the Creative Commons Attribution 4.0 License.
the Creative Commons Attribution 4.0 License.
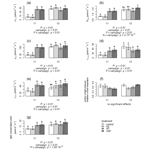
Phosphorus stress strongly reduced plant physiological activity, but only temporarily, in a mesocosm experiment with Zea mays colonized by arbuscular mycorrhizal fungi
Melanie S. Verlinden
Hamada AbdElgawad
Arne Ven
Lore T. Verryckt
Sebastian Wieneke
Ivan A. Janssens
Phosphorus (P) is an essential macronutrient for plant growth and one of the least available nutrients in soil. P limitation is often a major constraint for plant growth globally. Although P addition experiments have been carried out to study the long-term effects on yield, data on P addition effects on seasonal variation in leaf-level photosynthesis are scarce. Arbuscular mycorrhizal fungi (AMF) can be of major importance for plant nutrient uptake, and AMF growth may be important for explaining temporal patterns in leaf physiology. In a nitrogen (N) and P fertilization experiment with Zea mays, we investigated the effect of P limitation on leaf pigments and leaf enzymes, how these relate to leaf-level photosynthesis, and how these relationships change during the growing season. A previous study on this experiment indicated that N availability was generally high, and as a consequence, N addition did not affect plant growth, and also the leaf measurements in the current study were unaffected by N addition. Contrary to N addition, P addition strongly influenced plant growth and leaf-level measurements. At low soil P availability, leaf-level photosynthetic and respiratory activity strongly decreased, and this was associated with reduced chlorophyll and photosynthetic enzymes. Contrary to the expected increase in P stress over time following gradual soil P depletion, plant P limitation decreased over time. For most leaf-level processes, pigments and enzymes under study, the fertilization effect had even disappeared 2 months after planting. Our results point towards a key role for the AMF symbiosis and consequent increase in P uptake in explaining the vanishing P stress.
- Article
(359 KB) - Full-text XML
- BibTeX
- EndNote
Phosphorus (P) is a crucial element in natural ecosystems. It is present in the structure of DNA, in cell membranes, in molecules storing and supplying energy, and in several enzymes. As a consequence, P plays a crucial role in plant and soil processes; it regulates productivity and ecosystem functions and influences organisms from the individual to the community level (Elser et al., 2000; Vitousek et al., 2010; Peñuelas et al., 2013). The importance of P for the functioning of the Earth's biogeochemical cycles, especially the carbon cycle, is therefore being increasingly recognized (Vitousek et al., 2010; Wieder et al., 2015; Vicca et al., 2018), and this is reflected in the recent efforts to include P in terrestrial biosphere models (Wang et al., 2010; Thum et al., 2019).
In plants, P plays a role in most developmental and biochemical processes. Structurally, P is a component of RNA and membrane phospholipids, while metabolically, P functions in the storage and transfer of energy and in energizing of binding sites for metabolic turnover (Schulze et al., 2005; Veneklaas et al., 2012). However, P is one of the least available macronutrients in soils, and P limitation is often a major constraint for plant growth (Augusto et al., 2017). On more than one-third of the arable land worldwide, plant productivity is considered to be limited by P (Calderón-Vázquez et al., 2009).
Various experiments have been conducted to study the effect of P addition to crops, thereby mainly focusing on the long-term effect on yield (Khan et al., 2018; Johnston and Poulton, 2019). However, data on seasonal variation in leaf-level photosynthesis, especially in crops, are scarce (Rodríguez et al., 2000; Rogers, 2014), although accurate seasonal estimates of photosynthetic capacity are critical for modeling the time course of carbon fluxes (Miner and Bauerle, 2019). The majority of studies investigating effects of nutrients on photosynthesis focus on nitrogen (N) and much less on P and other nutrients (e.g., Brooks, 1986; Brooks et al., 1988; Rodríguez and Goudriaan, 1995; Rodríguez et al., 1998). In addition, it is unclear whether leaf traits, such as leaf nutrients, pigments and enzymes, change seasonally in relation to leaf-level photosynthesis.
Among others, plant P limitation typically results in reduced photosynthesis and plant growth, especially above ground. P is required for adenosine triphosphate (ATP) synthesis (Veneklaas et al., 2012), which is needed to regenerate ribulose 1,5-bisphosphate (RuBP) in the Calvin cycle of photosynthesis. Inorganic phosphate (Pi) directly affects the activity of Calvin cycle enzymes through the level of activation. For instance, Pi is required for light activation of rubisco (Parry et al., 2008). It also directly affects the maximum rate of CO2-limited carboxylation (vcmax) and triose phosphate utilization (Lewis et al., 1994) and RuBP-regeneration-limited rates of electron transport (Loustau et al., 1999). P deficiency therefore leads to a decrease in RuBP pool size and insufficient ATP and consequently to a decrease in photosynthetic C assimilation. The concentration and specific activity of rubisco, the primary CO2 fixing enzyme in photosynthesis, are generally little affected by P stress (Brooks, 1986; Paul and Stitt, 1993; Pieters et al., 2001; but see Jacob and Lawlor, 1991; Pieters et al., 2001).
Pi can also indirectly affect photosynthesis through the changes in stromal pH (Bhagwat, 1981), where the consumption of Pi as a substrate of photosynthesis could decrease photosynthesis by a direct effect of low stromal Pi concentration on rubisco. Moreover, the effect of P on photosynthesis depends on the dynamic interactions between sink and source tissues. Low P can reduce carbon export to sinks and thus decrease sink strength, thereby limiting photosynthesis (Pieters et al., 2001). Concomitantly, leaf starch can increase with P stress (Zhang et al., 2014) due to low availability of P for triphosphate translocation, although decreases in leaf starch have also been observed (Halsted and Lynch, 1996). Moreover, low sink strength restricts the recycling of Pi back to the chloroplast, further reducing photosynthesis (Paul and Foyer, 2001).
In a mesocosm nutrient manipulation experiment setup (previously described in Verlinden et al., 2018), maize (Zea mays L.) was planted at different soil N and P availabilities. As demonstrated in Verlinden et al. (2018), this resulted in a strong P, but no N effect on plant growth or photosynthesis at the mesocosm scale. In that study, also arbuscular mycorrhizal fungi (AMF) played an important role in explaining plant carbon uptake and allocation. AMF are important for nutrient uptake in maize (Hartnett and Wilson, 1999; Hoeksema et al., 2010), especially for P, and hence AMF growth may also be important for explaining variation in leaf physiology. The objective of the current study is to test the effect of P limitation on leaf pigments, sugars and photosynthetic enzymes; how they relate to leaf-level photosynthesis; and how these relationships change during the growing season. At low soil P availability, we expected low leaf-level photosynthetic and respiratory activity, associated with reduced chlorophyll and photosynthetic enzymes. Furthermore, P stress was expected to increase over time as plants were expected to gradually deplete the soil P.
2.1 Experimental design
A mesocosm experiment consisting of 20 (1 m × 1.2 m, 0.6 m high) insulated boxes was set up in a greenhouse in Sint-Katelijne-Waver, Belgium (51∘04′38′′ N, 4∘32′05′′ E). To each mesocosm we added soil, which was a homogenized mixture of sand originating from a pine forest in a nature reserve in Flanders, white river sand and a minority of compost (details of the experimental setup are described in Verlinden et al., 2018). On 20 May 2016, 12 seedlings of maize (Zea mays L., variety “Tom Thumb”) were planted per mesocosm. Different treatments (set up in five replicates) were distinguished in the level of nutrients added: the +N treatment was fertilized with calcium nitrate at a rate of 95.5 kg N ha−1 (YaraLiva® Calcinit®), the +P treatment received 20 kg P ha−1 as triple superphosphate (Janssens-Smeets®), and the combined +N and +P treatment (+NP) received both amounts together. The control treatment received, as all other treatments, only a basic level of micronutrients (Fertigreen®, Patentkali® and GroGreen® containing 79 kg K ha−1, 19 kg Mg ha−1, 53 kg S ha−1, 0.4 kg B ha−1, 0.1 kg Cu ha−1, 2.4 kg Fe ha−1, 1.1 kg Mn ha−1, 0.1 kg Mo ha−1 and 0.4 kg Zn ha−1). Spore-based inoculum of AMF (species Rhizophagus irregularis, Symplanta®) was added to all 20 (4 treatments × 5 replicates) mesocosms. Soil moisture was monitored and kept at a non-limiting (field capacity) level, similar in all plots.
2.2 Measurements and analyses
2.2.1 Leaf C, N and P concentration and specific leaf area
Carbon (C) and N concentrations were determined using an elemental analyzer (FLASH 2000 model, Thermo Fisher Scientific, Waltham, USA). Total leaf P concentration was determined by digestion in tubes with H2SO4–salicylic acid–H2O2 and selenium (Temminghoff and Houba, 2004). Specific leaf area (SLA; m2 kg−1) was determined as the ratio of the fresh leaf area to dry leaf mass.
2.2.2 Leaf photosynthesis
A portable gas exchange system, LI-6400 (LI-COR, Lincoln, NE, USA), was used for leaf-scale CO2 gas exchange measurements, operating as an open system (e.g., Verlinden et al., 2013). Leaf-scale measurements were performed during 2 weeks in late June (campaign 1, C1) and repeated at the end of July (campaign 2, C2), allowing the seasonal development to be studied. Mean daily photosynthetically active radiation (PAR) during C1 and C2 were respectively 17.1 and 17.7 mol m−2, and average temperature was respectively 21.7 and 23.3 ∘C.
In each plot photosynthetic CO2 response curves (i.e., photosynthesis (A, assimilation) responses to the CO2 concentration inside leaf air spaces (ci)) were measured on a recently matured leaf. Leaves were allowed to equilibrate at a CO2 concentration of 400 µmol mol−1 in the leaf cuvette, after which the net CO2 assimilation rate at a sequence of different CO2 concentrations (i.e., 400, 30, 50, 80, 110, 150, 250, 350, 500 and 1000 µmol mol−1) was measured. Photosynthetic photon flux density (PPFD) was fixed at a saturating value of 1200 µmol s−1 m−2. The resulting A–ci curves were fitted to the biochemical model of C4 photosynthesis as presented by von Caemmerer (2000) using the package “Plantecophys” (Duursma, 2015) in R version 3.3.1 (R Foundation for Statistical Computing, Vienna, Austria). The CO2 assimilation rate is approximated by the minimum of the expressions of an enzyme-limited and an electron-transport-limited CO2 assimilation rate. The parameters Jmax (maximum electron transport rate), vcmax (maximal rubisco carboxylation rate) and vpmax (maximum PEP carboxylation rate) were calculated through curve fitting based on minimum least squares.
Photosynthetic light response curves were obtained by measurements of the net CO2 assimilation rate at PPFDs of 1200, 500, 250, 100, 80, 60, 40, 30, 25, 20, 15, 10, 5 and 0 µmol m−2 s−1 (blue–red LED source type 6400-02B, 13 % blue light). Leaves were allowed to equilibrate at each step before logging the data. The CO2 concentration in the cuvette was maintained at 400 µmol mol−1 and the block temperature at 25 ∘C. From the light response curves, the net CO2 assimilation rate at light saturation (Amax) and leaf dark respiration (Rdark; net CO2 exchange at zero light) were derived. In addition, light-induced inhibition of leaf respiration was estimated from the light response curves (for PPFDs of 0 to 80 µmol m−2 s−1) from the intersections of the fitted lines above and below the light compensation point on the y axis, giving respectively Rlight and Rdark (Kok, 1948). All selected leaves were harvested and stored at −80 ∘C for later analyses.
2.2.3 Chemical analyses of leaf material
Rubisco activity was analyzed according to Sulpice et al. (2007). It was expressed as the conversion rate of glycerate kinase (3-PGA) of extracted leaf samples (µmol 3-PGA m−2 min−1). The activity of rubisco was determined directly (“direct rubisco”), without incubation of the extract in the presence of 10 mM HCO3− and 20 mM Mg2+ to convert the non-carbamylated rubisco into the carbamylated form. The assay of phosphoenolpyruvate carboxylase (PEPC) was coupled with the malate dehydrogenase reaction, and the resulting rate of PEPC activity was expressed in µmol HCO3 m−2 min−1.
Mono- and oligosaccharides in leaves were analyzed chromatographically according to AbdElgawad et al. (2014). Soluble sugar concentrations were measured by high-performance anion exchange chromatography of extracted leaf samples with pulsed amperometric detection (HPAEC-PAD), and the total soluble sugar concentration was calculated as their sum. The remaining pellet of soluble sugar extract was treated with a mixture of α-amylase and amyloglucosidase to extract starch.
High-performance liquid chromatography (HPLC) was used to analyze leaf pigments. The detection of the carotenoids and xanthophylls was done by a diode array detector (Shimadzu SPD-M10Avp, Kyoto, Japan) at four wavelengths (420, 440, 462, 660 nm) and integrated via the software Shimadzu LabSolutions Lite (Kyoto, Japan), in which the concentration was determined using a calibration curve.
2.2.4 Mycorrhizal fungi
Because AMF growth is potentially crucial for explaining patterns in the leaf response to P limitation, we determined the time course of AMF abundance in each of the mesocosms. To this end, five mesh bags filled with white river sand – and permeable for fungi but not for roots (30 µm mesh size) – were buried vertically into the topsoil of each mesocosm 1 week before planting. They were harvested consecutively 31 (corresponding to C1) and 61 d (right before C2) after planting. Hyphae were extracted from 4 g of mesh bag sand using the method of Rillig et al. (1999). After suspending, processing and staining the sample, hyphal intersects were counted at a magnification of 40 × 10 using a grid in the microscope ocular. Hyphal length density was calculated following Eq. (1) (Tennant, 1975; Rillig et al., 1999):
where HLD is hyphal length density (mm hyphae per g soil), n is number of intersects containing AMF hyphae, a is filter area (mm2) examined, d is the dilution factor, h is the total length of raster lines projected on filter (mm), and w is soil weight (g).
Mycorrhizal colonization was examined in C1 and C2 by sampling roots from two plants per mesocosm. Per plant, 20 cm of one lateral root containing root hair was excavated, cut and stored. Mycorrhizal colonization was quantified by counting arbuscules, vesicles and hyphae, applying the grid line intersection method (Vierheilig et al., 2005). The methodology for determination of root colonization is described more elaborately in Verlinden et al. (2018).
2.2.5 Statistical analyses
Data normality and homoscedasticity were checked using the Shapiro–Wilk and Levene test, respectively. A three-way mixed analyses of variance (ANOVA) was applied to test if the quantified variables differed between the treatments and between C1 and C2. N addition and P addition were both considered to be between-subject variables and time (campaign) to be within-subject variable. Non-significant interactions terms and, further, non-significant factors were removed from the model. In case of significant interaction between factors, the analysis included their multiplied factor levels. A Tukey post hoc test was applied for pairwise comparison in case of significant factor effects.
The addition of P fertilizer increased soil P availability (Verlinden et al., 2018) as well as leaf P concentration (Table 1). At the time of C1, leaf P concentration was 3 to 4 times higher in the +P and +NP treatments than in the non-P-fertilized control and +N treatments. Leaf N : P ratio was higher in the non-P-fertilized treatments than in the P-fertilized treatments (an average N : P ratio of 19.8 versus 37.2 for the non-P-fertilized treatments). However, in C2, the leaf P concentration had increased in all treatments to a similar level (Table 1), as well as the N : P ratio, which decreased for all treatments to a similar level, with a mean of 13.8. Leaves in the non-P-fertilized mesocosms were thinner and/or had a lower density than in the P-fertilized mesocosms (Table 1) during C1, with mean SLA values of respectively 52.9 ± 0.9 and 33.9 ± 1.9 m2 kg−1. Later in the season, SLA decreased in all mesocosms, and the difference between non-P-fertilized and P-fertilized mesocosms had disappeared at the time of C2.
Table 1Means (and standard errors) of chemically analyzed leaf traits, per campaign and per treatment.
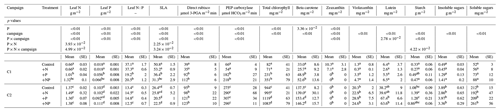
C1: campaign 1, end of June; C2: campaign 2, end of July. SE: standard error. Superscript letters indicate homogeneous groups as results from post hoc analysis of statistical analyses of variance.
The majority of leaf physiology parameters differed considerably between C1 versus C2 for the non-P-fertilized treatments, while for the P-fertilized treatments differences between C1 and C2 were much less pronounced. During C1, photosynthetic activity was very low in the non-P-fertilized treatments, with a mean Amax of 6.2 (± 4.1) µmol m−2 s−1 for the control and +N treatments. In contrast, the +P and +NP treatments showed a mean Amax more than 4 times higher than in the non-P-fertilized mesocosms (Fig. 1a). A similarly high Amax level was reached for all treatments in C2 (Fig. 1a). Also Rdark was smaller in the non-P-fertilized treatments in C1 (Fig. 1b) and reached a similar level as the +P and +NP treatments in C2. Photosynthetic parameters Jmax, vcmax and vpmax were all lower in the non-P-fertilized treatments than in the P-fertilized treatments during C1 (Fig. 1c–e), but by the time of C2, Jmax had increased in the non-P-fertilized mesocosms to the level of the P-fertilized mesocosms. Vcmax in the non-P-fertilized mesocosms had even increased to a level of about 45 % higher than the P-fertilized mesocosms, while the P-fertilized mesocosms showed very similar Jmax, vcmax and vpmax for C1 and C2. Light-induced inhibition of respiration (Fig. 1f) was variable amongst the mesocosms, though on average it tended to be higher in the non-P-fertilized mesocosms during C1, whereas no trend was observed during C2. The light compensation point was initially lower in the non-P-fertilized plants (i.e., in the stressed plants photosynthetic activity occurred at a lower light availability than in the P-fertilized treatments), whereas during C2 no differences were observed between the mesocosms (Fig. 1g).
Similar to the gas exchange measurements, the leaf chemistry showed a strong difference between non-P-fertilized and P-fertilized plots during C1, but not during C2. Direct rubisco concentration was initially lower in the non-P-fertilized mesocosms (Table 1), which was also true for the enzyme PEP carboxylase (Table 1). A P and campaign effect was observed for total chlorophyll (Table 1; similarly for chlorophyll a and chlorophyll b; data not shown); its concentration was 4 times higher in the P-fertilized mesocosms during C1. Also beta-carotene concentration was initially higher in the P-fertilized mesocosms (Table 1). Zeaxanthin was only detected in the non-P-fertilized leaves during C1 (Table 1). For both lutein and violaxanthin no differences among the treatments were observed during C1. There was a tendency of lower starch in the P-stressed mesocosms as compared to the P-fertilized mesocosms during C1, although there was no P effect, whereas the campaign effect and interactions P × campaign and N × P × campaign were significant.
During C2, direct rubisco concentration increased in the non-P-fertilized mesocosms to the same level as in P-fertilized mesocosms, while PEP carboxylase concentration increased in all mesocosms to reach a similar level in C2. Chlorophyll concentration increased more than 12-fold for the non-P-fertilized mesocosms from C1 to C2; for the P-fertilized mesocosms almost 4-fold. A similar trend was observed for beta-carotene (Table 1), of which concentrations increased 5- and 3-fold, respectively. Also lutein and violaxanthin were present in higher concentrations during C2 (Table 1). Zeaxanthin was not detected during C2. The leaf starch concentration differed over time; leaves contained much less starch during C1 than during C2 (Table 1).
One month after establishing the experimental setup (during C1), no AMF were detected in plant roots or in the mesh bags (Fig. 2). One month later, i.e., during C2, however, AMF had clearly established, with a mean hyphal length density of 760 mm per g soil in all treatments. The percentage of roots colonized was higher in the non-P-fertilized treatments than in the P-fertilized plots (67 % vs. 40 % on average) (Fig. 2; Ven et al., 2020a).
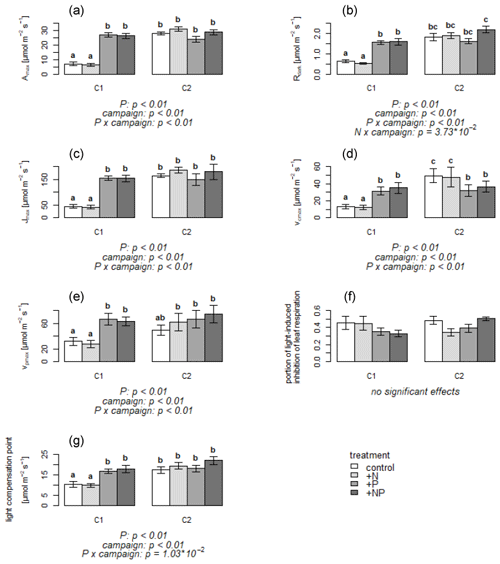
Figure 1(a–g) Means of parameters deduced from leaf CO2 exchange measurements per treatment and campaign. Error bars indicate standard error. C1: campaign 1, end of June; C2: campaign 2, end of July; control treatment: not fertilized; +N treatment: nitrogen-fertilized; +P treatment: phosphorus-fertilized; +NP treatment: both nitrogen- and phosphorus-fertilized. Letters above bars indicate significant differences. Significant effects are given with p value below the plots. Amax: maximal assimilation rate; Rdark: leaf dark respiration; : ratio of leaf dark respiration to maximal assimilation rate; Jmax: maximum electron transport rate; vcmax: maximal rubisco carboxylation rate; vpmax: maximum PEP carboxylation rate.
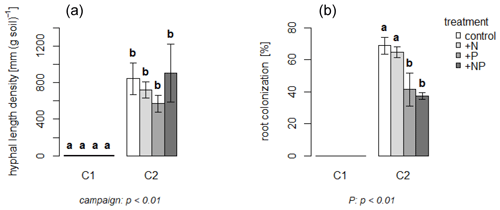
Figure 2Means of mycorrhizal hyphal length (a) and root colonization (b) by time of C1 and C2. Error bars indicate standard error. Letters above bars indicate statistical differences. Significant effects are given with p value below the plots. Note that the values for C1 were (close to) zero, indicating that root colonization with AMF was negligible.
The unfertilized soil in our experiment was clearly P-impoverished; addition of P increased plant productivity, whereas N addition did not. End-of-season dry biomass reached 81 (± 7) and 510 (± 24) g m−2 for the non-P-fertilized and P-fertilized treatments, respectively (as reported in an earlier publication of this experiment; Verlinden et al., 2018). N addition had no effect on the leaf-scale measurements; therefore we focus on effects of P.
Leaf photosynthetic parameters and most leaf chemistry parameters showed clear changes throughout the season, as verified by the significant P × campaign interaction effects (Fig. 1, Table 1). During C1, leaf P concentrations in the non-P-fertilized plants were 3 times lower than in the P-fertilized plants, whereas leaf P concentrations were similar for non-P-fertilized and P-fertilized treatments during C2. Since growth of plants with leaf N : P ratios higher than 16 (Koerselman and Meuleman, 1996) or 20 (Güsewell, 2004) is considered to be P-limited, the high leaf N : P ratios of about 37 illustrate a clear P limitation of plant growth for the non-P-fertilized treatments in C1, while P-fertilized treatments were close to P limitation. In C2, plants seemed to have reached a favorable allocation of N and P, as indicated by the favorable N : P ratio (i.e., between 9 and 18; Beauchamp and Hamilton, 1970) in all treatments. Accordingly, the leaves of the non-P-fertilized plants were yellow in the first weeks of the experiment but greened up later.
The initial P limitation present during C1 strongly limited leaf-level photosynthesis as Amax, Jmax and vcmax were 3 to 4 times lower in non-P-fertilized than in P-fertilized plants. This inhibitory effect can be attributed to the decrease in the pool size of ribulose-1,5-bisphosphate (RuBP) and its regeneration (Jacob and Lawlor, 1992; Pieters et al., 2001; Calderón-Vázquez et al., 2009) or by feedback inhibition of photosynthesis, but the latter was not specifically tested. Feedback inhibition of photosynthesis can be induced by elevated soluble sugar levels decreasing the gene expression of photosynthetic enzymes (e.g., PEPC, malic enzyme and rubisco) (Jeannette et al., 2000; AbdElgawad et al., 2020). This was not likely the case here since during C1 sugar levels tended to be lower in the non-P-fertilized than in the P-fertilized treatments. Lower starch and soluble sugar synthesis, like in the non-P-fertilized treatments, can slow Pi regeneration and limit ATP production and eventually the functioning of the Calvin cycle, which is known as short-term feedback regulation of photosynthesis (Griffin and Seemann, 1996).
Also rubisco levels were about 3 times lower in the non-P-fertilized plants than in the P-fertilized plants (Table 1). Insufficient P restricts the conversion of adenosine diphosphate (ADP) to ATP, limiting the RuBP regeneration (Rao and Terry, 1989; Calderón-Vázquez et al., 2009). C4 plants can maintain adequate levels of P in the bundle cells, and their growth is therefore generally less constrained by P limitation as compared to C3 plants (Calderón-Vázquez et al., 2009). This indicates that in our experiment, plants that did not receive P fertilizer must have experienced extreme P limitation early in the season in our experiment. Nonetheless, during C2, photosynthetic parameters reached similar values for all treatments.
Total chlorophyll can drop drastically in case of P deprivation (Jacob and Lawlor, 1991; Usuda and Shimogawara, 1991). In our experiment, chlorophyll concentration was initially lower in the non-P-fertilized mesocosms as compared to the P-fertilized mesocosms. During C2, however, chlorophyll concentration strongly increased in all treatments, in both the initially non-P-fertilized plants, where the chlorophyll increase was accompanied by increased photosynthesis, and in the P-fertilized plants. In the latter ones Amax did not differ between C1 and C2, indicating that photosynthesis did not increase despite the increase in chlorophyll concentration. Zeaxanthin was only detected in the non-P-fertilized plants during C1. Schlüter et al. (2013) showed the enhancement of protective pigments, such as zeaxanthin, in maize leaves when growing at low P availability. Zeaxanthin plays a key role in the protection of photosynthetic organisms against excess light, minimizing the over-excitation (Jahns and Holzwarth, 2012; Kuczyńska et al., 2012; Ashraf and Harris, 2013). The xanthophyll violaxanthin is reversibly de-epoxidized to zeaxanthin in the xanthophyll cycle when the light absorbed exceeds the capacity of photosynthesis. Zeaxanthin synthesis thus acts as a rescuing mechanism in strongly photo-oxidizing conditions (Dall'Osto et al., 2010), and increased zeaxanthin concentrations imply a decrease in light harvesting. In our experiment, no zeaxanthin was detected later in the season, suggesting that P stress was relieved and plant growth recovered, as also indicated by the increased net photosynthetic rate.
P deprivation has been found to increase the leaf starch concentration in maize (Zhang et al., 2014), although decreases in starch levels under low-P conditions have also been reported (Schlüter et al., 2013). In our experiment, reduced photosynthetic rates were unlikely due to reduced sink strength as P addition had no clear effect on the leaf starch concentration (Table 1). The starch concentration did show a significant campaign effect and more than doubled from C1 to C2. Both sucrose and starch synthesis play important roles in the cellular recycling of phosphate for photosynthesis (Schlüter et al., 2013). A decrease in sugars and starch might lead to lower vitality and productivity of plants, as was previously observed in stressed C4 leaves (da Silva and Arrabaça, 2004). In our experiment, while there was no effect of P for both sugars and starch, the campaign effect illustrated an increase in sugars and starch from C1 to C2, possibly suggesting that plants in all treatments experienced nutrient stress during C1. Moreover, the increasing sugar and starch levels between C1 and C2 confirm that the low photosynthetic rates for the low-P treatments were not due to reduced sink strength.
Foliar respiration rate is suppressed in the light. The abrupt decline in quantum yield of net CO2 assimilation that occurs at very low light, often near the photosynthetic light compensation point, is also known as the “Kok effect” (Kok, 1948). This light-induced inhibition of foliar respiration is reported to vary between 25 %–100 % (see references in Heskel et al., 2013) and is a source of uncertainty in current models of global terrestrial carbon cycling (Heskel and Tang, 2018). It can be impacted by environmental conditions such as temperature and soil nutrient availability (Heskel et al., 2012; Atkin et al., 2013). Here, the light-induced inhibition of respiration was highly variable among measured plants, largely ranging from 0.3 to 0.5, with high uncertainty levels. Several studies showed that increased soil nutrient availability can relax the degree of light-induced respiration, which was not confirmed in our experiment (Heskel et al., 2012; Atkin et al., 2013; Shapiro et al., 2004).
We applied 20 kg P ha−1 for the P treatment at which Amax reached its highest value of about 27 µmol m−2 s−1. Zhang et al. (2014) showed that the critical level of P application for the maximal net photosynthetic rate of maize (i.e., 30.3 µmol CO2 m−2 s−1) is between 15 and 28 kg P ha−1, which is in agreement with our study. Higher P application rates did not result in higher net photosynthetic rates in the study of Zhang et al. (2014). In our experiment the non-P-fertilized plants reached similar net photosynthetic rates, but only after colonization by AMF during C2. The campaign effect revealed in our experiment, i.e., the remarkable difference in P effect between C1 and C2, was associated with the (slow) establishment of AMF. This may suggest that increased plant P uptake following mycorrhization caused a recovery of the non-P-fertilized plants and was beneficial for productivity in the P-fertilized plants as well (Verlinden et al., 2018). In the same experiment, we found that the partitioning to roots and to AMF was larger in the non-P-fertilized mesocosms as compared to the P-fertilized mesocosms (Verlinden et al., 2018). Interestingly, in the absence of AMF, plants that did not receive extra P died prematurely in pasteurized mesocosms not included in this study (but reported in Verlinden et al., 2018).
The similar leaf P concentrations in all treatments during C2 further supports our assumption of a strong stimulation of P acquisition through mycorrhizae in the non-P-fertilized plants. The establishment of mycorrhizal symbioses is believed to be one of the most successful strategies to maximize the access of plant roots to available P and thus overcome P stress (Smith and Read, 2008; Sánchez-Calderón et al., 2010; Hu et al., 2022). The hyphal network of mycorrhizae extends over a very large surface area, increasing prominently the absorbing area of roots. Their extraradical hyphae extend beyond the P depletion zone, absorbing P that is otherwise not accessible for the plant (Plenchette et al., 2005; Roy-Bolduc and Hijri, 2011). Besides, mycorrhizal fungi improve phosphate solubility because they produce exudates that liberate P from the minerals (e.g., Smith et al., 2011; Burghelea et al., 2015; Kobae, 2019; Etesami et al., 2021; Jansa et al., 2021). For example glomalin, a glycoprotein secreted by AMF, aids the uptake of nutrients such as Fe and P that are difficult to dissolve (Miransari, 2010; Emran et al., 2017; Begum et al., 2019). Mycorrhizae thus significantly contribute to plant nutrition and to P uptake in particular (Wright et al., 2005), which in turn can positively affect leaf gas exchange rates (Smith and Read, 2008; Augé et al., 2016). Also other adaptations to P stress (e.g., changes in root exudation and root morphology) may have occurred (Lambers et al., 2008), but these were not investigated in this experiment. In any case, the fact that the increase in photosynthetic parameters in the non-P-fertilized plants was associated with increased mycorrhization, while in the absence of AMF the plants that did not receive extra P died prematurely, strongly indicates that the AMF strategy was critical for overcoming P stress in our experiment.
The leaf-scale responses reported here correspond well to the ecosystem-scale gross primary production (GPP) measurements reported for the same experiment in Verlinden et al. (2018). In the first weeks, both were (very) low in the absence of P addition but showed a sudden increase about 6 weeks after planting. Although ecosystem-level GPP remained lower for the non-P-fertilized treatments, the photosynthesis system seemed to have fully recovered, as indicated by similar levels of leaf photosynthesis among all treatments during C2. These results are in line with the study by Řezáčová et al. (2018), who reported photosynthetic upregulation following the establishment of mycorrhizal symbiosis. Also our follow-up experiment with a P gradient confirmed the important stimulating role of AMF for plant productivity and photosynthesis (see Ven et al., 2020b).
To conclude, low P availability significantly decreased photosynthetic capacity, associated with reduced concentrations of photosynthetic enzymes and pigments. In contrast to the expected increase in nutrient stress because of further depletion of the soil as the growing season progressed, nutrient stress decreased over time, and for most leaf processes, pigments and enzymes under study, the fertilization effect had disappeared 2 months after planting. Our results point towards a key role of the AMF symbiosis and consequent increase in P uptake in explaining the vanishing P stress. These results add to the mounting evidence of a key role of mycorrhizal fungi in mediating plant responses to environmental changes (e.g., Vicca et al., 2009; Terrer et al., 2016; Parihar et al., 2020). This emphasizes the need to take into account not only nutrient availability but also mycorrhizal symbionts when studying and modeling photosynthesis and carbon cycling in terrestrial ecosystems.
The used data are published on Zenodo (https://zenodo.org/record/6428027; Verlinden et al., 2022).
SV designed the experiment and research; MSV, HA and AV conducted the fieldwork and lab work; MSV made the first data analysis and draft of the manuscript; all authors provided expert advice and critically reviewed the manuscript.
At least one of the (co-)authors is a member of the editorial board of Biogeosciences. The peer-review process was guided by an independent editor, and the authors also have no other competing interests to declare.
Publisher’s note: Copernicus Publications remains neutral with regard to jurisdictional claims in published maps and institutional affiliations.
We gratefully acknowledge Fred Kockelbergh and Marc Wellens for technical support as well as Bart Hellemans, Lin Leemans and Eddy De Smet for logistic support at the field site.
This research has been supported by the Research Foundation – Flanders (FWO) (grant nos. G0D5415N, 12U8918N and 12K0316N), the European Research Council (IMBALANCE-P grant no. 610028), the European Cooperation in Science and Technology (grant no. ES1308), the H2020 Marie Skłodowska-Curie Actions (grant no. 795299), and the Universiteit Antwerpen (Methusalem grant).
This paper was edited by Dan Yakir and reviewed by four anonymous referees.
AbdElgawad, H., Avramova, V., Baggerman, G., Van Raemdonck, G., Valkenborg, D., Van Ostade, X., Guisez, Y., Prinsen, E., Asard, H., Van den Ende, W., Gerrit, T. S., and Beemster, G. T. S.: Starch biosynthesis contributes to the maintenance of photosynthesis and leaf growth under drought stress in maize, Plant Cell Environ., 43, 2254–2271, https://doi.org/10.1111/pce.13813, 2020.
AbdElgawad, H., Peshev, D., Zinta, G., Van den Ende, W., Janssens, I. A., and Asard, H.: Climate extreme effects on the chemical composition of temperate grassland species under ambient and elevated CO2: a comparison of fructan and non-Fructan accumulators, Plos One, 9, e92044, https://doi.org/10.1371/journal.pone.0092044, 2014.
Ashraf, M. and Harris, P. J. C.: Photosynthesis under stressful environments: An overview, Photosynthetica, 51, 163–190, https://doi.org/10.1007/s11099-013-0021-6, 2013.
Atkin, O. K., Turnbull, M. H., Zaragoza-Castells, J., Fyllas, N. M., Lloyd J., Meir, P., and Griffin, K. L.: Increased light inhibition of respiration as soil fertility declines along a post-glacial chronosequence in New Zealand, Plant Soil, 367, 163–182, https://doi.org/10.1007/s11104-013-1686-0, 2013.
Augé, R. M., Toler, H. D., and Saxton, A. M.: Mycorrhizal stimulation of leaf gas exchange in relation to root colonization, shoot size, leaf phosphorus and nitrogen: a quantitative analysis of the literature using meta-regression, Front. Plant Sci., 7, 1084, https://doi.org/10.3389/fpls.2016.01084, 2016.
Augusto, L., Achat, D. L., Jonard, M., Vidal, D., and Ringeval, B.: Soil parent material – A major driver of plant nutrient limitations in terrestrial ecosystems, Glob. Change Biol., 23, 3808–3824, https://doi.org/10.1111/gcb.13691, 2017.
Beauchamp, E. G. and Hamilton, H. A.: Optimum ratios of nitrogen and phosphorus fertilizers for corn determined by Homes' method of systematic variation, Can. J. Plant Sci., 50, 141–150, https://doi.org/10.4141/cjps70-027, 1970.
Begum, N., Qin, C., Ahanger, M. A., Raza, S., Khan, M. I., Ashraf, M., Ahmed, N., and Zhang, L.: Role of arbuscular mycorrhizal fungi in plant growth regulation: implications in abiotic stress tolerance, Front. Plant Sci., 10, 1068, https://doi.org/10.3389/fpls.2019.01068, 2019.
Brooks, A.: Effects of phosphorus nutrition on ribulose 1,5-bisphosphate carboxylase activation, photosynthetic quantum yield and amounts of some Calvin-cycle metabolites in spinach leaves, Aust. J. Plant Physiol., 13, 221–237, https://doi.org/10.1071/PP9860221, 1986.
Brooks, A., Woo, K. C., and Wong, S. C.: 1988 Effects of phosphorus nutrition on the response of photosynthesis to CO2 and O2, activation of ribulose bisphosphate carboxylase and amounts of ribulose bisphosphate and 3-phosphoglycerate in spinach leaves, Photosyn. Res., 15, 133–141, https://doi.org/10.1007/bf00035257, 1988.
Bhagwat, A. S.: Activation of spinach ribulose 1,5-bisphosphate carboxylase by inorganic phosphate, Plant Sci. Lett., 23, 197–206, 1981.
Burghelea, C., Zaharescu, D. G., Dontsova, K., Maier, R., Huxman, T., and Chorover, J.: Mineral nutrient mobilization by plants from rock: influence of rock type and arbuscular mycorrhiza, Biogeochemistry, 124, 187–203, https://doi.org/10.1007/s10533-015-0092-5, 2015.
Calderón-Vázquez, C., Alatorre-Cobos, F., Simpson-Williamson, J., and Herrera-Estrella, L.: Maize under phosphate limitation, in: Handbook of Maize: Its Biology, edited by: Bennetzen, J. L. and Hake, S. C., Springer, New York, USA, 381–404, https://doi.org/10.1007/978-0-387-79418-1_19, 2009.
Dall'Osto, L., Cazzaniga, S., Havaux, M., and Bassi, R.: Enhanced photoprotection by protein-bound vs free xanthophyll pools: a comparative analysis of chlorophyll b and xanthophyll biosynthesis mutants, Mol. Plant., 3, 576–593, https://doi.org/10.1093/mp/ssp117, 2010.
da Silva, J. M. and Arrabaça, M. C.: Contributions of soluble carbohydrates to the osmotic adjustment in the C4 grass Setaria sphacelata: A comparison between rapidly and slowly imposed water stress, J. Plant Physiol., 161, 551–555, https://doi.org/10.1078/0176-1617-01109, 2004.
Duursma, R. A.: Plantecophys - An R Package for Analysing and Modelling Leaf Gas Exchange Data, Plos One, 10, e0143346, https://doi.org/10.1371/journal.pone.0143346, 2015.
Elser, J. J., Fagan, W. F., Denno, R. F., Dobberfuhl, D. R., Folarin, A., Huberty, A., Interlandi, S., Kilham, S. S., McCauley, E., Schulz, K. L., Siemann E. H., and Sterner, R. W.: Nutritional constraints in terrestrial and freshwater food webs, Nature, 408, 578–580, https://doi.org/10.1038/35046058, 2000.
Emran, M., Rashad, M., Gispert, M., and Pardini, G.: Increasing soil nutrients availability and sustainability by glomalin in alkaline soils, Agricul. Biosystems Eng., 2, 74–84, 2017.
Etesami, H., Jeong, B. R., and Glick, B. R.: Contribution of arbuscular mycorrhizal fungi, phosphate–solubilizing bacteria, and silicon to P uptake by plant, Front. Plant Sci., 12, 1355, https://doi.org/10.3389/fpls.2021.699618, 2021.
Griffin, K. L. and Seemann, J. R.: Plants, CO2 and photosynthesis in the 21st century, Chem. Biol., 3, 245–254, https://doi.org/10.1016/S1074-5521(96)90104-0, 1996.
Güsewell, S.: N : P ratios in terrestrial plants: Variation and functional significance, New Phytol., 164, 243–266, https://doi.org/10.1111/j.1469-8137.2004.01192.x, 2004.
Halsted, M. and Lynch, J. P.: Phosphorus responses of C3 and C4 species, J. Exp. Bot., 47, 497–505, https://doi.org/10.1093/jxb/47.4.497, 1996.
Hartnett, D. C. and Wilson, G. W. T.: Mycorrhizae influence plant community structure and diversity in tallgrass prairie, Ecology, 80, 1187–1195, https://doi.org/10.1890/0012-9658(1999)080[1187:MIPCSA]2.0.CO;2, 1999.
Heskel, M. A. and Tang, J.: Environmental controls on light inhibition of respiration and leaf and canopy daytime carbon exchange in a temperate deciduous forest, Tree Physiol., 38, 1886–1902, https://doi.org/10.1093/treephys/tpy103, 2018.
Heskel, M. A., Anderson, O. R., Atkin, O. K., Turnbull, M. H., and Griffin, K. L.: Leaf- and cell-level carbon cycling responses to a nitrogen and phosphorus gradient in two Arctic tundra species, Am. J. Bot., 99, 1702–1714, https://doi.org/10.3732/ajb.1200251, 2012.
Heskel, M. A., Atkin, O. K., Turnbull, M. H., and Griffin, K. L.: Bringing the Kok effect to light: A review on the integration of daytime respiration and net ecosystem exchange, Ecosphere, 4, 1–14, https://doi.org/10.1890/ES13-00120.1, 2013.
Hoeksema, J. D., Chaudhary, V. B., Gehring, C. A., Johnson, N. C., Karst, J., Koide, R. T., Pringle, A., Zabinski, C., Bever, J. D., Moore, J. C., Wilson, G. W. T., Klironomos, J. N., and Umbanhowar, J.: A meta-analysis of context-dependency in plant response to inoculation with mycorrhizal fungi, Ecol. Lett., 13, 394–407, https://doi.org/10.1111/j.1461-0248.2009.01430.x, 2010.
Hu, Y., Chen, J., Hui, D., Wang, Y.-P., Li, J., Chen, J., Chen, G., Zhu, Y., Zhang, L., Zhang, D., and Deng, Q.: Mycorrhizal fungi alleviate acidification-induced phosphorus limitation: Evidence from a decade-long field experiment of simulated acid deposition in a tropical forest in south China, Glob. Change Biol., 00, 1–15, https://doi.org/10.1111/gcb.16135, 2022.
Jacob, J. and Lawlor, D. W.: Stomatal and mesophyll limitations of photosynthesis in phosphate deficient sunflower, maize and wheat plants, J. Exp. Bot., 42, 1003–1011, https://doi.org/10.1093/jxb/42.8.1003, 1991.
Jacob, J. and Lawlor, D. W.: Dependence of photosynthesis of sunflower and maize leaves on phosphate supply, ribulose-1,5-bisphosphate carboxylase/oxygenase activity, and ribulose-1,5-bisphosphate pool size, Plant Physiol., 98, 801–807, https://doi.org/10.1104/pp.98.3.801, 1992.
Jahns, P. and Holzwarth, A. R.: The role of the xanthophyll cycle and of lutein in photoprotection of photosystem II, Biochim. Biophys. Ac., 1817, 182–193, https://doi.org/10.1016/j.bbabio.2011.04.012, 2012.
Jansa, J., Finlay, R., Wallander, H., Smith, F. A., and Smith, S. E.: Role of mycorrhizal symbioses in phosphorus cycling, in: Phosphorus in Action. Soil Biology, vol 26, edited by: Bünemann, E., Oberson, A. and Frossard, E., Springer, Berlin, Heidelberg, Germany, 137–168, https://doi.org/10.1007/978-3-642-15271-9_6, 2011.
Jeannette, E., Reyss, A., Gregory, N., Gantet, P., and Prioul, J. L.: Carbohydrate metabolism in a heat-girdled maize source leaf, Plant Cell Environ., 23, 61–69, https://doi.org/10.1046/j.1365-3040.2000.00519.x, 2000.
Johnston, A. E. and Poulton, P. R.: Phosphorus in Agriculture: A Review of Results from 175 Years of Research at Rothamsted, UK, J. Environ. Qual., 48, 1133–1144, https://doi.org/10.2134/jeq2019.02.0078, 2019.
Khan, A., Lu, G., Ayaz, M., Zhang, H., Wang, R., Lv, F., Yang, X., Sun, B., and Zhang, S.: Phosphorus efficiency, soil phosphorus dynamics and critical phosphorus level under long-term fertilization for single and double cropping systems, Agr. Ecosyst. Environ., 256, 1–11, https://doi.org/10.1016/j.agee.2018.01.006, 2018.
Kobae, Y.: Dynamic phosphate uptake in arbuscular mycorrhizal roots under field conditions, Front. Environ. Sci., 6, 159, https://doi.org/10.3389/fenvs.2018.00159, 2019.
Koerselman, W. and Meuleman, A. F. M.: The vegetation N : P ratio: a new tool to detect the nature of nutrient limitation, J. Appl. Ecol., 33, 1441–1450, https://doi.org/10.2307/2404783, 1996.
Kok, B.: A critical consideration of the quantum yield of Chlorella-photosynthesis, Enzymologia, 13, 1–56, 1948.
Kuczyńska, P., Latowski, D., Niczyporuk, S., Olchawa-Pajor, M., Jahns, P., Gruszecki, W. I., and Strzałka, K.: Zeaxanthin epoxidation – an in vitro approach, Acta Biochim. Pol. 59, 105–107, https://doi.org/10.18388/abp.2012_2182, 2012.
Lambers, H., Raven, J. A., Shaver, G. R., and Smith, S. E.: Plant nutrient-acquisition strategies change with soil age, Trends Ecol. Evol., 23, 95–103, https://doi.org/10.1016/j.tree.2007.10.008, 2008.
Lewis, J. D., Griffin, K. L., Thomas, R. B., and Strain, B. R.: Phosphorus supply affects the photosynthetic capacity of loblolly pine grown in elevated carbon dioxide, Tree Physiol., 14, 1229–1244, https://doi.org/10.1093/treephys/14.11.1229, 1994.
Loustau, D., Brahim, M. B., Gaudillère, J. P., and Dreyer, E.: Photosynthetic responses to phosphorus nutrition in two-year-old maritime pine seedlings, Tree Physiol., 19, 707–715, https://doi.org/10.1093/treephys/19.11.707, 1999.
Miner, G. L. and Bauerle, W. L.: Seasonal responses of photosynthetic parameters in maize and sunflower and their relationship with leaf functional traits, Plant Cell Environ., 42, 1561–1574, https://doi.org/10.1111/pce.13511, 2019.
Miransari, M.: Contribution of arbuscular mycorrhizal symbiosis to plant growth under different types of soil stress, Plant Biol., 12, 563–569, https://doi.org/10.1111/j.1438-8677.2009.00308.x, 2010.
Parihar, M., Rakshit, A., Meena, V. S., Gupta, V. K., Rana, K., Choudhary, M., Tiwari, G., Mishra, P. K., Pattanayak, A., Bisht, J. K., Jatav, S. S., Khati, P., and Jatav, H. S.: The potential of arbuscular mycorrhizal fungi in C cycling: a review, Arch. Microbiol., 202, 1581–1596, https://doi.org/10.1007/s00203-020-01915-x, 2020.
Parry, M. A., Keys, A. J., Madgwick, P. J., Carmo-Silva, A. E., and Andralojc, P. J.: Rubisco regulation: a role for inhibitors, J. Exp. Bot., 59, 1569–1580, https://doi.org/10.1093/jxb/ern084, 2008.
Paul, M. J. and Foyer, C. H.: Sink regulation of photosynthesis, J. Exp. Bot., 52, 1383–1400, https://doi.org/10.1093/jexbot/52.360.1383, 2001.
Paul, M. J. and Stitt, M.: Effects of nitrogen and phosphorus deficiencies on levels of carbohydrates, respiratory enzymes and metabolites in seedlings of tobacco and their response to exogenous sucrose, Plant Cell Environ., 16, 1047–1057, https://doi.org/10.1111/j.1365-3040.1996.tb02062.x, 1993.
Peñuelas, J., Poulter, B., Sardans, J., Ciais, P., van der Velde, M., Bopp, L., Boucher, O., Godderis, Y., Hinsinger, P., Llusia, J., Nardin, E., Vicca, S., Obersteiner, M., and Janssens, I. A.: Human-induced nitrogen-phosphorus imbalances alter natural and managed ecosystems across the globe. Nat. Commun., 4, 2934, https://doi.org/10.1038/ncomms3934, 2013.
Pieters, A. J., Paul, M. J., and Lawlor, D. W.: Low sink demand limits photosynthesis under P(i) deficiency, J. Exp. Bot., 52, 1083–1091, https://doi.org/10.1093/jexbot/52.358.1083, 2001.
Plenchette, C., Clermont-Dauphin, C., Meynard, J. M., and Fortin, J. A.: Managing arbuscular mycorrhizal fungi in cropping systems, Can. J. Plant Sci., 85, 31–40, https://doi.org/10.4141/P03-159, 2005.
Rao, I. M. and Terry, N.: Leaf phosphate status, photosynthesis, and carbon partitioning in sugar beet I. Changes in growth, gas exchange, and Calvin cycle enzymes, Plant Physiol., 90, 814–819, https://doi.org/10.1104/pp.90.3.814, 1989.
Řezáčová, V., Slavíková, R., Zemková, L., Konvalinková, T., Procházková, V., Št'ovíček, V., Hršelová, H., Beskid, O., Hujslová, M., Gryndlerová, H., Gryndler, M., Püschel, D., and Jansa, J.: Mycorrhizal symbiosis induces plant carbon reallocation differently in C3 and C4 Panicum grasses, Plant Soil, 425, 441–456, https://doi.org/10.1007/s11104-018-3606-9, 2018.
Rillig, M. C., Field, C. B., and Allen, M. F.: Soil biota responses to long-term atmospheric CO2 enrichment in two California annual grasslands, Oecologia, 119, 572–577, https://doi.org/10.1007/s004420050821, 1999.
Rodríguez, D. and Goudriaan, J.: Effects of phosphorus and drought stresses on dry-matter and phosphorus allocation in wheat, J. Plant Nutr., 18, 2501–2517, https://doi.org/10.1080/01904169509365080, 1995.
Rodríguez, D., Keltjens, W. G., and Goudriaan, J.: Plant leaf area expansion and assimilate production in wheat (Triticum aestivum L.) growing under low phosphorus conditions, Plant Soil, 200, 227–240, https://doi.org/10.1023/a:1004310217694, 1998.
Rodríguez, D., Andrade, F. H., and Goudriaan, J.: Does assimilate supply limit leaf expansion in wheat grown in the field under low phosphorus availability?, Field Crops Res., 67, 227–238, https://doi.org/10.1016/s0378-4290(00)00098-8, 2000.
Rogers, A.: The use and misuse of Vcmax in Earth System Models, Photosynth. Res., 119, 15–29, https://doi.org/10.1007/s11120-013-9818-1, 2014.
Roy-Bolduc, A. and Hijri, M.: The use of mycorrhizae to enhance phosphorus uptake: A way out the phosphorus crisis, J. Biofertil. Biopestici., 2, 104, https://doi.org/10.4172/2155-6202.1000104, 2011.
Sánchez-Calderón, L., Chacon-López, A., Perez-Torres, C., and Herrera-Estrella, L.: Phosphorus: Plants strategies to cope with its scarcity, in: Cell Biology of Metals and Nutrients, Plant Cell Monographs, vol 17, edited by: Hell, R. and Mendel, R. R., Springer, Berlin, Heidelberg, Germany, 173–198, https://doi.org/10.1007/978-3-642-10613-2_8, 2010.
Schlüter, U., Colmsee, C., Scholz, U., Bräutigam, A., Weber, A. P. M., Zellerhoff, N., Bucher, M., Fahnenstich, H., and Sonnewald, U.: Adaptation of maize source leaf metabolism to stress related disturbances in carbon, nitrogen and phosphorus balance, BMC Genomics 14, 442, https://doi.org/10.1186/1471-2164-14-442, 2013.
Schulze, E.-D., Beck, E., and Müller-Hohenstein, K. (Eds.): Plant Ecology, Springer, Berlin, Heidelberg, Germany, ISBN 3-540-20833-X, 2005.
Shapiro, J. B., Griffin, K. L., Lewis, J. D., and Tissue, T. D.: Response of Xanthium strumarium leaf respiration in the light to elevated CO2 concentration, nitrogen availability and temperature, New Phytol., 162, 377–386, https://doi.org/10.1111/j.1469-8137.2004.01046.x, 2004.
Smith, S. E. and Read, D. J.: Mycorrhizal Symbiosis, 3rd Edn., Academic Press, London, UK, ISBN 9780123705266, 2008.
Smith, S. E., Jakobsen, I., Grønlund, M., and Smith, F. A.: Roles of arbuscular mycorrhizas in plant phosphorus nutrition: Interactions between pathways of phosphorus uptake in arbuscular mycorrhizal roots have important implications for understanding and manipulating plant phosphorus acquisition, Plant Physiol., 156, 1050–1057, https://doi.org/10.1104/pp.111.174581, 2011.
Sulpice, R., Tschoep, H., von Korff, M., Bussis, D., Usadel, B., Hohne, M., Witucka-Wall, H., Altmann, T., Stitt, M., and Gibon, Y.: Description and applications of a rapid and sensitive non-radioactive microplate-based assay for maximum and initial activity of D-ribulose-1,5-bisphosphate carboxylase/oxygenase, Plant Cell Environ., 30, 1163–1175, https://doi.org/10.1111/j.1365-3040.2007.01679.x, 2007.
Temminghoff, E. E. J. M. and Houba, V. J. G.: Plant Analysis Procedures, Springer Netherlands, Dordrecht, https://doi.org/10.1007/978-1-4020-2976-9, 2004.
Tennant, D.: A test of a modified line intersect method of estimating root length, J. Ecol., 63, 995–1001, https://doi.org/10.2307/2258617, 1975.
Terrer, C., Vicca, S., Hungate, B. A., Phillips, R. P., and Prentice, I. C.: Mycorrhizal association as a primary control of the CO2 fertilization effect, Science, 353, 72–74, https://doi.org/10.1126/science.aaf4610, 2016.
Thum, T., Caldararu, S., Engel, J., Kern, M., Pallandt, M., Schnur, R., Yu, L., and Zaehle, S.: A new model of the coupled carbon, nitrogen, and phosphorus cycles in the terrestrial biosphere (QUINCY v1.0; revision 1996), Geosci. Model Dev., 12, 4781–4802, https://doi.org/10.5194/gmd-12-4781-2019, 2019.
Usuda, H. and Shimogawara, K.: Phosphate deficiency in maize, I. Leaf phosphate status, growth, photosynthesis and carbon partitioning, Plant Cell Physiol., 32, 497–504, https://doi.org/10.1093/oxfordjournals.pcp.a078107, 1991.
Ven, A., Verbruggen, E., Verlinden, M. S., Olsson, P. A., Wallander, H., and Vicca, S.: Mesh bags underestimated arbuscular mycorrhizal abundance but captured fertilization effects in a mesocosm experiment, Plant Soil, 446, 563–575, https://doi.org/10.1007/s11104-019-04368-4, 2020a.
Ven, A., Verlinden, M. S., Fransen, E., Olsson, P. A., Verbruggen, E., Wallander, H., and Vicca, S.: Phosphorus addition increased carbon partitioning to autotrophic respiration but not to biomass production in an experiment with Zea mays, Plant Cell Environ., 43, 2054–2065, https://doi.org/10.1111/pce.13785, 2020b.
Veneklaas, E. J., Lambers, H., Bragg, J., Finnegan, P. M., Lovelock, C. E., Plaxton, W. C., Price, C., Scheible, W.-R., Shane, M. W., White, P. J., and Raven, J. A.: Opportunities for improving phosphorus-use efficiency in crop plants, New Phytol., 195, 306–320, https://doi.org/10.1111/j.1469-8137.2012.04190.x, 2012.
Verlinden, M. S., Broeckx, L. S., Zona, D., Berhongaray, G., De Groote, T., Camino Serrano, M., Janssens, I. A., and Ceulemans, R.: Net ecosystem production and carbon balance of an SRC poplar plantation during its first rotation, Biomass Bioenerg., 56, 412–422, https://doi.org/10.1016/j.biombioe.2013.05.033, 2013.
Verlinden, M. S., Ven, A., Verbruggen, E., Janssens, I. A., Wallander, H., and Vicca, S.: Favorable effect of mycorrhizae on biomass production efficiency exceeds their carbon cost in a fertilization experiment, Ecology, 99, 2525–2534, https://doi.org/10.1002/ecy.2502, 2018.
Verlinden, M. S., AbdElgawad, H., Ven, A., Verryckt, L. T., Wieneke, S., Janssens, I. A., and Vicca, S.: Phosphorus stress strongly reduced plant physiological activity, but only temporarily, in a mesocosm experiment with Zea mays colonized by arbuscular mycorrhizal fungi, Zenodo [data set], https://zenodo.org/record/6428027 (last access: 9 April 2022), 2022.
Vicca, S., Zavalloni, C., Fu, Y., Voets, L., Dupré de Boulois, H., Declerck, S., Ceulemans, R., Nijs, I., and Janssens, I.A.: Arbuscular mycorrhizal fungi may mitigate the influence of a joint rise of temperature and atmospheric CO2 on soil respiration in grasslands, Int. J. Ecol., 2009, ID 209768, 10 pp., https://doi.org/10.1155/2009/209768, 2009.
Vicca, S., Stocker, B., Reed, S. C., Wieder, W. R., Bahn, M., Fay, P. A., Janssens, I. A., Lambers, H., Peñuelas, J., Piao, S., Rebel, K., Sardans, J., Sigurdsson, B. D., Van Sundert, K., Wang, Y., Zaehle, S., and Ciais, P.: Using research networks to create the comprehensive datasets needed to assess nutrient availability as a key determinant of terrestrial carbon cycling, Environ. Res. Lett., 13, 125006, https://doi.org/10.1088/1748-9326/aaeae7, 2018.
Vierheilig, H., Schweiger, P., and Brundrett, M.: An overview of methods for the detection and observation of arbuscular mycorrhizal fungi in roots, Physiol. Plantarum, 125, 393–404, https://doi.org/10.1111/j.1399-3054.2005.00564.x, 2005.
Vitousek, P. M., Porder, S., Houlton, B. Z., and Chadwick, O. A.: Terrestrial phosphorus limitation: mechanisms, implications, and nitrogen-phosphorus interactions, Ecol. Appl., 20, 5–15, https://doi.org/10.1890/08-0127.1, 2010.
von Caemmerer, S. (Ed.): Biochemical models of leaf photosynthesis, CSIRO Publishing, Melbourne, Australia, ISBN 0 643 06379 X, 2000.
Wang, Y. P., Law, R. M., and Pak, B.: A global model of carbon, nitrogen and phosphorus cycles for the terrestrial biosphere, Biogeosciences, 7, 2261–2282, https://doi.org/10.5194/bg-7-2261-2010, 2010.
Wieder, W. R., Cleveland, C. C., Smith, W. K., and Todd-Brown, K.: Future productivity and carbon storage limited by terrestrial nutrient availability, Nat. Geosci., 8, 441–444, https://doi.org/10.1038/ngeo2413, 2015.
Wright, D. P., Scholes, J. D., Read, D. J., and Rolfe, S. A.: European and African maize cultivars differ in their physiological and molecular responses to mycorrhizal infection, New Phytol., 167, 881–896, https://doi.org/10.1111/j.1469-8137.2005.01472.x, 2005.
Zhang, K., Liu, H., Tao, P., and Chen, H.: Comparative proteomic analyses provide new insights into low phosphorus stress responses in maize leaves, Plos One, 9, e98215, https://doi.org/10.1371/journal.pone.0098215, 2014.