., Beardall, J., and Griffiths, H.: Inorganic C-sources for Lemanea, Cladophora, and Ranunculus in a fast-flowing stream: measurements of gas exchange and of carbon isotope ratio and their ecological implications, Oecologia, 53, 68–78, https://https://doi.org/10.1007/BF00377138, 1982.
Raven, J. A. and Beardall, J.: The ins and outs of CO2, J. Exp. Bot., 67, 1–13, https://doi.org/10.1093/jxb/erv451, 2016.
Raven, J. A., Johnston, A. M., Kübler, J. E., Korb, R. E., McInroy, S. G., Handley, L. L., Scrimgeour, C. M., Walker, D. I., Beardall, J., Clayton, M. N., Vanderklift, M., Fredriksen, S., and Dunton, K. H.: Seaweeds in cold seas: evolution and carbon acquisition, Ann. Bot., 90, 525–536, https://doi.org/10.1093/aob/mcf171, 2002a.
Raven, J. A., Johnshton, A. M., Kübler, J. E., Korb, R. E., Mclnroy, S. G., Handley, L. L., Scrimgeour, C. M., Walker, D. I., Beardall, J., Vanderklift, M., Fredriksen, S., and Dunton, K. H.: Mechanistic interpretation of carbon isotope discrimination by marine macroalgae and seagrasses, Funct. Plant Biol., 29, 355–378, https://doi.org/10.1071/PP01201, 2002b.
Raven, J. A., Ball, L. A., Beardall, J., Giordano, M., and Maberly, S. C.: Algae lacking carbon-concentrating mechanisms, Can. J. Bot., 83, 879–890, https://doi.org/10.1139/b05-074, 2005.
Roberts, K., Granum, E., Leegood, R. C., and Raven, J. A.: C3 and C4 pathways of photosynthetic carbon assimilation in marine diatoms are under genetic, not environmental control, Plant Physiol., 145, 230–235, https://doi.org/10.1104/pp.107.102616, 2007.
Robles-Tamayo, C. M., Valdez-Holguín, J. E., García-Morales, R., Figueroa-Preciado, G., Herrera-Cervantes, H., López-Martínez, J., and Enríquez-Ocaña, L. F.: Sea surface temperature (SST) variability of the eastern coastal zone of the gulf of California, Remote Sens., 10, 1434, https://doi.org/10.3390/rs10091434, 2018.
Roden, G. I.: Oceanographic and meteorological aspects of the Gulf of California, Pac. Sci., 12, 21–45, 1958.
Roden, G. I. and Emilsson, L.: Physical oceanography of the Gulf of California, Simposium Golfo de California, Universidad Nacional Autónoma de México, Mazatlán, Sinaloa, México, 1979.
Roden, G. I. and Groves, G. W.: Recent oceanographic investigations in the Gulf of California, J. Mar. Res., 18, 10–35, 1959.
Roleda, M. Y. and Hurd, C. L.: Seaweed responses to ocean acidification, in: Seaweed biology (Novel Insights into Ecophysiology, Ecology and Utilization), edited by: Caldwell, M. M., Heldmaier, G., Jackson, R. B., Lange, O. L., Mooney, H. A., Schulze, E.-D., and Sommer, U., Springer, Berlin, Heidelberg, 407–431, 2012.
Roleda, M. Y., Boyd, P. W., and Hurd, C. L.: Before ocean acidification: calcifier chemistry lessons, J. Phycol., 48, 840–843, 2012.
Rusnak, G. A., Fisher, R. L., and Shepard, F. P.: Bathymetry and faults of Gulf of California, in: Marine Geology of the Gulf of California: A symposium, edited by: van Andel, T. H. and Shor Jr., G. G., AAPG Memoir, Tulsa, OK, USA, 3, 59–75, https://doi.org/10.1306/M3359C3, 1964.
Sand-Jensen, K. and Gordon, D.: Differential ability of marine and freshwater macrophytes to utilize HCO and CO2, Mar. Biol., 80, 247–253, https://doi.org/10.1111/j.1469-8137.1981.tb03198.x, 1984.
Sanford, L. P. and Crawford, S. M.: Mass transfer versus kinetic control of uptake across solid-water boundaries, Limnol. Oceanogr., 45, 1180–1186, https://doi.org/10.4319/lo.2000.45.5.1180, 2000.
Santamaría-del-Angel, E., Alvarez-Borrego, S., and Müller-Karger, F. E.: Gulf of California biogeographic regions based on coastal zone color scanner imagery, J. Geophys. Res., 99, 7411–7421, https://doi.org/10.1029/93JC02154, 1994.
Santos, G. M., Ferguson, J., Acaylar, K., Johnson, K. R., Griffin, S., and Druffel, E.: Δ14C and δ13C of seawater DIC as tracers of coastal upwelling: A 5-year time series from Southern California, Radiocarbon, 53, 669–677, https://doi.org/10.1017/S0033822200039126, 2011.
Setchell, W. and Gardner, N.: The marine algae of the Pacific Coast of North America. Part II Chlorophyceae, Univ. Calif. Publ. Bot., 8, 139–374, https://doi.org/10.5962/bhl.title.5719, 1920.
Setchell, W. and Gardner, N.: The marine algae: Expedition of the California Academy of Sciences to the Gulf of California in 1921, Proc. Calif. Acad. Sci., 4th series, California Academy of Sciences, San Francisco, CA, USA, 12, 695–949, 1924.
Sharkey, T. D. and Berry, J. A.: Carbon isotope fractionation of algae as influenced by an inducible CO2 concentrating mechanism, Inorganic carbon uptake by aquatic photosynthetic organisms, American Society of Plant Physiologists, Rockville, MD, USA, 389–401, 1985.
Soto-Jimenez, M. F., Velázquez-Ochoa, R., and Ochoa Izaguirre, M. J.: Analysis of the variation of stable carbon isotopes in macroalgae communities from shallow marine habitats in the Gulf of California ecoregion, Earth and Space Science Open Archive ESSOAr, https://doi.org/10.1002/essoar.10504972.1, online first, 2020.
Stepien, C. C.: Impacts of geography, taxonomy and functional group on inorganic carbon use patterns in marine macrophytes, J. Ecol., 103, 1372–1383, https://doi.org/10.1111/1365-2745.12451, 2015.
Stroup, W. W., Milliken, G. A., Claassen, E. A., and Wolfinger, R. D.: SAS for mixed models: introduction and basic applications, SAS Institute, Cary, NC, USA, 1–48, 2018.
Teichberg, M., Fox, S. E., Olsen, Y. S., Valiela, I., Martinetto, P., Iribarne, O., Muto, E. Y., Petti, M. A., Cobrisier, T. N., Soto-Jiménez, M., Páez-Osuna, F., Castro, P., Freitas, H., Zitelli, A., Cardinaletti, M., and Tagliapietra, D.: Eutrophication and macroalgal blooms in temperate and tropical coastal waters: nutrient enrichment experiments with Ulva spp., Glob. Change Biol., 16, 2624–2637, https://doi.org/10.1111/j.1365-2486.2009.02108.x, 2010.
Valiela, I., Liu, D., Lloret, J., Chenoweth, K., and Hanacek, D.: Stable isotopic evidence of nitrogen sources and C4 metabolism driving the world's largest macroalgal green tides in the Yellow Sea, Sci. Rep., 8, 1–12, https://doi.org/10.1038/s41598-018-35309-3, 2018.
Vásquez-Elizondo, R. M. and Enríquez, S.: Light absorption in coralline algae (Rhodophyta): a morphological and functional approach to understanding species distribution in a coral reef lagoon, Front. Mar. Sci., 4, 297, https://doi.org/10.3389/fmars.2017.00297, 2017.
Vásquez-Elizondo, R. M., Legaria-Moreno, Pérez-Castro, M. A., Krämer, W. E., Scheufen, T., Iglesias-Prieto, R., and Enríquez, S.: Absorptance determinations on multicellular tissues, Photosynth. Res., 132, 311–324, https://doi.org/10.1007/s11120-017-0395-6, 2017.
Velasco-Fuentes, O. V. and Marinone, S. G.: A numerical study of the Lagrangian circulation in the Gulf of California, J. Marine Syst., 22, 1–12, https://doi.org/10.1016/S0924-7963(98)00097-9, 1999.
Young, E. B. and Beardall, J.: Modulation of photosynthesis and inorganic carbon acquisition in a marine microalga by nitrogen, iron, and light availability, Can. J. Bot., 83, 917–928, https://doi.org/10.1139/b05-081, 2005.
Young, J. N., Heureux, A. M., Sharwood, R. E., Rickaby, R. E., Morel, F. M., and Whitney, S. M.: Large variation in the Rubisco kinetics of diatoms reveals diversity among their carbon-concentrating mechanisms, J. Exp. Bot., 67, 3445–3456, https://doi.org/10.1093/jxb/erw163, 2016.
Xu, J., Fan, X., Zhang, X., Xu, D., Mou, S., Cao, S., Zheng, Z., Miao, J., and Ye, N.: Evidence of coexistence of C3 and C4 photosynthetic pathways in a green-tide-forming alga, Ulva prolifera, PloS one, 7, e37438, https://doi.org/10.1371/journal.pone.0037438, 2012.
Xu, J., Zhang, X., Ye, N., Zheng, Z., Mou, S., Dong, M., Xu, D., and Miao, J.: Activities of principal photosynthetic enzymes in green macroalga Ulva linza: functional implication of C4 pathway in CO2 assimilation, Sci. China Life Sci., 56, 571–580, https://doi.org/10.1007/s11427-013-4489-x, 2013.
Wiencke, C. and Fischer, G.: Growth and stable carbon isotope composition of cold-water macroalgae in relation to light and temperature, Mar. Ecol.-Prog. Ser., 65, 283–292, 1990.
Wilkinson, T. E., Wiken, E., Creel, J. B., Hourigan, T. F., and Agardy, T.: Marine Ecoregions of North America, Commission of Environmental Cooperation, Montreal, Canada, Montreal, Canada, 1–177, 2009.
Zabaleta, E., Martin, M. V., and Braun, H. P.: A basal carbon concentrating mechanism in plants?, Plant Sci., 187, 97–104, https://doi.org/10.1016/j.plantsci.2012.02.001, 2012.
Zeebe, R. E. and Wolf-Gladrow, D.: CO2 in seawater: equilibrium, kinetics, isotopes, No. 65, Gulf Professional Publishing, Elsevier Oceanography Series, Oxford, United Kingdom, 1–341, 2001.
Zeitzschel, B.: Primary productivity in the Gulf of California, Mar. Biol., 3, 201–207, https://doi.org/10.1007/BF00360952, 1969.
Zou, D., Xia, J., and Yang, Y.: Photosynthetic use of exogenous inorganic carbon in the agarophyte Gracilaria lemaneiformis (Rhodophyta), Aquaculture, 237, 421–431, https://doi.org/10.1016/j.aquaculture.2004.04.020, 2004.