the Creative Commons Attribution 4.0 License.
the Creative Commons Attribution 4.0 License.
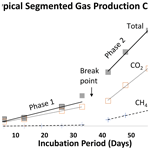
Quantification of potential methane emissions associated with organic matter amendments following oxic-soil inundation
Brian Scott
Andrew H. Baldwin
Stephanie A. Yarwood
Methane (CH4) emissions are a potent contributor to global warming, and wetlands can be a significant CH4 source. In a microcosm study, we evaluated how the practice of amending soils with organic matter as part of wetland restoration projects may affect CH4 production potential. Organic amendments including hay, manure, biosolids, composted yard waste, and wood mulch were evaluated at three different levels. Using 1 L glass microcosms, we measured the production of biogenic gases over 60 d in two soils designated by texture: a sandy loam (SL) and a sandy clay loam (SCL). Fresh organic amendments increased CH4 production, leading to potentially higher global warming potential and wetland C loss, and CH4 production was more pronounced in SL. We observed biogenic gas production in two sequential steady-state phases: Phase 1 produced some CH4 but was mostly carbon dioxide (CO2), followed by Phase 2, 2 to 6 weeks later, with higher total gas and nearly equal amounts of CH4 and CO2. If this is generally true in soils, it may be appropriate to report CH4 emissions in the context of inundation duration. The CH4 from the SCL soil ranged from 0.003–0.8 in Phase 1 to 0.75–28 in Phase 2 and from SL range from 0.03–16 in Phase 1 to 1.8–64 in Phase 2. Adding fresh organic matter (e.g., hay) increased concentrations of ferrous iron (Fe2+), whereas in some cases composted organic matter decreased both Fe2+ concentrations and CH4 production. Methanogenesis normally increases following the depletion of reducible Fe; however, we observed instances where this was not the case, suggesting other biogeochemical mechanisms contributed to the shift in gas production.
- Article
(1326 KB) - Full-text XML
-
Supplement
(5804 KB) - BibTeX
- EndNote
The ecological benefits of wetlands are well documented, including their role as carbon (C) sinks to stabilize global climate (Mitsch et al., 2015). Driven in part by this ecological contribution, from 1970 to 2015 human-made wetlands increased 233 % (Darrah et al., 2019). Between 2004 and 2009 the United States saw a net gain of 16 670 ha of freshwater wetlands: 360 820 ha of new wetlands to offset 344 140 ha of existing (presumably C-sink) wetlands that were destroyed (Dahl, 2011). Although created or restored wetlands may effectively sequester C, it may take hundreds of years to offset their radiative forcing due to methane (CH4) emissions (Neubauer, 2014). With such a large number of human-made wetlands and their potential to increase global warming, it is vital to consider factors that may contribute to CH4 emissions.
Organic amendments such as straw, wood mulch, manure, and biosolids, mixed into the soil, are thought to accelerate C storage by enhancing the conversion of plant-derived compounds to microbial residues (Richardson et al., 2016). Microbial residues, largely aliphatic C from cell membrane lipids, can accumulate in soil and are not directly accessible by methanogens (Chen et al., 2018). Plants contribute both above- and belowground organic matter (OM). Belowground plant materials are preferentially converted to soil organic carbon (SOC) (Mazzilli et al., 2015). In saturated soils root residues of wetland plants contain suberin and cutin (Watanabe et al., 2013), which persist, reducing biogenic gas production (Mikutta et al., 2006). Before contributing to SOC, standing litter in natural wetlands is partially decomposed by fungi (Kuehn et al., 2011) and further decomposed by aerobic bacteria (Yarwood, 2018). Allochthonous organic amendments are derived from aboveground material, but they have not been subjected to wetland biogeochemical processes. Studies suggest these materials are less amenable to soil C stabilization compared to natural plant inputs and may increase CH4 production (Scott et al., 2020). In addition to increasing CH4 production directly, organic amendments may cause SOC priming that produces additional CH4 (Nottingham et al., 2009) and can lead to an increase in iron (Fe) reduction and toxicity (Saaltink et al., 2017).
Iron oxides play multiple roles in anoxic soils, being both an electron acceptor for organic C metabolism (Straub et al., 2001) and a stabilizing agent for SOC on mineral surfaces (Lehmann and Kleber, 2015). As a metabolite, Fe reduction competes with CH4 production (Huang et al., 2009) and can facilitate sulfur recycling (which also competes with CH4 production) in freshwater sediments (Hansel et al., 2015). However, recent literature suggests the relationship of Fe reduction and methanogenesis is more complex. Some methanogens appear capable of switching between methanogenesis and Fe reduction (Sivan et al., 2016). In cultures with Methanosarcina acetivorans, adding Fe oxides increased methane production (Ferry, 2020), presumably by the utilization of a metabolic pathway where electron flow is bifurcated with some electrons going toward Fe reduction to increase energy yield (Zhuang et al., 2015; Prakash et al., 2019). In systems that are nearly pH neutral, Fe reduction does not necessarily have an energetic competitive advantage over CH4 production (Bethke et al., 2011). In addition to influencing metabolic pathways, metal-oxide surfaces can stabilize organic matter, making it less bioavailable, which can affect Fe reduction (Poggenburg et al., 2018), C mineralization (Amendola et al., 2018; Lalonde et al., 2012), and the production of CH4.
We carried out a lab experiment using organic amendments commonly used in wetland restoration (biosolids (Bloom®) – B, manure – M, composted yard waste (Leafgro®) – L, wood chips – W, and hay – H) and measured how they affected CH4 production and Fe reduction. Glass jar microcosms (1 L) were incubated with two different soils collected from sites where freshwater wetlands were recently created. The microcosms were kept under anaerobic conditions to compare the ability of these substrates to support anaerobic metabolism. We hypothesized that organic amendments would stimulate dissimilatory Fe reduction in soils (measured as soluble ferrous iron, Fe2+). Further, we hypothesized that amendments promoting Fe reduction would limit methanogenesis. We also tested differences between cured (i.e., aged/composted) and uncured (fresh) organic amendments and hypothesized that uncured amendments would increase Fe reduction due to the presence of more labile, soluble compounds. In the United States organic amendments are often required in mitigation wetlands, that is, wetlands created or restored to offset wetland losses; however, there has not been a systematic evaluation of whether or not amendments promote hydric soil conditions (Fe reduction), lead to Fe toxicity (from Fe reduction), or increase CH4 production.
2.1 Microcosm setup
Saturated incubations were established using soil from two recent mitigation wetlands located in Maryland, USA. The first site (76∘50′40.35′′ W, 38∘47′5.41′′ N) was most recently a horse pasture and will be referred to as SCL, denoting the texture (sandy clay loam). The second site (75∘47′40.20′′ W, 39∘01′52.42′′ N) was most recently a corn–soy farm with tile drains and was likely a wetland prior to conversion to farmland. The second site will be referred to as SL (sandy loam). Both sites had been recently graded to establish wetland topography, so the upper portion of the soils, where soil samples were collected, were blended with no ped structure: from an aquic hapludult (SCL) or a mixture from an aquic hapludult and/or a typic endoaquult and/or hapludult (SL). Soil was collected from these recently constructed surface horizons to a depth of 15 cm, a typical depth for mixing in organic amendments; sieved (2 mm); and homogenized prior to use. Additional soil information is shown in Table S1 in the Supplement.
Microcosm experiments were conducted in 1 L glass straight-sided wide-mouth food canning jars. Each microcosm had a total of 600 cm3 of solid material and was filled with water for a total volume of 660 cm3. The volumes needed to be precise in order to facilitate headspace and liquid sampling and to allow for space for soil expansion. When amendments were added, an equal volume of soil needed to be removed so that the total volume of solid material was a constant 600 cm3. At the start of the experiment, the headspace was purged with nitrogen (N) gas. The incubation temperature was 20 ∘C. Jar lids had precision-drilled holes fitted with grey butyl rubber stoppers, making it possible to non-destructively remove the overlying liquid (for Fe and pH analyses) using a 7.5 cm needle. Since the headspace pressure increased due to biogenic gas production, atmospheric pressure was re-established during gas sampling events by piercing the septa with a 24-gauge needle connected to a 50 mL gas-tight syringe. This procedure allowed us to record the total volume of gas produced and collect gas samples (0.01–1000 µL) under atmospheric pressure (Fig. S1 in the Supplement). A small coating of silicone applied to stoppers after piercing prevented leaks. All microcosm trials were run with three replicates except where noted.
2.2 Microcosm experiments
2.2.1 Experiment 1
We measured CH4 and Fe2+ production with various organic amendments, including composted yard waste (L; Leafgro), composted wood chips (W), class 1 biosolids – (B), manure (M), and hay (H) at three treatment levels: 8.8 % (), 26 %, and 53 % in two soils, an SL and an SCL. We used horse M for the SCL incubations and cow M for the SL incubations. This matched the wetland mitigation conditions at each field location. The treatment levels reflect the Maryland Department of the Environment (MDE) recommendation for wetland restoration (60 yd3 per acre – 113 m3 ha−1 – assuming a 6 in. – 15 cm – mixing depth) at 1, 3, and 6 times the MDE recommended level. All amendments were sieved to 5 mm. Hay was chopped with a Wiley mill, blended, or cut with scissors until it could easily pass a 5 mm sieve.
2.2.2 Experiment 2
We measured CH4 and Fe2+ production using cured (aged) and uncured (fresh) organic materials. We used two amendments, B and M. The two cured materials were from the same two sources as the fresh material but had been cured for a minimum of 3 months. We added the same amount of amendment to each microcosm based on OM content. Each amendment was evaluated for OM by loss on ignition (LOI) (550 ∘C for 2 h). Based on the percent OM we adjusted the amount of amendment so the final loading rate was 20 g OM per 600 cm3 soil. The microcosm setup was the same as Experiment 1 except that we used the same volume of soil (600 cm3) in all microcosms. These microcosms were incubated for 13 d and sampled periodically for Fe2+ and biogenic gases.
2.2.3 Experiment 3
We measured (a) CH4 and (b) Fe2+ production as a function of pH. We used H leachate as a substrate (McMahon et al., 2005). We leached 5.63 g H with 125 cm3 cold deionized water, shaking horizontally at 5 ∘C for 24 h. The leachate was filtered to 20 µm and immediately placed into jars with 600 cm3 SL soil and incubated for 22 d. The pH was adjusted to target levels of 5.6, 6.1, and 6.6 using a non-substrate buffer: 2-(N-morpholino) ethanesulfonic acid (MES). To determine the necessary concentration of MES, we titrated SL (pH 5.8) to our maximum desired pH (6.6). We determined that the buffering capacity of the soils corresponded to ∼ 2 mN in the 125 cm3 of liquid (leachate volume), so we prepared microcosms using 125 cm3 of 20 mN MES buffer.
2.2.4 Experiment 4
We measured Fe2+ production using leached H as a substrate (as in Experiment 3) but compared these finding to those with unleached H and the H residuals.
2.3 Soil, liquid, and gas analyses
Prior to the start of the experiments, we analyzed the SL and SCL for soil texture, percent soil C, and extractable Fe (Table S1). Soil texture was determined by adding 50 g soil to a 1000 mL cylinder with 0.5 % hexametaphosphate. Sand settled after 1 min, and silt settled after 24 h. The soil moisture content was determined as the weight loss of approximately 5 g of soil dried at 105 ∘C for 48 h. We determined percent soil C using thermal combustion at 950 ∘C on a LECO CHN-2000 analyzer (LECO Corp., St. Joseph, Michigan, USA). Iron extractions were performed sequentially with 1 M hydroxylamine hydrochloride (HHCL) in 25 % acetic acid, 50 g / 1 sodium dithionite in solution 0.35 M acetic acid / 0.2 M sodium citrate buffered to pH 4.8, and 0.2 M ammonium oxalate / 0.17 M oxalic acid (pH 3.2) (Poulton and Canfield, 2005). The HHCL extraction targets bioavailable iron, primarily ferrihydrite and lepidocrocite. Dithionite also includes more crystalline iron oxide forms, hematite, and goethite. Oxalate includes the bioavailable-iron oxides and magnetite.
Throughout the experiments we measured Fe2+, pH, and biogenic gases in the headspace. In some cases, Fe2+ and pH were measured only at the end of the incubation. Using a 3 in. (7.6 cm) needle, we extracted 0.3–1 cm3 (for Fe2+) and 1 cm3 (for pH) of the supernatant liquid to avoid disturbing soil in the jars. Samples of liquid supernatant were removed during gas sampling, when atmospheric pressure was maintained, to avoid loss of biogenic gases and atmospheric contamination. For the final sample point the jar contents were thoroughly mixed prior to sampling to include pore water and gases. Ferrous iron in supernatant liquid was measured with a Hach DR4000 spectrophotometer. The spectrophotometer was also used to measure Fe in the Fe oxide extractions. Prior to analysis, extracted Fe oxides were reduced by adding thioglycolic acid. To confirm the spectrophotometer accuracy, a subset of samples was also analyzed on a PerkinElmer PinAAcle 900T atomic absorption spectrometer. An Orion 9142BN electrode was used to determine pH.
Gas samples were collected in 12 cm3 N-purged exetainer vials and analyzed by injecting 5 cm3 into a Varian 450-GC gas chromatograph. Since sample volume was typically 1 cm3 or less, 5 cm3 N gas was added to the vials immediately prior to analysis for CO2 and CH4, and measured concentrations were corrected for dilution and prior headspace gas concentrations. We also performed fluorescent spectral scans on dissolved organic matter that was extracted from organic materials with 1:10 solid (weight) / deionized water (volume) for 24 h and filtered to 0.45 µm (Fischer et al., 2020). After diluting samples, emission spectra were recorded using an Aqualog fluorometer (HORIBA Scientific, Edison, New Jersey, USA).
2.4 Data analysis
Unless otherwise noted, statistical determinations were done using ANOVA (analysis of variance) in R or SAS. The Fe2+ concentrations were evaluated using contrasts for each of the amendments compared to the control using the R “multcomp” package. The gas curves were modeled as piecewise, bimodal linear functions using the R “segmented” package (Muggeo, 2008). Breakpoints were determined using the total gas curves, but, in some cases, the segmented package could not identify a breakpoint in the total gas curve, so CH4 curves were used as noted in Figs. S2 and S3 in the Supplement. Gas curves from H amendments did not fit a piecewise model and were modeled as sigmoidal functions using the “SSgompertz” function in R. However, SSgompertz is sensitive to data scatter, particularly at the beginning and end of the curve, so the gas curves for H6× in SL were fitted with a power function in Excel.
We present results from four separate experiments, summarized in Table 1. In Experiment 1, we evaluated Fe and CH4 production by varying OM type and dose and soil type (SL versus SCL). In Experiment 2 we controlled other factors and compared composted versus fresh OM. In Experiment 3 we characterized the effects of pH. In Experiment 4 we compared iron reduction from the soluble and particulate fraction of fresh hay, and the results were used to emphasize the pH effect.
Table 2(a) Experiment 1a – Phase 1. Carbon dioxide (CO2), methane (CH4), and total gas production. Organic amendment types – B (biosolids), M (manure), L (composted yard waste), and W (composted wood chips) – and levels (1, 3, or 6 times the amount of organic matter equivalent to 60 yd3 per acre – 113 m3 ha−1 – to a depth of 6 in. – 15 cm) in sandy clay loam (SCL) and sandy loam (SL). Instances where organic amendments did not increase CH4 production are in bold. Note: CO2-to-CH4 ratios are based on calculated gas production rates, not total gas produced. (b) Experiment 1a – Phase 2. Carbon dioxide (CO2), methane (CH4), and total gas production and the Phase 1:Phase 2 breakpoint. Organic amendment types – B (biosolids), M (manure), L (composted yard waste), and W (composted wood chips) – and levels (1, 3, or 6 times the amount of organic matter equivalent to 60 yd3 per acre to a depth of 6 in.) in sandy clay loam (SCL) and sandy loam (SL). Instances where organic amendments did not increase CH4 production are in bold. Note: r2 values represent the combined best-fit curve, using triplicate samples, for Phase 1 (Table 1a) and Phase 2. (c) Experiment 1a. Carbon dioxide (CO2), methane (CH4), and total gas production with the hay (H) amendment. H-amended trials fit a sigmoidal, not segmented, pattern, and therefore there was no breakpoint. We present p values for the sigmoidal fit, except for H6 SL rates, where we used a power function in Excel and report the r2 value. Gas production rates (cm3 kg−1 d−1) represent the maximum at the inflection point. The amendment floated to the surface in the SCL H3 and H6 trials, which resulted in unusually low CH4 production rates.
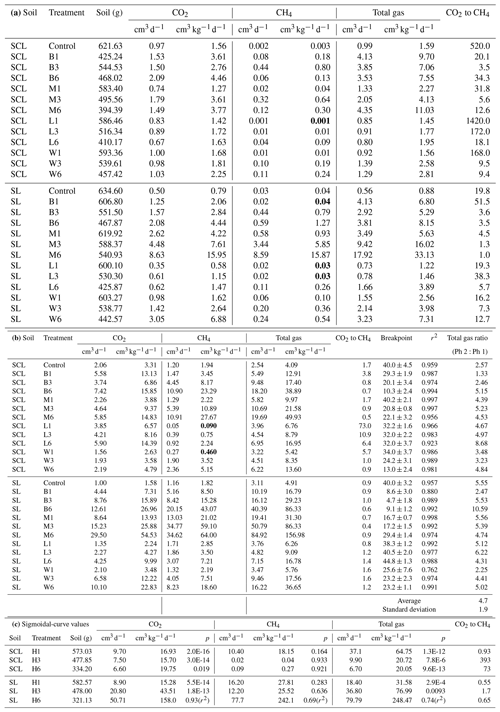
3.1 Experiment 1a: effect of organic amendments and soil type on CH4 gas production
Gas production occurred in two distinct steady-state gas production periods, which we identified as Phase 1 and, after a breakpoint, Phase 2 (Fig. 1), with individual gas curves shown in Figs. S2 (SCL) and S3 (SL). Some CH4 was produced almost immediately upon inundation (Table 2a), but after the breakpoint (40 d in both the SL and SCL soils), there is a large increase in CH4 as well as an average 4.7 × ± 1.9 increase in total gas production (Table 2b). One of our amendments, H, did not fit the linear bimodal pattern, so we reported rates separately on Table 2c.
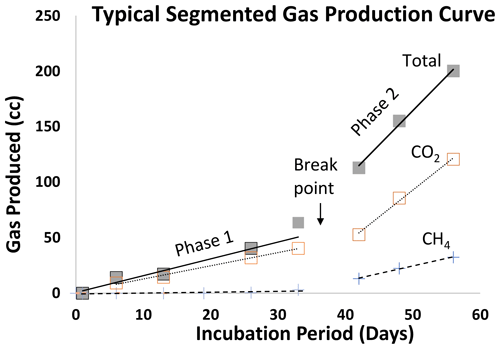
Figure 1Typical gas production in saturated soils amended with organic matter (all experiments). Gases were best modeled using a segmented linear function. After a breakpoint the average total gas production increased by a factor of ∼ 5, whereas there is a sharp (≫ 5×) increase in methane production. Data presented are from the manure-amended (1×) trials in sandy clay loam. Note that hay-amended trials exhibited a typical sinusoidal pattern (shown in Figs. S2 and 3h, i, and j).
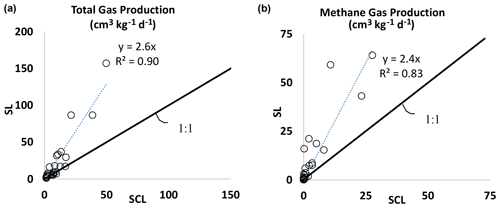
Figure 2(a) Experiment 1. Total biogenic gas production rate in the SL soil versus the SCL mesocosms. The SL mesocosms had, on average, 2.6 times higher gas production than SCL. (b) Experiment 1. Biogenic methane gas production rate in the SL soil versus the SCL mesocosms. The SL mesocosms had, on average, 2.4 times higher methane gas production than SCL.
Gas production varied by soil texture. In general, the SL soil produced 2.6 times as much total gas (Fig. 2a) and 2.4 times as much CH4 as SCL (Fig. 2b). In the SCL soil, CH4 production in Phase 1 was 0.003 cm3 kg−1 d−1 and with amendments increased to as much as 0.8 cm3 kg−1 d−1 (Table 2a). In Phase 2 1.9 cm3 kg−1 d−1 was produced in control soils and with amendments increased to as much as 28 cm3 kg−1 d−1 (Table 2b). In the SL soil, amendments increased the rate from 0.04 to 16 cm3 kg−1 d−1 in Phase 1 and from 1.8 to 64 cm3 kg−1 d−1 in Phase 2.
Gas production rates generally increased with amendment loading rate (Table 2a and b), as expected. With the exception of L in SL, all amendments reduced the time required to transition from Phase 1 to Phase 2 (i.e., the breakpoint). Biosolids caused the largest shift, decreasing the breakpoint to as little as 5 d. While amendments generally increased CH4 production, there were exceptions. Low loading rates of cured amendments (L and W) had lower CH4 production rates than unamended soil: L1 in Phase 1 in both soils, L3 in SL, L3 in SCL (Phase 2 only), and W1 in SCL (Phase 2). Biosolids (B1) also lowered CH4 production rates in the SL soil (Phase 1) (Table 2a). We examined the normalized CH4 production rates (per g C in soil), but in most cases results were not statistically different at p < 0.05 (Fig. S4 in the Supplement). The general trends indicate that uncured amendments (e.g., B and M) produce more methane per unit carbon than cured amendments (L).
Using fresh H, biogenic gas production followed a sinusoidal pattern, and we reported maximum CH4 production rate at the inflection point (Table 2c). Hay was prone to floating at higher loading rates and was present in the water column above the surface (not in contact with soil). In the instances where this occurred (H3 and H6 in SCL), there was a decrease in the overall gas production rate and very low CH4 – much lower than unamended soils (Table 2c and Fig. S2z). Floating also occurred in one replicated for H6 in SL – the pattern is shown in Figs. S2 and 3z but not used in the average reported value (Table 2c).
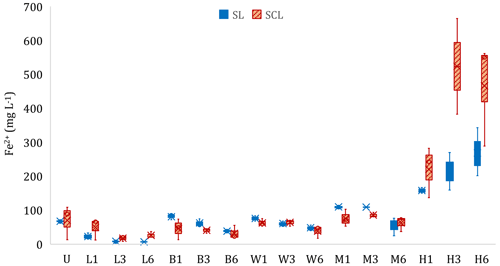
Figure 3Experiment 1b. Concentration of ferrous iron (Fe2+) in the liquid phase at the end of the incubation period. Microcosms receiving different organic amendment types and levels in sandy clay loam (SCL) and sandy loam (SL). U: no-amendment control, L: Leafgro (yard waste), B: biosolids, W: wood chips, M: manure, and H: hay. Numbers signify treatment level (1, 3, or 6 times the amount of organic matter equivalent to 60 yd3 per acre to a depth of 6 in.). Different lowercase letters signify differences (p < 0.05) based on contrasts compared to U, and brackets signify that all results in the bracketed group were not statistically different. H increased total Fe2+ production compared to U in both soils, and L decreased total Fe2+ production compared to U (SL only).
3.2 Experiment 1b: effect of organic amendments and soil type on Fe2+
The type and loading rate of organic amendments affected total soluble Fe2+ production, compared to the unamended control, in a limited number of cases (Fig. 3, Table S2). In the SL soil, L caused a decrease (p < 0.05) in supernatant Fe2+ concentrations, whereas H increased supernatant Fe2+ in both soils (p < 0.05). In a separate set of experiments, we documented the relationship between supernatant Fe and pore water Fe (Fig. S5 in the Supplement). Soil type affected the amount of soluble Fe2+ produced (p < 0.05). We did not see a difference in Fe2+ in the unamended microcosms even though SCL had 2.2 times the amount of hydroxylamine-hydrochloride-extractable Fe (FeHHCl) compared to SL and had 7.6 times more dithionite-extractable Fe (Table S1). Of the FeHHCl in soil, 19 % or less in SCL and 61 % or less in SL was reduced to Fe2+. Hay was an exception, where up to 155 % of the FeHHCl in SCL and 236 % in SL was reduced to Fe2+ (Table S2). During the SL soil incubations, aqueous Fe2+ was measured simultaneously to CH4 production. In the H and M treatments, there was a marked increase in CH4 production when Fe2+ became asymptotic. However, with the other amendments, Fe2+ production continued or even increased during periods of high CH4 production. Figure 4 shows two examples that highlight this pattern, and the complete set of curves is in Fig. S6 in the Supplement.
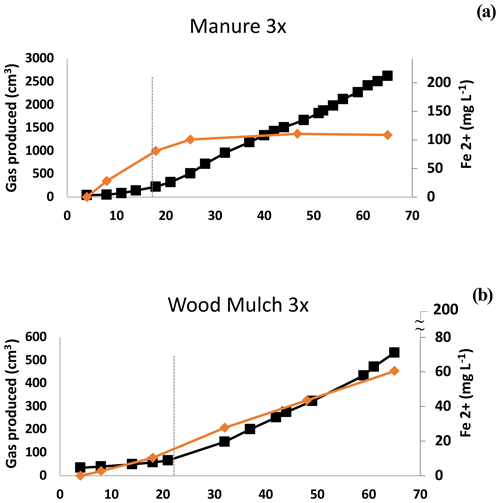
Figure 4Experiment 1b. Ferrous iron (Fe2+) and methane (CH4) in selected microcosms. Depletion of Fe coincided with the breakpoint (dashed line) with manure but not with wood mulch. Other examples of this pattern are shown in Fig. S6. The maximum value on the secondary x axis is the maximum expected Fe2+ concentration based on the HHCL extraction.
3.3 Experiment 2a: effect of cured versus fresh organic amendments on CH4 gas production
In Experiment 1a, it appeared that curing may have had an effect on CH4 production. Fresh H produced the most CH4. The H1 trials had maximum production rates of 18.2 and 27.8 cm3 kg−1 d−1 in the SCL and SL soils, respectively (Table 2c). The H3 and H6 loading rates would likely have been higher had some portion of the H not floated. The M6 trials produced the most CH4 at 27.7 and 64.0 cm3 kg−1 d−1 in the SCL and SL soils, respectively. Of the amendments used, M was cured the least (after fresh H, which was uncured). The Leafgro was cured the most and produced very little CH4, in some cases less than the controls. Since we could not specify precisely how long the organic material had been cured, we conducted a separate experiment with organic materials of known curing periods (at least 90 d), using B and M. Rather than use the same volumetric quantities, we used the same loading rate based on OM content. The results confirmed that curing has a strong influence on CH4 production. Methane production was higher using fresh material in both cases, and cured material sometimes decreased CH4 production (Table 3).
3.4 Experiment 2b: effect of cured versus fresh organic amendments on Fe2+ production
In Experiment 1b, we observed that curing also had an effect on the amount of Fe2+ produced. Hay was the only amendment that produced significantly more Fe2+, and L produced a significant reduction in Fe2+ (Fig. 3). In Experiment 2 we used biosolids (B) and manure (M) that had been cured at least 3 months. Whether the material had been cured had a strong influence on Fe2+ production, and Fe2+ was higher using fresh material in both cases (Fig. 5).
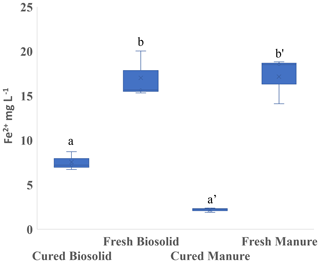
Figure 5Experiment 2b. Concentration of ferrous iron (Fe2+) in the liquid phase at the end of the incubation period (13 d). Incubation was carried out in sandy loam. Different letters indicate a difference at p < 0.001.
3.4.1 Spectral analysis: effect of organic amendments and soil type on CH4 gas production
We observed differences in CH4 and Fe reduction rates when using organic material that had been cured versus uncured. The fluorescent spectral signatures of the cured materials (B and M) were similar as were the signatures of fresh material (Fig. S7 in the Supplement), so curing differentiated the materials more than the source. The difference in signatures was indicative of higher concentrations of organic (humic) acids and lower nominal oxidation state in the cured materials. We considered other organic matter characterization methods such as the material's C-to-N ratio, but we did not find another reliable predictor of CH4 and Fe2+ production other than curing.
3.5 Experiment 3: effect of pH on (a) CH4 and (b) Fe2+ production
The soil pH affected both CH4 and Fe2+ production. In Experiment 1, we observed that Fe2+ varied with pH in the SL soil (p < 0.001; Fig. S8a in the Supplement), but there was little variation in SCL (p = 0.45; Fig. S8b). In order to isolate the effect of pH, we performed Experiment 3 using a single substrate (H leachate) in the SL soil. Higher pH increased the CH4 production rate in both Phase 1 and Phase 2 (Table 4) and reduced the production of Fe2+ (Fig. 6).
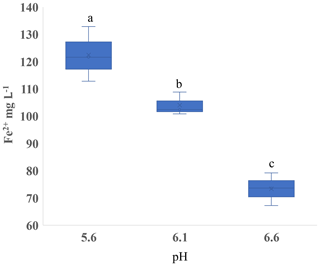
Figure 6Experiment 3. Concentration of ferrous iron (Fe2+) in the liquid phase with varied pH in microcosms receiving hay in sandy loam. Different letters indicate a difference at p < 0.05.
3.6 Experiment 4: leached versus unleached H and pH considerations
In Experiment 4 we measured Fe2+ produced from H, H leachate, and H residuals (Fig. 7). We expected the soluble fraction to be more labile and produce more Fe2+; however, the H residuals (solid fraction) appeared to produce more Fe2+ than the leachate. As noted on the figure, separate leached fractions changed the system pH. Using the results from Experiment 2, we predict that at comparable pH there would have been no difference in Fe2+ production between H, H residuals, and leachate (Fig. S9 in the Supplement). Given the potentially strong influence of pH, we re-evaluated the results from Experiment 2b, correcting for pH, and confirmed that the organic material age accounts for differences in Fe2+ production (Fig. S10 in the Supplement). Similarly, we considered whether pH may have affected the outcome of Experiment 1. A MANOVA (multivariate analysis of variance) analysis of the Experiment 1 data (Table S3) indicated that pH and soil type had a small effect (p = 0.30 and 0.81, respectively) compared to organic matter type and loading rate (p < 0.0001).
Table 3Experiment 2a. Methane gas data for incubations with fresh and cured organic matter in sandy loam soil.
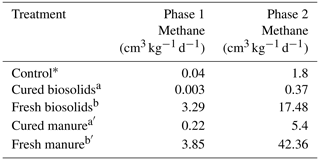
Control data (∗) from Experiment 1a (Table 2a) included for reference. Different letters indicate a difference at p < 0.001.
Net CH4 emissions are a primary factor that determines whether a wetland is a C sink or contributes to long-term global warming (Neubauer and Verhoeven, 2019). Soil management practices, such as wetland restoration methods, can have a large impact on CH4 production and total greenhouse gas emissions (Paustian et al., 2016). Our data indicate that organic amendments used in created or restored wetlands may have a large influence on CH4 production. Organic amendments that had been cured (L and W) only slightly increased CH4 emissions, but fresh material (M and H) resulted in large increases (Table 1a and b). This is consistent with field studies where comparable cured amendments (composted wood and yard waste) did not result in increased CH4 emissions (Winton and Richardson, 2015), but straw (Ballantine et al., 2015) and peat bales (Green, 2014) increased CH4 emissions. Organic material is commonly cured or composted to remove plant pathogens (Noble and Roberts, 2004) and to reduce the amount of cellulosic material (Hubbe et al., 2010), which competes for oxygen, contributing to phytotoxicity (Saidpullicino et al., 2007; Hu et al., 2011). Curing produces humic acids and increases the nominal oxidation state (NOSC) of C (Guo et al., 2019). When cured material is then subjected to anaerobic conditions, less CH4 is produced (Yao and Conrad, 1999), which would make composted material more suitable in a wetland restoration context.
Following soil inundation, we observed two distinct gas production phases (Phase 1 and Phase 2). This pattern is difficult to distinguish in unamended soils but has been reported previously (Yao and Conrad, 1999; Drake et al., 2009). Our breakpoint (5–45 d, Table 2b) was similar to Yao and Conrad (1999) (5–36 d). The Phase 2 rates in unamended soils were also similar: 0.96–3.98 in Yao and Conrad (1999) and 1.82–1.94 in our study (Table 2b).
There are several explanations that could account for the observed gas production pattern. One is the lag period required to re-establish populations of methanogenic archaea, which are likely dormant under oxic conditions, and regrowth can be on the order of days (Jabłoński et al., 2015). In our study, B had the earliest shift to Phase 2 CH4 production (Table 2b), possibly due to elevated levels of dormant methanogens present from anaerobic digestion. The two-phase gas production could also be due to depletion of bioavailable-Fe oxides, thus relieving the competition between Fe reducers and methanogens (Megonigal et al., 2004). Our data were mixed, with some treatments showing evidence of competition by Fe reducers, but in other cases we did not see competition. In treatment M1, for example, ferrous Fe in the supernatant plateaued at about the same time as the breakpoint (Fig. 4b), after which CH4 production increased. In contrast, in W3 soluble Fe continued to be produced well after the breakpoint, and the amount of bioavailable Fe used during the course of the incubation was less than 28 ± 4 % (Fig. 4b, Table S2). In addition to quantifying Fe oxide concentrations, the CO2:CH4 ratios can be indicative of interactions between methanogens and other reducers (Bridgham et al., 2013). If Fe reduction or other reduction stops during Phase 2, we would expect the CO2:CH4 ratio to be near 1:1 (Bridgham et al., 2013). However, we observed notable exceptions. The SCL L1 treatment had a ratio of 73:1 in Phase 2 (Table 2b) yet still had the characteristic shift to higher overall gas production (4.7×). Other treatments also had higher CO2:CH4 ratios: L3, L6, W1, B1, C, and W1–W3 in the SL soil (Table 2b). Our mixed observations may have been due to microsite formation. In high-production microcosms, microsite development may have been disrupted by gas ebullition, which was substantial enough in H-amended trials to cause effervescence. Amendments with low gas production and limited gas ebullition (e.g., L, W, and C) continued to produce Fe2+ after the breakpoint, possibly because methanogens were active in undisturbed microsites, as described in Yang et al. (2017).
The increased gas production from organic amendments was more pronounced in SL compared to SCL, where there was 2.4 times higher CH4 and 2.6 times higher gas production (Fig. 2a and b). We observed a more pronounced effect than a recent rice field study where there was more CH4 from SL soils versus SCL, although in that study results were not statistically significant (Kim et al., 2018). Yagi and Minami (1990) observed that compost (approximate loading rate was the same as our 1× treatment) increased respiration rates by 1.8 times in a SCL versus a loam soil. Maietta et al. (2020a) observed that respiration rates were higher in a sandy loam soil compared to a silty clay, with and without 3.3 % and 23 % wetland hay amendments. Thus, we might conclude that in general coarser grained (sandy) soil textures emit more CH4; however, there are a number of investigations where this was not the case (Yagi and Minami, 1990; Glissmann and Conrad, 2002). Other factors may have contributed. In our experiment SCL had 7.6 times dithionite-extractable Fe and 4.6 times as much percentage of C (Table S1), so additional studies would be needed to isolate texture as the controlling factor.
We considered the gas production from H microcosms separately because they followed a different pattern than the other amendments, but the pattern was similar to other studies using hay (Glissmann and Conrad, 2002) and wetland hay (Maietta et al., 2020b). Our study adds to these findings by observing that H produced very low CH4 in the water column (after floating) compared to being mixed with soil (Table 2c). This may merit further study because if this is generally true, applying fresh organic matter as a mulch, rather than mixed into the soil, could greatly reduce the adverse consequence of increased CH4 emissions.
Reduction of Fe oxides occurs in saturated soils in the presence of an organic substrate and is a key biogeochemical process in wetland soils. With sufficient time, hydric soils may develop redoximorphic features from Fe reduction; however, studies have not shown lasting redoximorphic development due to organic amendments (Gray, 2010; Ott et al., 2020). Organizations responsible for constructing mitigation wetlands have an interest in documenting Fe reduction prior to redoximorphic feature development as evidence soils that are hydric. Some mitigation wetland practitioners experience challenges meeting hydric soil testing standards. Although reports in the scientific literature are rare, there are examples of sites meeting vegetation and hydrology wetland indicators but not hydric soils (Berkowitz et al., 2014). Both the soils we tested produced sufficient Fe2+ and would have passed hydric soils tests, so a soil amendment would not be needed.
We observed that fresh organic matter resulted in increased Fe2+ compared to cured organic matter (Fig. 3), likely due to the presence of labile carbon, allowing access to more crystalline Fe oxides (Lentini et al., 2012). In some soils, Fe-reducing bacteria using fresh organic matter amendments could access crystalline Fe, making it more bioavailable. However, without an anoxic–oxic cycle, increased Fe2+ production could lead to Fe2+ toxicity and ferrolysis (Kirk, 2004), similar to the way fresh organic matter leads to SOC priming (Blagodatsky et al., 2010). Ferrolysis occurs when bioavailable-Fe oxides are reduced to Fe2+ and are subject to hydraulic transport. We observed that cured amendments, like L, lowered Fe2+ concentrations (Fig. 3), possibly due to the presence of humic acids that are generated during curing (Guo et al., 2019). Humic acids often contain insufficient biogeochemical energy to drive dissimilatory Fe reduction (Keiluweit et al., 2017); chelate Fe2+, removing it from the liquid phase (Catrouillet et al., 2014); and create insoluble precipitates (Shimizu et al., 2013).
Regulating Fe2+ production, through the selection of the appropriate OM amendment, could influence the growth of wetland plants. For example, rice growth may be stimulated under low Fe2+ doses of 1 (Müller et al., 2015), but higher doses can produce detrimental Fe plaque (Pereira et al., 2014). Some native wetland species are adapted to high Fe2+ concentrations. Juncus effusus growth is stimulated at 25 Fe2+ (Deng et al., 2009). North American native reed, Phragmites australis ssp. americanus, was stimulated at 11 Fe2+ from ferrous sulfate (Willson et al., 2017), but the invasive Eurasian lineage of Phragmites australis seedling growth was inhibited by Fe2+ as low as 1 (Batty, 2003). Soils high in free Fe2+ adversely affected P. australis growth by creating an Fe oxide plaque on roots (Saaltink et al., 2017).
Our results show that pH has a significant effect on both the production of Fe2+ (Fig. 3) and CH4 (Table 3). Between pH 5.6 and 6.6, the lower pH produced more Fe2+ and less CH4, consistent with thermodynamic predictions (Ye et al., 2012). Hydrogenotrophic methanogens can maximize CH4 production at pH 5 (Bräuer et al., 2004). In rice paddy soils, CH4 emissions had a clear peak at pH 7, but almost none was below pH 5.5 (Wang et al., 1993). The strong effect of pH underscores the need to take this parameter into account when interpreting data from experiments evaluating Fe reduction and methanogenesis. Attempting to control the pH of soils could potentially introduce confounding effects. We used an MES buffer with 10 times the quantity we estimated from a soil titration and still saw shifts in the pH after incubation. With a high residual soil acidity, the amount of buffer needed to control soil pH may increase the ionic strength to a level that could influence cellular sorption to mineral and Fe oxide surfaces (Mills et al., 1994) as well as enzyme activity (Leprince and Quiquampoix, 1996).
In our experiment, we observed that organic amendments can increase CH4 production, particularly after extended anaerobic periods. We quantified CH4 production potential from several organic amendments and in a separate field experiment (unpublished) show that these results are useful in predicting field CH4 production. There is mounting concern that CH4 from restored and created wetlands may result in net global warming for decades to centuries (Neubauer, 2014). Our results suggest that not only do organic amendments increase CH4 gas production overall but also uncured amendments can decrease the time it takes before there is a large increase in both total gas production and CH4. Methane production is not constant and dramatically increases after several weeks. Because of this, it may be beneficial to report wetland CH4 data along with inundation duration, which can strongly affect CH4 (Hondula et al., 2021). It may be possible to limit CH4 in many wetland settings, particularly mitigation wetlands where hydrology is part of the design: shorter flooding or inundation durations with alternating drier conditions. This strategy has been proposed for rice paddy fields (Souza, 2021). Our lab study demonstrates the potential for significant CH4 emissions, but in a real system, methanotrophic activity could attenuate some of the emissions (Chowdhury and Dick, 2013); however, this would not decrease the overall C loss from soils, it only changes the pathway. If organic amendments are to be used, cured amendments may be preferable because they are not as prone to high CH4 generation and may attenuate Fe2+ toxicity. Amendments that lower the soil pH increase Fe reduction and limit methanogenesis (Marquart et al., 2019). When deciding whether or not to use organic amendments for wetland mitigation, consideration should be given to whether or not the material has been cured, the pH, the soil texture, and expected hydroperiod.
Significant data detail is available in the Supplement. Additional raw data are available upon request.
The supplement related to this article is available online at: https://doi.org/10.5194/bg-19-1151-2022-supplement.
BS and SAY designed the study. BS collected data and wrote the paper, with significant guidance, input, and editing from AHB.
The contact author has declared that neither they nor their co-authors have any competing interests.
Publisher's note: Copernicus Publications remains neutral with regard to jurisdictional claims in published maps and institutional affiliations.
William Buettner of the Maryland State Highway Administration inspired the idea for this work. We thank all members of the Baldwin/Yarwood lab for their assistance with the experimental setup and data collection.
This work was made possible by funding from the Maryland State Highway Administration (grant no. SHA/UM/4-53) and the Maryland Water Resources Research Center (grant no. 2017MD340B). Research was also supported by the U.S. Department of Agriculture (grant no. MD-ENST-7741).
This paper was edited by Andrew Thurber and reviewed by two anonymous referees.
Amendola, D., Mutema, M., Rosolen, V., and Chaplot, V.: Soil hydromorphy and soil carbon: A global data analysis, Geoderma, 324, 9–17, https://doi.org/10.1016/j.geoderma.2018.03.005, 2018.
Ballantine, K. A., Lehmann, J., Schneider, R. L., and Groffman, P. M.: Trade-offs between soil-based functions in wetlands restored with soil amendments of differing lability, Ecol. Appl., 25, 215–225, https://doi.org/10.1890/13-1409.1, 2015.
Batty, L. C.: Effects of External Iron Concentration upon Seedling Growth and Uptake of Fe and Phosphate by the Common Reed, Phragmites australis (Cav.) Trin ex. Steudel, Ann. Bot.-LOndon, 92, 801–806, https://doi.org/10.1093/aob/mcg205, 2003.
Berkowitz, J. F., Page, S., and Noble, C. V.: Potential Disconnect between Observations of Hydrophytic Vegetation, Wetland Hydrology Indicators, and Hydric Soils in Unique Pitcher Plant Bog Habitats of the Southern Gulf Coast, Southeast. Nat., 13, 721, https://doi.org/10.1656/058.013.0410, 2014.
Bethke, C. M., Sanford, R. A., Kirk, M. F., Jin, Q., and Flynn, T. M.: The thermodynamic ladder in geomicrobiology, Am. J. Sci., 311, 183–210, https://doi.org/10.2475/03.2011.01, 2011.
Blagodatsky, S., Blagodatskaya, E., Yuyukina, T., and Kuzyakov, Y.: Model of apparent and real priming effects: Linking microbial activity with soil organic matter decomposition, Soil Biol. Biochem., 42, 1275–1283, https://doi.org/10.1016/j.soilbio.2010.04.005, 2010.
Bräuer, S. L., Yavitt, J. B., and Zinder, S. H.: Methanogenesis in McLean Bog, an Acidic Peat Bog in Upstate New York: Stimulation by H2/CO2 in the Presence of Rifampicin, or by Low Concentrations of Acetate, Geomicrobiol. J., 21, 433–443, https://doi.org/10.1080/01490450490505400, 2004.
Bridgham, S. D., Cadillo-Quiroz, H., Keller, J. K., and Zhuang, Q.: Methane emissions from wetlands: biogeochemical, microbial, and modeling perspectives from local to global scales, Glob. Change Biol., 19, 1325–1346, https://doi.org/10.1111/gcb.12131, 2013.
Catrouillet, C., Davranche, M., Dia, A., Bouhnik-Le Coz, M., Marsac, R., Pourret, O., and Gruau, G.: Geochemical modeling of Fe(II) binding to humic and fulvic acids, Chem. Geol., 372, 109–118, https://doi.org/10.1016/j.chemgeo.2014.02.019, 2014.
Chen, X., Xu, Y., Gao, H., Mao, J., Chu, W., and Thompson, M. L.: Biochemical stabilization of soil organic matter in straw-amended, anaerobic and aerobic soils, Sci. Total Environ., 625, 1065–1073, https://doi.org/10.1016/j.scitotenv.2017.12.293, 2018.
Chowdhury, T. R. and Dick, R. P.: Ecology of aerobic methanotrophs in controlling methane fluxes from wetlands, Appl. Soil Ecol., 65, 8–22, https://doi.org/10.1016/j.apsoil.2012.12.014, 2013.
Dahl, T. E.: Status and Trends of Wetlands in the Conterminous United States 2004 to 2009, US Dep. Inter. Fish Wildl. Wash. DC, 108 pp., 2011.
Darrah, S. E., Shennan-Farpón, Y., Loh, J., Davidson, N. C., Finlayson, C. M., Gardner, R. C., and Walpole, M. J.: Improvements to the Wetland Extent Trends (WET) index as a tool for monitoring natural and human-made wetlands, Ecol. Indic., 99, 294–298, https://doi.org/10.1016/j.ecolind.2018.12.032, 2019.
Deng, H., Ye, Z. H., and Wong, M. H.: Lead, zinc and iron (Fe2+) tolerances in wetland plants and relation to root anatomy and spatial pattern of ROL, Environ. Exp. Bot., 65, 353–362, https://doi.org/10.1016/j.envexpbot.2008.10.005, 2009.
Drake, H. L., Horn, M. A., and Wüst, P. K.: Intermediary ecosystem metabolism as a main driver of methanogenesis in acidic wetland soil, Environ. Microbiol. Rep., 1, 307–318, https://doi.org/10.1111/j.1758-2229.2009.00050.x, 2009.
Ferry, J. G.: Methanosarcina acetivorans: A Model for Mechanistic Understanding of Aceticlastic and Reverse Methanogenesis, Front. Microbiol., 11, 1806, https://doi.org/10.3389/fmicb.2020.01806, 2020.
Fischer, S., McCarty, G., Ramirez, M., and Torrents, A.: Nitrogen release and leachable organic matter decomposition of anaerobically digested biosolids with variable pre-treatments, Waste Manage., 104, 82–93, https://doi.org/10.1016/j.wasman.2019.12.049, 2020.
Glissmann, K. and Conrad, R.: Saccharolytic activity and its role as a limiting step in methane formation during the anaerobic degradation of rice straw in rice paddy soil, Biol. Fert. Soils, 35, 62–67, https://doi.org/10.1007/s00374-002-0442-z, 2002.
Gray, A. L.: Redoximorphic Features Induced by Organic Amendments and Simulated Wetland Hydrology, Master's thesis, Univrersity of Maryland, College Park, 2010.
Green, H. E.: Use of theoretical and conceptual frameworks in qualitative research, Nurse Res., 21, 34–38, 2014.
Guo, X., Liu, H., and Wu, S.: Humic substances developed during organic waste composting: Formation mechanisms, structural properties, and agronomic functions, Sci. Total Environ., 662, 501–510, https://doi.org/10.1016/j.scitotenv.2019.01.137, 2019.
Hansel, C. M., Lentini, C. J., Tang, Y., Johnston, D. T., Wankel, S. D., and Jardine, P. M.: Dominance of sulfur-fueled iron oxide reduction in low-sulfate freshwater sediments, ISME J., 9, 2400–2412, https://doi.org/10.1038/ismej.2015.50, 2015.
Hondula, K. L., Jones, C. N., and Palmer, M. A.: Effects of seasonal inundation on methane fluxes from forested freshwater wetlands, Environ. Res. Lett., 16, 084016, https://doi.org/10.1088/1748-9326/ac1193, 2021.
Hu, Z., Liu, Y., Chen, G., Gui, X., Chen, T., and Zhan, X.: Characterization of organic matter degradation during composting of manure–straw mixtures spiked with tetracyclines, Bioresource Technol., 102, 7329–7334, https://doi.org/10.1016/j.biortech.2011.05.003, 2011.
Huang, B., Yu, K., and Gambrell, R. P.: Effects of ferric iron reduction and regeneration on nitrous oxide and methane emissions in a rice soil, Chemosphere, 74, 481–486, https://doi.org/10.1016/j.chemosphere.2008.10.015, 2009.
Hubbe, M. A., Nazhad, M., and Sánchez, C.: Composting as a Way to Convert Cellulosic Biomass and Organic Waste into High-Value Soil Amendments: A Review, BioResources, 5, 2808–2854, 2010.
Jabłoński, S., Rodowicz, P., and Łukaszewicz, M.: Methanogenic archaea database containing physiological and biochemical characteristics, Int. J. Syst. Evol. Microbiol., 65, 1360–1368, https://doi.org/10.1099/ijs.0.000065, 2015.
Keiluweit, M., Wanzek, T., Kleber, M., Nico, P., and Fendorf, S.: Anaerobic microsites have an unaccounted role in soil carbon stabilization, Nat. Commun., 8, 1771, https://doi.org/10.1038/s41467-017-01406-6, 2017.
Kim, S., Lee, J., Lim, J., Shinog, Y., Lee, C., and Oh, T.: Comparison of Methane Emissions on Soil Texture in Korean Paddy Fields, J. Fac. Agric. Kyushu Univ., 63, 393–397, https://doi.org/10.5109/1955660, 2018.
Kirk, G.: The Biogeochemistry of Submerged Soils, 1st edn., Wiley, West Sussex, England, https://doi.org/10.1002/047086303X, 2004.
Kuehn, K. A., Ohsowski, B. M., Francoeur, S. N., and Neely, R. K.: Contributions of fungi to carbon flow and nutrient cycling from standing dead Typha angustifolia leaf litter in a temperate freshwater marsh, Limnol. Oceanogr., 56, 529–539, https://doi.org/10.4319/lo.2011.56.2.0529, 2011.
Lalonde, K., Mucci, A., Ouellet, A., and Gélinas, Y.: Preservation of organic matter in sediments promoted by iron, Nature, 483, 198–200, https://doi.org/10.1038/nature10855, 2012.
Lehmann, J. and Kleber, M.: The contentious nature of soil organic matter, Nature, https://doi.org/10.1038/nature16069, 2015.
Lentini, C. J., Wankel, S. D., and Hansel, C. M.: Enriched iron (III)-reducing bacterial communities are shaped by carbon substrate and iron oxide mineralogy, Front. Microbiol., 3, 2012.
Leprince, F. and Quiquampoix, H.: Extracellular enzyme activity in soil: effect of pH and ionic strength on the interaction with montmorillonite of two acid phosphatases secreted by the ectomycorrhizal fungus Hebeloma cylindrosporum, Eur. J. Soil Sci., 47, 511–522, https://doi.org/10.1111/j.1365-2389.1996.tb01851.x, 1996.
Maietta, C. E., Hondula, K. L., Jones, C. N., and Palmer, M. A.: Hydrological Conditions Influence Soil and Methane-Cycling Microbial Populations in Seasonally Saturated Wetlands, Front. Environ. Sci., 8, 593942, https://doi.org/10.3389/fenvs.2020.593942, 2020a.
Maietta, C. E., Monsaint-Queeney, V., Wood, L., Baldwin, A. H., and Yarwood, S. A.: Plant litter amendments in restored wetland soils altered microbial communities more than clay additions, Soil Biol. Biochem., 147, 107846, https://doi.org/10.1016/j.soilbio.2020.107846, 2020b.
Marquart, K. A., Haller, B. R., Paper, J. M., Flynn, T. M., Boyanov, M. I., Shodunke, G., Gura, C., Jin, Q., and Kirk, M. F.: Influence of pH on the balance between methanogenesis and iron reduction, Geobiology, 17, 185–198, https://doi.org/10.1111/gbi.12320, 2019.
Mazzilli, S. R., Kemanian, A. R., Ernst, O. R., Jackson, R. B., and Piñeiro, G.: Greater humification of belowground than aboveground biomass carbon into particulate soil organic matter in no-till corn and soybean crops, Soil Biol. Biochem., 85, 22–30, https://doi.org/10.1016/j.soilbio.2015.02.014, 2015.
McMahon, S. K., Williams, M. A., Bottomley, P. J., and Myrold, D. D.: Dynamics of Microbial Communities during Decomposition of Carbon-13 Labeled Ryegrass Fractions in Soil, Soil Sci. Soc. Am. J., 69, 1238, https://doi.org/10.2136/sssaj2004.0289, 2005.
Megonigal, J. P., Hines, M. E., and Visscher, P. T.: Anaerobic Metabolism: Linkages to Trace Gases and Aerobic Processes, in: Biogeochemistry, edited by: Schlesinger, W. H., Elsevier-Pergamon, Oxford, UK., 317–424, 2004.
Mikutta, R., Kleber, M., Torn, M. S., and Jahn, R.: Stabilization of Soil Organic Matter: Association with Minerals or Chemical Recalcitrance?, Biogeochemistry, 77, 25–56, https://doi.org/10.1007/s10533-005-0712-6, 2006.
Mills, A. L., Herman, J. S., Hornberger, G. M., and DeJesús, T. H.: Effect of Solution Ionic Strength and Iron Coatings on Mineral Grains on the Sorption of Bacterial Cells to Quartz Sand, Appl. Environ. Microbiol., 60, 3300–3306, https://doi.org/10.1128/AEM.60.9.3300-3306.1994, 1994.
Mitsch, W. J., Bernal, B., and Hernandez, M. E.: Ecosystem services of wetlands, Int. J. Biodivers. Sci. Ecosyst. Serv. Manag., 11, 1–4, https://doi.org/10.1080/21513732.2015.1006250, 2015.
Müller, C., Kuki, K. N., Pinheiro, D. T., de Souza, L. R., Silva, A. I. S., Loureiro, M. E., Oliva, M. A., and Almeida, A. M.: Differential physiological responses in rice upon exposure to excess distinct iron forms, Plant Soil, 16, 2015.
Muggeo, V. M. R.: segmented: An R Package to Fit Regression Models with Broken-Line Relationships, R News, 8, 20–25, 2008.
Neubauer, S. C.: On the challenges of modeling the net radiative forcing of wetlands: reconsidering Mitsch et al. 2013, Landscape Ecol., 29, 571–577, https://doi.org/10.1007/s10980-014-9986-1, 2014.
Neubauer, S. C. and Verhoeven, J. T. A.: Wetland effects on global climate: mechanisms, impacts, and management recommendations, in: Wetlands: ecosystem services, restoration and wise use, edited by: An, S. and Verhoeven, J. T. A., Springer, Cham, Springer, 39–62, 2019.
Noble, R. and Roberts, S. J.: Eradication of plant pathogens and nematodes during composting: a review, Plant Pathol., 53, 548–568, https://doi.org/10.1111/j.0032-0862.2004.01059.x, 2004.
Nottingham, A. T., Griffiths, H., Chamberlain, P. M., Stott, A. W., and Tanner, E. V. J.: Soil priming by sugar and leaf-litter substrates: A link to microbial groups, Appl. Soil Ecol., 42, 183–190, https://doi.org/10.1016/j.apsoil.2009.03.003, 2009.
Ott, E. T., Galbraith, J. M., Daniels, W. L., and Aust, W. M.: Effects of amendments and microtopography on created tidal freshwater wetland soil morphology and carbon, Soil Sci. Soc. Am. J., 84, 638–652, https://doi.org/10.1002/saj2.20057, 2020.
Paustian, K., Lehmann, J., Ogle, S., Reay, D., Robertson, G. P., and Smith, P.: Climate-smart soils, Nature, 532, 49–57, https://doi.org/10.1038/nature17174, 2016.
Pereira, E. G., Oliva, M. A., Siqueira-Silva, A. I., Rosado-Souza, L., Pinheiro, D. T., and Almeida, A. M.: Tropical Rice Cultivars from Lowland and Upland Cropping Systems Differ in Iron Plaque Formation, J. Plant Nutr., 37, 1373–1394, https://doi.org/10.1080/01904167.2014.888744, 2014.
Poggenburg, C., Mikutta, R., Schippers, A., Dohrmann, R., and Guggenberger, G.: Impact of natural organic matter coatings on the microbial reduction of iron oxides, Geochim. Cosmochim. Acta, 224, 223–248, https://doi.org/10.1016/j.gca.2018.01.004, 2018.
Poulton, S. W. and Canfield, D. E.: Development of a sequential extraction procedure for iron: implications for iron partitioning in continentally derived particulates, Chem. Geol., 214, 209–221, https://doi.org/10.1016/j.chemgeo.2004.09.003, 2005.
Prakash, D., Chauhan, S. S., and Ferry, J. G.: Life on the thermodynamic edge: Respiratory growth of an acetotrophic methanogen, Sci. Adv., 5, eaaw9059, https://doi.org/10.1126/sciadv.aaw9059, 2019.
Richardson, C. J., Bruland, G. L., Hanchey, M. F., and Sutton-Grier, A. E.: Soil Restoration: The Foundation of Successful Wetland Reestablishment, Chapter 19, in: Wetland Soils: Genesis, Hydrology, Landscapes, and Classification, vol. Chapter 19, edited by: Vepraskas, M. J. and Craft, C. B., CRC Press, 469–493, 2016.
Saaltink, R. M., Dekker, S. C., Eppinga, M. B., Griffioen, J., and Wassen, M. J.: Plant-specific effects of iron-toxicity in wetlands, Plant Soil, 416, 83–96, https://doi.org/10.1007/s11104-017-3190-4, 2017.
Saidpullicino, D., Erriquens, F., and Gigliotti, G.: Changes in the chemical characteristics of water-extractable organic matter during composting and their influence on compost stability and maturity, Bioresource Technol., 98, 1822–1831, https://doi.org/10.1016/j.biortech.2006.06.018, 2007.
Scott, B., Baldwin, A. H., Ballantine, K., Palmer, M., and Yarwood, S.: The role of organic amendments in wetland restorations, Restor. Ecol., 28, 776–784, https://doi.org/10.1111/rec.13179, 2020.
Shimizu, M., Zhou, J., Schröder, C., Obst, M., Kappler, A., and Borch, T.: Dissimilatory Reduction and Transformation of Ferrihydrite-Humic Acid Coprecipitates, Environ. Sci. Technol., 47, 13375–13384, https://doi.org/10.1021/es402812j, 2013.
Sivan, O., Shusta, S. S., and Valentine, D. L.: Methanogens rapidly transition from methane production to iron reduction, Geobiology, 14, 190–203, https://doi.org/10.1111/gbi.12172, 2016.
Souza, R.: Optimal drainage timing for mitigating methane emissions from rice paddy fields, Geoderma, 9, 2021.
Straub, K. L., Benz, M., and Schink, B.: Iron metabolism in anoxic environments at near neutral pH, FEMS Microbiol. Ecol., 34, 181–186, https://doi.org/10.1111/j.1574-6941.2001.tb00768.x, 2001.
Wang, Z. P., DeLaune, R. D., Patrick, W. H., and Masscheleyn, P. H.: Soil Redox and pH Effects on Methane Production in a Flooded Rice Soil, Soil Sci. Soc. Am. J., 57, 382, https://doi.org/10.2136/sssaj1993.03615995005700020016x, 1993.
Watanabe, K., Nishiuchi, S., Kulichikhin, K., and Nakazono, M.: Does suberin accumulation in plant roots contribute to waterlogging tolerance?, Front. Plant Sci., 4, https://doi.org/10.3389/fpls.2013.00178, 2013.
Willson, K. G., Perantoni, A. N., Berry, Z. C., Eicholtz, M. I., Tamukong, Y. B., Yarwood, S. A., and Baldwin, A. H.: Influences of reduced iron and magnesium on growth and photosynthetic performance of Phragmites australis subsp. americanus (North American common reed), Aquat. Bot., 137, 30–38, https://doi.org/10.1016/j.aquabot.2016.11.005, 2017.
Winton, R. S. and Richardson, C. J.: The Effects of Organic Matter Amendments on Greenhouse Gas Emissions from a Mitigation Wetland in Virginia's Coastal Plain, Wetlands, 35, 969–979, https://doi.org/10.1007/s13157-015-0674-y, 2015.
Yagi, K. and Minami, K.: Effect of organic matter application on methane emission from some Japanese paddy fields, Soil Sci. Plant Nutr., 36, 599–610, https://doi.org/10.1080/00380768.1990.10416797, 1990.
Yang, W. H., McNicol, G., Teh, Y. A., Estera-Molina, K., Wood, T. E., and Silver, W. L.: Evaluating the Classical Versus an Emerging Conceptual Model of Peatland Methane Dynamics: Peatland Methane Dynamics, Glob. Biogeochem. Cy., 31, 1435–1453, https://doi.org/10.1002/2017GB005622, 2017.
Yao, H. and Conrad, R.: Thermodynamics of methane production in different rice paddy soils from China, the Philippines and Italy, Soil Biol. Biochem., 31, 463–473, 1999.
Yarwood, S. A.: The role of wetland microorganisms in plant-litter decomposition and soil organic matter formation: a critical review, FEMS Microbiol. Ecol., 94, 1–17, https://doi.org/10.1093/femsec/fiy175, 2018.
Ye, R., Jin, Q., Bohannan, B., Keller, J. K., McAllister, S. A., and Bridgham, S. D.: pH controls over anaerobic carbon mineralization, the efficiency of methane production, and methanogenic pathways in peatlands across an ombrotrophic–minerotrophic gradient, Soil Biol. Biochem., 54, 36–47, https://doi.org/10.1016/j.soilbio.2012.05.015, 2012.
Zhuang, L., Xu, J., Tang, J., and Zhou, S.: Effect of ferrihydrite biomineralization on methanogenesis in an anaerobic incubation from paddy soil: Iron Mineralization and Methanogenesis, J. Geophys. Res.-Biogeo., 120, 876–886, https://doi.org/10.1002/2014JG002893, 2015.