the Creative Commons Attribution 4.0 License.
the Creative Commons Attribution 4.0 License.
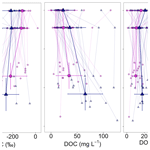
Age and chemistry of dissolved organic carbon reveal enhanced leaching of ancient labile carbon at the permafrost thaw zone
Heather M. Throckmorton
Jeffrey M. Heikoop
Brent D. Newman
Alexandra L. Hedgpeth
Marisa N. Repasch
Thomas P. Guilderson
Cathy J. Wilson
Climate change will alter the balance between frozen and thawed conditions in Arctic systems. Increased temperatures will make the extensive northern permafrost carbon stock vulnerable to decomposition and translocation. Production, cycling, and transport of dissolved organic carbon (DOC) are crucial processes for high-latitude ecosystem carbon loss that result in considerable export off the Arctic landscape. To identify where and under what conditions permafrost DOC is mobilized in an Arctic headwater catchment, we measured radiocarbon (14C) of DOC and assessed DOC composition with ultraviolet–visible spectroscopy (UV–Vis) of surface waters and shallow and deep subsurface porewaters from 17 drainages in the Barrow Environmental Observatory in Alaska. Samples were collected in July and September 2013 to assess changes in age and chemistry of DOC over time. DOC age was highly variable ranging from modern (19 ‰ Δ14C) to approximately 7000 BP (−583 ‰ Δ14C). DOC age increased with depth, over the summer as the active layer deepened, and with increasing drainage size. DOC quality indicators reflected a DOC source rich in high molecular-weight and aromatic compounds, characteristics consistent with vegetation-derived organic matter that had undergone little microbial processing, throughout the summer and a weak relationship with DOC age. In deep porewaters, DOC age was also correlated with several biogeochemical indicators (including dissolved methane concentration, δ13C, and the apparent fractionation factor), suggesting a coupling between carbon and redox biogeochemistry influencing methane production. In the drained thawed lake basins included in this study, DOC concentrations and contributions of vegetation-derived organic matter declined with increasing basin age. The weak relationship between DOC age and chemistry and consistency in DOC chemical indicators over the summer suggest a high lability of old DOC released by thawing permafrost.
- Article
(4920 KB) - Full-text XML
-
Supplement
(390 KB) - BibTeX
- EndNote
Soils of the northern permafrost region store approximately 1300 Pg of organic carbon (Hugelius et al., 2014). This extensive carbon pool is vulnerable to climate change as warmer temperatures increase thawing, microbial decomposition, fire frequency, and erosion (Schuur et al., 2013, 2008). Loss of this permafrost carbon to the atmosphere poses a considerable potential feedback to climate change (Schaefer et al., 2014; Koven et al., 2011), with an estimated 5 %–15 % of permafrost soil carbon projected to be released to the atmosphere by the end of this century under the current warming trajectory (Schuur et al., 2015).
Carbon mobilized from thawing permafrost and warmer active-layer soils may be microbially transformed into carbon dioxide (CO2) or methane (CH4), which can be emitted directly to the atmosphere (Schadel et al., 2016; Hicks Pries et al., 2013). Less well-studied than these direct fluxes to the atmosphere (Vonk et al., 2019), dissolved organic carbon (DOC) provides the carbon source for microbes and the subsequent production of gaseous fluxes (Charman et al., 1999; Chanton et al., 2008; Molot and Dillon, 1997; Corbett et al., 2013; Shirokova et al., 2013). Crucially, DOC also has the potential to be transported, potentially off the landscape through drainages, streams, and ultimately export to the ocean (Raymond et al., 2007; Holmes et al., 2011; Cole et al., 2007). Lateral transport of dissolved carbon is a crucial mechanism for terrestrial carbon loss in the Arctic and results in an export off the landscape of up to 25 % of net ecosystem productivity (calculated from Mcguire et al., 2009), may exceed net ecosystem exchange of carbon in some northern ecosystems (Aurela et al., 2002; Billett et al., 2004; Christensen et al., 2007; Roulet et al., 2007), and at the global scale is comparable in magnitude to the land and ocean CO2 sinks (Drake et al., 2018a; Tank et al., 2018).
DOC accounts for much of this carbon loss (Billett et al., 2004; Mcguire et al., 2009; McClelland et al., 2016), and several studies have reported ancient radiocarbon ages for DOC in rivers, including 2000–500 BP in erosion-impacted streams in the Kolyma River basin (Mann et al., 2015) to over 20 000 BP in streams freshly formed from thawing ice-rich Yedoma permafrost (Vonk et al., 2013). Furthermore, laboratory incubation experiments show that a substantial portion of this DOC (20 %–50 %) is labile (Mann et al., 2012, 2015; Holmes et al., 2008; Liu et al., 2019) and as such could be decomposed and released back to the atmosphere as CO2 or CH4 from soils, surface waters, or drainages (Kling et al., 1991; Cole et al., 2007; Drake et al., 2015). In fact, increasing terrestrial DOC loads have been linked to increased CO2 emissions from aquatic systems (Lapierre et al., 2013), although field studies suggest biological activity in Arctic aquatic and anoxic systems may be fueled largely by modern C (Dean et al., 2020; Estop-Aragonés et al., 2020; Tanentzap et al., 2021).
The combination of thawing permafrost, vegetation changes, increased snowfall, and subsequent snowmelt is likely to result in an increase in the role of DOC as a mode of carbon transport within and across the Arctic landscape (Frey, 2005; Finlay et al., 2006; Kawahigashi et al., 2004). How the production and fate of terrigenous DOC will respond to future climate change is not well constrained. Studies suggest that increasing temperature increases DOC production along with soil carbon decomposition (Neff and Hooper, 2002; Freeman et al., 2001). Increased DOC production is also expected as permafrost thaws, active layers deepen, and shrub encroachment occurs (Frey and McClelland, 2009). However, numerous studies have shown that the fate of DOC is highly dependent on hydrology (Pastor et al., 2003; Kellerman et al., 2019; Raymond et al., 2016). Specifically, in wet years or in cases where hydrologic flow paths facilitate lateral movement of DOC, warming may increase DOC production, loss from terrestrial ecosystems, and export to surface waters (Freeman et al., 2001). This seems to be the current case for the Arctic as DOC export by streams and rivers largely occurs during the increase in streamflow associated with snowmelt, implying that DOC transport and production is water, not carbon, limited (Finlay et al., 2006; Raymond et al., 2007; Guo and Macdonald, 2006; Guo et al., 2007; Townsend-Small et al., 2011; Prokushkin et al., 2011). However, in drier conditions or when flow to surface waters is slow, DOC produced in soil and peat may be decomposed in situ, increasing soil and ecosystem CO2 and CH4 fluxes to the atmosphere (Pastor et al., 2003; Estop-Aragonés et al., 2020). Alternatively, DOC may move down the soil profile where it may interact with mineral soils and be sorbed, stabilized, or remineralized to CO2 or CH4 (Fan et al., 2010; Frey and McClelland, 2009) and could provide a priming effect for decomposition of old carbon stores (Fan et al., 2010; Hayes et al., 2014; Wild et al., 2014).
Radiocarbon analyses and characterization of DOC provide insights into the age of sources, transfer, and lability of DOC from Arctic and subarctic ecosystems (Olefeldt and Roulet, 2012; Benner et al., 2004; Raymond et al., 2007; Guo and Macdonald, 2006; Amon and Meon, 2004) and can therefore provide crucial information about mechanisms behind carbon loss and transport that is not currently captured appropriately in high-latitude models. Large stores of inert yet labile permafrost carbon have, as a function of age, a unique 14C isotopic signature that follows the carbon into the DOC pool. Soil C age typically increases with depth and so deepening flow paths associated with seasonal thaw may be reflected in the 14C age of DOC downstream. For example, inland headwater systems showed an increase in the age of DOC seasonally as deepening of the active layer mobilized older soil C (Dean et al., 2018). In Arctic rivers, seasonal patterns of DOC loadings and 14C age of DOC exhibited a nearly consistent pattern with high concentrations of young (i.e., recently fixed) DOC following spring thaw and slightly lower concentrations of DOC with an older 14C age associated with baseflow (Guo and Macdonald, 2006; Guo et al., 2007; Raymond et al., 2007; Striegl et al., 2007; Neff et al., 2006; Amon et al., 2012; Wild et al., 2019) Very high concentrations of old DOC (≥ 20 ky) have been observed in streams with drainage basins encompassing thawing Yedoma permafrost, demonstrating that actively thawing systems are, in fact, releasing large amounts of old, previously frozen carbon (Vonk et al., 2013).
The molecular chemistry and biolability of DOC also change seasonally, at least in large Arctic streams and rivers. For example, in large Arctic rivers, assessments of DOC optical properties (by UV–Vis absorbance) have indicated higher aromaticity, lignin phenol concentrations, and molecular weights associated with high flows during snowmelt (Spencer et al., 2008; Mann et al., 2016) and declines in aromaticity between summer and winter (O'Donnell et al., 2012; Striegl et al., 2007), suggesting a larger contribution of terrestrial carbon at freshet as these indicators have been attributed to terrestrial carbon inputs rich in vegetation-derived compounds that have undergone limited microbial processing (Spencer et al., 2008). A similar decline in aromaticity of DOC in the Kolyma River and its tributaries coincided with a decline in DOC 14C values from spring to late summer, indicating that earlier in the thaw season DOC was sourced from younger carbon derived from terrestrial vegetation and litter or from unprocessed soil organic matter (Neff et al., 2006). Furthermore, results of a pan-Arctic study of DOC biodegradability assessed with incubations observed declines in biodegradability of DOC in large streams and rivers over the year, but the biolability of DOC in small streams and soil leachates was consistent over the year (Vonk et al., 2015a). However, seasonal studies of DOC chemistry and biolability in Arctic headwater catchments and small watersheds are largely missing from the literature.
Watershed characteristics and the extent of permafrost degradation also impact DOC content and biolability. For example, in Western Siberia thaw lakes, high molecular-weight compounds, also indicative of unprocessed terrestrially derived C, as well as DOC concentrations declined across a chronosequence of lake development (Pokrovsky et al., 2011). In drained thaw lake basins (DTLBs) in the Coastal Plain of Alaska, organic layer thickness and a degree of soil organic matter decomposition increased with increasing DTLB age (Hinkel et al., 2003; Bockheim et al., 2004). These trends are likely to translate to changes in the lability and flux of DOC with lake and basin development. Studies across gradients in permafrost thaw found that rivers in areas with continuous permafrost had lower DOC concentrations than those in areas with discontinuous permafrost (Johnston et al., 2021; Prokushkin et al., 2011). Areas with more continuous permafrost had lower aromaticity than those undergoing more extensive thaw in the Central Siberian Plateau (Prokushkin et al., 2011), and in the North Slope of Alaska, rivers in watersheds with continuous permafrost had DOC with higher amounts of aliphatic compounds (indicative of microbial processing), while watersheds with more extensive permafrost thaw yielded DOC with characteristics indicative of unprocessed vegetation-derived organic matter including higher relative amounts of aromatics, polyphenols, and lignin (Johnston et al., 2021). In discontinuous permafrost in interior Alaska, streams in watersheds with more permafrost had higher DOC and dissolved organic nitrogen (DON) concentrations, higher DOC:DON ratios, and higher aromaticity than streams in areas with less permafrost, suggesting that extensive permafrost degradation resulted in lower fluxes composed of more processed, lower-quality dissolved organic matter (Balcarczyk et al., 2009). In pan-Arctic laboratory extraction and incubation experiments, soils under continuous permafrost produced more DOC and DOC that was more biolabile than soils from areas with discontinuous permafrost, suggesting that soils under discontinuous permafrost are actively losing labile carbon to aquatic systems as a result of increasing thaw (Vonk et al., 2015a). Comparisons of DOC biolability and chemistry across fluvial networks indicate that DOC from soil leachates and small streams (including thaw and headwater streams) is more biolabile than DOC in larger streams and rivers (Vonk et al., 2015a; Mann et al., 2015). Furthermore, this very labile DOC derived from thawing permafrost is also enriched in aromatics, and the permafrost-derived DOC entering thaw streams and headwaters preferentially supports microbial demand as waters move to larger rivers (Mann et al., 2015).
The information gained from stream water samples is valuable, but these streams integrate processes across the landscape and do not provide information about the specific locations within their watersheds where older or more labile C is being mobilized, limiting our ability to attribute these observations to processes. Yet, small watersheds and headwater streams remain understudied relative to large river basins (Vonk et al., 2015b; Shogren et al., 2019) even though these headwater catchments release DOC that is older and more biodegradable than larger rivers further along fluvial networks (Vonk et al., 2015a; Coch et al., 2019; Drake et al., 2018b; Textor et al., 2019). To bring us closer to identifying the conditions and processes influencing the production and transport of permafrost-derived DOC in headwater Arctic catchments, we measured DOC age (14C) and chemistry of surface waters and soil porewaters in a survey of 17 drainages with different characteristics in the Barrow Environmental Observatory, Alaska. We integrate these new data (14C of DOC and UV–Vis) with previously published biogeochemical tracers from the same sampling campaign (Throckmorton et al., 2015b) to elucidate the mechanisms behind DOC cycling across these drainages. This approach addresses some of the current challenges in this area of research by providing field implementation of biogeochemical tracers that can be used to infer changes in DOC cycling (Vonk et al., 2019). We hypothesized that in most drainages, DOC would become older and more enriched in aromatics and high molecular-weight compounds over the course of the summer as active layers deepened, exposing permafrost organic matter that had not been microbially processed. We also expected to find spatial patterns in DOC age and chemistry reflecting differences in drainage characteristics such as a shift toward DOC sourced from older, more processed soil organic matter in older drained thaw lake basins as well as with increasing catchment size.
2.1 Study area and field sampling
The study area and sample collection have been described previously (Throckmorton et al., 2015b). Briefly, surface and porewater samples were collected in July and September of 2013 from 17 watershed drainages in and around the Barrow Environmental Observatory on the Arctic Coastal Plain near Utqiaġvik, Alaska (71.3∘ N, 156.6∘ W, Fig. 1). Sampling locations were along the periphery of drainages that included drained thaw lake basins of differing age, interlake basin areas, and different types of polygonal terrain. Sampling locations are further classified by water flow as either “stagnant” wetlands (no observable flow during sampling) or “gentle flow” (channels with gentle lateral surficial flow observed during sampling), although samples were collected from areas that appeared stagnant prior to sampling. Samples were collected from three depths at each location: surface water, shallow subsurface soil porewater, and deep subsurface soil porewater. Grab samples were collected from surface waters at the edges of drainages. Shallow porewaters were collected with stainless-steel drive points from 7.5–15 cm below the surface, which was typically organic soil. Deep porewaters were collected with macro-rhizon samplers from the maximum depth to the frost table, which ranged from 27–64 cm below the surface and varied across sites and sampling month (34 cm in July and 43 cm in September, on average). Samples were filtered in the field through 0.45 or 0.2 µm Fisherbrand nylon syringe filters, depending on the analyte of interest (see below).
2.2 Surface and soil porewater measurements
The chemistry of DOC was assessed based on spectral properties with UV–Vis absorbance (of samples filtered in the field through 0.45 µm Fisher syringe filters) at room temperature (quartz tube; 1 cm path) at Los Alamos National Laboratory. Absorbance at 254, 350, and 440 nm were selected as spectral wavelengths of interest as they have been shown to correlate strongly with DOC concentration in rivers, particularly in those dominated by terrestrial organic matter inputs (Spencer et al., 2012). Absorption coefficients were calculated following Eq. (1):
where Aλ is spectral absorbance at a specific wavelength (λ) and l is the cell path length in meters. We observed that absorption coefficients for all three wavelengths of interest positively correlated with DOC concentration (p<0.01). Therefore, we weighted the absorbance coefficients (from Eq. 1) by DOC concentration (in mg L−1) for further statistical analysis of carbon-specific ultraviolet absorbance (SUVA) in L mg C−1 m−1 for the targeted wavelengths as has been done by others previously (Weishaar et al., 2003). Some samples had an absorbance less than the blank at 350 and 440 nm and were set to zero for further statistical analysis. SUVA values for three samples with DOC concentrations below 3 mg L−1 were removed as outliers because low DOC concentrations yielded very high SUVA values (1–2 orders of magnitude higher than the other samples).
Of the 102 total water samples collected, 80 had enough sample (0.45 µm filtered and stored in amber glass vials) after other analyses to send to Lawrence Livermore National Laboratory for radiocarbon analysis. Samples were freeze-dried, treated with 1 N HCl at 70 ∘C to remove residual carbonate, and dried at 70 ∘C to remove acid without rinsing. Samples were then combusted to CO2 and reduced to graphite onto Fe powder in the presence of H2 (Vogel et al., 1984). Measured δ13C values were used to correct for mass-dependent fractionation and 14C values are reported as Δ14C (‰) corrected to the year of measurement, i.e., either 2016 or 2017 (Stuiver and Polach, 1977).
A suite of biogeochemical indicators was analyzed on these samples with results presented previously (Throckmorton et al., 2015b). These analytes add to the interpretation of the new data presented in this paper (14C of DOC and SUVA). Briefly, samples were analyzed in the field for Fe2+, temperature, dissolved oxygen, and pH. Samples were analyzed at Los Alamos National Laboratory for concentrations and δ13C of dissolved CH4 and dissolved inorganic carbon (DIC) (from samples filtered in the field through 0.2 µm Fisher syringe filters), for concentrations and δ13C or δ15N of DOC and DON (from samples filtered in the field through 0.45 µm Fisher syringe filters), and the major cations and trace metals (for more, see Throckmorton et al., 2015a, b).
2.3 Statistical analysis
Statistical analyses were performed in R v. 3.614 (R Core Team, 2019) at α=0.05 significance level for analyses of the entire dataset. Analyses for subsets that resulted in limited sample size (e.g., analysis of individual porewater depths or analysis of only the DTLB drainages) were performed at α=0.1, as noted in the text. Pearson correlation analysis was used to assess dependence among DOC variables and other geochemical indicators using the Hmisc package (Harrell, 2019). Depth and sampling month effects were tested using mixed-effect models with repeated measures for depth and time using the nlme package (Pinheiro et al., 2019). When present, interaction effects were investigated using the Phia package (De Rosario-Martinez, 2015). We evaluated the role of site characteristics in driving DOC characteristics using stepwise linear regression with the MASS package (Venables and Ripley, 2002), which evaluates alternate regression models based on Akaike information criteria (AIC). Results are reported as means followed by standard deviations.
3.1 Temporal and depth patterns of DOC age and chemistry
DOC Δ14C values ranged from 19‰ to −583‰ or from an average conventional radiocarbon age of modern to 7000 BP, and decreased from July to September (p<0.01, Fig. 2a), consistent with a decline in DOC 14C values from spring through late summer and fall reported for Arctic headwater catchments (Dean et al., 2018) as well as Arctic rivers and their tributaries (Neff et al., 2006; Wild et al., 2019). DOC Δ14C also declined with depth, consistent with declines in 14C values in soil pore-space CO2 with depth and from summer to fall in locations in our study area (Vaughn and Torn, 2018). DOC concentrations increased from July to September in deep porewater (p<0.01) and increased with depth in September (p<0.01, not significant, NS, in July, Fig. 2b). DOC concentration and 14C values were not correlated to one another. Interestingly, the DOC:DON ratio did not change with depth but did increase from July to September (p=0.01, Fig. 2c) – an indication that dissolved organic matter (DOM) quality or source may have shifted over the summer. DON increased with depth (p<0.01) but did not change between July and September (Fig. 2d). An increase in microbially derived or highly processed organic matter should have included an increase in DON and a decrease in DOC:DON ratio as microbially derived organic matter has a lower C:N ratios than plant litter and soil organic matter (Cleveland and Liptzin, 2007) and C:N ratios decline with increased microbial processing of litter and soil organic matter (Lavallee et al., 2020; Moore et al., 2011). Thus, these patterns are consistent with increased mobilization of unprocessed organic matter from old permafrost as the thaw table deepened from an average of 34 cm in July to 43 cm in September.
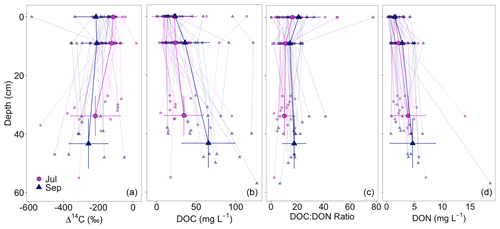
Figure 214C of DOC (a), DOC concentration (b), DOC:DON ratio by mass (g:g) (c), and DON concentration (d) by depth. Large symbols are means ± SD. DOC and DON concentration data were presented previously Throckmorton et al. (2015b).
We further assessed the chemistry of DOC with UV–Vis absorbance at 254, 350, and 440 nm to identify trends in DOC chemical composition over the sampling period. We targeted these wavelengths because absorption increases with increasing aromaticity at 254 nm (Weishaar et al., 2003), increasing lignin phenol content at 350 nm (Spencer et al., 2008; Mann et al., 2016), and increasing molecular size at 440 nm (Yacobi et al., 2003). We found that absorbances at all three wavelengths were positively correlated with DOC and DON concentrations (Figs. S1 and S2 in the Supplement). Averaged across depths, the absorbance coefficient at 440 nm increased from 10 m−1 in July to 20 m−1 in September (p=0.03), consistent with a shift toward a more plant-derived source, but this difference was not significant when weighted by DOC concentration (SUVA440, p=0.09, Fig. 3c). SUVA350 increased with depth (p=0.03, Fig. 3b), suggesting relatively higher lignin phenols at the thaw table where DOC was produced from actively thawing permafrost. Sampling month and depth effects on SUVA254 were not statistically significant. However, correlation analyses suggested SUVA350 increased with increasing DON concentration (ρ=0.26, p=0.01) and SUVA254 increased with decreasing DOC:DON ratio (, p<0.01). Surprisingly, across the entire dataset, none of the SUVA indicators were correlated with DOC 14C (Fig. S3 in the Supplement).
We observed SUVA254 values that are generally higher than those reported for Arctic rivers and streams, which tend to be less than 5 L mg C−1 m−1 (O'Donnell et al., 2012; Spencer et al., 2008; Neff et al., 2006; Drake et al., 2018b), and within the range of those reported for soil leachates from elsewhere in northern Alaska (Gao et al., 2018; Whittinghill et al., 2014). Comparable SUVA350 and SUVA440 for Arctic terrestrial or aquatic systems are not available in the literature, but in general, the absorption coefficients at these wavelengths observed in this study (Figs. S1 and S2) are also higher than those reported for streams and rivers (Spencer et al., 2012, 2008; Mann et al., 2016). Our observed patterns reflect a DOC source that is high in plant-derived, aromatic, phenolic, and high molecular-weight compounds throughout the summer. These trends likely reflect in situ production of DOC at depth from thawing permafrost that has not previously undergone microbial processing and may be biolabile because DOC biolability (the amount of DOC lost during incubation) has been shown to be positively correlated to SUVA254 (Mann et al., 2015).
Other biogeochemical indicators (previously published by Throckmorton et al., 2015b) also changed with sampling depth, consistent with other research on active layer hydrogeochemistry in the area (Newman et al., 2015). Specifically, concentrations of dissolved inorganic carbon (DIC), methane, Fe2+, and Fe3+ all increased with depth, while dissolved oxygen (DO) decreased with depth – trends that were attributed to increased availability and use of iron as a terminal electron acceptor in deeper mineral soils (Throckmorton et al., 2015b). Because many of the biogeochemical indicator species changed with depth, the relationships between these variables and DOC characteristics were further assessed separately by sampling depth (surface, shallow, or deep) with an alpha value of 0.1 to account for reduced sample size. In surface and shallow porewaters, both DOC Δ14C values and CH4 concentrations decreased from July to September (Fig. 4a, p=0.04), but DOC Δ14C values were not correlated with any of the other measured variables.
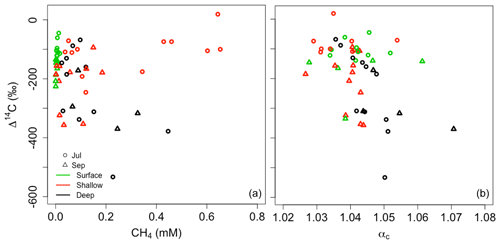
Figure 4DOC 14C (reported in this study) versus dissolved methane concentration (a) and the apparent fractionation factor for methanogenesis, αc (b). Methane concentration, δ13C, and αc data were presented previously in Throckmorton et al. (2015b).
In contrast, deep porewater DOC Δ14C was negatively correlated with DON (, n=24, p=0.06), SUVA350 (, n=24, p=0.06), DIC (, n=21, p=0.06), Fe3+ (, n=24, p<0.01), and dissolved methane (, n=15, p=0.02, Fig. 4a). None of these variables differed between sampling months, suggesting that these correlations reflect differences across sampling sites impacting carbon cycling and redox biogeochemistry. In addition to the trend of older deep porewater DOC with increasing dissolved methane concentrations, deep porewater DOC Δ14C values were positively correlated with δ13C of CH4 (ρ=0.45, n=16, p=0.08) and negatively correlated with the apparent fractionation factor (αc= [(δ13CO2 + 1000) (δ13CH4 + 1000)]) for methanogenesis (, n=15, p=0.01, Fig. 4b). Methane δ13C values can indicate the dominant pathway for methanogenesis (more depleted values associated with greater hydrogenotrophic methanogenesis and less depleted values associated with greater acetoclastic methanogenesis) but are also affected by other processes including diffusion and methanotrophy (Chanton, 2005). Thus, αc values, which represent the difference in stable C isotopic values of both DIC and CH4, are used to detect shifts in methanogenic pathways, with higher αc values indicative of hydrogenotrophic and lower αc values typical of acetoclastic methanogenesis (Whiticar et al., 1986; Wilson et al., 2016; Hines et al., 2008). In addition, αc values increased with depth (p<0.01) and from July to September in deep porewaters only (p<0.01). The correlation between αc values and DOC 14C values is likely not a causal relationship but rather points to a tendency towards increased hydrogenotrophic relative to acetoclastic methanogenesis in deeper porewaters later in the summer when DOC:DON ratios were also higher. Indeed, Throckmorton et al. (2015b) found that acetoclastic methanogenesis was the dominant pathway for methane production at all 17 of our sampling locations in July but that by September four drainages had become predominantly hydrogenotrophic (sites 3, 9, 10, and 15). Vaughn et al. (2016) measured CO2 and CH4 fluxes at sites across a permafrost degradation gradient within some of our study drainages and found that more degraded sites had lower net CH4 emissions, higher CH4 oxidation, and methanogenesis dominated by the hydrogenotrophic pathway (Vaughn et al., 2016).
3.2 Spatial patterns of DOC age and chemistry
Correlation analyses also suggested that DOC characteristics differed with sampling location features. DOC concentration decreased with increasing drainage size (, p<0.01, Fig. 5a), which ranged from 0.8 to 171 ha. Stepwise linear regression indicated that including drainage size improved model fit compared to depth and month alone. DOC 14C decreased with increasing drainage size (, p<0.01). DOC 14C was also correlated to water flow (stagnant or gentle flow observed upon sampling, ρ=0.28, p=0.01). Drainage size and water flow (stagnant or gentle flow) were correlated to one another (, p<0.01), such that wetland drainages with more stagnant waters tended to be smaller than channel drainages with gentle lateral flow, so stepwise linear regression was used to identify that the best model for predicting DOC 14C values included sampling month, depth, and drainage size. Controlling for month and depth, DOC 14C values decreased with increasing drainage size (p=0.01, Fig. 5b), likely reflecting greater production of relatively young DOC within the smaller drainages because these streams are not as deeply incised as the larger basins and thus tap into a younger permafrost carbon reservoir. Others have reported that small headwater streams and catchments had higher DOC 14C values (Neff et al., 2006), higher SUVA254 (Coch et al., 2019) and larger fractions of biodegradable DOC (Vonk et al., 2015a) than large rivers, while decreasing DOC concentration with increasing size of thaw lakes has been observed in areas of Western Siberia undergoing permafrost thaw (Shirokova et al., 2013).
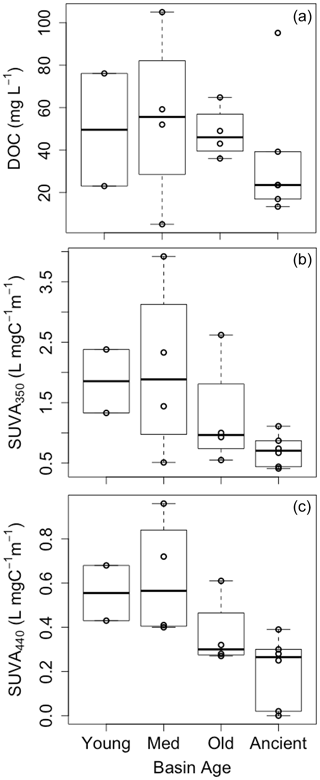
Figure 6DOC concentration (a), SUVA350 (b), and SUVA440 (c) in deep porewaters versus DTLB age (young: <50 years; medium: 50–300 years; old: 300–2000 years; and ancient 2000–5500 years).
In the DTLBs, depth to the thaw table in young (<50 years) and medium-aged (50–300 years) DTLBs was shallower by 10 cm on average than in old (300–2000 years) and ancient (2000–5500 years) DTLBs (p<0.01). Others have reported an increase in the organic layer thickness with increasing DTLB age (Bockheim et al., 2004; Hinkel et al., 2003), although no difference was reported for active layer thickness with basin age (Bockheim and Hinkel, 2005). In deep porewaters, DOC concentrations decreased with increasing basin age (p=0.03, Fig. 6a) controlling for depth and month. Also in deep porewaters, correlation analyses indicated that SUVA350 (, n=16, p=0.03, Fig. 6b) and SUVA440 (, n=16, p<0.01, Fig. 6c) decreased with increasing basin age, suggesting a decrease in the relative amount of lignin phenols and high molecular-weight compounds associated with fresh vegetation-derived organic matter as DTLBs age. Our interpretation is limited, as we only sampled from eight DTLBs, only one of which was younger than 50 years. However, our observed shifts in DOC chemistry are consistent with patterns of ecological succession and landform development for DTLBs. In our study region, young and medium-aged basins (<300 years) contain relatively productive plant communities dominated by grass and sedge species, while overall productivity declines coincide with ponding and polygonal ground development in old and ancient basins (Hinkel et al., 2003). Such declines in vegetation productivity should result in decreased carbon input to soils and in decreases in the indictors for vegetation-derived, unprocessed organic matter, including the SUVA values observed in this study. In fact, the degree of soil organic matter decomposition increased with increasing DTLB age based on decreasing fiber content (Bockheim et al., 2004; Hinkel et al., 2003) and extractable organic matter (Bockheim et al., 2004). We suggest that our observed trends reflect a decline in readily decomposable carbon (e.g., vegetation-derived, unprocessed organic matter) and an increase in the degree of organic matter decomposition as DTLBs age.
By studying 17 drainages in and around the Barrow Experimental Observatory, we found that DOC age and concentration in surface, shallow porewater, and deep porewaters increased with depth and as the thaw table deepened from July to September. We observed small shifts in DOC chemistry over the summer, including an increase in DOC:DON ratios and α440, the absorption coefficient that correlates with high molecular-weight organic matter. Indicators of DOC chemistry reflected a DOC source consistent with unprocessed organic matter throughout the summer: high in aromaticity, lignin phenols, and high molecular-weight compounds. Smaller wetland drainages released younger DOC that was higher in aromatics relative to larger drainage channels, while younger DTLBs released younger DOC that was higher in lignin phenols and high molecular-weight compounds than older DTLBs. Across our study area, we found that old and young DOC have similar chemical indicators of lability, suggesting that the production of old and labile DOC may continue with increased permafrost thaw as the active layer deepens and thaw seasons lengthen in the northern Arctic.
New data associated with this paper have been added to Throckmorton et al. (2015a). All data are available at https://doi.org/10.5440/1221564.
The supplement related to this article is available online at: https://doi.org/10.5194/bg-19-1211-2022-supplement.
KJM, HMT, JMH, BDN, and CJW designed the research. KJM, TPG, and CJW secured funding. HMT, JMH, BDN, and CJW performed fieldwork. KJM, ALH, and HMT performed laboratory analyses. KJM performed data analysis. All authors contributed to interpretation. KJM wrote the paper with input from all authors. KJM, MNR, and HMT interpreted data and made figures.
The contact author has declared that neither they nor their co-authors have any competing interests.
Publisher’s note: Copernicus Publications remains neutral with regard to jurisdictional claims in published maps and institutional affiliations.
This work was funded by the Office of Biological and Environmental Research in the U.S. Department of Energy Office of Science through the Next-Generation Ecosystem Experiments (NGEE Arctic) project and Award SCW1447 to LLNL. This work was performed under the auspices of the U.S. Department of Energy by Lawrence Livermore National Laboratory under contract DE-AC52-07NA27344 (LLNL-JRNL-808899) and by Los Alamos National Laboratory under contract DE-AC52-06NA25396. The publisher, by accepting this work for publication, acknowledges that the United States Government retains a nonexclusive, paid-up, irrevocable, worldwide license to publish or reproduce this work or allow others to do so for the United States Government purposes.
This research has been supported by the Office of Biological and Environmental Research (grant no. SCW1447 and the NGEE Arctic project).
This paper was edited by Steven Bouillon and reviewed by two anonymous referees.
Amon, R. M. W. and Meon, B.: The biogeochemistry of dissolved organic matter and nutrients in two large Arctic estuaries and potential implications for our understanding of the Arctic Ocean system, Mar. Chem., 92, 311–330, https://doi.org/10.1016/j.marchem.2004.06.034, 2004.
Amon, R. M. W., Rinehart, A. J., Duan, S., Louchouarn, P., Prokushkin, A., Guggenberger, G., Bauch, D., Stedmon, C., Raymond, P. A., Holmes, R. M., McClelland, J. W., Peterson, B. J., Walker, S. A., and Zhulidov, A. V.: Dissolved organic matter sources in large Arctic rivers, Geochim. Cosmochim. Ac., 94, 217–237, https://doi.org/10.1016/j.gca.2012.07.015, 2012.
Aurela, M., Laurila, T., and Tuovinen, J.-P.: Annual CO2 balance of a subarctic fen in northern Europe: Importance of the wintertime efflux, J. Geophys. Res., 107, 4607, https://doi.org/10.1029/2002jd002055, 2002.
Balcarczyk, K. L., Jones, J. B., Jaffé, R., and Maie, N.: Stream dissolved organic matter bioavailability and composition in watersheds underlain with discontinuous permafrost, Biogeochemistry, 94, 255–270, https://doi.org/10.1007/s10533-009-9324-x, 2009.
Benner, R., Benitez-Nelson, B., Kaiser, K., and Amon, R. M. W.: Export of young terrigenous dissolved organic carbon from rivers to the Arctic Ocean, Geophys. Res. Lett., 31, L05305, https://doi.org/10.1029/2003gl019251, 2004.
Billett, M. F., Palmer, S. M., Hope, D., Deacon, C., Storeton-West, R., Hargreaves, K. J., Flechard, C., and Fowler, D.: Linking land-atmosphere-stream carbon fluxes in a lowland peatland system, Global Biogeochem. Cy., 18, GB1024, https://doi.org/10.1029/2003gb002058, 2004.
Bockheim, J. G. and Hinkel, K. M.: Characteristics and Significance of the Transition Zone in Drained Thaw-Lake Basins of the Arctic Coastal Plain, Alaska, Arctic, 58, 406–417, 2005.
Bockheim, J. G., Hinkel, K. M., Eisner, W. R., and Dai, X. Y.: Carbon Pools and Accumulation Rates in an Age-Series of Soils in Drained Thaw-Lake Basins, Arctic Alaska, Soil Sci. Soc. Am. J., 68, 697–704, https://doi.org/10.2136/sssaj2004.6970, 2004.
Chanton, J. P.: The effect of gas transport on the isotope signature of methane in wetlands, Org. Geochem., 36, 753–768, https://doi.org/10.1016/j.orggeochem.2004.10.007, 2005.
Chanton, J. P., Glaser, P. H., Chasar, L. S., Burdige, D. J., Hines, M. E., Siegel, D. I., Tremblay, L. B., and Cooper, W. T.: Radiocarbon evidence for the importance of surface vegetation on fermentation and methanogenesis in contrasting types of boreal peatlands, Global Biogeochem. Cy., 22, GB4022, https://doi.org/10.1029/2008gb003274, 2008.
Charman, D., Aravena, R., Bryant, C. L., and Harkness, D. D.: Carbon isotopes in peat, DOC, CO2, and CH4 in a Holocene peatland on Dartmoor, southwest England, Geology, 27, 539–542, 1999.
Christensen, T. R., Johansson, T., Olsrud, M., Ström, L., Lindroth, A., Mastepanov, M., Malmer, N., Friborg, T., Crill, P., and Callaghan, T. V.: A catchment-scale carbon and greenhouse gas budget of a subarctic landscape, Philos. T. R. Soc. A, 365, 1643–1656, https://doi.org/10.1098/rsta.2007.2035, 2007.
Cleveland, C. C. and Liptzin, D.: stoichiometry in soil: is there a “Redfield ratio” for the microbial biomass?, Biogeochemistry, 85, 235–252, https://doi.org/10.1007/s10533-007-9132-0, 2007.
Coch, C., Juhls, B., Lamoureux, S. F., Lafrenière, M. J., Fritz, M., Heim, B., and Lantuit, H.: Comparisons of dissolved organic matter and its optical characteristics in small low and high Arctic catchments, Biogeosciences, 16, 4535–4553, https://doi.org/10.5194/bg-16-4535-2019, 2019.
Cole, J. J., Prairie, Y. T., Caraco, N. F., McDowwell, W. H., Tranvik, L. J., Striegl, R. G., Duarte, C. M., Kortelainen, P., Downing, J. A., Middleburg, J. J., and Melack, J.: Plumbing the global carbon cycle: integrating inland waters into the terrestrial carbon budget, Ecosystems, 10, 171–184, https://doi.org/10.1007/s10021-006-9013-8, 2007.
Corbett, J. E., Tfaily, M., Burdige, D., Cooper, W., Glaser, P., and Chanton, J.: Partitioning pathways of CO2 production in peatlands with stable carbon isotopes, Biogeochemistry, 114, 327–340, https://doi.org/10.1007/s10533-012-9813-1, 2013.
Dean, J. F., van der Velde, Y., Garnett, M. H., Dinsmore, K. J., Baxter, R., Lessels, J. S., Smith, P., Street, L. E., Subke, J. A., Tetzlaff, D., Washbourne, I., Wookey, P. A., and Billett, M. F.: Abundant pre-industrial carbon detected in Canadian Arctic headwaters: implications for the permafrost carbon feedback, Environ. Res. Lett., 13, 034024, https://doi.org/10.1088/1748-9326/aaa1fe, 2018.
Dean, J. F., Meisel, O. H., Martyn Rosco, M., Marchesini, L. B., Garnett, M. H., Lenderink, H., van Logtestijn, R., Borges, A. V., Bouillon, S., Lambert, T., Röckmann, T., Maximov, T., Petrov, R., Karsanaev, S., Aerts, R., van Huissteden, J., Vonk, J. E., and Dolman, A. J.: East Siberian Arctic inland waters emit mostly contemporary carbon, Nat. Commun., 11, 1627, https://doi.org/10.1038/s41467-020-15511-6, 2020.
De Rosario-Martinez, H.: phia: Post-Hoc Interaction Analysis, CRAN repository [code], https://cran.r-project.org/web/packages/phia/index.html (last access: 26 April2 2019), 2015.
Drake, T. W., Wickland, K. P., Spencer, R. G., McKnight, D. M., and Striegl, R. G.: Ancient low-molecular-weight organic acids in permafrost fuel rapid carbon dioxide production upon thaw, P. Natl. Acad. Sci. USA, 112, 13946–13951, https://doi.org/10.1073/pnas.1511705112, 2015.
Drake, T. W., Raymond, P. A., and Spencer, R. G. M.: Terrestrial carbon inputs to inland waters: A current synthesis of estimates and uncertainty, Limnol. Oceanogr. Lett., 3, 132–142, https://doi.org/10.1002/lol2.10055, 2018a.
Drake, T. W., Guillemette, F., Hemingway, J. D., Chanton, J. P., Podgorski, D. C., Zimov, N. S., and Spencer, R. G. M.: The Ephemeral Signature of Permafrost Carbon in an Arctic Fluvial Network, J. Geophys. Res.-Biogeo., 123, 1475–1485, https://doi.org/10.1029/2017jg004311, 2018b.
Estop-Aragonés, C., Olefeldt, D., Abbott, B. W., Chanton, J. P., Czimczik, C. I., Dean, J. F., Egan, J. E., Gandois, L., Garnett, M. H., Hartley, I. P., Hoyt, A., Lupascu, M., Natali, S. M., O'Donnell, J. A., Raymond, P. A., Tanentzap, A. J., Tank, S. E., Schuur, E. A. G., Turetsky, M., and Anthony, K. W.: Assessing the Potential for Mobilization of Old Soil Carbon After Permafrost Thaw: A Synthesis of 14C Measurements From the Northern Permafrost Region, Global Biogeochem. Cy., 34, e2020GB006672, https://doi.org/10.1029/2020GB006672, 2020.
Fan, Z., Neff, J. C., and Wickland, K. P.: Modeling the production, decomposition, and tranport of dissolved organic carbon in boreal soils, Soil Sci., 175, 223–232, 2010.
Finlay, J., Neff, J., Zimov, S., Davydova, A., and Davydov, S.: Snowmelt dominance of dissolved organic carbon in high-latitude watersheds: Implications for characterization and flux of river DOC, Geophys. Res. Lett., 33, L10401, https://doi.org/10.1029/2006gl025754, 2006.
Freeman, C., Evans, C. D., Monteith, D. T., Reynolds, B., and Fenner, N.: Export of organic carbon from peat soils, Nature, 412, 785–785, https://doi.org/10.1038/35090628, 2001.
Frey, K. E.: Amplified carbon release from vast West Siberian peatlands by 2100, Geophys. Res. Lett., 32, L09401, https://doi.org/10.1029/2004gl022025, 2005.
Frey, K. E. and McClelland, J. W.: Impacts of permafrost degradation on arctic river biogeochemistry, Hydrol, Process., 23, 169–182, https://doi.org/10.1002/hyp.7196, 2009.
Gao, L., Zhou, Z., Reyes, A. V., and Guo, L.: Yields and Characterization of Dissolved Organic Matter From Different Aged Soils in Northern Alaska, J. Geophys. Res.-Biogeo., 123, 2035–2052, https://doi.org/10.1029/2018jg004408, 2018.
Guo, L. and Macdonald, R. W.: Source and transport of terrigenous organic matter in the upper Yukon River: Evidence from isotope (δ13C, Δ14C, and δ15N) composition of dissolved, colloidal, and particulate phases, Global Biogeochem. Cy., 20, GB2011, https://doi.org/10.1029/2005gb002593, 2006.
Guo, L., Ping, C.-L., and Macdonald, R. W.: Mobilization pathways of organic carbon from permafrost to arctic rivers in a changing climate, Geophys. Res. Lett., 34, L13603, https://doi.org/10.1029/2007gl030689, 2007.
Harrell Jr, F. E.: Hmisc: Harrell Miscellaneous, CRAN repository [code], https://cran.r-project.org/web/packages/Hmisc/, last access: 8 November 2019.
Hayes, D. J., Kicklighter, D. W., McGuire, A. D., Chen, M., Zhuang, Q., Yuan, F., Melillo, J. M., and Wullschleger, S. D.: The impacts of recent permafrost thaw on land–atmosphere greenhouse gas exchange, Environ. Res. Lett., 9, 045005, https://doi.org/10.1088/1748-9326/9/4/045005, 2014.
Hicks Pries, C. E., Schuur, E. A. G., and Crummer, K. G.: Thawing permafrost increases old soil and autotrophic respiration in tundra: Partitioning ecosystem respiration using δ13C and Δ14C, Glob. Change Biol., 19, 649–661, https://doi.org/10.1111/gcb.12058, 2013.
Hines, M. E., Duddleston, K. N., Rooney-Varga, J. N., Fields, D., and Chanton, J. P.: Uncoupling of acetate degradation from methane formation in Alaskan wetlands: Connections to vegetation distribution, Global Biogeochem. Cy., 22, GB2017, https://doi.org/10.1029/2006gb002903, 2008.
Hinkel, K. M., Eisner, W. R., Bockheim, J. G., Nelson, F. E., Peterson, K. M., and Dai, X.: Spatial Extent, Age, and Carbon Stocks in Drained Thaw Lake Basins on the Barrow Peninsula, Alaska, Arct. Antarct. Alp. Res., 35, 291–300, https://doi.org/10.1657/1523-0430(2003)035[0291:Seaacs]2.0.Co;2, 2003.
Holmes, R. M., McClelland, J. W., Raymond, P. A., Frazer, B. B., Peterson, B. J., and Stieglitz, M.: Lability of DOC transported by Alaskan rivers to the Arctic Ocean, Geophys. Res. Lett., 35, L03402, https://doi.org/10.1029/2007gl032837, 2008.
Holmes, R. M., McClelland, J. W., Peterson, B. J., Tank, S. E., Bulygina, E., Eglinton, T. I., Gordeev, V. V., Gurtovaya, T. Y., Raymond, P. A., Repeta, D. J., Staples, R., Striegl, R. G., Zhulidov, A. V., and Zimov, S. A.: Seasonal and Annual Fluxes of Nutrients and Organic Matter from Large Rivers to the Arctic Ocean and Surrounding Seas, Estuar. Coast., 35, 369–382, https://doi.org/10.1007/s12237-011-9386-6, 2011.
Hugelius, G., Strauss, J., Zubrzycki, S., Harden, J. W., Schuur, E. A. G., Ping, C.-L., Schirrmeister, L., Grosse, G., Michaelson, G. J., Koven, C. D., O'Donnell, J. A., Elberling, B., Mishra, U., Camill, P., Yu, Z., Palmtag, J., and Kuhry, P.: Estimated stocks of circumpolar permafrost carbon with quantified uncertainty ranges and identified data gaps, Biogeosciences, 11, 6573–6593, https://doi.org/10.5194/bg-11-6573-2014, 2014.
Johnston, S. E., Carey, J. C., Kellerman, A., Podgorski, D. C., Gewirtzman, J., and Spencer, R. G. M.: Controls on Riverine Dissolved Organic Matter Composition Across an Arctic-Boreal Latitudinal Gradient, J. Geophys. Res.-Biogeo., 126, e2020JG005988, https://doi.org/10.1029/2020JG005988, 2021.
Kawahigashi, M., Kaiser, K., Kalbitz, K., Rodionov, A., and Guggenberger, G.: Dissolved organic matter in small streams along a gradient from discontinuous to continuous permafrost, Glob. Change Biol., 10, 1576–1586, https://doi.org/10.1111/j.1365-2486.2004.00827.x, 2004.
Kellerman, A. M., Arellano, A., Podgorski, D. C., Martin, E. E., Martin, J. B., Deuerling, K. M., Bianchi, T. S., and Spencer, R. G. M.: Fundamental drivers of dissolved organic matter composition across an Arctic effective precipitation gradient, Limnol. Oceanogr., 65, 1217–1234, https://doi.org/10.1002/lno.11385, 2019.
Kling, G. W., Kipphut, G. W., and Miller., M. C.: Arctic Lakes and streams as gas conduits to the atmosphere: implications for tundra carbon budgets, Science, 251, 298–301, 1991.
Koven, C. D., Ringeval, B., Friedlingstein, P., Ciais, P., Cadule, P., Khvorostyanov, D., Krinner, G., and Tarnocai, C.: Permafrost carbon-climate feedbacks accelerate global warming, P. Natl. Acad. Sci. USA, 108, 14769–14774, https://doi.org/10.1073/pnas.1103910108, 2011.
Lapierre, J.-F., Guillemette, F., Berggren, M., and del Giorgio, P. A.: Increases in terrestrially derived carbon stimulate organic carbon processing and CO2 emissions in boreal aquatic ecosystems, Nat. Commun., 4, 2972, https://doi.org/10.1038/ncomms3972, 2013.
Lavallee, J. M., Soong, J. L., and Cotrufo, M. F.: Conceptualizing soil organic matter into particulate and mineral-associated forms to address global change in the 21st century, Glob. Change Biol., 26, 261–273, https://doi.org/10.1111/gcb.14859, 2020.
Liu, F., Kou, D., Abbott, B. W., Mao, C., Chen, Y., Chen, L., and Yang, Y.: Disentangling the Effects of Climate, Vegetation, Soil and Related Substrate Properties on the Biodegradability of Permafrost-Derived Dissolved Organic Carbon, J. Geophys. Res.-Biogeo., 124, 3377–3389, https://doi.org/10.1029/2018JG004944, 2019.
Mann, P. J., Davydova, A., Zimov, N., Spencer, R. G. M., Davydov, S., Bulygina, E., Zimov, S., and Holmes, R. M.: Controls on the composition and lability of dissolved organic matter in Siberia's Kolyma River basin, J. Geophys. Res.-Biogeo., 117, G01028, https://doi.org/10.1029/2011jg001798, 2012.
Mann, P. J., Eglinton, T. I., McIntyre, C. P., Zimov, N., Davydova, A., Vonk, J. E., Holmes, R. M., and Spencer, R. G.: Utilization of ancient permafrost carbon in headwaters of Arctic fluvial networks, Nat. Commun., 6, 7856, https://doi.org/10.1038/ncomms8856, 2015.
Mann, P. J., Spencer, R. G. M., Hernes, P. J., Six, J., Aiken, G. R., Tank, S. E., McClelland, J. W., Butler, K. D., Dyda, R. Y., and Holmes, R. M.: Pan-Arctic Trends in Terrestrial Dissolved Organic Matter from Optical Measurements, Front. Earth Sci., 4, 25, https://doi.org/10.3389/feart.2016.00025, 2016.
McClelland, J. W., Holmes, R. M., Peterson, B. J., Raymond, P. A., Striegl, R. G., Zhulidov, A. V., Zimov, S. A., Zimov, N., Tank, S. E., Spencer, R. G. M., Staples, R., Gurtovaya, T. Y., and Griffin, C. G.: Particulate organic carbon and nitrogen export from major Arctic rivers, Global Biogeochem. Cy., 30, 629–643, https://doi.org/10.1002/2015gb005351, 2016.
McGuire, A. D., Anderson, L. G., Christensen, T. R., Dallimore, S., Guo, L., Hayes, D. J., Heimann, M., Lorenson, T. D., Macdonald, R. W., and Roulet, N.: Sensitivity of the carbon cycle in the Arctic to climate change, Ecol. Monogr., 79, 523–555, https://doi.org/10.1890/08-2025.1, 2009.
Molot, L. A. and Dillon, P. J.: Photolytic regulation of dissolved organic carbon in northern lakes, Global Biogeochem. Cy., 11, 357–365, https://doi.org/10.1029/97gb01198, 1997.
Moore, T. R., Trofymow, J. A., Prescott, C. E., Titus, B. D., and Group, C. W.: Nature and nurture in the dynamics of C, N and P during litter decomposition in Canadian forests, Plant Soil, 339, 163–175, https://doi.org/10.1007/s11104-010-0563-3, 2011.
Neff, J. C. and Hooper, D. U.: Vegetation and climate controls on potential CO2, DOC and DON production in northern latitude soils, Global Change Biol., 8, 872–884, 2002.
Neff, J. C., Finlay, J. C., Zimov, S. A., Davydov, S. P., Carrasco, J. J., Schuur, E. A. G., and Davydova, A. I.: Seasonal changes in the age and structure of dissolved organic carbon in Siberian rivers and streams, Geophys. Res. Lett., 33, L23401, https://doi.org/10.1029/2006gl028222, 2006.
Newman, B. D., Throckmorton, H. M., Graham, D. E., Gu, B., Hubbard, S. S., Liang, L., Wu, Y., Heikoop, J. M., Herndon, E. M., Phelps, T. J., Wilson, C. J., and Wullschleger, S. D.: Microtopographic and depth controls on active layer chemistry in Arctic polygonal ground, Geophys. Res. Lett., 42, 1808–1817, https://doi.org/10.1002/2014gl062804, 2015.
O'Donnell, J. A., Aiken, G. R., Walvoord, M. A., and Butler, K. D.: Dissolved organic matter composition of winter flow in the Yukon River basin: Implications of permafrost thaw and increased groundwater discharge, Global Biogeochem. Cy., 26, GB0E06, https://doi.org/10.1029/2012gb004341, 2012.
Olefeldt, D. and Roulet, N. T.: Effects of permafrost and hydrology on the composition and transport of dissolved organic carbon in a subarctic peatland complex, J. Geophys. Res.-Biogeo., 117, G01005, https://doi.org/10.1029/2011jg001819, 2012.
Pastor, J., Solin, J., Bridgham, S. D., Updegraff, K., Harth, C., Weishampel, P., and Dewey, B.: Global warming and the export of dissolved organic carbon from boreal peatlands, Oikos, 100, 380–386, 10.1034/j.1600-0706.2003.11774.x, 2003.
Pinheiro, J., Bates, D., DebRoy, S., Sarkar, D., and R Core Team: nlme: Linear and Nonlinear Mixed Effects Models, CRAN repository [code], https://cran.r-project.org/web/packages/nlme/index.html, last access: 5 July 2019.
Pokrovsky, O. S., Shirokova, L. S., Kirpotin, S. N., Audry, S., Viers, J., and Dupré, B.: Effect of permafrost thawing on organic carbon and trace element colloidal speciation in the thermokarst lakes of western Siberia, Biogeosciences, 8, 565–583, https://doi.org/10.5194/bg-8-565-2011, 2011.
Prokushkin, A. S., Pokrovsky, O. S., Shirokova, L. S., Korets, M. A., Viers, J., Prokushkin, S. G., Amon, R. M. W., Guggenberger, G., and McDowell, W. H.: Sources and the flux pattern of dissolved carbon in rivers of the Yenisey basin draining the Central Siberian Plateau, Environ. Res. Lett., 6, 045212, https://doi.org/10.1088/1748-9326/6/4/045212, 2011.
Raymond, P. A., Saiers, J. E., and Sobczak, W. V.: Hydrological and biogeochemical controls on watershed dissolved organic matter transport: pulse-shunt concept, Ecology, 97, 5–16, https://doi.org/10.1890/14-1684.1, 2016.
Raymond, P. A., McClelland, J. W., Holmes, R. M., Zhulidov, A. V., Mull, K., Peterson, B. J., Striegl, R. G., Aiken, G. R., and Gurtovaya, T. Y.: Flux and age of dissolved organic carbon exported to the Arctic Ocean: A carbon isotopic study of the five largest arctic rivers, Global Biogeochem. Cy., 21, GB4011, https://doi.org/10.1029/2007gb002934, 2007.
R Core Team: R: A language and environment for statistical computing., R Foundation for Statistical Computing [code], https://www.R-project.org, last access: 5 November 2019.
Roulet, N. T., Lafleur, P. M., Richard, P. J. H., Moore, T. R., Humphreys, E. R., and Bubier, J.: Contemporary carbon balance and late Holocene carbon accumulation in a northern peatland, Glob. Change Biol., 13, 397–411, https://doi.org/10.1111/j.1365-2486.2006.01292.x, 2007.
Schadel, C., Bader, M. K. F., Schuur, E. A. G., Biasi, C., Bracho, R., Capek, P., De Baets, S., Diakova, K., Ernakovich, J., Estop-Aragones, C., Graham, D. E., Hartley, I. P., Iversen, C. M., Kane, E. S., Knoblauch, C., Lupascu, M., Martikainen, P. J., Natali, S. M., Norby, R. J., O'Donnell, J. A., Chowdhury, T. R., Santruckova, H., Shaver, G., Sloan, V. L., Treat, C. C., Turetsky, M. R., Waldrop, M. P., and Wickland, K. P.: Potential carbon emissions dominated by carbon dioxide from thawed permafrost soils, Nat. Clim. Change, 6, 950–953, https://doi.org/10.1038/Nclimate3054, 2016.
Schaefer, K., Lantuit, H., Romanovsky, V. E., Schuur, E. A. G., and Witt, R.: The impact of the permafrost carbon feedback on global climate, Environ. Res. Lett., 9, 085003, https://doi.org/10.1088/1748-9326/9/8/085003, 2014.
Schuur, E. A. G., Bockheim, J., Canadell, J. G., Euskirchen, E., Field, C. B., Goryachkin, S. V., Hagemann, S., Kuhry, P., Lafleur, P. M., Lee, H., Mazhitova, G., Nelson, F. E., Rinke, A., Romanovsky, V. E., Shiklomanov, N., Tarnocai, C., Venevsky, S., Vogel, J. G., and Zimov, S. A.: Vulnerability of permafrost carbon to climate change: Implications for the global carbon cycle, Bioscience, 58, 701–714, https://doi.org/10.1641/B580807, 2008.
Schuur, E. A. G., Abbott, B. W., Bowden, W. B., Brovkin, V., Camill, P., Canadell, J. G., Chanton, J. P., Chapin III, F. S., Christensen, T. R., Ciais, P., Crosby, B. T., Czimczik, C. I., Grosse, G., Harden, J., Hayes, D. J., Hugelius, G., Jastrow, J. D., Jones, J. B., Kleinen, T., Koven, C. D., Krinner, G., Kuhry, P., Lawrence, D. M., McGuire, A. D., Natali, S. M., O'Donnell, J. A., Ping, C. L., Riley, W. J., Rinke, A., Romanovsky, V. E., Sannel, A. B. K., Schädel, C., Schaefer, K., Sky, J., Subin, Z. M., Tarnocai, C., Turetsky, M. R., Waldrop, M. P., Walter Anthony, K. M., Wickland, K. P., Wilson, C. J., and Zimov, S. A.: Expert assessment of vulnerability of permafrost carbon to climate change, Climatic Change, 119, 359–374, https://doi.org/10.1007/s10584-013-0730-7, 2013.
Schuur, E. A. G., McGuire, A. D., Schadel, C., Grosse, G., Harden, J. W., Hayes, D. J., Hugelius, G., Koven, C. D., Kuhry, P., Lawrence, D. M., Natali, S. M., Olefeldt, D., Romanovsky, V. E., Schaefer, K., Turetsky, M. R., Treat, C. C., and Vonk, J. E.: Climate change and the permafrost carbon feedback, Nature, 520, 171–179, https://doi.org/10.1038/nature14338, 2015.
Shirokova, L. S., Pokrovsky, O. S., Kirpotin, S. N., Desmukh, C., Pokrovsky, B. G., Audry, S., and Viers, J.: Biogeochemistry of organic carbon, CO2, CH4, and trace elements in thermokarst water bodies in discontinuous permafrost zones of Western Siberia, Biogeochemistry, 113, 573–593, https://doi.org/10.1007/s10533-012-9790-4, 2013.
Shogren, A. J., Zarnetske, J. P., Abbott, B. W., Iannucci, F., Frei, R. J., Griffin, N. A., and Bowden, W. B.: Revealing biogeochemical signatures of Arctic landscapes with river chemistry, Scientific Reports, 9, 12894, https://doi.org/10.1038/s41598-019-49296-6, 2019.
Spencer, R. G. M., Aiken, G. R., Wickland, K. P., Striegl, R. G., and Hernes, P. J.: Seasonal and spatial variability in dissolved organic matter quantity and composition from the Yukon River basin, Alaska, Global Biogeochem. Cy., 22, GB4002, https://doi.org/10.1029/2008gb003231, 2008.
Spencer, R. G. M., Butler, K. D., and Aiken, G. R.: Dissolved organic carbon and chromophoric dissolved organic matter properties of rivers in the USA, J. Geophys. Res.-Biogeo., 117, G03001, https://doi.org/10.1029/2011JG001928, 2012.
Striegl, R. G., Dornblaser, M. M., Aiken, G. R., Wickland, K. P., and Raymond, P. A.: Carbon export and cycling by the Yukon, Tanana, and Porcupine rivers, Alaska, 2001–2005, Water Resour. Res., 43, W02411, https://doi.org/10.1029/2006wr005201, 2007.
Stuiver, M. and Polach, H. A.: Reporting of C-14 data, Radiocarbon, 19, 355–363, 1977.
Tanentzap, A. J., Burd, K., Kuhn, M., Estop-Aragonés, C., Tank, S. E., and Olefeldt, D.: Aged soils contribute little to contemporary carbon cycling downstream of thawing permafrost peatlands, Glob. Change Biol., 27, 5368–5382, https://doi.org/10.1111/gcb.15756, 2021.
Tank, S. E., Fellman, J. B., Hood, E., and Kritzberg, E. S.: Beyond respiration: Controls on lateral carbon fluxes across the terrestrial-aquatic interface, Limnol. Oceanogr. Lett., 3, 76–88, https://doi.org/10.1002/lol2.10065, 2018.
Textor, S. R., Wickland, K. P., Podgorski, D. C., Johnston, S. E., and Spencer, R. G. M.: Dissolved Organic Carbon Turnover in Permafrost-Influenced Watersheds of Interior Alaska: Molecular Insights and the Priming Effect, Front. Earth Sci., 7, 275, https://doi.org/10.3389/feart.2019.00275, 2019.
Throckmorton, H. M., Heikoop, J. M., McFarlane, K., Newman, B. D., and Wilson, C. J.: Inorganic Carbon Isotopes and Chemical Characterization of Watershed Drainages, Barrow, Alaska, 2013, U.S. Department of Energy, Oak Ridge national Laboratory [data set], https://doi.org/10.5440/1221564, 2015a.
Throckmorton, H. M., Heikoop, J. M., Newman, B. D., Altmann, G. L., Conrad, M. S., Muss, J. D., Perkins, G. B., Smith, L. J., Torn, M. S., Wullschleger, S. D., and Wilson, C. J.: Pathways and transformations of dissolved methane and dissolved inorganic carbon in Arctic tundra watersheds: Evidence from analysis of stable isotopes, Global Biogeochem. Cy., 29, 1893–1910, https://doi.org/10.1002/2014gb005044, 2015b.
Townsend-Small, A., McClelland, J. W., Max Holmes, R., and Peterson, B. J.: Seasonal and hydrologic drivers of dissolved organic matter and nutrients in the upper Kuparuk River, Alaskan Arctic, Biogeochemistry, 103, 109–124, https://doi.org/10.1007/s10533-010-9451-4, 2011.
Vaughn, L. J. S. and Torn, M. S.: Radiocarbon measurements of ecosystem respiration and soil pore-space CO2 in Utqiaġvik (Barrow), Alaska, Earth Syst. Sci. Data, 10, 1943–1957, https://doi.org/10.5194/essd-10-1943-2018, 2018.
Vaughn, L. J. S., Conrad, M. E., Bill, M., and Torn, M. S.: Isotopic insights into methane production, oxidation, and emissions in Arctic polygon tundra, Glob. Change Biol., 22, 3487–3502, https://doi.org/10.1111/gcb.13281, 2016.
Venables, W. N. and Ripley, B. D.: Modern Applied Statistics with S, Fourth, Springer, New York, ISBN 0-387-95457-0, 2002.
Vogel, J. S., Southon, J. R., Nelson, D. E., and Brown, T. A.: Performance of catalytically condensed carbon for use in accelerator mass-spectrometry, Nucl. Instrum. Meth. B, 5, 289–293, https://doi.org/10.1016/0168-583X(84)90529-9, 1984.
Vonk, J. E., Mann, P. J., Davydov, S., Davydova, A., Spencer, R. G. M., Schade, J., Sobczak, W. V., Zimov, N., Zimov, S., Bulygina, E., Eglinton, T. I., and Holmes, R. M.: High biolability of ancient permafrost carbon upon thaw, Geophys. Res. Lett., 40, 2689–2693, https://doi.org/10.1002/grl.50348, 2013.
Vonk, J. E., Tank, S. E., Mann, P. J., Spencer, R. G. M., Treat, C. C., Striegl, R. G., Abbott, B. W., and Wickland, K. P.: Biodegradability of dissolved organic carbon in permafrost soils and aquatic systems: a meta-analysis, Biogeosciences, 12, 6915–6930, https://doi.org/10.5194/bg-12-6915-2015, 2015a.
Vonk, J. E., Tank, S. E., Bowden, W. B., Laurion, I., Vincent, W. F., Alekseychik, P., Amyot, M., Billet, M. F., Canário, J., Cory, R. M., Deshpande, B. N., Helbig, M., Jammet, M., Karlsson, J., Larouche, J., MacMillan, G., Rautio, M., Walter Anthony, K. M., and Wickland, K. P.: Reviews and syntheses: Effects of permafrost thaw on Arctic aquatic ecosystems, Biogeosciences, 12, 7129–7167, https://doi.org/10.5194/bg-12-7129-2015, 2015b.
Vonk, J. E., Tank, S. E., and Walvoord, M. A.: Integrating hydrology and biogeochemistry across frozen landscapes, Nat. Commun., 10, 5377, https://doi.org/10.1038/s41467-019-13361-5, 2019.
Weishaar, J. L., Aiken, G. R., Bergamaschi, B. A., Fram, M. S., Fujii, R., and Mopper, K.: Evaluation of Specific Ultraviolet Absorbance as an Indicator of the Chemical Composition and Reactivity of Dissolved Organic Carbon, Environ. Sci. Technol., 37, 4702–4708, https://doi.org/10.1021/es030360x, 2003.
Whiticar, M. J., Faber, E., and Schoell, M.: Biogenic methane formation in marine and freshwater environments: CO2 reduction vs. acetate fermentation – Isotope evidence, Geochim. Cosmochim. Ac., 50, 693–709, https://doi.org/10.1016/0016-7037(86)90346-7, 1986.
Whittinghill, K. A., Finlay, J. C., and Hobbie, S. E.: Bioavailability of dissolved organic carbon across a hillslope chronosequence in the Kuparuk River region, Alaska, Soil Biol. Biochem., 79, 25–33, https://doi.org/10.1016/j.soilbio.2014.08.020, 2014.
Wild, B., Schnecker, J., Alves, R. J. E., Barsukov, P., Bárta, J., Čapek, P., Gentsch, N., Gittel, A., Guggenberger, G., Lashchinskiy, N., Mikutta, R., Rusalimova, O., Šantrůčková, H., Shibistova, O., Urich, T., Watzka, M., Zrazhevskaya, G., and Richter, A.: Input of easily available organic C and N stimulates microbial decomposition of soil organic matter in arctic permafrost soil, Soil Biol. Biochem., 75, 143–151, https://doi.org/10.1016/j.soilbio.2014.04.014, 2014.
Wild, B., Andersson, A., Bröder, L., Vonk, J., Hugelius, G., McClelland, J. W., Song, W., Raymond, P. A., and Gustafsson, Ö.: Rivers across the Siberian Arctic unearth the patterns of carbon release from thawing permafrost, P. Natl. Acad. Sci. USA, 116, 10280, https://doi.org/10.1073/pnas.1811797116, 2019.
Wilson, R. M., Hopple, A. M., Tfaily, M. M., Sebestyen, S. D., Schadt, C. W., Pfeifer-Meister, L., Medvedeff, C., McFarlane, K. J., Kostka, J. E., Kolton, M., Kolka, R. K., Kluber, L. A., Keller, J. K., Guilderson, T. P., Griffiths, N. A., Chanton, J. P., Bridgham, S. D., and Hanson, P. J.: Stability of peatland carbon to rising temperatures, Nat. Commun., 7, 13723, https://doi.org/10.1038/ncomms13723, 2016.
Yacobi, Y., Alberts, J., Takacs, M., and McElvaine, M.: Absorption spectroscopy of colored dissolved organic carbon in Georgia (USA) rivers: the impact of molecular size distribution, J. Limnol., 62, 41–46, https://doi.org/10.4081/jlimnol.2003.41, 2003.