the Creative Commons Attribution 4.0 License.
the Creative Commons Attribution 4.0 License.
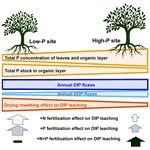
Leaching of inorganic and organic phosphorus and nitrogen in contrasting beech forest soils – seasonal patterns and effects of fertilization
Emmanuel Frossard
Klaus Kaiser
Frank Hagedorn
Leaching is one major pathway of phosphorus (P) and nitrogen (N) losses from forest ecosystems. Using a full factorial N×P fertilization and irrigation experiment, we investigated the leaching of dissolved organic and inorganic P (DOP and DIP) and N (DON and DIN) from organic layers (litter, horizons) and mineral A horizons at two European beech sites of contrasting P status. Leachates showed the highest DIP and DIN concentrations in summer and lowest in winter, while dissolved organic forms remained rather constant throughout seasons. During the dry and hot summer of 2018, DOC:DOP and DOC:DON ratios in leachates were particularly narrow, suggesting a release of microbial P due to cell lysis by drying and rewetting. This effect was stronger at the low-P site. The estimated annual mean fluxes from the horizons in the non-fertilized treatment were 60 and 30 for dissolved total P and 730 and 650 for dissolved total N at the high-P and the low-P site, respectively. Fluxes of P were highest in the organic layers and decreased towards the A horizon likely due to sorption by minerals. Fertilization effects were additive at the high-P but antagonistic at the low-P site: at the high-P site, fertilization with +N, +P, and +N+P increased total P fluxes from the horizon by +33 %, +51 %, and +75 %, while the respective increases were +198 %, +156 %, and +10 % at the low-P site. The positive N effect on DIP leaching possibly results from a removed N limitation of phosphatase activity at the low-P site. Fluxes of DOP remained unaffected by fertilization. Fluxes of DIN and DON from the horizons increased upon +N and +N+P but not upon +P fertilization. In conclusion, the estimated P fluxes from the A horizons were comparable in magnitude to reported atmospheric P inputs, suggesting that these systems do not deplete in P due to leaching. However, a particularly high sensitivity of DIP leaching to hotter and drier conditions suggests accelerated P losses under the expected more extreme future climate conditions. Increases in P leaching due to fertilization and drying–rewetting were higher in the low-P system, implying that the low-P system is more susceptible to environmental future changes.
- Article
(2021 KB) - Full-text XML
-
Supplement
(846 KB) - BibTeX
- EndNote
Leaching is one major pathway of phosphorus (P) and nitrogen (N) loss from forest ecosystems (e.g. Bol et al., 2016; Hedin et al., 1995). Leaching refers to water-flow-related transport of solutes within and from soil. Leaching losses are a result of the balance between mineralization, dissolution, and desorption processes on one side and biological uptake, as well as abiotic precipitation and sorption, on the other side. High water flow conditions may support leaching since transport becomes faster than the uptake of nutrients by roots and microorganisms and sorption (e.g. Backnäs et al., 2012; Barrow, 1983). Phosphorus and N are leached in dissolved inorganic and organic forms, or sorbed to colloids, with concentrations and fluxes varying strongly among soils and ecosystem types (Bol et al., 2016; Kaiser et al., 2003; Qualls and Haines, 1991a). Dissolved organic N was found to dominate the N losses from unpolluted ecosystems (Perakis and Hedin, 2001, 2002), while inorganic N dominates in leachates from most temperate forest ecosystems receiving high atmospheric N deposition (Hagedorn et al., 2001). Substantially less is known about P leaching (Bol et al., 2016; Hannapel et al., 1964a, b), but the importance of organic forms for leaching may be even greater for P than for N (Qualls, 2000; Qualls and Haines, 1991a). Inorganic P forms strongly bind to or become incorporated into secondary minerals (e.g. Walker and Syers, 1976) or can be taken up by roots and microorganisms. Thus, concentrations of inorganic P in soil solutions are usually low even in soils where high amounts of soil organic matter are mineralized (Bol et al., 2016; Kaiser et al., 2000; Qualls and Haines, 1991a). Some organic P compounds sorb less strongly on mineral surfaces than inorganic P (Anderson and Magdoff, 2005; Berg and Joern, 2006; Celi et al., 2003; Lilienfein et al., 2004), and it has been suggested that the most P-rich fractions of dissolved organic matter (DOM) are more mobile than the rest (Frossard et al., 1989; Kaiser et al., 2000, 2001; Qualls, 2000; Qualls and Haines, 1991a). Accordingly, much of the P in soil solution has been found in organic form (Hedin et al., 2003; Kaiser et al., 2000, 2003; Qualls, 2000; Qualls and Haines, 1991b), and it might lead to long-term P depletion of soils (Alvarez-Cobelas et al., 2009; Frossard et al., 1989; Hedin et al., 2003).
As outlined above, leaching is the net result of the interplay of biotic and abiotic processes: models of C–N–P cycles assume that microbial activity is driven by temperature, moisture, and organic matter bioavailability being key determinants for nutrient mineralization in soil (Colman and Schimel, 2013; Davies et al., 2016; Yu et al., 2020). Besides seasonal variation (Kaiser et al., 2003; Kalbitz et al., 2000; Michalzik et al., 2001), extreme changes in temperatures by freeze–thaw cycles or in the precipitation regime were found to increase nutrient release (e.g. Gao et al., 2020, 2021). Drying–rewetting effects can trigger release pulses of nutrients (Birch, 1958) that are then prone to leaching (Achat et al., 2012; Blackwell et al., 2010; Borken and Matzner, 2009; Brödlin et al., 2019b; Dinh et al., 2017, 2016). Climatic warming and the increasing frequency and severity of droughts influence soil organic matter (SOM) dynamics and the associated release and leaching of P and N (e.g. Gao et al., 2020).
In addition, the nutritional status of soils, defined by parent material, climatic conditions, and atmospheric input (Augusto et al., 2017), exerts strong influence on nutrient release (Mooshammer et al., 2014). The stoichiometry of SOM has been identified as a key parameter: while critical C-to-nutrient ratios – above which nutrients that are mineralized during decomposition become immobilized by soil microorganisms and below which they are released in excess of biological demand – are well established for N, they remain uncertain for P (e.g. Davies et al., 2016; Mooshammer et al., 2014). However, the concept of resource limitation has shifted from an earlier paradigm of single-resource limitation (e.g. van der Ploeg et al., 1999) towards co-limitation by multiple resources (e.g. Harpole et al., 2011). Synergistic interactions between N and P have frequently been observed in aquatic and terrestrial ecosystems (Elser et al., 2007) but have not – to our knowledge – been studied in field studies on nutrient leaching in temperate forest ecosystems. Rising atmospheric CO2, N, and P depositions may induce imbalances between C, N, and P, impacting the ratio of SOM and hence the cycling and leaching of P and N (Fröberg et al., 2013; Mortensen et al., 1998; Peñuelas et al., 2013; Vogel et al., 2021; Wang et al., 2014).
While several studies addressed these potential biotic and abiotic factors individually, none has examined simultaneous effects under field conditions. Here, we studied the leaching of dissolved organic and inorganic P and N in temperate beech forest soils as affected by seasons, nutrient status, and fertilization. For that, we used zero-tension lysimeters in three soil horizons that were artificially irrigated to standardize water flow. To cover different nutrient statuses, we took advantage of two sites of contrasting nutrient availability (a low-P sandy soil with low sorption capacities and a high-P loamy soil on basalt with high sorption capacities) that were subjected to a full factorial N×P fertilization experiment. To cover seasonal differences, we sampled leachates and soil solutions five times during 18 months.
With this study we aimed to quantify annual organic and inorganic P and N fluxes from organic layers and from the mineral topsoil. We primarily focused on P fluxes since the leaching of N has been extensively studied before. We hypothesized the following. (i) Leaching of dissolved inorganic P (DIP) and N (DIN) from organic forest floor layers will show stronger seasonal variations and stronger fertilization effects than dissolved organic P (DOP) and N (DON) as inorganic forms are more strongly controlled by mineralization and biotic uptake; in the mineral soil, seasonal and fertilization effects on leaching will be superimposed by sorption processes. (ii) The contribution of dissolved organic forms to total P and N leaching from organic layers will be greater at the low-P site due to stronger biotic uptake of inorganic nutrients. (iii) Fertilization with N and P removes nutrient limitations and thus will enhance P and N leaching, with effect sizes depending on the site due to differences in nutrient status and mineral assemblage; effect sizes will also differ between P and N due to differences in sorption and uptake affinities of the two nutrients. (iv) We expect synergistic effects of the combined N and P fertilization since N addition will remove N limitation for biologically mediated P mobilization processes.
2.1 Study site description
The study was conducted in two mature beech forest stands in Germany with contrasting parent material and P availability. The stands are dominated by 120–140 year old Fagus sylvatica (Lang et al., 2017). The soil with high P stock (Table 4) is a loamy Cambisol developed on basalt at Bad Brückenau (BBR; 809 m a.s.l.; 50.35∘ N, 9.27∘ E; mean annual precipitation: 1021 mm; referred to as “high-P site”) and a mull-like moder organic forest floor layer (Lang et al., 2017). The soil with the lower P stock is a sandy Cambisol, featuring a thicker organic layer and initial podzolization at Unterlüss (LUE; 115 m a.s.l.; 52.8∘ N, 10.3∘ E; mean annual precipitation: 779 mm; referred to as “low-P site”) that developed from glacial till. The organic forest floor layer is a mor-like moder (Lang et al., 2017). The organic and A horizons of the sites have greater C:P ratios at the low-P site but similar C:N ratios (Table 1, Sects. S1 and S8 in the Supplement). The differing P status is also mirrored by leaf P, being higher at the high-P site with 0.71±0.02 compared to 0.58±0.2 at the low-P site. Compared to the low-P site, the high-P site is characterized by a slightly higher pH (3.2 vs. 3.0), higher cation exchange capacity (371 vs. 108 meq kg−1), and higher proportion of aluminum and iron oxides (29.3 and 8.4 vs. 0.9 and 0.3 g kg−1 in the A horizon; Lang et al., 2017; Sect. S1). More details on the two sites are reported in Lang et al. (2017).
Table 1Soil organic carbon (SOC), total soil nitrogen (N), and total soil phosphorus (P) concentrations in litter, , and A horizons from the control treatment (unfertilized) at the high-P and the low-P site. Samples were taken in July 2019; values represent means ± standard error of three field replicates.
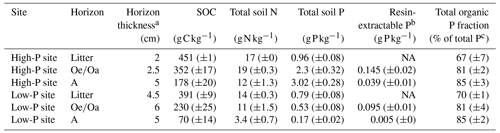
a Average horizon thickness above installed lysimeters (n=12). b Measured by Maja Siegenthaler on air-dried material, sampled in September 2018 from the same sites. c Total organic P determined based on Saunders and Williams (1955) and related to total P determined in H2SO4 extracts. NA: not available.
2.2 Experimental setup and in situ measurements
2.2.1 Fertilization
At each site, plots of 20 m×20 m were established with 20 m space in between. The plots were fertilized with either +N, +P, or +N+P, plus unfertilized control plots. They were replicated three times and arranged in blocks, resulting in a total of 12 plots per site (details on experimental setup are given in, for example, Hauenstein et al., 2020). A total of 5.0 g P m−2 was applied as KH2PO4 in a single dose in 2016 (0.6 % and 3.0 % of total P stock at the high-P and the low-P site; Table 5). This amount accounts for 0.6 % and 3 % of the total P stock within the top 1 m of soil plus forest floor at both sites (Table 5). To compensate for the K input to the P fertilized plots, 6.3 g m−2 was applied as KCl at the N-fertilized and control plots. Nitrogen was added as NH4NO3 in five equal doses from 2016 to 2018, amounting to a total of 15 g N m−2 (1.2 % and 2.1 % of total N stock at the high-P and the low-P site; Table 6).
2.2.2 Zero-tension lysimeters
Zero-tension lysimeters were installed beneath the litter layer, the fermented and/or humified horizon (), and the mineral topsoil (A) in November 2017 at both study sites. Zero-tension (or gravity) lysimeters mainly sample macro-pore flow during rain events and when the soil is draining to field capacity (Litaor, 1988), and, therefore, they provide a sample of soil solution exported from the respective soil horizon. The two uppermost lysimeters were 20 cm×20 cm acrylic glass plates with a mesh and three holes ensuring contact with the soil underneath. The third lysimeter in the mineral A horizon was a 19.5 cm×25.5 cm pod with a 3.3 cm high rim that was filled with three layers of acid-washed quartz sand of different grain sizes to ensure a hydrological continuum with the mineral soil, having a design similar to those used and described in greater detail by Makowski et al. (2020b). The lysimeters at the three depths were installed adjacent to each other. They were slightly inclined and connected to polyethylene (PE) bottles. Following installation, the plots were left to recover from disturbance for five months. For more details see Fetzer et al. (2021) and Sect. S2 in the Supplement.
2.2.3 Artificial irrigation and sampling
To measure solute leaching under standardized conditions with little storage time, an area of 1 m2 above the installed lysimeters at each plot was irrigated in April 2018, July 2018, October 2018, February–March 2019, and July 2019. The irrigation water was P- and N-free artificial rainwater matching the pH (5.5) and electrical conductivity (25 µS cm−1) of the average local throughfall of both sites. The artificial rainwater was applied at a constant rate of 20 using an Accu-Power sprayer (Birchmeier Sprühtechnik AG, Switzerland). This volume corresponds to 71 % and 61 % of the pore volume above the lysimeter in the mineral soil at the low-P and the high-P site, respectively. Soil solutions were collected over a period of 1.5 h from the start of the irrigation. The collection represents the “first flush” exporting the majority of P leached during rainfall events (Bol et al., 2016; Makowski et al., 2020a; Rinderer et al., 2021). The application rate represents maximum rainfall intensities at the study sites. Rainfall intensities larger than 20 have been observed once at the low-P site and three times at the high-P site during the last 10 years (Bayerische Landesanstalt für Wald und Forstwirtschaft (LWF) and Nordwestdeutsche Forstliche Versuchsanstalt (NW-FVA)). The amount of water added with irrigation corresponds to the average weekly precipitation at the high-P site and exceeds it by 33 % at the low-P site. In 2018, the three irrigations, totaling 60 L m−2, accounted for approx. 8 % of measured throughfall at the high-P site and 16 % at the low-P site (see Table 2). The two irrigations in 2019 added 40 L m−2. Overall, leachates were collected on five dates, at two sites, at three soil depths, and at four fertilization treatments applied in three blocks, resulting in 360 samples. Leachates were collected in PE bottles, stored in cooling boxes, and transported within 24 to 48 h to the laboratory. Samples were filtered through 0.45 µm nitrocellulose filters (GVS Life Sciences, Zola Predosa, Italy) and stored at 4 ∘C prior to analysis.
Table 2Annual water fluxes measured by zero-tension lysimeters below the litter horizon, the horizon, and the A horizon at the high-P site and the low-P site from July 2018 to July 2019. For comparison, the modeled fluxes from the same sites (both ICP Forest level II sites) are presented. Details are in Sect. S7 in the Supplement.
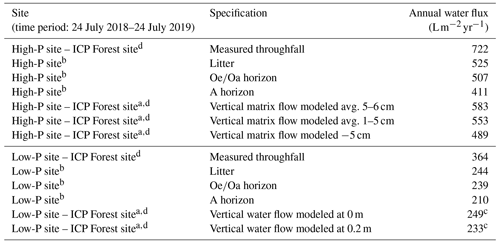
a Modeled with LWF-Brook90R model. b Includes average volume of artificial irrigation. c Time period: modeled only until 14 July 2019. d Data source: personal communication. Northwest German Forest Research Institute (NW-FVA) and Bayrische Landesanstalt für Wald und Forstwirtschaft (LWF).
To quantify annual natural water and solute flux, we additionally collected leachate draining into the lysimeters between samplings using polyethylene canisters. To keep evaporation minimal, the sampling containers were placed in a covered soil pit. Their water volume was determined gravimetrically.
2.3 Leachate analysis
Dissolved inorganic P was estimated spectrophotometrically as molybdate-reactive P (MRP) using the molybdate-ascorbic acid method (Murphy and Riley, 1962) and a flow injection analyzer (Scan+, Skalar, Breda, The Netherlands). Dissolved total P (DTP) concentrations in leachates were measured by inductive coupled plasma optical emission spectroscopy (ICP-OES; ULTIMA 2, Horiba Jobin-Yvon, Longjumeau, France). Dissolved organic P was calculated as the difference between DTP and DIP. Concentrations of dissolved organic carbon (DOC) and total nitrogen (TN) were measured with a FormacsHT/TN analyzer (Skalar). Dissolved nitrate concentrations were measured by ion chromatography (ICS 3000, Dionex, Sunnyvale, CA, USA) and dissolved ammonium concentrations with a FIAS 300 (PerkinElmer, Waltham, MA, USA). Electrical conductivity was measured with a LF 325 probe (WTW, Weilheim, Germany) and pH with a LL ecotrode (Metrohm, Herisau, Switzerland).
2.4 Soil samples
Soil samples of each plot and horizon were taken in July 2019 (2 sites × 12 plots × 3 horizons, total n=72). The material was freeze-dried and ground with a ball mill. The concentrations of N and C were measured using an automated elemental analyzer (Euro EA3000: Euro Vector, Pavia, Italy). Fused beads of sample aliquots ashed at 1000 ∘C were used to determine total P by sequential wavelength dispersive X-ray fluorescence spectroscopy (S8 Tiger Series 2, Bruker AXS, Karlsruhe, Germany). Phosphorus extraction with resin (PResin) (Hedley et al., 1982; Moir and Tiessen, 2007) was carried out using samples taken in autumn 2018 at 1:30 soil : solution ratio, followed by colorimetric phosphate determination (UV-1800, Shimadzu, Canby, USA) with the malachite green method of Ohno and Zibilske (1991). Total organic P and H2SO4 extractable total P were determined with the ignition method (Saunders and Williams, 1955). Two subsamples of 0.5 g of dried and <2 mm sieved soil were taken, one of them being ignited (550 ∘C, 2 h). Ignited and unignited soils were extracted with 25 mL of 0.5 M H2SO4 for 16 h and subsequently filtered. In both extracts inorganic P was determined with the malachite green method (Ohno and Zibilske, 1991). Organic P was calculated as the difference between the inorganic P in the ignited and unignited samples.
2.5 Soil microclimatic measurements
Soil temperature and soil moisture sensors were installed in April 2018 and remained in place throughout the experimental duration. Soil temperature (5 cm depth) was recorded by buried iButtons (iButton DS1922L-F5, Maxim Integrated, USA) installed at 9 out of 12 plots at 5 cm depth in the mineral soil at both sites. In the same plots, moisture was measured in the mineral soil at a depth of 5 cm with three EC-5 soil moisture sensors per plot (Decagon Devices Inc., Pullman, WA, USA, validated with gravimetrically measured soil moisture).
2.6 Data analysis and statistics
2.6.1 Artificial irrigation and sampling
For annual concentration averages, the four seasons were weighed equally, meaning that for the summer concentration values from summer 2018 and summer 2019 were averaged. Annual element fluxes (July 2018 to July 2019), being the mass transfer per area unit from one compartment into the next (), were estimated as the sum of daily water volume (L m−2) multiplied with their nutrient concentration (mg L−1, interpolated from the point measurements). To obtain continuous concentration data, the exponential relationship with soil temperature was used, expressed as Q10 values. The Q10 value is a measure of temperature sensitivity that is exponential and based on biological, chemical, or physicals reaction rates. Q10 values were calculated from the concentration data during the five irrigation events and the soil temperature delta between the samplings using non-linear regression. Results showed that Q10 values averaged to 4.3 and 3.2 for DIP and DIN and 1.8 and 3.2 for DOP and DON, respectively (Sect. S3 in the Supplement). Out of 360 possible sampled leachates, 12 were missing due to natural disturbances, such as damage to lysimeters by wild boars or mice. For calculation of annual fluxes, data of the missing 12 samples were extrapolated from the other two field replicates, considering the ratio between the replicates during the other samplings. For the interpolation of concentration data, first, the fitting parameters β0 and β1 were obtained according to Eq. (1), where T (∘C) is the soil temperature at 5 cm depth.
Second, Q10 values were calculated based on β1 according to Eq. (2) (Guelland et al., 2013).
This was done for each soil depth at each site independently (Q10 values and coefficient of determination in Sect. S3). Nutrient concentrations were interpolated according to Eq. (3).
where R1 (mg L−1) is the predicted daily nutrient concentration in the leachate, T2 (∘C) and R2 (mg L−1) are temperature and nutrient concentration measured at the sampling event, T1 (∘C) is the daily measured soil temperature, and Q10 is the estimated temperature dependency. Interpolation was done for each of the five samplings and then averaged. Predicted values for dissolved total N (DTN) and DTP differed on average less than 50 % from measured concentrations (Sect. S4 in the Supplement).
Daily water flux was interpolated from daily rainfall measurements, assuming that daily water volume from the zero-tension lysimeters was directly proportional to daily rainfall quantity (for water yields see Table 2). Measured water fluxes were compared with throughfall records and with water fluxes modeled using BROOK90R within the International Co-operative Programme on Assessment and Monitoring of Air Pollution Effects on Forests (ICP Forests; Table 2). As the plots received an artificial irrigation solution lacking N and P and, therefore, direct inputs from throughfall were excluded, the fluxes represent rather conservative estimates as compared to standard soil solution monitoring.
2.6.2 Calculation of balances and stocks
Balances were calculated for the horizon and the A horizon as the difference between input (influx from the horizon above) and the output (export flux to the horizon beneath).
Stocks were calculated by multiplying soil nutrient concentrations with fine earth densities. Nutrient concentrations were measured on soil samples taken in July 2019. Soil densities in the mineral horizon were determined with a core cutter of 1 L volume, and soil densities from the organic layers were taken from Lang et al. (2017).
2.6.3 Statistical analysis with linear mixed-effect models
We assessed treatment effects on element concentrations, fluxes, and element ratios using linear mixed-effects models with the lmer function from the lme4 package (Bates et al., 2015). In the case of the unbalanced data set of concentrations, type 3 ANOVA was used; p values were obtained with the lmerTest package (Kuznetsova et al., 2017). Due to non-normal distributed residuals, all tested parameters were log-transformed for the statistical analysis. According to the experimental design, we included site, +N, +P, season, and soil horizon with an interaction between site, +N, and +P, as well as an interaction between horizon and sampling event as fixed effects. Blocks (level of replication, n=3) and plots (fertilized squares) were used as random effects. For all statistical analyses, fixed effects were considered significant at p<0.05; p values between 0.05 and 0.1 were considered as marginally significant. Error estimates and error bars are standard errors of the means. All analyses were carried out using R version 3.6.3 (R Core Team, 2020).
3.1 Soil temperature and moisture
The two study years, 2018 and 2019, were exceptionally dry and warm. In 2018, annual precipitation amounted to 772 mm at the high-P site and 463 mm at the low-P site, which corresponded to only 68 % and 60 % of the long-term mean annual precipitation. This was even more pronounced for the period May to October, receiving only 50 % and 40 % of the long-term average at the high-P and the low-P site, respectively. Accordingly, volumetric water contents reached values below 10 % in both summers (Fig. 1). Maximum soil temperatures in summer were 18 ∘C at both sites (Fig. 1). In winter, the high-P site experienced longer periods with soil temperatures around 2 ∘C. At the low-P site, winter soil temperatures dropped that low only for a few days and were generally higher than at the high-P site. From July 2018 to July 2019, the average soil temperature was 9.8 ∘C at the low-P site and 7.9 ∘C at the high-P site (Fig. 1). There was a statistically significant negative linear relationship between soil temperature and soil moisture at the low-P site (R2=0.61, p<0.01) and at the high-P site (R2=0.28, p<0.01).
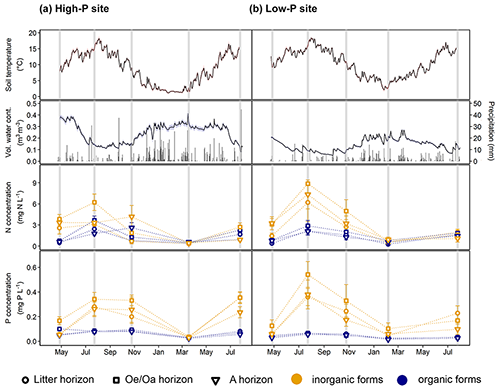
Figure 1Soil temperature, soil moisture, and concentration of inorganic and organic nitrogen (N) and phosphorus (P) form during the experimental duration at the high-P site (a) and the low-P site (b). Row 1 and 2: soil temperature and volumetric water content measured at 5 cm in the A horizon during the sampling period. Row 3 and 4: organic and inorganic N and P concentrations in leachates from litter, the horizon, and A horizon for each site and horizon (including fertilization treatments, n=12), at five samplings at the two sites. Error bars are standard error. Data for dissolved organic carbon can be found in Sect. S6.
3.2 Patterns of dissolved carbon, nitrogen, and phosphorus in leachates
Dissolved total P concentrations in leachates differed significantly between horizons (pHorizon<0.01; Sect. S5 in the Supplement), with the highest concentrations in leachates from the horizons. Despite strongly differing total soil P (Table 1), there was no statistical difference in DTP concentration between the two sites in any of the horizons (pSite=n.s., not significant). Concentrations of DTP in leachates varied strongly by season (pSeason<0.01; Fig. 1), being highest in summer (control means of horizon: 0.34 and 0.17 mg L−1 for the high-P and the low-P site) and lowest in winter (control means of horizon: 0.03 and 0.04 mg L−1 for the high-P and the low-P site). The increased DTP concentrations in summer were mainly due to increased DIP concentrations, exceeding those in winter on average by a factor of 20 at the high-P site and by a factor of 10 at the low-P site. Concentrations of DOP increased from winter to summer on average only by a factor of 2.5 at both sites. Averaged across sites, DOP constituted 49 % of DTP in spring and winter and 26 % of DTP in summer and fall. Overall, the contribution of DOP to DTP was higher at the high-P than at the low-P site (pSite=0.01).
Dissolved total N (DTN) concentrations varied between horizons (pHorizon<0.01) but not between sites (pSite=n.s. for all horizons). The highest concentrations occurred in leachates from the horizons. As for DTP, DTN concentrations showed strong seasonal differences (pSeason<0.01; Fig. 1), with the highest values in summer (control means of horizon: 5.0 and 3.0 mg L−1 for the high-P and the low-P site) and lowest values in winter (control means of horizon: 0.5 and 1.0 mg L−1 for the high-P and the low-P site). This pattern persisted for all N forms (pSeason<0.01), with DIN showing stronger seasonal variations than DON. While DIN concentrations were 9.5 and 3.5 times higher in summer than in winter at the high-P and the low-P site, respectively, DON concentrations increased from winter to summer by a factor of 3.8 at the high-P site and 3.4 at the low-P site.
Concentrations of DOC differed strongly between the two sites (pSite<0.01; Sect. S6 in the Supplement). They increased from the litter to the A horizon at the high-P site (pHorizon<0.01). At the low-P site, the highest concentrations occurred in leachates from the horizon. When averaged, DOC concentrations were twice as high at the low-P site than at the high-P site (pSite<0.01). The concentrations varied strongly by season (pSeason<0.01), and patterns differed between sites and horizons (pSeason:Horizon<0.01). At the low-P site, DOC concentrations in leachates from the horizons of the control plots were highest in spring (24.8 mg L−1) and lowest in autumn (10.3 mg L−1). At the high-P site, the DOC concentrations in leachates from the horizons of the control plots were highest in autumn (13.3 mg L−1) and lowest in winter (1.7 mg L−1).
3.3 Solute concentrations as related to temperature and moisture
The seasonal variations of solute leaching were mirrored by positive relationships of mean DIP, DOP, , , and DON concentrations with soil temperature (Sect. S3), being stronger for P than for the N and for inorganic than for organic forms (higher Q10 values for inorganic N and P forms and higher Q10 values for DTP than DTN; Sect. S3). The temperature–concentration relationships were better reflected by exponential than linear models (higher R2). Mean concentrations were negatively correlated with soil moisture, especially for DON and DIP. Relations were less strong to moisture than to soil temperature.
3.4 Annual fluxes of water and solutes
Annual measured vertical water fluxes, averaged over all horizons, amounted to 480 at the high-P site and 230 at the low-P site, corresponding to 57 %–73 % of measured throughfall. They decreased slightly with soil depth (Table 2). Modeling of water fluxes using LWF-Brook90 (done by the Northwest German Forest Research Institute (NW-FVA) and Bayrische Landesanstalt für Wald und Forstwirtschaft (LWF)) gave similar estimates, differing from the measured water fluxes by 4 to 79 (+2 % to +19 % of measured flux) at different depths at the low-P and the high-P site, respectively (Table 2).
The annual DTP fluxes in the control plots from all horizons ranged between 12 and 60 . They were similar at both sites (pSite=n.s. for all horizons), with the lowest values in the A horizons (pHorizon<0.01). The A horizons at both sited were P sinks as the balances (the difference between the fluxes into and out of a given horizons) were positive (Table 3). At the high-P site, the horizon was a source for P, exhibiting a negative balance, while at the low-P site the horizon was a P sink (Table 3). The DTP fluxes from the litter layer corresponded to 17 % of the litter P stock at the high-P site and 9 % of litter P stock at the low-P site (Table 3). The portion of P stock leached decreased with soil depth. Fluxes of DTP from the A horizon represented 0.1 % of the horizon's P stock at the high-P site and 0.2 % at the low-P site (Table 3). Annual DIP fluxes in the control plots did not differ between sites, whereas DOP fluxes were higher at the high-P than at the low-P site (pSite<0.01). From the horizon, mean DOP fluxes in the control plots were 20 at the high-P site and 6 at the low-P site. The contribution of DOP to DTP was more similar across sites, being on average 33 % at the high-P site and 21 % at the low-P site.
Table 3Comparison of dissolved organic, inorganic, and total phosphorus fluxes (DOP, DIP, DTP) from litter, , and A horizons with respective stocks (average of three field replicates taken in July 2019) of each horizon at the high-P site and the low-P site. Balances: the difference between the fluxes into and out of a given horizon; positive values reflect net accumulations and negative values net losses. Data from unfertilized plots (n=3). Means ± standard error (SE). Data for dissolved organic carbon can be found in Sect. S6.
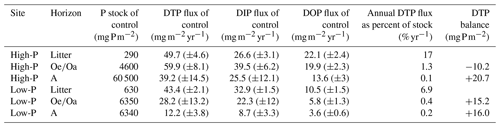
Effects of fertilization on fluxes of DTP and DIP differed among sites, with a comparable pattern for all horizons (pSite:N:P=0.05 and 0.07; Fig. 2). At the low-P site, DTP leaching from the horizon was increased by separate N and P additions (pP=0.07; horizon: +198 % and 156 % compared to leaching in the control plots) but not when combined. At the high-P site, +N, +P, and +N+P fertilization increased total P leaching ( horizon: +33 %, +51 %, and +75 % compared to the control). However, only the increase for the N×P treatment was statistically significant (pSite:N:P=0.07). Fertilization did not affect DOP at either site or soil depth (pSite:N:P=n.s., Fig. 2).
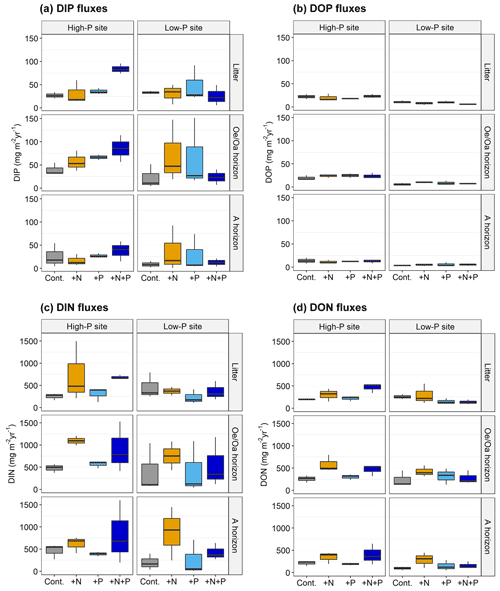
Figure 2Estimated annual fluxes of dissolved (a) inorganic and (b) organic phosphorus (DIP and DOP), as well as of dissolved (c) inorganic and (d) organic nitrogen (DIN and DON), from in the litter horizon, the horizon, and the A horizon at the high-P site and the low-P site, as affected by N addition (+N), P addition (+P), and the combined N and P addition (+N+P), as compared to the control (Cont.). The annual flux was estimated for the time period July 2018 to July 2019. Data for dissolved organic carbon can be found in Sect. S6.
Fluxes of DTN in control plots ranged between 292 and 734 and were similar at the two sites (pSite=n.s. for all horizons) and differed between horizons (pHorizon=0.01). The high-P site had the smallest fluxes in the litter layer, while at the low-P site the smallest fluxes occurred in the A horizon (Table 4). Since N-free rainwater was applied, the fluxes basically reflect N release from soils. The average contribution of DON to DTN was 36 % at both sites. There were differences between sites in distribution of and . At the high-P site, 20 % of DTN leached was and 44 % . The contributions of and were each 30 % at the low-P site. Fertilization affected leaching at sites and horizons in a similar way: N addition increased the DTN leaching (pN<0.01); P addition alone did not affect leaching (pP=0.40) (Fig. 2); N×P addition increased leaching at the high-P but not at the low-P site.
Table 4Comparison of dissolved organic, inorganic, and total nitrogen fluxes (DON, DIN, DTN) from litter, , and A horizons with respective stocks (average of three field replicates taken in July 2019) of each horizon at the high-P site and the low-P site. Balances: the difference between the fluxes into and out of a given horizon; positive values reflect net accumulations and negative values net losses. Data from unfertilized plots (n=3). Means ± standard error (SE). Data for dissolved organic carbon can be found in Sect. S6.
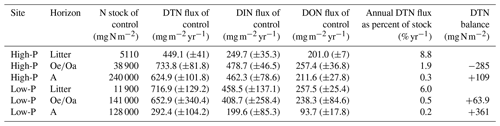
Annual DOC fluxes in control plots ranged between 1.68 and 3.40 (Sect. S6). They were higher at LUE and highest in the horizon (pSite=0.02, pHorizon<0.01) at both sites. Fertilization affected DOC fluxes at the sites differently (pSite:N=0.05). At the high-P site, DOC leaching increased from all horizons upon N fertilization. At the low-P site, fertilization only affected the A horizon, where DOC leaching was increased by all fertilization treatments () on average by a factor of 1.6 compared to the control treatment.
3.5 Stoichiometry of inorganic and organic nitrogen and phosphorus leaching
Ratios of DTN:DTP and DIN:DIP in leachates and soil solutions increased with soil depth from litter to A horizon (pHorizon<0.1 and pHorizon=0.1). On average, ratios were greater at the low-P than at the high-P site (pSite=0.09 and 0.07). Concentrations of DOC and DOP correlated significantly, with stronger correlations at the high-P site than at the low-P site. Ratios of DOC:DOP in control plots ranged between 85 and 605 (Sect. S8). Mean DOC:DON ratios ranged from 7 to 21 and mean DON:DOP ratios from 9 to 37 (Sect. S8). The ratios were significantly higher at the low-P than at the high-P site (pSite<0.01), paralleling the C:N and C:P ratios in soil (Table 1). However, the correlations between ratios in leachates and soils were not significant (Sect. S9 in the Supplement). Stocks of PResin, as a measure of available P, did only correlate with leached DIP in autumn (October 2018, R2=28, p=0.06) and winter (February–March 2019, R2=33, p=0.03). All element ratios in leached DOM showed a pronounced seasonality (pSeason<0.01) with smaller DOC:DON ratios and DOC:DOP ratios but higher DON:DOP ratios in summer and fall (Fig. 3). DIN:DIP ratios were highest in spring at both sites (Fig. 3).
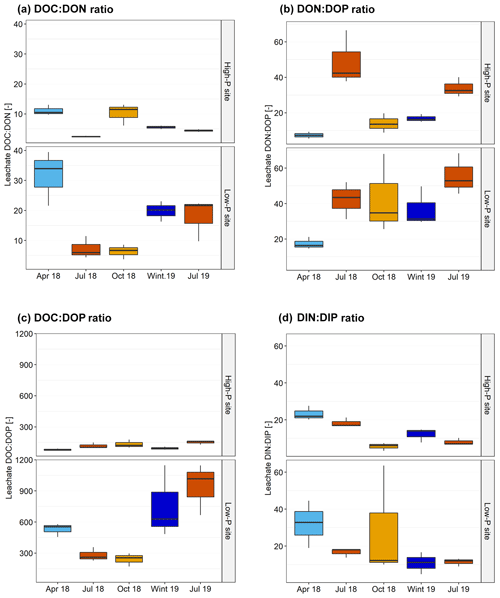
Figure 3Temporal variations in ratios among dissolved organic carbon (DOC), nitrogen (DON), and phosphorus (DOP), as well as dissolved inorganic N (DIN) and P (DIP) in leachates, from the horizon at the high-P site and the low-P site. The same seasonal patterns were observed for the litter and the A horizon. Data averaged over fertilization treatments, and boxplots depict variation from three field replicates. Wint 19 = February–March 2019.
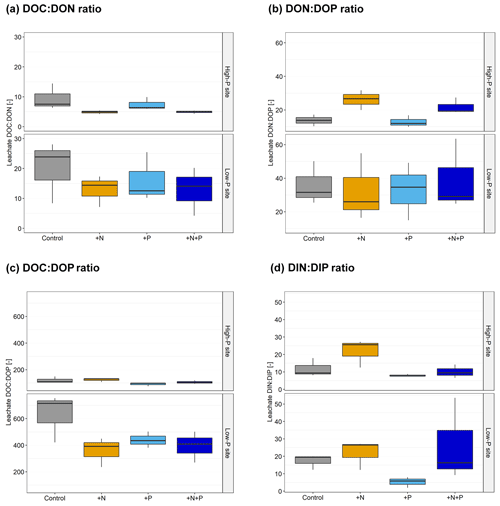
Figure 4Ratios of dissolved organic carbon (DOC), dissolved organic and inorganic nitrogen (DON and DIN), and dissolved organic and inorganic phosphorus (DOP and DIP) in leachates from the horizon as affected by +N, +P, and +N+P fertilization at the high-P and the low-P site. Data averaged before over seasons (seasons were equally weighted), and the boxplots depict variation from the three field replicates.
Nitrogen addition decreased DOC:DON ratios significantly (pN<0.01; Fig. 4) and increased the DON:DOP and DIN:DIP ratios (pN<0.01). Fertilization with P had no effect at the high-P site but caused decreases in DON:DOP and DIN:DIP ratios at the low-P site (pSite:P=0.04 and pSite:N:P=0.06).
4.1 Seasonal patterns
In agreement with our hypothesis, DIP concentrations varied more strongly by season than DOP. Concentrations of DIP represent the net result of release and retention processes, and thus, seasonal variations can be attributed to changes (i) in mineralization rates of SOM, (ii) in demand and uptake by plants and microorganisms, (iii) in sorption kinetics, or (vi) due to the rewetting, especially of dry soil during summer. Demand and uptake of phosphate are greatest in summer, and consequently the activity of phosphomonoesterases was found to be substantially higher in summer than in winter in the same soils (Fetzer et al., 2021). Also, desorption of phosphate increases with temperature and sudden rewetting of dry soil can cause desorption as well (Barrow, 1983), both potentially increasing DIP concentrations in summer. However, we assume that P from lysed microbial cells following drying–rewetting most strongly contributed to the summer peak of P concentrations. In support, there was a strong decline of DOC:DON ratios and DOC:DOP ratios from spring to summer 2018, as well as a smaller summer peak of DIN than of DIP in forest floor leachates (Fig. 3). The decline in these element ratios mirror a higher share of microbial metabolites in the leachates since released microbial cytoplasm has very narrow ratios of at the same sites (Siegenthaler et al., 2021). The lysis of microbial cells leads to a pulse of N and P from microbial metabolites (Gao et al., 2020; Schimel, 2018). In our study, the N and P summer peak was more pronounced at the low-P site, which experienced a longer and stronger dry phase than the high-P site and resulted in a drop of DOC:DON ratios from 28 in spring to ratios below 10 in summer. Artificial irrigation during the dry summer of 2018 with 20 mm h−1 could have promoted the drying and rewetting effect by rapidly leaching DIP and DOP, reducing the time for a biological uptake or sorption. This effect was not observed in a microcosm study, where soils of the same sites were subjected to drying and rewetting (Gerhard et al., 2021). However, in their study, the drying was only moderate, and the focus was laid on the mineral soil with lower SOM contents and hence less microbial biomass that can be released upon drying and rewetting.
In contrast to DIP, DOP shows similar concentrations throughout the year and hence a low temperature dependency. In agreement, DON also varied less by season than DIN. We relate the low temperature effects to the canceling out of the production and mineralization of DOP and DON, which – as they are both microbial processes – are temperature sensitive. An analogous conclusion has been drawn in soil warming studies in the laboratory and field, where leaching of DOC – correlating with DOP in our study – showed less of a response to temperature than respiratory processes (Gödde et al., 1996; Hagedorn et al., 2010; Müller et al., 2009).
4.2 Link of leaching rates to site properties
We have expected that DTP fluxes would be higher in the high-P than in the low-P soil. In contrast to our hypothesis, there was no statistically significant difference in DTP fluxes between the sites in all horizons. The surprisingly negligible site effects could result from sorption that could have balanced out differences in the P release. Generally, phosphate can sorb to charged surfaces, which represents a dominant retention mechanism in mineral soils (Barrow, 1983; Berg and Joern, 2006; Rechberger et al., 2021). Sorption seemed particularly strong in the mineral soil at the high-P site, which had higher contents of clay and aluminum and iron oxides that provide more sorptive mineral surfaces than the sandy low-P site with bleached quartz grains (Lang et al., 2017). The stronger sorption at the high-P site is supported by the smaller proportion of the annual DTP flux compared to the P stock in the A horizon at the high-P site (Table 3). Sorption might also occur in the organic horizons, especially in the Oa horizons, as they can contain minerals due to aeolian deposition or bioturbation, with the latter being more pronounced at the high-P site and showing signs of high faunal activity. Phosphate can also sorb via cation bridges in the organic horizons (Gaume et al., 2000; Gerke and Hermann, 1992; Rechberger et al., 2021). Here, phosphate competes for substantially smaller amounts of sorptive minerals than in the mineral soil, which is largely occupied by organic matter (Rechberger et al., 2021). As a result, sorption in the organic horizons is less important than in mineral horizons. Nevertheless, our assessment showed that the thick horizon was a sink for P at the low-P site (Table 3), which we primarily relate to biotic uptake under P-poor conditions. However, unexpectedly, also in the litter and the horizon, P leaching did not statistically differ between sites. This finding can be explained by a canceling out of the potentially higher P release from the organic layer at the high-P site (reflected in higher PResin contents), by the greater thicknesses of the organic layers at the low-P site, and hence by a greater reservoir from which P can be mobilized. We presume that the greater P stock in the organic layers at the low-P soil (Table 3) is an inherent site property linked to low-P sandy parent material that promoted accumulation of organic material on top of mineral soils due to low biological activity (Hauenstein et al., 2018). Consequently, P fluxes in relative terms – when compared to P stocks – were larger from both organic horizons in the high-P soil than in the low-P soil (Table 3) due to thinner horizons. In contrast to the organic layers, P leaching from A horizons relative to P stocks was twice as high in the low-P than in the high-P soil possibly due to smaller sorptive retention (Table 3). The smaller P leaching from the A horizon at the high-P site will foster the difference in P storage between the two soils in the long-run.
In contrast to DTP fluxes, we expected a higher contribution of DOP to total P leaching at the low-P site because DOP, as part of DOM, is leached during SOM processing, while phosphate is assumed to be more efficiently retained by plant and microbial uptake in low-P forest ecosystems (Hedin et al., 2003). However, contrary to these expectations, the absolute DOP fluxes, as well as their contribution to total P fluxes, were higher in the high-P soil than in the low-P soil. We relate this finding to the higher soil organic P content at the high-P site (Table 1), resulting in a smaller C:P ratio of SOM in all horizons, which translates also into smaller DOC:DOP ratios.
4.3 Fluxes
Dissolved total P concentrations in the leachates following the experimental irrigation used to overcome site and weather variations corresponded closely to those measured in an adjacent plot receiving natural precipitation. While the annual average concentration in the leachate from the organic layer (only control plots) following irrigation was 0.19 mg P L−1 at the low-P site and 0.24 mg P L−1 at the high-P site, those under natural precipitation were 0.56 mg P L−1 at the low-P site and 0.26 mg P L−1 at the high-P site (Klaus Kaiser, unpublished data; mean over the 4 previous years). We therefore assume that concentrations and fluxes estimated here are representative for the sites. The DTP fluxes ranged between 12 and 60 across all horizons (Table 3), which compare well with the P fluxes measured in other forest ecosystems, ranging from 9 to 62 (Qualls, 2000; Fitzhugh et al., 2001; Hedin et al., 2003; Piirainen et al., 2007; Sohrt et al., 2019; Rinderer et al., 2021b). The P fluxes observed here are about 1 magnitude lower than those determined in a laboratory study with isolated horizons from the same sites (ranging from 70 to 320 across all horizons; Brödlin et al., 2019a). The higher P release in the laboratory than in situ can be attributed to the regular leaching, the longer as well as more complete contact of soil with excessive artificial rainwater, and the lack of uptake by plants, rather reflecting potential release rates. The comparison between these two approaches demonstrates that in soil continuous release and immobilization of P takes place, but only a small proportion of released P eventually becomes leached.
How relevant are these P leaching fluxes? The P export from the horizon observed in the present study is on the same order of magnitude of reported P inputs with bulk precipitation or throughfall at another German beech forest (60 ; Sohrt et al., 2019). In Germany, atmospheric P inputs may largely originate from fertilized agricultural land, but in general, information on P deposition is still scarce (Bol et al., 2016; Tipping et al., 2014; Vogel et al., 2021; Wang et al., 2014) and not available for the two study sites. The P fluxes from the A horizon at the high-P and at the low-P site are approx. 150 % and 50 % of reported atmospheric P deposition in Germany. In comparison, N fluxes from the A horizons are only 30 % and 28 % of atmospheric N depositions that have been measured at the same high-P and low-P site (Brumme et al., 2021; NW-FVA, 2020), which might even represent an underestimate as atmospheric N inputs are generally not completely captured due to canopy exchange processes (Talkner et al., 2010). Therefore, it seems that the leaching losses relative to atmospheric inputs are greater for P than for N, which likely fosters the nutrient imbalances between N and P (Peñuelas et al., 2013).
Since the P fluxes decreased towards the A horizon, they likely decrease further in deeper mineral soils due to lower release of P and higher retention of DOP and DIP by sorption to reactive minerals (Barrow, 1983; Berg and Joern, 2006; Brödlin et al., 2019a), as well as uptake by roots and microorganisms in subsoils. Consequently, P leaching losses from entire soil profiles are likely even smaller than from the topsoil observed here. This is supported by annual export rates of P measured in the runoff of forested catchments in Germany of 2–9 (Julich et al., 2017; Sohrt et al., 2019). Therefore, P losses from the entire soil profile likely do not exceed P depositions, contradicting the idea of these forest ecosystems being depleted in P due to leaching, as suggested by decreasing leaf P concentrations in beech trees (Lang et al., 2017; Talkner et al., 2015). The estimated P fluxes from the horizon are even 1 order of magnitude lower than the P input via annual litterfall, which amounts to 229 at the high-P site and 156 at the low-P site (Lang et al., 2017). This implies that a large proportion of litterfall P must be taken up by plants and microorganisms. However, without the current “P pollution” and at the centennial and millennial timescale, relevant for soil and ecosystem development, the small P leaching in the A horizon, with 0.1 % and 0.2 % of its P stock being leached during 1 year (Table 3), is important for redistributing P within the soil profile. When leached from the entire profile or exported via lateral flow (Rinderer et al., 2021), it may also contribute to the depletion of the ecosystems in P (e.g. Hedin et al., 2003; Richardson et al., 2004).
4.4 Fertilization effects
Phosphorus fluxes. In agreement with our hypothesis, fertilization had stronger effects on the leaching of inorganic than on organic P and N. Also, the fertilization effects were stronger at the low-P than at the high-P site. However, counterintuitively, DIP leaching increased more strongly upon fertilization with N than with P at the low-P site. We relate this to the overcoming of N limitation for the production of enzymes hydrolyzing organic P, which is indicated by the increased activity of phosphomonoesterases after N addition in the studied leachates and soil solutions (Fetzer et al., 2021). Similar effects have been reported for grassland soils (Widdig et al., 2019) and in mesocosm experiments with soils from the low-P site (Holzmann et al., 2016). Also, Siegenthaler et al. (2021) showed that N addition was the main factor driving changes in bacteria and fungi communities at the low-P site. Finally, N fertilization might have stimulated overall microbial processing of SOM (Griepentrog et al., 2014; Hagedorn et al., 2012), which is supported by observed increased DOC leaching at the high-P site (Sect. S6).
Although the amount of added P was much higher than that of N when compared to soil N and P stocks (Tables 5 and 6), P fertilization affected P leaching less than N fertilization. In the horizon, P addition corresponded to 109 % and 79 % of the P stock at the high-P and the low-P site, while N addition was 39 % and 11 % of the N stock at the high-P and the low-P site. Nevertheless, N leaching increased more than P leaching (Tables 5 and 6). Most probably, a large fraction of added P was either rapidly sorbed to reactive minerals, taken up by plants (Hauenstein et al., 2020), or immobilized by soil microorganisms (Bünemann et al., 2012). Microbial biomass in the horizons at two study sites had very low microbial C:P ratios of 12 before the nutrient addition (Siegenthaler et al., 2021). Fertilization with P did not affect the microbial P in the horizons at the high-P site but increased it at the low-P site with the +N+P treatment compared to the +N treatment (Siegenthaler et al., 2021), strongly suggesting microbial immobilization of added P. In temperate German beech forests of the same region, Zederer et al. (2017) found that in horizons with average soil C:P ratios of 390, microbial biomass had C:P ratios of 13 and comprised approx. 30 %–50 % of total P. This indicates that organic layers have a high capacity to retain microbially bound P. Our leaching study shows that very little of retained P is re-released into soil solution, indicating that P retention is effective in the organic layer and not only in mineral soils where sorption is higher.
Table 5Soil phosphorus (P) stocks from the control plots (n=3) at the high-P and the low-P site for the litter and the horizon, as well as added amounts of P, and the change in annual leaching of dissolved total P (DTP) and in element stocks due to fertilization. Other treatments are given in Sect. S10 in the Supplement.
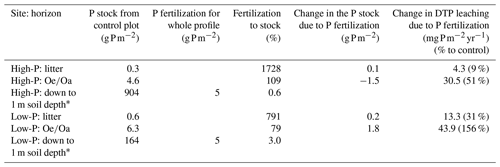
∗ From Lang et al. (2017), soil and forest floor.
Table 6Soil nitrogen (N) stocks from the control plots (n=3) at the high-P and the low-P site for the litter and the horizon, as well as added amounts of N, and the change in annual leaching of dissolved total N (DTN) and in element stocks due to fertilization. Other treatments are given in Sect. S10.
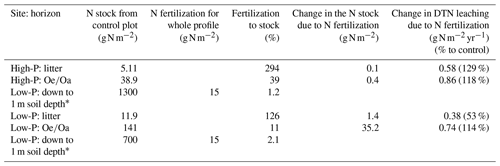
∗ From Lang et al. (2017), soil and forest floor.
In the longer term, however, continuous P retention in the organic layer, presumably by microbial P immobilization, decreases soil total C:P ratios as already observed for the horizon at the low-P site. Here, the 3-year-long P fertilization decreased the measured soil total C:P ratios from 597 (control) to 354 (P addition; Sect. S8), and P stocks increased by 0.2 and 1.8 g P m−2 in the litter and in the horizon (Table 5). The decline in soil C:P ratios might enhance net P release and leaching, as observed in leaching studies with the same soils by Brödlin et al. (2019a). Although the low-P soil is more retentive for P due to its higher soil total C:P ratios and hence a higher P demand by plants and microorganisms, effects of P fertilization on P leaching were greater at the low-P than at the high-P site (+156 % vs. +51 % in the horizon; Table 5). We attribute this apparent conflict to the low N availability at the low-P site and thus a possible co-limitation by N which reduced the biological P uptake.
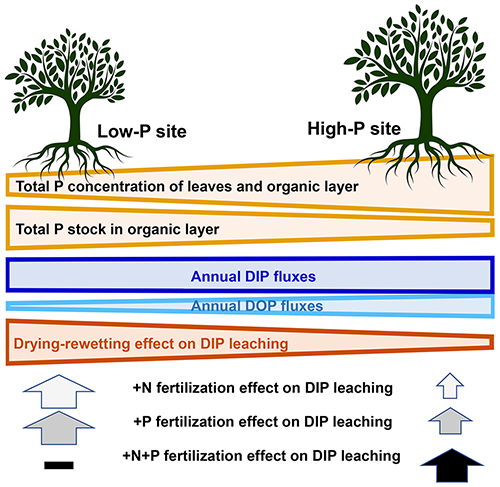
Figure 5Summary graph of the effects of fertilization and drying–rewetting on dissolved inorganic and organic phosphorus (DIP and DOP) annual fluxes from the organic layers, dependent on the two study sites that exhibit contrasting characteristics.
The effects of the combined N and P addition on P leaching depended on the site. It only increased P leaching at the high-P site. Here, the effects of fertilization with N and P were largely additive but not synergistic, i.e. the positive effect of N addition (+33 % in the horizon), presumably by supporting increased phosphatase activity, and the enhanced P leaching with P fertilization (+51 % in the horizons) resulted in an overall increase of 75 %. In contrast, at the low-P site, there was an antagonistic interaction as the combined addition did not affect P leaching as compared to the control and even reduced it as compared to the fertilization with N only. This is in accordance with results by Siegenthaler et al. (2021), who observed increased microbial P at the low-P site but only for the +N+P treatment. Here, we can only speculate about the mechanisms behind this finding as P leaching is the net product of various counteracting mechanisms. One reason could be that only the combined addition removed nutrient limitation and promoted an increase in microbial biomass at the low-P site, which might have increased microbial P immobilization and thus reduced P leaching. Laboratory fertilization experiments with mineral soils from the same sites indicated mostly microbial C and P limitation for the low-P soil, and N and N–P co-limitation for the high-P soil (Chen et al., 2019; Rodionov et al., 2020). However, a study on P concentrations in xylem sap on the fertilized plots likewise observed an antagonistic interaction between P concentrations in xylem after the +N+P treatment at the low-P site but a significant synergistic effect of the combined +N+P fertilization at the high-P site (Hauenstein et al., 2020), supporting the theory of a co-limitation of N and P at the low-P site.
Nitrogen fluxes. In contrast to DIP, DIN leaching responded consistently positive to N fertilization (Table 6), which signifies that the N additions led to an N surplus in both soils. Phosphorus addition did not affect N leaching, indicating that the release of N is not related to P availability. This is in accordance with N fertilization experiments in grasslands (Schleuss et al., 2021). Although to a lesser extent than for DIN, N fertilization increased DON concentrations, which went along with significant decreases in DOC:DON ratios (Fig. 4). This decline is consistent with findings of lower C:N ratios in extracted OM after +N+P fertilization in grasslands (Neff et al., 2000), suggesting that added N was immobilized in the solubilizable SOM pool (Schleuss et al., 2021) and/or that N fertilization might have increased the contribution of microbial metabolites with low C:N ratios to DOM. In support, N fertilization of a young beech and spruce forest promoted the production of new fungal residues in the soil and hence fungal turnover (Griepentrog et al., 2014), potentially leading to the release of fungal metabolites.
Firstly, our study shows that climatic conditions were the strongest drivers of N and P leaching. In the exceptionally hot and dry summer of 2018, DIP concentrations were particularly high, while DOC:DON and DOC:DOP ratios were extraordinary low, which strongly suggests N and P release from lysed cells upon drying–rewetting. This effect was stronger at the low-P site. The magnitude of seasonal variation differed between dissolved organic and inorganic compounds, especially in the case of P. Here, DIP but not DOP showed a pronounced summer peak. These results suggest that changes in seasonal conditions towards more frequent extremes can alter stoichiometries and the nutrient forms in leachates of forest soils, causing accelerated DIP leaching.
Secondly, the differences in P leaching between sites were smaller than expected (Fig. 5). The organic layers at the low-P soil with lower P concentrations had similar DIP fluxes than the high-P soil as the pool sizes of the organic layer were larger under low-P conditions and “compensated” for the lower P release per unit organic matter. In the mineral soil, the high-P site had more charged surfaces and hence stronger sorption capacity that likely influenced the magnitude of leaching. Phosphorus leaching from the A horizons relative to P stocks was twice as high in the low-P than in the high-P soil, fostering the difference in P storage between the two soils in the long-run.
Thirdly, our estimated P fluxes were comparable to reported atmospheric P inputs, implying that these forest ecosystems likely do not deplete in P by leaching as long as there are atmospheric P inputs on similar orders of magnitude. Phosphorus fluxes from the A horizons, relative to reported P deposition, were much higher than the N fluxes, relative to reported N depositions, which lends support that leaching contributes to the nutritional imbalance between N and P due to divergent atmospheric inputs of the two elements.
Fourthly, we showed that intrinsic soil N and P availability determined the effect of N and P addition on nutrient release and leaching. Fertilization effects were additive at the high-P site, with significant increases in P leaching only after +N+P addition. At the low-P site, fertilization effects were antagonistic, with only +N and +P leading to an increase in P leaching, hinting at a N and P co-limitation of microorganisms at the low-P site. Additionally, effect sizes of the increase in leaching due to nutrient addition were higher in the low-P system, and hence, the low-P ecosystem was more responsive to fertilization than the high-P system. Overall, this implies that a low-P ecosystem is likely more vulnerable to environmental future changes.
All datasets for this study are online at EnviDat: https://doi.org/10.16904/envidat.234 (Fetzer and Hagedorn, 2021).
The supplement related to this article is available online at: https://doi.org/10.5194/bg-19-1527-2022-supplement.
FH, KK, EF, and JF contributed to the conception and design of the study. JF and FH conducted the field experiments. JF and KK did the analytical measurements. JF did the data analyses and the data visualization and wrote the first draft of the manuscript. All authors contributed to the interpretation of the findings and to the manuscript revision, and they read and approved the submitted version.
At least one of the (co-)authors is a member of the editorial board of Biogeosciences. The peer-review process was guided by an independent editor, and the authors also have no other competing interests to declare.
Publisher's note: Copernicus Publications remains neutral with regard to jurisdictional claims in published maps and institutional affiliations.
We thank all people involved in establishing and maintaining the monitoring sites (“Bayerische Landesanstalt für Wald und Forstwirtschaft” (LWF) and “Nordwestdeutsche Forstliche Versuchsanstalt” (NW-FVA)), as well as the fertilization experiment (Jaane Krüger and many more). Many thanks to Roger Köchli for great help during the many field trips and Dominik Brödlin, Anna Missong, Claudia Schmidt-Cotta, Irina Vögtli, Lukas Jansing, and Daniel Kaiser for support in the field. We thank the WSL central laboratory (Alessandro Schlumpf, Karin von Känel, Janka Bollenbach, Ursula Graf, Daniele Pezzotta) and the WSL forest soil laboratory (Alois Zürcher, Behzad Rahimi, Daniel Christen) for chemical analyses and support. Alexandra Boritzki and Pauline Winkler at the Halle soil laboratory and Laurie Schönholzer at the Eschikon laboratory are gratefully acknowledged for phosphorus and carbon analyses. We thank Maja Siegenthaler for the PResin data.
This research has been supported by the Swiss National Science Foundation (SNF) (project no. 171171) that supports Jasmin Fetzer and Frank Hagedorn, as well as the German Research Foundation (DFG) that funded the Priority Program SPP 1685 “Ecosystem nutrition: Forest strategies for limited phosphorus resources” (grant no. KA1673/9-2) supporting Jasmin Fetzer and Klaus Kaiser.
This paper was edited by Ivonne Trebs and reviewed by Lukas Kohl and one anonymous referee.
Achat, D. L., Augusto, L., Gallet-Budynek, A., and Bakker, M. R.: Drying-induced changes in phosphorus status of soils with contrasting soil organic matter contents – Implications for laboratory approaches, Geoderma, 187–188, 41–48, https://doi.org/10.1016/j.geoderma.2012.04.014, 2012.
Alvarez-Cobelas, M., Sánchez-Carrillo, S., Angeler, D. G., and Sánchez-Andrés, R.: Phosphorus export from catchments: A global view, J. N. Am. Benthol. Soc., 28, 805–820, https://doi.org/10.1899/09-073.1, 2009.
Anderson, B. H. and Magdoff, F. R.: Relative movement and soil fixation of soluble organic and inorganic phosphorus, J. Environ. Qual., 34, 2228–2233, https://doi.org/10.2134/jeq2005.0025, 2005.
Augusto, L., Achat, D. L., Jonard, M., Vidal, D., and Ringeval, B.: Soil parent material – A major driver of plant nutrient limitations in terrestrial ecosystems, Glob. Change Biol., 23, 3808–3824, https://doi.org/10.1111/gcb.13691, 2017.
Backnäs, S., Laine-Kaulio, H., and Kløve, B.: Phosphorus forms and related soil chemistry in preferential flowpaths and the soil matrix of a forested podzolic till soil profile, Geoderma, 189–190, 50–64, https://doi.org/10.1016/j.geoderma.2012.04.016, 2012.
Barrow, N. J.: A mechanistic model for describing the sorption and desorption of phosphate by soil, J. Soil Sci., 34, 733–750, https://doi.org/10.1111/ejss.12198_2, 1983.
Bates, D., Mächler, M., Bolker, B., and Walker, S.: Fitting Linear Mixed-Effects Models using lme4, J. Stat. Softw., 67, https://doi.org/10.18637/jss.v067.i01, 2015.
Berg, A. S. and Joern, B. C.: Sorption dynamics of organic and inorganic phosphorus compounds in soil, J. Environ. Qual., 35, 1855–1862, https://doi.org/10.2134/jeq2005.0420, 2006.
Birch, H. F.: The effect of soil drying on humus decomposition and nitrogen availability, Plant Soil, 10, 1, 9–31, 1958.
Blackwell, M. S. A., Brookes, P. C., de la Fuente-Martinez, N., Gordon, H., Murray, P. J., Snars, K. E., Williams, J. K., Bol, R., and Haygarth, P. M.: Cha. 1 – Phosphorus Solubilization and Potential Transfer to Surface Waters from the Soil Microbial Biomass Following Drying–Rewetting and Freezing–Thawing, in: Sparks, D. L. B. T.-A. A., Advances in Agronomy v106, Vol. 106, 1–35, Academic Press, https://doi.org/10.1016/S0065-2113(10)06001-3, 2010.
Bol, R., Julich, D., Brödlin, D., Siemens, J., Kaiser, K., Dippold, M. A., Spielvogel, S., Zilla, T., Mewes, D., von Blanckenburg, F., Puhlmann, H., Holzmann, S., Weiler, M., Amelung, W., Lang, F., Kuzyakov, Y., Feger, K. H., Gottselig, N., Klumpp, E., Missong, A., Winkelmann, C., Uhlig, D., Sohrt, J., von Wilpert, K., Wu, B., and Hagedorn, F.: Dissolved and colloidal phosphorus fluxes in forest ecosystems – an almost blind spot in ecosystem research, J. Plant Nutr. Soil Sc., 179, 425–438, https://doi.org/10.1002/jpln.201600079, 2016.
Borken, W. and Matzner, E.: Reappraisal of drying and wetting effects on C and N mineralization and fluxes in soils, Glob. Change Biol., 15, 808–824, https://doi.org/10.1111/j.1365-2486.2008.01681.x, 2009.
Brödlin, D., Kaiser, K., and Hagedorn, F.: Divergent patterns of carbon, nitrogen, and phosphorus mobilization in forest soils, Front. For. Glob. Chang., 2, 66, https://doi.org/10.3389/ffgc.2019.00066, 2019a.
Brödlin, D., Kaiser, K., Kessler, A., and Hagedorn, F.: Drying and rewetting foster phosphorus depletion of forest soils, Soil Biol. Biochem., 128, 22–34, https://doi.org/10.1016/j.soilbio.2018.10.001, 2019b.
Brumme, R., Ahrends, B., Block, J., Schulz, C., Meesenburg, H., Klinck, U., Wagner, M., and Khanna, P. K.: Cycling and retention of nitrogen in European beech (Fagus sylvatica L.) ecosystems under elevated fructification frequency, Biogeosciences, 18, 3763–3779, https://doi.org/10.5194/bg-18-3763-2021, 2021.
Bünemann, E. K., Oberson, A., Liebisch, F., Keller, F., Annaheim, K. E., Huguenin-Elie, O., and Frossard, E.: Rapid microbial phosphorus immobilization dominates gross phosphorus fluxes in a grassland soil with low inorganic phosphorus availability, Soil Biol. Biochem., 51, 84–95, https://doi.org/10.1016/j.soilbio.2012.04.012, 2012.
Celi, L., De Luca, G., and Barberis, E.: Effects of Interaction of Organic and Inorganic P With Ferrihydrite and Kaolinite-Iron Oxide Systems on Iron Release, Soil Sci., 168, 479–488, https://doi.org/10.1097/01.ss.0000080333.10341.a4, 2003.
Chen, J., Seven, J., Zilla, T., Dippold, M. A., Blagodatskaya, E., and Kuzyakov, Y.: Microbial stoichiometry and turnover depend on nutrients availability in soil: A 14C, 15N and 33P triple labelling study, Soil Biol. Biochem., 131, 206–216, https://doi.org/10.1016/j.soilbio.2019.01.017, 2019.
Colman, B. P. and Schimel, J. P.: Drivers of microbial respiration and net N mineralization at the continental scale, Soil Biol. Biochem., 60, 65–76, https://doi.org/10.1016/j.soilbio.2013.01.003, 2013.
Davies, J. A. C., Tipping, E., Rowe, E. C., Boyle, J. F., Pannatier, E. G., and Martinsen, V.: Long-term P weathering and recent N deposition control contemporary plant-soil C, N, and P, Global Biogeochem. Cy., 30, 231–249, https://doi.org/10.1002/2015GB005167, 2016.
Dinh, M. V., Schramm, T., Spohn, M., and Matzner, E.: Drying–rewetting cycles release phosphorus from forest soils, J. Plant Nutr. Soil Sc., 179, 670–678, https://doi.org/10.1002/jpln.201500577, 2016.
Dinh, M.-V., Guhr, A., Spohn, M., and Matzner, E.: Release of phosphorus from soil bacterial and fungal biomass following drying/rewetting, Soil Biol. Biochem., 110, 1–7, https://doi.org/10.1016/j.soilbio.2017.02.014, 2017.
Elser, J. J., Bracken, M. E. S., Cleland, E. E., Gruner, D. S., Harpole, W. S., Hillebrand, H., Ngai, J. T., Seabloom, E. W., Shurin, J. B., and Smith, J. E.: Global analysis of nitrogen and phosphorus limitation of primary producers in freshwater, marine and terrestrial ecosystems, Ecol. Lett., 10, 1135–1142, https://doi.org/10.1111/j.1461-0248.2007.01113.x, 2007.
Fetzer, J. and Hagedorn, F.: Phosphorus and nitrogen leaching from beech forest soils, EnviDat [data set], https://doi.org/10.16904/envidat.234, 2021.
Fetzer, J., Loeppmann, S., Frossard, E., Manzoor, A., Brödlin, D., Kaiser, K., and Hagedorn, F.: Leaching of phosphomonoesterase activities in beech forest soils: consequences for phosphorus forms and mobility, Front. For. Glob. Chang., 4, 684069, https://doi.org/10.3389/ffgc.2021.684069, 2021.
Fitzhugh, R. D., Driscoll, C. T., Groffman, P. M., Tierney, G. L., Fahey, T. J., and Hardy, J. P.: Effects of soil freezing disturbance on soil solution nitrogen, phosphorus, and carbon chemistry in a northern hardwood ecosystem, Biogeochemistry, 56, 215–238, 2001.
Fröberg, M., Grip, H., Tipping, E., Svensson, M., Strömgren, M., and Kleja, D. B.: Long-term effects of experimental fertilization and soil warming on dissolved organic matter leaching from a spruce forest in Northern Sweden, Geoderma, 200–201, 172–179, https://doi.org/10.1016/j.geoderma.2013.02.002, 2013.
Frossard, E., Stewart, J. W. B., and Arnaud, R. J. St.: Distribution and mobility of phosphorus in grassland and forest soils of Saskatchewan, Can. J. Soil Sci., 69, 401–416, https://doi.org/10.4141/cjss89-040, 1989.
Gao, D., Bai, E., Li, M., Zhao, C., Yu, K., and Hagedorn, F.: Responses of soil nitrogen and phosphorus cycling to drying and rewetting cycles: A meta-analysis, Soil Biol. Biochem., 148, 107896, https://doi.org/10.1016/j.soilbio.2020.107896, 2020.
Gao, D., Bai, E., Yang, Y., Zong, S., and Hagedorn, F.: A global meta-analysis on freeze-thaw effects on soil carbon and phosphorus cycling, Soil Biol. Biochem., 159, 108283, https://doi.org/10.1016/j.soilbio.2021.108283, 2021.
Gaume, A., Weidler, P. G., and Frossard, E.: Effect of maize root mucilage on phosphate adsorption and exchangeability on a synthetic ferrihydrite, Biol. Fert. Soils, 31, 525–532, https://doi.org/10.1007/s003740000206, 2000.
Gerhard, L., Puhlmann, H., Vogt, M., and Luster, J.: Phosphorus leaching from naturally structured forest soilso is more affected by soil properties than by drying and rewetting, Front. For. Glob. Chang., 4, 1–14, https://doi.org/10.3389/ffgc.2021.543037, 2021.
Gerke, J. and Hermann, R.: Adsorption of orthophosphate to humic-Fe-Complexes and to amorphous Fe-Oxide, Z. Pflanz. Bodenkunde, 155, 233–236, https://doi.org/10.1002/jpln.19921550313, 1992.
Gödde, M., David, M. B., Christ, M. J., Kaupenjohann, M., and Vance, G. F.: Carbon mobilization from the forest floor under red spruce in the Northeastern U. S. A., Soil Biol. Biochem., 28, 1181–1189, https://doi.org/10.1016/0038-0717(96)00130-7, 1996.
Griepentrog, M., Bodé, S., Boeckx, P., Hagedorn, F., Heim, A., and Schmidt, M. W. I.: Nitrogen deposition promotes the production of new fungal residues but retards the decomposition of old residues in forest soil fractions, Glob. Change Biol., 20, 327–340, https://doi.org/10.1111/gcb.12374, 2014.
Guelland, K., Hagedorn, F., Smittenberg, R. H., Göransson, H., Bernasconi, S. M., Hajdas, I., and Kretzschmar, R.: Evolution of carbon fluxes during initial soil formation along the forefield of Damma glacier, Switzerland, Biogeochemistry, 113, 545–561, https://doi.org/10.1007/s10533-012-9785-1, 2013.
Hagedorn, F., Bucher, J. B., and Schleppi, P.: Contrasting dynamics of dissolved inorganic and organic nitrogen in soil and surface waters of forested catchments with Gleysols, Geoderma, 100, 173–192, https://doi.org/10.1016/S0016-7061(00)00085-9, 2001.
Hagedorn, F., Martin, M., Rixen, C., Rusch, S., Bebi, P., Zürcher, A., Siegwolf, R. T. W., Wipf, S., Escape, C., Roy, J., and Hättenschwiler, S.: Short-term responses of ecosystem carbon fluxes to experimental soil warming at the Swiss alpine treeline, Biogeochemistry, 97, 7–19, https://doi.org/10.1007/s10533-009-9297-9, 2010.
Hagedorn, F., Kammer, A., Schmidt, M. W. I., and Goodale, C. L.: Nitrogen addition alters mineralization dynamics of 13C-depleted leaf and twig litter and reduces leaching of older DOC from mineral soil, Glob. Change Biol., 18, 1412–1427, https://doi.org/10.1111/j.1365-2486.2011.02603.x, 2012.
Hannapel, R. J., Fuller, W. H., and Fox, R. H.: Phosphorus movement in a calcareous soil: II. Soil microbial activity and organic phosphorus movement, Soil Sci., 97, 421–427, 1964a.
Hannapel, R. J., Fuller, W. H., Bosma, S., and Bullock, J. S.: Phosphorus movement in a calcareous soil: l. Predominance of organic forms of phosphorus in phosphorus movement, Soil Sci., 97, 350–357, 1964b.
Harpole, W. S., Ngai, J. T., Cleland, E. E., Seabloom, E. W., Borer, E. T., Bracken, M. E. S., Elser, J. J., Gruner, D. S., Hillebrand, H., Shurin, J. B., and Smith, J. E.: Nutrient co-limitation of primary producer communities, Ecol. Lett., 14, 852–862, https://doi.org/10.1111/j.1461-0248.2011.01651.x, 2011.
Hauenstein, S., Neidhardt, H., Lang, F., Krüger, J., Hofmann, D., Pütz, T., and Oelmann, Y.: Organic layers favor phosphorus storage and uptake by young beech trees (Fagus sylvatica L.) at nutrient poor ecosystems, Plant Soil, 432, 289–301, https://doi.org/10.1007/s11104-018-3804-5, 2018.
Hauenstein, S., Nebel, M., and Oelmann, Y.: Impacts of Fertilization on Biologically Cycled P in Xylem Sap of Fagus sylvatica L. Revealed by Means of the Oxygen Isotope Ratio in Phosphate, Front. For. Glob. Chang., 3, 1–11, https://doi.org/10.3389/ffgc.2020.542738, 2020.
Hedin, L. O., Armesto, J. J., and Johnson, A. H.: Patterns of nutrient loss from unpolluted, old-growth temperate forests: evaluation of biogeochemical theory, Ecology, 76, 493–509, https://doi.org/10.2307/1941208, 1995.
Hedin, L. O., Vitousek, P. M., and Matson, P. A.: Nutrient losses over four million years of tropical forest development, Ecol. Res., 84, 2231–2255, https://doi.org/10.1890/02-4066, 2003.
Hedley, M. J., Stewart, J. W. B., and Chauhan, B. S.: Changes in Inorganic and Organic Soil Phosphorus Fractions Induced by Cultivation Practices and by Laboratory Incubations1, Soil Sci. Soc. Am. J., 46, 970, https://doi.org/10.2136/sssaj1982.03615995004600050017x, 1982.
Holzmann, S., Missong, A., Puhlmann, H., Siemens, J., Bol, R., Klumpp, E., and von Wilpert, K.: Impact of anthropogenic induced nitrogen input and liming on phosphorus leaching in forest soils, J. Plant Nutr. Soil Sc., 179, 443–453, https://doi.org/10.1002/jpln.201500552, 2016.
Julich, S., Benning, R., Julich, D., and Feger, K. H.: Quantification of phosphorus exports from a small forested headwater-catchment in the Eastern Ore Mountains, Germany, Forests, 8, 206, https://doi.org/10.3390/f8060206, 2017.
Kaiser, K., Guggenberger, G., and Zech, W.: Organically bound nutrients in dissolved organic matter fractions in seepage and pore water of weakly developed forest soils, Acta Hydroch. Hydrob., 28, 411–419, 2000.
Kaiser, K., Guggenberger, G., Haumaier, L., and Zech, W.: Seasonal variations in the chemical compostion of dissolved organic matter in organic forest floor layer leachates of old-growth Scots pine and Europena beech stans in northeastern Bavaria, Germany, Biogeochemistry, 55, 103–143, https://doi.org/10.1023/A:1010694032121, 2001.
Kaiser, K., Guggenberger, G., and Haumaier, L.: Organic phosphorus in soil water under a European beech (Fagus sylvatica L.) stand in northeastern Bavaria, Germany: Seasonal variability and changes with soil depth, Biogeochemistry, 66, 287–310, https://doi.org/10.1023/B:BIOG.0000005325.86131.5f, 2003.
Kalbitz, K., Solinger, S., Park, J. H., Michalzik, B., and Matzner, E.: Controls on the dynamics dissolved organic matter in soils: A review, Soil Sci., 165, 277–304, https://doi.org/10.1097/00010694-200004000-00001, 2000.
Kuznetsova, A., Brockhoff, P. B., and Christensen, R. H. B.: lmerTest Package: Tests in Linear Mixed Effects Models, J. Stat. Softw., 82, 1–26, https://doi.org/10.18637/jss.v082.i13, 2017.
Lang, F., Krüger, J., Amelung, W., Willbold, S., Frossard, E., Bünemann, E. K., Bauhus, J., Nitschke, R., Kandeler, E., Marhan, S., Schulz, S., Bergkemper, F., Schloter, M., Luster, J., Guggisberg, F., Kaiser, K., Mikutta, R., Guggenberger, G., Polle, A., Pena, R., Prietzel, J., Rodionov, A., Talkner, U., Meesenburg, H., von Wilpert, K., Hölscher, A., Dietrich, H. P., and Chmara, I.: Soil phosphorus supply controls P nutrition strategies of beech forest ecosystems in Central Europe, Biogeochemistry, 136, 5–29, https://doi.org/10.1007/s10533-017-0375-0, 2017.
Lilienfein, J., Qualls, R. G., Uselman, S. M., and Bridgham, S. D.: Adsorption of dissolved organic and inorganic phosphorus in soils of a weathering chronosequence, Soil Sci. Soc. Am. J., 68, 620–628, https://doi.org/10.2136/sssaj2004.6200, 2004.
Litaor, M. I.: Review of soil solution samplers, Water Resour. Res., 24, 727–733, https://doi.org/10.1029/WR024i005p00727, 1988.
Makowski, V., Julich, S., Feger, K. H., Breuer, L., and Julich, D.: Leaching of dissolved and particulate phosphorus via preferential flow pathways in a forest soil: An approach using zero-tension lysimeters, J. Plant Nutr. Soil Sc., 183, 238–247, https://doi.org/10.1002/jpln.201900216, 2020a.
Makowski, V., Julich, S., Feger, K.-H., and Julich, D.: Soil Phosphorus Translocation via Preferential Flow Pathways: A Comparison of Two Sites With Different Phosphorus Stocks, Front. For. Glob. Chang., 3, 48, https://doi.org/10.3389/ffgc.2020.00048, 2020b.
Michalzik, B., Kalbitz, K., Park, J. H., Solinger, S., and Matzner, E.: Fluxes and concentrations of dissolved organic carbon and nitrogen – A synthesis for temperate forests, Biogeochemistry, 52, 173–205, https://doi.org/10.1023/A:1006441620810, 2001.
Moir, J. and Tiessen, H.: Characterization of Available P by Sequential Extraction, in: Soil Sampling and Methods of Analysis, edited by: Carter, M. R. and Gregorich, E. G., second edn., CRC Press, Canadian Soil Science Society – Taylor & Francis, Boca Raton, FL, https://doi.org/10.1201/9781420005271-33, 2007.
Mooshammer, M., Wanek, W., Zechmeister-Boltenstern, S., and Richter, A.: Stoichiometric imbalances between terrestrial decomposer communities and their resources: Mechanisms and implications of microbial adaptations to their resources, Front. Microbiol., 5, 1–10, https://doi.org/10.3389/fmicb.2014.00022, 2014.
Mortensen, J., Hauge Nielsen, K., and JØrgensen, U.: Nitrate leaching during establishment of willow (Salix viminalis) on two soil types and at two fertilization levels, Biomass Bioenerg., 15, 457–466, https://doi.org/10.1016/S0961-9534(98)00056-7, 1998.
Müller, M., Alewell, C., and Hagedorn, F.: Effective retention of litter-derived dissolved organic carbon in organic layers, Soil Biol. Biochem., 41, 1066–1074, https://doi.org/10.1016/j.soilbio.2009.02.007, 2009.
Murphy, J. and Riley, J. P.: A modified single solution method for the determination of phosphate in natural waters, Anal. Chim. Acta, 27, 31–36, https://doi.org/10.1016/S0003-2670(00)88444-5, 1962.
Neff, J. C., Hobbie, S. E., and Vitousek, P. M.: Nutrient and mineralogical control on dissolved organic C, N and P fluxes and stoichiometry in Hawaiian soils, Biogeochemistry, 51, 283–302, https://doi.org/10.1023/A:1006414517212, 2000.
NW-FVA: Waldzustands-Bericht 2020 (Hessen), Göttingen, https://fawf.wald-rlp.de/de/veroeffentlichungen/waldzustandsbericht/ (last access: 25 May 2021), 2020.
Ohno, T. and Zibilske, L. M.: Determination of low concentrations of phosphorus in soil extracts using malachite green, Soil Sci. Soc. Am. J., 55, 892–895, 1991.
Peñuelas, J., Poulter, B., Sardans, J., Ciais, P., van der Velde, M., Bopp, L., Boucher, O., Godderis, Y., Hinsinger, P., Llusia, J., Nardin, E., Vicca, S., Obersteiner, M., and Janssens, I. A.: Human-induced nitrogen–phosphorus imbalances alter natural and managed ecosystems across the globe, Nat. Commun., 4, 1–10, https://doi.org/10.1038/ncomms3934, 2013.
Perakis, S. S. and Hedin, L. O.: Fluxes and fates of nitrogen in soil of an unpolluted old-growth temperate forest southern Chile, Ecology, 82, 2245–2260, https://doi.org/10.1890/0012-9658(2001)082[2245:FAFONI]2.0.CO;2, 2001.
Perakis, S. S. and Hedin, L. O.: Nitrogen loss from unpolluted South American forests mainly via dissolved organic compounds, Nature, 415, 416–419, https://doi.org/10.1038/415416a, 2002.
Piirainen, S., Finér, L., Mannerkoski, H., and Starr, M.: Carbon, nitrogen and phosphorus leaching after site preparation at a boreal forest clear-cut area, Forest Ecol. Manag., 243, 10–18, https://doi.org/10.1016/j.foreco.2007.01.053, 2007.
Qualls, R. G.: Comparison of the behavior of soluble organic and inorganic nutrients in forest soils, Forest Ecol. Manag., 138, 29–50, 2000.
Qualls, R. G. and Haines, B. L.: Fluxes of dissolved organic nutrients and humic substances in a deciduous forest, Ecology, 72, 254–266, 1991a.
Qualls, R. G. and Haines, B. L.: Geochemistry of dissolved organic nutrients in water percolating through a forest ecosystem, Soil Sci. Soc. Am. J., 55, 1112–1123, https://doi.org/10.2136/sssaj1991.03615995005500040036x, 1991b.
R Core Team: R: A language and environment for statistical computing, https://www.r-project.org/ (last access: 12 July 2020), 2020.
Rechberger, M. V., Zehetner, F., and Gerzabek, M. H.: Phosphate sorption-desorption properties in volcanic topsoils along a chronosequence and a climatic gradient on the Galápagos Islands, J. Plant Nutr. Soil Sc., 184, 479–491, https://doi.org/10.1002/jpln.202000488, 2021.
Richardson, S. J., Peltzer, D. A., Allen, R. B., McGlone, M. S., and Parfitt, R. L.: Rapid development of phosphorus limitation in temperate rainforest along the Franz Josef soil chronosequence, Oecologia, 139, 267–276, https://doi.org/10.1007/s00442-004-1501-y, 2004.
Rinderer, M., Krüger, J., Lang, F., Puhlmann, H., and Weiler, M.: Subsurface flow and phosphorus dynamics in beech forest hillslopes during sprinkling experiments: how fast is phosphorus replenished?, Biogeosciences, 18, 1009–1027, https://doi.org/10.5194/bg-18-1009-2021, 2021.
Rodionov, A., Bauke, S. L., von Sperber, C., Hoeschen, C., Kandeler, E., Kruse, J., Lewandowski, H., Marhan, S., Mueller, C. W., Simon, M., Tamburini, F., Uhlig, D., von Blanckenburg, F., Lang, F., and Amelung, W.: Biogeochemical cycling of phosphorus in subsoils of temperate forest ecosystems, Biogeochemistry, 150, 313–328, https://doi.org/10.1007/s10533-020-00700-8, 2020.
Saunders, W. M. H. and Williams, E. G.: Observations on the determination of total organic phosphorus in soils, Soil Sci., 6, 254–267, https://doi.org/10.1111/j.1365-2389.1955.tb00849.x, 1955.
Schimel, J. P.: Life in dry soils: Effects of drought on soil microbial communities and processes, Annu. Rev. Ecol. Evol. S., 49, 409–432, https://doi.org/10.1146/annurev-ecolsys-110617-062614, 2018.
Schleuss, P. M., Widdig, M., Biederman, L. A., Borer, E. T., Crawley, M. J., Kirkman, K. P., Seabloom, E. W., Wragg, P. D., and Spohn, M.: Microbial substrate stoichiometry governs nutrient effects on nitrogen cycling in grassland soils, Soil Biol. Biochem., 155, 108–168, https://doi.org/10.1016/j.soilbio.2021.108168, 2021.
Siegenthaler, M. B., Ramoneda, J., Frossard, E., and Mészáros, É.: Microbial community responses to phosphorus and nitrogen inputs in the organic soil horizons of two contrasting temperature beech forests, Appl. Soil Ecol., 172, 104357, https://doi.org/10.1016/j.apsoil.2021.104357, 2021.
Sohrt, J., Uhlig, D., Kaiser, K., von Blanckenburg, F., Siemens, J., Seeger, S., Frick, D. A., Krüger, J., Lang, F., and Weiler, M.: Phosphorus Fluxes in a Temperate Forested Watershed: Canopy Leaching, Runoff Sources, and In-Stream Transformation, Front. For. Glob. Chang., 2, 1–14, https://doi.org/10.3389/ffgc.2019.00085, 2019.
Talkner, U., Krämer, I., Hölscher, D., and Beese, F. O.: Deposition and canopy exchange processes in central-German beech forests differing in tree species diversity, Plant Soil, 336, 405–420, https://doi.org/10.1007/s11104-010-0491-2, 2010.
Talkner, U., Meiwes, K. J., Potočić, N., Seletković, I., Cools, N., De Vos, B., and Rautio, P.: Phosphorus nutrition of beech (Fagus sylvatica L.) is decreasing in Europe, Ann. For. Sci., 72, 919–928, https://doi.org/10.1007/s13595-015-0459-8, 2015.
Tipping, E., Benham, S., Boyle, J. F., Crow, P., Davies, J., Fischer, U., Guyatt, H., Helliwell, R., Lawlor, A. J., Monteith, D. T., Rowe, E. C., and Toberman, H.: Atmospheric deposition of phosphorus to land and freshwater, Environ. Sci.-Proc. Imp., 16, 1608–1617, https://doi.org/10.1039/c3em00641g, 2014.
Van der Ploeg, R. R., Böhm, W., and Kirkham, M. B.: On the origin of the theory of mineral nutrition of plants and the law of the minimum, Soil Sci. Soc. Am. J., 63, 1055–1062, https://doi.org/10.2136/sssaj1999.6351055x, 1999.
Vogel, C., Helfenstein, J., Massey, M. S., Sekine, R., Kretzschmar, R., Beiping, L., Peter, T., Chadwick, O. A., Tamburini, F., Rivard, C., Herzel, H., Adam, C., Pradas del Real, A. E., Castillo-Michel, H., Zuin, L., Wang, D., Félix, R., Lassalle-Kaiser, B., and Frossard, E.: Microspectroscopy reveals dust-derived apatite grains in acidic, highly-weathered Hawaiian soils, Geoderma, 381, 114681, https://doi.org/10.1016/j.geoderma.2020.114681, 2021.
Walker, T. W. and Syers, J. K.: The fate of phosphorous during pedogenesis, Geoderma, 15, 1–19, https://doi.org/10.1016/0016-7061(76)90066-5, 1976.
Wang, R., Balkanski, Y., Boucher, O., Ciais, P., Peñuelas, J., and Tao, S.: Significant contribution of combustion-related emissions to the atmospheric phosphorus budget, Nat. Geosci., 8, 48–54, https://doi.org/10.1038/ngeo2324, 2014.
Widdig, M., Schleuss, P. M., Weig, A. R., Guhr, A., Biederman, L. A., Borer, E. T., Crawley, M. J., Kirkman, K. P., Seabloom, E. W., Wragg, P. D., and Spohn, M.: Nitrogen and Phosphorus Additions Alter the Abundance of Phosphorus-Solubilizing Bacteria and Phosphatase Activity in Grassland Soils, Front. Environ. Sci., 7, 1–15, https://doi.org/10.3389/fenvs.2019.00185, 2019.
Yu, L., Ahrens, B., Wutzler, T., Schrumpf, M., and Zaehle, S.: Jena Soil Model (JSM v1.0; revision 1934): a microbial soil organic carbon model integrated with nitrogen and phosphorus processes, Geosci. Model Dev., 13, 783–803, https://doi.org/10.5194/gmd-13-783-2020, 2020.
Zederer, D. P., Talkner, U., Spohn, M., and Joergensen, R. G.: Microbial biomass phosphorus and C/N/P stoichiometry in forest floor and A horizons as affected by tree species, Soil Biol. Biochem., 111, 166–175, https://doi.org/10.1016/j.soilbio.2017.04.009, 2017.