the Creative Commons Attribution 4.0 License.
the Creative Commons Attribution 4.0 License.
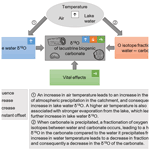
A modern snapshot of the isotopic composition of lacustrine biogenic carbonates – records of seasonal water temperature variability
Franziska Tell
Ulrich von Grafenstein
Dan Hammarlund
Henning Kuhnert
Bénédicte Minster
Carbonate shells and encrustations from lacustrine organisms provide proxy records of past environmental and climatic changes. The carbon isotopic composition (δ13C) of such carbonates depends on the δ13C of dissolved inorganic carbon (DIC). Their oxygen isotopic composition (δ18O) is controlled by the δ18O of the lake water and by water temperature during carbonate precipitation. Lake water δ18O, in turn, reflects the δ18O of atmospheric precipitation in the catchment area, water residence time and mixing, and evaporation. A paleoclimatic interpretation of carbonate isotope records requires a site-specific calibration based on an understanding of these local conditions.
For this study, samples of different biogenic carbonate components and water were collected in the littoral zone of Lake Locknesjön, central Sweden (62.99∘ N, 14.85∘ E, 328 ) along a water depth gradient from 1 to 8 m. Carbonate samples of living organisms and subfossil remains in surface sediments were taken from the calcifying alga Chara hispida, from bivalve mollusks of the genus Pisidium, and from adult and juvenile instars of two ostracod species, Candona candida and Candona neglecta.
Our results show that neither the isotopic composition of carbonates nor the δ18O of water vary significantly with water depth, indicating a well-mixed epilimnion. The mean δ13C of Chara hispida encrustations is 4 ‰ higher than the other carbonates. This is due to fractionation related to photosynthesis, which preferentially incorporates 12C into the organic matter and increases the δ13C of the encrustations. A small effect of photosynthetic 13C enrichment in DIC is seen in contemporaneously formed valves of juvenile ostracods. The largest differences in the mean carbonate δ18O between species are caused by vital offsets, i.e., the species-specific deviations from the δ18O of inorganic carbonate which would have been precipitated in isotopic equilibrium with the water. After subtraction of these offsets, the remaining differences in the mean carbonate δ18O between species can mainly be attributed to seasonal water temperature changes. The lowest δ18O values are observed in Chara hispida encrustations, which form during the summer months when photosynthesis is most intense. Adult ostracods, which calcify their valves during the cold season, display the highest δ18O values. The seasonal and interannual variability in lake water δ18O is small (∼ 0.5 ‰) due to the long water residence time in the lake. Seasonal changes in the temperature-dependent fractionation are therefore the dominant cause of carbonate δ18O differences between species when vital offsets are corrected.
Temperature reconstructions based on paleotemperature equations for equilibrium carbonate precipitation using the mean δ18O of each species and the mean δ18O of lake water are well in agreement with the observed seasonal water temperature range. The high carbonate δ18O variability of samples within a species, on the other hand, leads to a large scatter in the reconstructed temperatures based on individual samples. This implies that care must be taken to obtain a representative sample size for paleotemperature reconstructions.
- Article
(15953 KB) - Full-text XML
- BibTeX
- EndNote
The oxygen and carbon isotopic compositions of biogenic carbonates from lake sediments are powerful tools for reconstructing past climatic and environmental changes. Despite the complex influences of climate, hydrology, catchment and lake processes, as well as biological effects, they may be interpreted, depending on the local context, as proxies of temperature, evaporation, the isotopic composition of atmospheric precipitation, or atmospheric circulation patterns (e.g., Holmes et al., 2020; Andersson et al., 2010; Jonsson et al., 2010; Hammarlund et al., 2003; Schwalb, 2003; Hammarlund et al., 2002; von Grafenstein et al., 1999a).
Calcium carbonate (CaCO3) remains of lacustrine organisms can be identified based on the characteristic morphology of shells, valves or encrustations. For paleoclimate reconstructions, it is important to assess the isotopic composition of specific biogenic carbonates to avoid the difficulties involved in the interpretation of isotopic records obtained on bulk carbonate matter in lake sediments. Changes in the bulk carbonate composition can be caused by variations in the assemblage of calcifying organisms, in clastic carbonate input, or in the amount of inorganic calcite precipitated in the lake (e.g., Bright et al., 2006; Hammarlund and Buchardt, 1996). Furthermore, carbonate minerals such as calcite, aragonite or dolomite differ in their isotopic compositions, so a potentially variable carbonate mineralogy both related to coeval sedimentation and to early diagenesis must be considered (McCormack and Kwiecien, 2021; McCormack et al., 2019).
The δ18O and δ13C of lacustrine biogenic carbonates depend on the isotopic composition of the oxygen and carbon sources, water and dissolved inorganic carbon (DIC), respectively. The isotopic composition of these sources can be variable over space and time, and is modified during carbonate precipitation, mainly through temperature-dependent fractionation and fractionation during physiological processes.
The δ18O of biogenic carbonates carries the isotopic signature of the lake water, which, in turn, depends on the δ18O of atmospheric precipitation in the catchment area, and is increased by evaporation from the lake (Gat, 1996). An increase in air temperature leads to a higher δ18O of lake water through its effect on the δ18O of atmospheric precipitation and on the evaporative enrichment of lake water, and consequently to an increase in carbonate δ18O (see Fig. 1). Biogenic carbonates forming at different water depths may differ in their δ18O, e.g., only those formed above the thermocline are affected by higher temperatures and evaporation during times of thermal stratification of the lake.
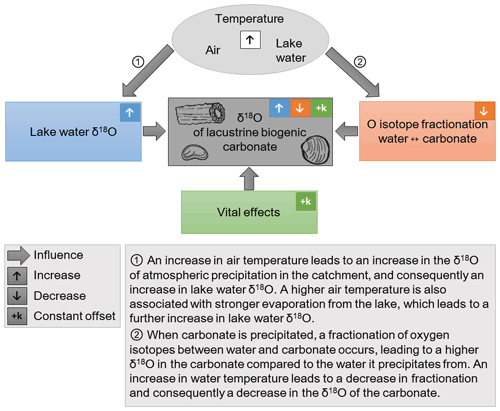
Figure 1A simplified schematic representation of the influences on the oxygen isotopic composition (δ18O) of lacustrine biogenic carbonates. The isotopic composition of precipitation is correlated with air temperature in middle and high latitudes (Rozanski et al., 1992). A higher air temperature leads to a higher lake water δ18O, as indicated in ①, which is reflected in the δ18O of the carbonates. There are additional influences on lake water δ18O, such as catchment and lake hydrology, relative humidity, and the seasonal distribution of atmospheric precipitation, which are not represented in the figure. The fractionation of oxygen isotopes between water and carbonate is also temperature-dependent, as indicated in ②. Note the opposite directions of the temperature influence on ① lake water δ18O and on ② fractionation. As a consequence, temperature has two opposing effects on the δ18O of carbonates. Lastly, “vital effects” which are dependent on the specific physiology of each species, lead to a constant offset between the δ18O of the biogenic carbonate and inorganic carbonate which would precipitate in isotopic equilibrium with the water.
The δ13C of biogenic carbonates carries the isotopic signature of DIC (McConnaughey, 1989; Ito, 2001). It depends on the δ13C signal of DIC in recharging groundwater that is transferred into the lake. The δ13C of groundwater DIC is controlled by exchanges between atmospheric CO2, vegetation and soil air CO2, and carbonate bedrock under open or closed system conditions (Clark and Fritz, 1997). In lake water, the δ13C of DIC can vary seasonally, depending on photosynthetic activity in the photic zone, the oxidation of organic matter, and thermal stratification.
The fractionation of oxygen isotopes between water and carbonate is temperature-dependent (Grossman and Ku, 1986; Kim and O'Neil, 1997; Beck et al., 2005; Kim et al., 2007). Biogenic carbonates formed during different times of the year can therefore differ in δ18O due to seasonal water temperature changes, which are especially strong in shallow water. Fractionation factors for carbonate precipitated in isotopic equilibrium with the water are known from empirical studies. However, many earth surface carbonates precipitate out of isotopic equilibrium (Daëron et al., 2019). An increase in water temperature leads to a decrease in the fractionation of oxygen isotopes between water and carbonate (see Fig. 1), and consequently to a decrease in the δ18O of equilibrium carbonate by −0.24 ‰ ∘C−1 (Craig and Gordon, 1965; Kim and O'Neil, 1997). Note that the relationship between atmospheric precipitation δ18O and air temperature is opposite, with a δ18O increase of ∼ 0.6 ‰ ∘C−1 in mid-latitudes (Rozanski et al., 1992). Kinetic effects on fractionation occur in the case of fast carbonate precipitation, observed, e.g., in Chara due to intense photosynthesis (Apolinarska et al., 2016; Andrews et al., 2004), which lowers the carbonate δ18O. Furthermore, variations in the pH have an influence on the isotopic composition of carbonates, with an increasing pH lowering the δ-values (Herbst et al., 2018; Teranes et al., 1999; McConnaughey, 1989).
Fractionation due to physiological processes in a calcifying organism leads to a different isotopic composition of the biogenic calcite compared to inorganic calcite precipitated in isotopic equilibrium. This species-specific vital offset can be quantified through laboratory studies under controlled conditions (Li and Liu, 2010; Chivas et al., 2002; Xia et al., 1997b) or through monitoring in the field (Börner et al., 2017; Decrouy et al., 2011b; Keatings et al., 2002; von Grafenstein et al., 1999b). In addition to the vital offsets, the conditions in the micro-habitat of the carbonate-producing species can play a role as well (Decrouy et al., 2011a; Xia et al., 1997a). If the vital offsets for a given species are known and appear to be constant, they can be corrected for and do not hamper proxy interpretations. However, the causes of vital effects are not yet fully understood, and it has been suggested that varying water chemistry has an influence (Devriendt et al., 2017; Decrouy et al., 2011b).
Recent studies have highlighted the seasonal bias in proxies of past sea surface temperatures and the importance of distinguishing between annual and seasonal temperature reconstructions (Bova et al., 2021; Hertzberg, 2021). Lacustrine biogenic carbonates formed during different times of the year can provide insights into seasonal temperature variations. In this context, seasonal influences under modern conditions must be understood. Potential past water depth changes must be considered, because these could impact the location of carbonate formation with respect to a thermocline, changing the relationship between carbonates from organisms living at times of lake water stratification vs. at times of a mixed water column.
For the use of δ18O and δ13C in lacustrine biogenic carbonates as paleoenvironmental proxies, the different influencing factors must be known or constrained. For example, benthic ostracods in deep lakes living below the permanent thermocline form their calcitic valves at a nearly constant temperature around 4 ∘C, close to the density maximum of freshwater. Variations in the δ18O therefore primarily reflect variations in the δ18O of atmospheric precipitation if evaporative enrichment in 18O of the lake water is negligible (von Grafenstein and Labuhn, 2021). Under such optimal conditions, the δ18O of ostracod valves provides the basis for reconstructing precipitation δ18O (e.g., von Grafenstein et al., 1999a). However, ostracod population densities are usually low and carbonates are not always preserved in profundal sediments. Carbonate-producing organisms (including algae that need light for photosynthesis) are often more abundant at littoral sites, where the interpretation of isotope proxies is complicated by a larger seasonal water temperature amplitude and a stronger influence of evaporation.
Disentangling the influences on the isotopic composition of specific biogenic carbonates is possible based on monitoring of the relevant parameters (such as temperature, δ18O and pH of lake water, and the δ13C of DIC), for a sufficiently long time to represent the entire time period of calcification of each organism and to capture seasonal and interannual variability. Monitoring can provide detailed understanding for interpretation of the proxies in the local context of each study (Börner et al., 2017; Meyer et al., 2017; Mayr et al., 2015), but long-term investigations (e.g., von Grafenstein et al., 1999b; Decrouy et al., 2011b) are rare and subject to logistic and financial constraints.
In this study, we therefore rely on a single sampling occasion to investigate the oxygen and carbon isotopic composition (δ18O and δ13C) of different carbonate-producing species from Lake Locknesjön in central Sweden. At shallow water sites, the following types of samples were taken from living organisms and their subfossil remains in surface sediments: the calcite encrustations of the algae Chara hispida, the aragonitic valves of the bivalve mollusk Pisidium sp., and the calcitic valves of two species of ostracods, Candona candida and Candona neglecta. In addition, two species of charophytes, Chara aspera and Chara hispida were sampled in the nearby Lake Blektjärnen.
Our goals are (1) to test whether this approach of a “snapshot” study with short-term sampling can narrow down the primary environmental drivers of the isotopic composition of specific biogenic carbonates when long-term monitoring studies are not feasible. We focus on interspecies and intraspecies variations and compare them against the δ18O of local atmospheric precipitation, streams and lake water, considering vital offsets and the timing of calcification for each species. We further (2) assess the uncertainty in seasonal water temperature reconstructions based on isotope measurements of lake water and of carbonates from multiple species. Lastly (3), this snapshot breaks down the details of what could be represented in a single measurement when building long isotope time series from sediment records. We discuss the sensitivity of such a measurement to the choice of the sampled material, and the implications for sub-sampling of sediment cores for paleoenvironmental studies. These investigations will also aid the site-specific paleoenvironmental interpretation of equivalent measurements from Holocene sediments in Lake Locknesjön.
2.1 Study site
Lake Locknesjön is located in central Sweden (62.99∘ N, 14.85∘ E, 328 , Fig. 2). It is one of the few carbonate lakes in northern Scandinavia, and abundant biogenic carbonate deposits are found in its littoral sediments, with charophyte encrustations as the dominant sediment component. The lake has an area of 27 km2, a maximum depth of 57.4 m and a mean depth of 17.6 m (SMHI, 2020a). The catchment area is 134 km2, of which about 20 % is the lake itself, 65 % is coniferous forest, 10 % is agricultural land, and the rest is heathland, peat bogs and rural settlements (SMHI, 2020a). There are no other lakes upstream in the catchment area.
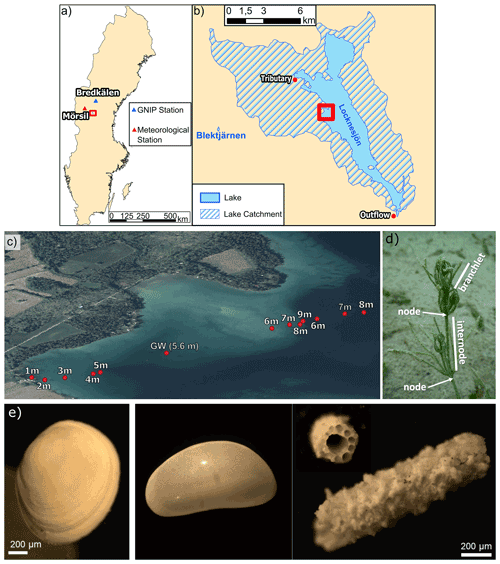
Figure 2(a) Map of Sweden showing the location of the study area (red rectangle), of the GNIP station Bredkälen and of the meteorological station Mörsil. (b) Lake Locknesjön with its catchment (hatched) and Lake Blektjärnen. (c) Locations of the sampling sites on a transect from the shore towards the center of the lake. The area shown in (b) is marked by the red rectangle in (a) and the area shown in (c) is marked in (b) (country outline from USGS, lake and catchment outline from SMHI, 2020a; orthophoto © Lantmäteriet 2020). (d) Photograph of a Chara hispida in Lake Locknesjön. An internode (between two nodes) and a branchlet are indicated on the image. The part of the Chara hispida visible on the photograph measures about 2 cm in height. (e) Images of different lacustrine biogenic carbonates analyzed in this study: (left) Pisidium valve, (middle) adult Candona candida valve, (right) Chara hispida encrustations.
The bedrock in the catchment area is dominated by Cambrian to Silurian carbonate-rich sedimentary rock, with some Precambrian crystalline bedrock in the southern part. The lake's central part lies within the Lockne Crater, which was formed by a meteorite impact 458 Ma ago (Ormö et al., 2010). The impact structure consists of impact breccia and resurge deposits (Sturkell et al., 2013). The fracture frequency of the breccia is estimated to be 25 times higher than in the surrounding crystalline bedrock, and the porosity is 2–10 times higher (Bäckström, 2005). These characteristics, together with the karstified carbonate bedrock, facilitate groundwater flow in the catchment area (Dahlqvist et al., 2018). The Quaternary deposits consist mostly of glacial till with a typical thickness of 3–5 m (Dahlqvist et al., 2018). During the last deglaciation, the ice margin retreated northwestward from the study area around 10 000 cal. yr BP (Stroeven et al., 2015; Labuhn et al., 2018).
The hydrological model of the Swedish Meteorological and Hydrological Institute (SMHI) gives an average discharge at the outflow of Lake Locknesjön of 1.28 m3 s−1 (with a mean high water discharge of 3.10 m3 s−1 and a mean low water discharge of 0.55 m3 s−1), and a residence time of 11.5 years. Inflow of surface water to the lake is limited to a few small streams. The modeled average discharge of the largest inflows combined is 0.37 m3 s−1 (SMHI, 2020a). Based on this modeled river discharge data, groundwater can be estimated to make up about 70 % of the inflow to the lake. During our field work, groundwater inflows were identified based on a bubbling movement of fine sediment on the lake floor, which was observed on several locations along the sampled transect (see https://doi.org/10.5446/56815, Labuhn et al., 2021). Dahlqvist et al. (2018) described traces of underwater erosion as further indications of groundwater inflow, with water temperatures at such inflows 6–8 ∘C lower than in the surrounding lake water.
Lake Locknesjön is ice-covered from early December to mid-May (1961–1990 average; SMHI, 2020a). It is a dimictic lake, which is stratified during January and from July to September, and mixed between these periods (Fig. 3a; Eklund, 1998). Autumn mixing occurred at temperatures of about 9 ∘C in 1957 (the only year for which monthly water temperature measurements are available). The average pH of the lake water is 8.16, with values up to 8.33 above the thermocline during summer (Fig. 3b; data from the Triple Lakes Project, http://www.triplelakes.se/, last access: 16 February 2021).
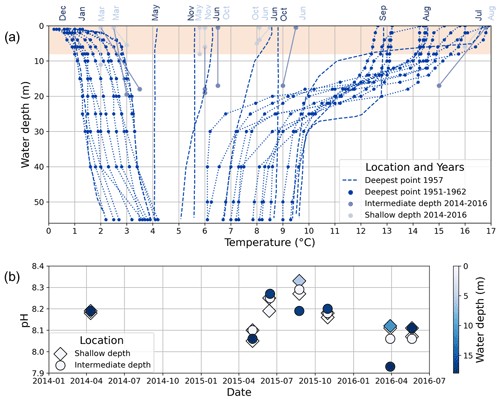
Figure 3(a) Water temperature profiles from Lake Locknesjön. Dark blue dots represent measurements taken between 1951 and 1962, two times per year approximately at the time when the water was coldest during winter (February–March) and warmest during summer (August). Profiles extend from the surface to the bottom at the lake's deepest point. The dashed lines show measurements taken at the same point throughout the year 1957 in the indicated months (data from Eklund, 1998). Other dots represent measurements taken between 2014 and 2016 from surface and bottom water at two sites of shallow (<11 m, light blue dots) and intermediate (<20 m, light gray dots) water depth (data from the Triple Lakes Project, http://www.triplelakes.se/, last access: 16 February 2021). Dotted and solid lines connect measurements taken from different depths at the same location and on the same day. The shaded area marks the water depths from which carbonate samples were taken. (b) pH measured in surface and bottom water at two sites of shallow and intermediate depth between 2014 and 2016 (data from the Triple Lakes Project).
The average annual air temperature at the meteorological station of Mörsil, 70 km northwest of the lake, is 2.3 ∘C, and the average annual precipitation is 663 mm (1975–2005 averages; SMHI, 2020b). In the year of sampling (2018), the average May–July temperature was 2 ∘C higher and the May–July precipitation sum was 40 mm lower than in the reference period (Fig. 4a). The modeled discharge at the lake's inflow and outflow in 2018 shows no significant difference from the average conditions (SMHI, 2020a). The Global Network of Isotopes in Precipitation (GNIP) station closest to our study area, Bredkälen, is located 100 km north of Lake Locknesjön at 400 and provides monthly measurements of δ18O for the period of 1975–1980, but no measurements of δD (Fig. 4b; IAEA/WMO, 2019).
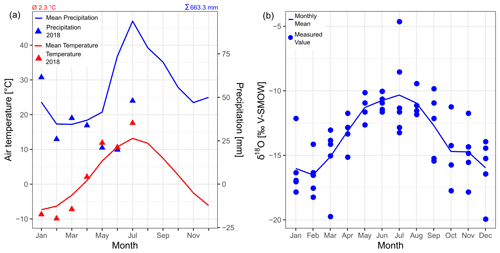
Figure 4(a) Monthly climatology of air temperature and precipitation in the study area from the meteorological station Mörsil for the period of 1975–2005 (data from SMHI, 2020b). The values of the year 2018 until the time of sampling (July) are indicated by the triangles. The average annual air temperature and the average annual precipitation are given at the top of the plot. (b) δ18O in precipitation at the GNIP station Bredkälen for the period of 1975–1980 (data from IAEA/WMO, 2019). See Fig. 2 for the locations of the stations.
2.2 Field sampling
In July 2018, a diving team collected samples of water, surface sediments and living Chara from the lake. Samples were taken at 13 locations along a transect from the western shoreline towards the center of Lake Locknesjön (Fig. 2c). Water depths at the sampling locations range from 1 to 8 m. Surface sediment samples of about 8×8 cm2 and less than 1 cm depth were taken with a small shovel and filled into sealable plastic bags. Water samples were taken directly into small glass bottles. Living Chara hispida were only found down to 7 m water depth. Up to six individuals were sampled at each location cutting off the whole algae by hand. For a comparison of different species of Chara, samples of Chara hispida and Chara aspera were collected at a depth of 0.3 m in the nearby Lake Blektjärnen. In Locknesjön only Chara hispida were found. At 5.6 m water depth in Locknesjön, water from a groundwater inflow was sampled with a syringe. Water samples were also collected at the outflow of Lake Locknesjön, and from surface water of Lake Blektjärnen (Fig. 2b). Further water samples were taken in 2013 and 2014 at depths of 0–20 m at Lake Locknesjön (11 samples), at one of its main tributaries (Musån river, 13 samples), and at its outflow (Forsaån river, 6 samples). Water samples down to 20 m depth were taken with a UWITEC gravity corer, the tributary and outflow samples were taken from the shore into small glass bottles.
2.3 Carbonate sample preparation
The following biogenic carbonate components were obtained from the samples and prepared for stable isotope measurements: encrustations around the internodes and branchlets of Chara, valves of Pisidium, and valves of juvenile and adult ostracods (see examples in Fig. 2).
Chara species were determined based on the number of cells of the main stem and the differences between primary and secondary cells (Caisova and Gabka, 2009). A further indicator was the relation between the number of cells in the main stem and the number of branchlets in a whorl. Since the whorls of branchlets are not preserved in the sediments (only branchlet fragments), this was only useful for the determination of living algae. Internode and branchlet samples were cut off from different parts of the living Chara and dried. The organic matter was removed from the calcite encrustations by putting the samples in 10 % hydrogen peroxide (H2O2) for at least 48 h. They were then cleaned with a thin paintbrush to remove fine-grained calcite sticking to the encrustations. The removed fine calcite was also kept for isotope measurements in order to test if it is composed of inorganically precipitated calcite, or if it originated from the encrustations themselves.
Sediment samples were wet sieved to obtain residual size fractions containing specific biogenic carbonates (>400 and >200 µm). Valves of ostracods and Pisidium and encrustations of Chara were picked out under a binocular microscope. Based on their size, the latter were assumed to be fragments of internodes. Ostracod species and Pisidium were identified based on the characteristics of their valves, following Meisch (2000) for ostracod identification. The dominant ostracod species were Candona candida and Candona neglecta. Other species (Cytherissa lacustris, Candona caudata, Cypridopsis vidua, and Limnocythere inopinata) were present in insufficient numbers for isotope measurements. Adult (A) and juvenile instars (A-1 to A-4) of ostracods were separated. A number of living specimens of ostracods and Pisidium were found in the sediment samples. These were briefly boiled so that organic matter could be easily removed from the valves. All valves were rinsed with ethanol and air dried (von Grafenstein et al., 1992; Mischke et al., 2007). In the following, the carbonates obtained from living organisms are referred to as living samples, the carbonates deposited in surface sediments are referred to as subfossil samples.
In order to obtain sufficient sample weights for isotope measurements, several ostracod valves from the same sampling location, species and instar were combined. Valves of living and subfossil specimens were kept separate. The required numbers of valves in a sample were 2 for adults, 5 for A-1, 8 for A-2, and 15–25 for A-3 and A-4. If the sample weights were not sufficient due to few available valves, successive instars were combined for some locations, leading to combinations of A-2 and A-3, or A-3 and A-4 depending on the sample. Pisidium samples were composed of individual valves. Chara samples were composed of single stalks from an internode or branchlet.
2.4 Stable isotope measurements
For this study, 49 water samples and 271 carbonate samples were measured. Measurements of δ18O and δD of water samples were conducted at the Laboratoire des Sciences du Climat et de l'Environnement (LSCE), Gif-sur-Yvette, France. The δ18O was measured with a Finnigan MAT252 isotope ratio mass spectrometer with a precision of ±0.05 ‰ (2 standard deviations). The δD was measured with a Picarro Analyzer (Cavity Ring-Down Spectroscopy) with a precision of ±0.7 ‰ (1 standard deviation). The results are given relative to the V-SMOW standard.
Carbonate δ18O and δ13C measurements were carried out at MARUM, Bremen, Germany, using a ThermoFisher Scientific 253plus gas isotope ratio mass spectrometer with a Kiel IV automated carbonate preparation device. The standard deviation of the reference standards over the measurement period was 0.06 ‰ for δ18O and 0.03 ‰ for δ13C. The results are given relative to the V-PDB standard. Some sample weights were <20 µg, resulting in low signal intensities (1800–3000 mV for mass 44). A control standard (ground limestone) showed no deviation over this signal range from the expected values within the above errors. Of the samples 29 had a signal intensity <1800 mV and were excluded from further analyses. One major outlier in δ-values was identified in a sample of living Candona neglecta, instar A-2, taken at 5 m water depth (sampling location 5) and also excluded from further analysis. Its δ13C value of −9.63 ‰ was below the outer limit of the interquartile range of all ostracod δ13C values.
2.5 Data analysis
Statistical analyses were performed using R v. 3.6.1 (R Core Team, 2018) and the statistical package PAST v. 3.25 (Hammer et al., 2001). All data sets of individual species were tested for normal distribution using the Shapiro-Wilk test (Shapiro and Wilk, 1965). For normally distributed data, correlations were calculated using the Pearson method (Pearson, 1895), and t-tests assuming unequal variances were calculated to compare different sample sets. For samples lacking normal distribution, this comparison was done using the Mann-Whitney test (Mann and Whitney, 1947) and the Spearman method was used to calculate correlations (Spearman, 1904).
Assuming calcite precipitation in isotopic equilibrium with the water, theoretical water temperatures were calculated from the mean, minimum and maximum δ18O values of water and calcite (corrected for species-specific vital offsets). We used the empirical equation by Kim and O'Neil (1997), with δ18Ocalcite(SMOW)−δ18Owater(SMOW) as an approximation of the fractionation factor, and the conversion of calcite δ18O values from the PDB to the SMOW scale according to Coplen et al. (1983). For the aragonitic Pisidium valves, we assume a temperature dependence of the δ18O like for inorganic calcite (Grossman and Ku, 1986; Kim et al., 2007), and the species-specific offset correction accounts for the offset between the valve carbonate and inorganic calcite.
This section presents the results of our stable isotope measurements of water and biogenic carbonates, and explains the influences on their isotopic composition. The subsequent Discussion (Sect. 4) then evaluates how far these influences can be constrained through our “snapshot” approach by comparing different species, and which paleoclimate information can potentially be obtained from the carbonates.
3.1 Isotopic composition of water
The δ18O of monthly precipitation at the Bredkälen GNIP station averaged over the period of 1975–1980 closely follows the annual cycle of air temperature. Values of individual months range between −4.9 ‰ and −19.9 ‰ (Fig. 4b). Although the precipitation isotope time series and the local water isotope data were collected during different periods, some relationships can be inferred from the distributions of the isotope values.
The δD and δ18O of water from the tributary of Lake Locknesjön plot close to the Global Meteoric Water Line (GMWL). The seasonal range of δ18O in the tributary (−13.11 ‰ to −11.57 ‰) is reduced compared to the range in precipitation (Fig. 5b), which indicates a substantial groundwater contribution to the stream flow. Still, a clear shift to higher δ18O and δD values in the tributary shows a marked influence of precipitation on river supply to the lake during the warm season. The isotopic values of the groundwater inflow are more negative than all values of the tributary, and the δ18O is close to the amount-weighted mean of precipitation for 1976–1980 (−13.5 ‰), which as expected indicates a negligible influence of evaporation.
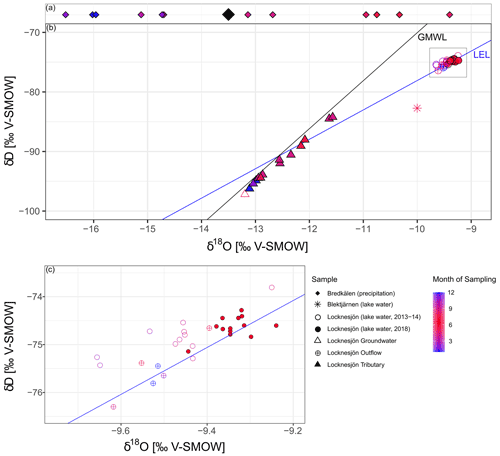
Figure 5Isotopic composition of water in the study area. The symbols represent different locations and years of sampling. The color scale indicates the month of sampling, with blue values representing the cold period and red values representing the warm period of the year. (a) Average monthly δ18O of atmospheric precipitation for the period of 1975–1980 at the GNIP station Bredkälen (data from IAEA/WMO, 2019, note that δD is not available for this station). The large black diamond shows the mean δ18O for 1976–1980 weighted by the amount of precipitation. (b) δD and δ18O of lake water (sampled in 2013–2014 and 2018), the groundwater inflow (2018), and water in the tributary (2013–2014) and outflow (2013–2014) of Lake Locknesjön, and lake water from Lake Blektjärnen (2018). The Global Meteoric Water Line (GMWL), is plotted in black, and a local evaporation line (LEL; ) estimated from the isotopic composition of Locknesjön lake water in blue. (c) Zoom on the lake water isotope data from Lake Locknesjön.
Water samples from the lake and its outflow, on the other hand, show effects of evaporative enrichment in the heavy isotopes compared to groundwater and the tributary by about 3 ‰ for δ18O and 17 ‰ for δD. This enrichment is seen in samples from all investigated years and in all seasons. The inferred a local evaporation line with a slope of 4.9 and an intercept of 29 ‰ is typical for lakes with evaporative water loss (Craig and Gordon, 1965; Craig, 1961).
The difference between lake water samples taken in winter and summer is small, with values ranging from −9.66 ‰ to −9.24 ‰ for δ18O and from −75.4 ‰ to 73.6 ‰ for δD. The exceptional meteorological conditions during the summer of 2018 did not lead to a significant effect on the δ18O of lake water, e.g., through increased evaporation. The δ18O of water sampled in 2018 is only about 0.1 ‰ higher than in other warm season water samples (Fig. 5c). A potential effect of the dry and warm summer conditions 2018 is either masked through the mixing of lake water, or could arrive in the lake with a delay. The small seasonal and interannual changes of the isotopic composition can be explained by the relatively long water residence time of over 10 years in Lake Locknesjön, caused by its large volume in relation to its limited catchment size (Fig. 2b). Interannual variations in the isotopic composition of precipitation are only reflected in lake water δ18O if they last longer than the lake water residence time (von Grafenstein and Labuhn, 2021).
Variations in δ18O and δD between 0 and 20 m water depth in April 2014 do not exceed analytical uncertainty, with a range of 0.02 ‰ for δ18O and 0.3 ‰ for δD. The δ18O and δD vary only minimally between 1 and 8 m water depth in July 2018 and do not show any trends with depth. The ranges of 0.17 ‰ for δ18O and 0.8 ‰ for δD are only slightly larger than the measurement error, indicating that the lake water is well mixed above the thermocline. Lake Locknesjön displays a distinct summer stratification with a thermocline generally below 10 m (Fig. 3; Eklund, 1998). Although only one summer temperature profile per year is available, it can be concluded that the rapid transition from ice-covered conditions in May to maximum air temperature in July, together with the effects of strong winds, leads to a rather deep and well-mixed summer epilimnion.
Lake Blektjärnen shows a 0.66 ‰ lower δ18O and a 13.1 ‰ lower δD compared to Lake Locknesjön in the summer of 2018. These measurements are in agreement with previously published isotope data from Lake Blektjärnen (Andersson et al., 2010).
3.2 Isotopic composition of biogenic carbonates
3.2.1 Pisidium
The δ18O values of the aragonite valves of Pisidium samples range between −8.83 ‰ and −7.38 ‰ and the δ13C values lie between −7.20 ‰ and −4.00 ‰ (Table 1, Fig. 6a). The relatively narrow ranges can be explained by the accumulation of shell carbonate throughout their lifetime of up to 3 years (Holopainen and Jónasson, 1989). This averages out seasonal and interannual variations in the water isotopic composition and water temperature. There is no significant difference in isotope values between the valves of living specimens sampled in 2018 and the subfossil valves (i.e., which were formed and deposited during previous years), possibly because their time spans of carbonate accumulation overlap. The mean δ18O is the same, and the mean δ13C differs by 0.19 ‰.
Table 1Isotopic composition of specific biogenic carbonates from living organisms and from subfossil samples in surface sediments of Lake Locknesjön. Mean, standard deviation (SD), minimum, maximum and range of δ18O and δ13C values, as well as the number of samples are presented for Chara hispida, Candona candida, Candona neglecta and Pisidium sp. All values are reported in ‰ relative to V-PDB. Note that these are the measured values without a correction of vital offsets. The results of individual sample measurements are available in the Pangaea database, see Sect. “Data availability”.
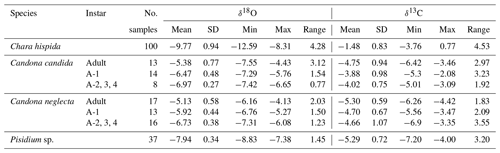
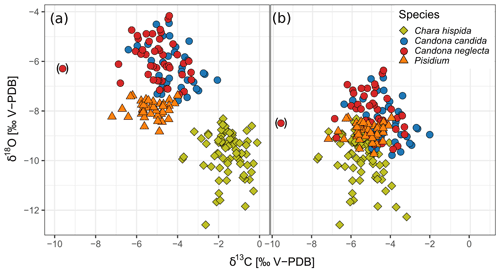
Figure 6Isotopic composition of specific biogenic carbonate samples collected from living organisms and from subfossil remains in surface sediments of Lake Locknesjön. (a) δ18O and δ13C of calcite encrustations from Chara hispida and of valves from Candona candida, Candona neglecta and Pisidium. (b) The same data with published vital offsets corrected for: δ13C Chara hispida: −4 ‰ (value from Hammarlund and Keen, 1994), δ18O ostracods: −2.2 ‰, δ18O Pisidium: −0.9 ‰ (values from von Grafenstein et al., 1999b). The data point marked in brackets was identified as a major outlier and left out from further analysis and figures.
The previously reported vital offset on the oxygen isotopic composition of Pisidium valves of +0.9 ‰ (von Grafenstein et al., 1999b) is confirmed by this study. After correction for this offset, the Pisidium δ18O falls close to expected values in equilibrium with the lake water, as observed in other studies of mollusk shells (Apolinarska et al., 2016).
3.2.2 Chara
Samples of calcite encrustations from Chara hispida in Lake Locknesjön show the largest range in isotope values compared to other species (Table 1). Chara can be annual or perennial (Martin et al., 2003; Pełechaty et al., 2013). It is not known whether overwintering occurs in Locknesjön, but different parts of the algae are formed during different times of the growing season, or possibly during different years. The growth of Chara is apical, i.e., calcification at a given point in time occurs only around these apices (Coletta et al., 2001). Each analyzed sample consisted of only one internode or branchlet, which means that environmental variations within the growing season or between different years are not averaged in individual samples. The samples represent only the short period of time when the respective part of the Chara grew. There is no difference between the isotopic compositions of encrustations from living Chara hispida and subfossil encrustations collected from surface sediments (Fig. 7a), indicating that the environmental influences on the δ18O and δ13C of the encrustations were similar over the past few years. The possible perennial growth also means that there could be an overlap in the years of growth represented in living and subfossil samples.
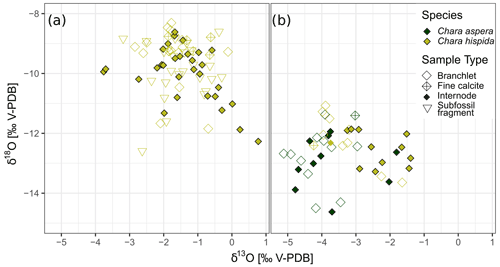
Figure 7Isotopic composition of calcite encrustations from different types of Chara samples. Internodes, branchlets and fine calcite were collected from living Chara, “subfossil fragment” refers to fragments of Chara encrustations taken from surface sediments. (a) δ18O and δ13C of Chara hispida samples from Lake Locknesjön. (b) δ18O and δ13C of Chara hispida and Chara aspera samples from Lake Blektjärnen.
Chara growth is restricted to the time of photosynthetic activity, i.e., the summer months in our study area (Hammarlund et al., 2002). The δ13C is driven by fractionation during photosynthesis, which preferentially incorporates 12C into the organic matter. Consequently, DIC in the surrounding water and, in turn, the calcitic encrustations are enriched in 13C (McConnaughey and Falk, 1991). This process is reinforced by proton pumping, leading to additional 13C enrichment in the encrustations (Hammarlund et al., 1997). After correcting Chara hispida δ13C for the vital offset of 4 ‰, the values are largely similar to the δ13C values of other biogenic carbonate samples from Lake Locknesjön (Fig. 6b). The magnitude of carbon isotope fractionation in Chara encrustations observed here is therefore the same as in previous studies (Hammarlund and Keen, 1994; von Grafenstein et al., 2000).
The mean δ18O of the Chara hispida encrustations in Lake Locknesjön (−9.77 ‰) is close to that of inorganic calcite that would be precipitated in isotopic equilibrium with lake water, which has a mean δ18O of −9.53 ‰. Other studies, on the contrary, have found significant offsets from equilibrium due to kinetic effects during intense photosynthesis associated with fast calcite precipitation (Apolinarska et al., 2016; Andrews et al., 2004).
Fine calcite δ18O values fall within the range of δ18O of the encrustations, but their mean is higher than the mean of the encrustations. The observed δ13C values are similar for fine calcite and encrustations. This indicates that the fine calcite, which also makes up the matrix of the carbonate-rich sediments of Lake Locknesjön, is composed of disaggregated Chara hispida encrustations. Allochthonous carbonates would be expected to have a higher δ13C than the fine calcite, close to the δ13C of bedrock in the catchment area (around −0.5 ‰, see Sect. 4.3). Other autochthonous carbonates which are not influenced by fractionation due to photosynthesis have a lower δ13C.
The two Chara species sampled in Lake Blektjärnen differ in the isotopic composition of their encrustations (Fig. 7b). The average δ13C in Chara hispida is significantly higher (1.04 ‰) than in Chara aspera. Such differences can be caused by habitat conditions, such as stand density and by metabolic effects (Pronin et al., 2018; Coletta et al., 2001). The δ13C is driven by photosynthesis. The Chara hispida in Blektjärnen grew in dense swards, which limits the water exchange and therefore the removal of DIC enriched in 13C due to photosynthesis, leading to a corresponding enrichment of 13C of the encrustations (Coletta et al., 2001). The average difference in δ18O (0.46 ‰) is not significant. The δ18O of Chara is driven by water δ18O, which leads to a corresponding species-independent δ18O of encrustations formed in the same water.
Internodes and branchlets show small differences in their mean isotope values, but these differences are not consistent between species and lakes. In living Chara hispida from Lake Locknesjön, the mean δ18O of branchlets is 0.56 ‰ higher and the mean δ13C is not significantly different from internodes. In samples from Lake Blektjärnen, internodes and branchlets are not significantly different in the δ18O and δ13C of Chara aspera, nor in the δ18O of Chara hispida, whereas the δ13C of Chara hispida is higher on average in the internodes. Consequently, it does not seem to be necessary to distinguish between these parts of the alga in isotopic analyses of the encrustations.
3.2.3 Ostracods
The δ18O values of ostracod valves range between −7.55 ‰ and −4.13 ‰, and the δ13C values range between −6.90 ‰ and −2.08 ‰ (Table 1). There is no significant difference between the δ18O values of Candona candida and C. neglecta. The difference in δ13C is statistically significant, but the mean δ13C is only 0.67 ‰ lower for C. neglecta. This general similarity in the isotopic composition of the two species is in agreement with previous studies (von Grafenstein et al., 1999b). With known vital offsets subtracted (2.2 ‰ for both species; von Grafenstein et al., 1999b), the δ18O values of both species are close to the values of other biogenic carbonates from Lake Locknesjön (Fig. 6b).
Comparing different developmental stages of the ostracods, there is a clear decrease in the average δ18O values from adult valves to A-1 to A-2, A-3 and A-4 instars, although the values of individual samples partly overlap (Fig. 8). The timing of the molting of ostracods is controlled by temperature. In southern Germany, it has been observed that co-existing Candona instars A-4 to A-2 form their valves during early to mid summer, A-1 at the onset of autumn cooling and adults between late autumn and early spring (von Grafenstein et al., 1999b). Consequently, seasonal changes in water temperature and water δ18O have an influence on the δ18O of the valves formed during different times of the year. Higher water temperatures lead to reduced fractionation and a decrease in the δ18O of the precipitated carbonate.
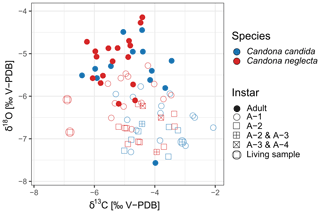
Figure 8Isotopic composition (δ18O and δ13C) of the valves from two species of ostracods, Candona candida (blue) and Candona neglecta (red), taken from living specimens (encircled symbols) and subfossil samples in the surface sediments of Lake Locknesjön. The symbols represent the instars. See Sect. 2.3 for explanation of the combinations of different instars.
Juvenile ostracods form their valves when the lake is thermally stratified during the warm season. As the instars A-2 to A-4 form at the time of maximum water temperature, the mean δ18O of their valves is significantly lower than in A-1 and adult samples. There is no significant difference between A-2, A-3 and A-4 instars. The average δ18O of A-1 samples is higher than in younger instars because A-1 instars develop when the water temperature starts to decrease in autumn. Moreover, by the end of the summer the water in the epilimnion is more enriched in 18O by evaporation compared to the time when the younger instars calcify their valves, leading to a further increase in the δ18O of A-1 valves. The valves of adult ostracods start forming in late autumn. When overturning occurs in the lake, stratification ceases and the isotopically enriched surface water is mixed with deep water with a lower δ18O. Nevertheless, adult ostracods have significantly higher δ18O values on average compared to juvenile instars, because their valves are formed at the lowest water temperature. However, the lowest δ18O value of all ostracod samples was recorded in an adult specimen from 1 m water depth.
The patterns of average δ13C values of ostracod valves from different instars are less clear compared to δ18O. The average δ13C of all juvenile instars are generally similar, whereas the average δ13C of adult ostracods is slightly lower. The highest values, however, were observed in samples of A-1 instars. The δ13C of ostracod valves depends on the carbon isotopic composition of DIC in the surrounding water. The higher δ13C of juvenile ostracods suggests that DIC is slightly enriched in 13C as a result of Chara photosynthesis and other primary productivity during the summer when juveniles calcify their valves. When adult valves are formed, the lake is no longer stratified and the overturning brings DIC with a lower δ13C from depths below the summer thermocline to the surface.
4.1 Climate signal in the δ18O of lake water
In order to interpret the isotopic oxygen composition of lacustrine biogenic carbonates, it is important to understand the causes of variations in the δ18O of the oxygen source, i.e., the lake water. Generally, lake water δ18O depends on the water balance as the δ18O of the water input (atmospheric precipitation, potentially modified though processes in the catchment) can be increased through evaporation in the lake (Mayr et al., 2007; von Grafenstein and Labuhn, 2021). The measured values of river and groundwater inflow into Lake Locknesjön show that seasonal variations in the isotopic composition of atmospheric precipitation are smoothed through groundwater supply and long lake water residence time. The difference between tributary and lake water indicates evaporative enrichment in 18O. In the absence of long-term monitoring, it is not possible to disentangle the relative importance of precipitation and evaporative enrichment for the δ18O of the lake water. However, since both factors increase with increasing temperature, lake water δ18O should contain a signal of multiannual temperature variability. As shown by our lake water δ18O and water temperature profiles (Figs. 3a, 9), the lake water is well mixed down to a depth of about 8 m, i.e., water depth is not a decisive factor across the range of sampled depths.
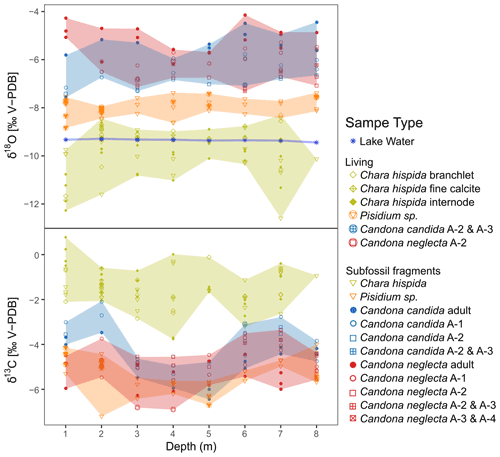
Figure 9Interspecies and intraspecies variations in the isotopic composition (δ18O and δ13C) of biogenic carbonates sampled along a transect in Lake Locknesjön at water depths from 1 to 8 m. The colors indicate the species, and the shaded areas represent the ranges of their δ-values. The symbols represent different sample types (as in Fig. 7 for Chara hispida and as in Fig. 8 for ostracods). The blue asterisks represent δ18O of water samples taken at the same locations. The values of lake water are reported in ‰ relative to V-SMOW.
4.2 Influence of water depth on the isotopic composition of carbonates
None of the investigated species show any significant trends in average δ18O or δ13C, or in the range of isotope values, along the transect from 1 to 8 m water depth (Fig. 9). The largest temperature changes can be expected in shallow water, which would lead to larger ranges of δ18O values at the shallowest sampling depths. However, since no systematic changes were observed in the carbonate isotopic compositions or in the δ18O of the lake water, we conclude that the calcification conditions and the isotopic compositions of the carbon and oxygen sources did not significantly vary within the sampled range of water depths. The effects of water temperature changes and evaporative enrichment in 18O of lake water are the same, and the water at 8 m depth appears to be well mixed and clearly above the summer thermocline for the time span represented in the living and subfossil samples.
4.3 Causes of interspecies differences in the isotopic composition of carbonates
Vital offsets are the major cause of differences in the oxygen isotopic composition between species (Table 1, Fig. 6a). With these known and constant offsets corrected for, the mean δ18O values of all species analyzed in our study plot closer to each other and their ranges show larger overlaps (Fig. 6b). The remaining differences can be explained by environmental influences, which vary with the timing and length of the respective calcification periods. The mean values of each species depend on the time of the year when the carbonate is precipitated, involving potentially large differences in water temperature. The range of values of a species depends on the length of the calcification period of each sample, a longer period smoothing seasonal and interannual variability.
The mean δ18O value of Chara hispida encrustations lies clearly below the mean δ18O values of all other species. The mean δ18O values of Pisidium and juvenile ostracods are higher, and adult ostracods show the highest mean. The timing of calcification in Chara hispida is limited to periods of maximum photosynthetic activity in summer, which can last only a few weeks. These periods are likely also the periods of highest temperatures, which would explain the low δ18O of Chara hispida encrustations compared to other carbonates. Seasonal temperature variations also cause the differences observed between adult and juvenile ostracods (see Sect. 3.2.3). As juvenile ostracods precipitate calcite mainly during the summer months, their values overlap with values of Chara hispida encrustations. The calcification period of Pisidium is the longest of all studied organisms and lasts throughout the ice-free period from May to November (Andersson et al., 2010). Most calcification occurs during summer, whereas their growth is substantially suppressed during winter (von Grafenstein et al., 1999b). The mean δ18O value of Pisidium is slightly lower than that observed for juvenile ostracods A-2,A-3 and A-4, although the former are exposed to lower average water temperatures during their calcification period and thus a higher carbonate δ18O would be expected due to temperature-dependent fractionation. The lower Pisidium δ18O values may reflect the influence of relatively 18O-depleted snow-melt supply to the lake in spring and from mixing of surface water with 18O-depleted bottom water in autumn, processes that juvenile ostracods are not exposed to.
The range of δ18O is smallest for Pisidium (1.45 ‰) and largest for Chara hispida (4.28 ‰). Chara can be perennial, but the samples measured in this study, encrustations from single internodes or branchlets, were precipitated at different times as the Chara grew, i.e., individual samples may represent different times within a growing season and possibly different years, hence explaining their larger isotopic range. The average lifetime of Pisidium of 3 years, together with their long annual calcification period probably explain their relatively narrow range of δ18O.
Overall, seasonal water temperature changes seem to be the dominant cause of differences in the δ18O of the investigated biogenic carbonates (after vital offset correction). The δ18O of the lake water, on the contrary, does not have a strong influence due to the small seasonal and interannual variability around the mean of −9.53 ‰ during the years of observation.
The carbon isotopic composition of lacustrine biogenic carbonates depends primarily on the δ13C of DIC in lake water. Observations of δ13C of DIC in groundwater or lake water are not available for Lake Locknesjön, but some general assumptions can be made. The δ13C of groundwater DIC is dependent on the open or closed system conditions, which determine the relative contributions from bedrock carbonates and soil air CO2 (Clark and Fritz, 1997). The δ13C of carbonate bedrock in the catchment area, i.e., resurge deposits in the Lockne Crater and post-impact sediments, is close to −0.5 ‰ (Ormö et al., 2010). The δ13C of microbially respired soil air CO2 reflects that of the local vegetation, which can be estimated to be around −25 ‰ based on tree ring cellulose data from the study area (unpublished data). It is increased by ∼4 ‰ through fractionation by diffusion as CO2 outgasses along the pCO2 gradient between the soil and the atmosphere (Cerling et al., 1991). As a result of the well-mixed character of groundwater recharging the lake as evidenced by water isotope monitoring data (Fig. 5), likely reflecting a relatively long residence time, seasonal variations in δ13C of groundwater DIC are probably small or absent.
On the contrary, DIC in lake water usually shows substantial seasonal variations, increasing in the epilimnion during the stratified warm period and decreasing to the lake-wide mean δ13C during the holomixis (Decrouy et al., 2011a; von Grafenstein et al., 1999b). In Lake Locknesjön, warm season increases in δ13C are most likely mainly induced by the photosynthetic activity of Chara (cf. Sect. 3.2.2), which can be assumed to also raise the mean δ13C of lake water DIC compared to groundwater.
Chara hispida encrustations exhibit a significantly higher δ13C than ostracod and Pisidium valves (Fig. 6a). While the δ13C of Pisidium and ostracod calcite reflects the δ13C of DIC, the δ13C of Chara hispida encrustations is influenced by fractionation between DIC and the organic matter produced during photosynthesis. Juvenile Candona show a slightly higher δ13C than adult Candona and Pisidium, which suggests that DIC is slightly enriched in 13C by Chara photosynthesis in summer when juvenile ostracods calcify their valves (Fig. 8). However, this effect on juvenile ostracods is much weaker than on the carbonate precipitated at the site of photosynthesis, i.e., in the Chara hispida encrustations. No other effects related to seasonal changes in δ13C of DIC or lake water temperature on the δ13C of biogenic carbonates can be inferred from our data.
Although probably minor in the carbonate-rich and therefore well-buffered system of Lake Locknesjön, seasonal variations in lake water pH may contribute to the observed interspecies differences in δ13C and δ18O. The pH measured at depths above 8 m ranges from 8.05 to 8.33, the maximum occurring in August. In cultured foraminifera, a slight lowering of δ18O and δ13C has been observed in response to increasing pH (Spero et al., 1997). Field observations of sediment trap calcite and lake water pH confirm this relationship (Teranes et al., 1999). However, further measurements of lake water pH and contemporaneously precipitated calcite would be needed to investigate the influence of pH variations on biogenic carbonates in Lake Locknesjön.
An additional factor that could play a role for interspecies differences is the microhabitat of the respective species. The most prominent spatial change in microhabitat at our sampling sites is the disappearance of Chara below 7 m depth. No influence of this change is evident on the carbonate isotopic compositions of the other species (Fig. 9). Furthermore, groundwater inflow may lead to local decreases in temperature and δ18O of the lake water (cf. Sect. 2.1), but such inflows were not identified in the direct vicinity of the sampling sites, and no local decreases in carbonate δ18O related to groundwater inflow were observed. On the contrary, the example of Lake Blektjärnen shows that the δ13C of different Chara species can be influenced by their microhabitat, and that consequently a distinction at the species level is necessary for the interpretation of isotope records.
4.4 Uncertainties in water temperature reconstruction
Water temperature measurements contemporaneous with the formation of the analyzed biogenic carbonate samples are not available. Nevertheless, the range of observed seasonal water temperature changes in previous years (Fig. 3) is consistent with the reconstructed water temperature ranges based on mean lake water δ18O and mean carbonates δ18O for the respective species (Fig. 10). The highest reconstructed temperatures were obtained from Chara hispida calcifying in summer and the lowest temperatures from adult Candona calcifying in winter. This observation confirms that the differences in carbonate δ18O between species (after correcting for vital offsets) are driven by varying water temperatures during their respective periods of calcification.
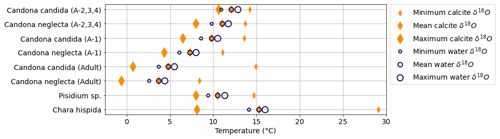
Figure 10Theoretical temperature calculated from water and carbonate δ18O, assuming the carbonate was precipitated in isotopic equilibrium with the water (according to Kim and O'Neil, 1997). Temperatures are calculated using the mean water δ18O and the range of measured carbonate δ18O values (minimum, mean and maximum) for each species and instar (orange diamonds), as well as using the mean carbonate δ18O of each species and instar and the range of observed water δ18O values (dark blue circles). The measured carbonate δ18O values have been corrected for known vital offsets.
For a given carbonate δ18O value, reconstructed temperatures calculated based on minimum and maximum observed lake water δ18O values yield a range of 1.9 ∘C (Fig. 10). The variability in δ18O between individual carbonate samples, on the contrary, has a larger influence on the reconstructed temperature than the variability in lake water δ18O. Temperatures calculated based on the mean δ18O of lake water samples and the minimum and maximum values of carbonates from the respective species yield temperature ranges of 3.6 ∘C for juvenile Candona candida, >5 ∘C for all other ostracod instars, and 21.0 ∘C for Chara hispida (Fig. 10). The lowest reconstructed water temperatures, near 0 ∘C for adult Candona, match the lowest observed lake water temperatures at Lake Locknesjön. The maximum reconstructed water temperature of 29 ∘C for Chara hispida, however, is rarely reached in maximum daily air temperatures in the study area, and therefore impossible to occur in the open water column.
A part of these large ranges in reconstructed temperatures is probably related to temporary variations in shallow water temperature that greatly exceed those indicated by the observed annual minimum and maximum values (Fig. 3). In particular, calcification of Chara encrustations may take place in secluded microhabitats at anomalously high water temperatures in summer, potentially also involving oxygen-isotope disequilibrium effects (Pentecost et al., 2006). In addition, deviations from normal seasonal calcification patterns may occur. For example, summer generations of adult ostracods have been observed, likely as a consequence of thunderstorms, leading to temporary cooling of surface water (von Grafenstein et al., 1999b).
4.5 Implications for paleoclimate studies
Our data exhibit relatively large isotopic variability within one species (Fig. 6a), based on samples collected either from living organisms or from their subfossil remains in surface sediments, i.e., representing calcification during a time interval of a few years in the recent past. Analytical techniques today allow the analysis of small quantities of carbonate corresponding to, e.g., a few ostracod valves or a single stalk of a Chara encrustation. However, our results demonstrate that the range of reconstructed temperatures from such individual samples is large (Fig. 10), whereas the temperature reconstructed from an average of all samples per species/instar is in good agreement with observations. Consequently, it must be assured that isotopic records obtained from lacustrine biogenic carbonates for the study of past climate changes yield representative values for the time span covered by each analyzed sediment interval, through either (i) sufficiently large numbers of individual samples, or (ii) homogenized samples incorporating sufficiently large numbers of specimens.
The observed differences between our investigated species highlight the importance of performing isotopic measurements on well-identified remains of specific biogenic carbonate components in lake sediments rather than on bulk carbonate. Isotope records obtained on bulk sediment may reflect changes in the relative contributions of carbonate from different species and variations in detrital carbonate deposition that are not directly related to the environmental variables under study, thus adding complexity to interpretations (Hammarlund and Buchardt, 1996). For example, Chara encrustations are clearly the dominant component of the coarse fraction of the surface sediments of Lake Locknesjön at the sampled locations at water depths of up to 8 m. Changes in lake level at a coring site could lead to disappearance of Chara if wave and ice action (in shallow water) or lack of light (in deep water) hinder their growth, which would shift bulk carbonate δ18O to higher values. If bulk carbonate isotope records are complemented with contemporary monitoring data and proper characterization of the sediment composition, their cautious interpretation may still yield important information. However, the isotopic analysis of specific carbonate components, preferably involving multiple taxa, can provide more detailed insight into past changes in climate because of their differences in the timing of calcification, and consequently water temperature and water δ18O.
Past changes in water depth, caused by sediment accumulation and compaction or hydrological changes, may also influence isotopic records by affecting bottom water temperatures independently of other changes in climate. However, at Lake Locknesjön, potential changes in water depth in the range of 1 to 8 m do not seem to exert any significant influence on the isotopic composition of biogenic carbonates under modern conditions. We can expect that isotope proxy records from sediment cores at this site remain unaffected by possible water depth changes in this range in the past, as long as the sediment surface remains above the summer thermocline.
This study presents a modern snapshot of the variability of the carbon and oxygen isotopic compositions of lacustrine biogenic carbonates from living organism and from their subfossil remains in the surface sediments of Lake Locknesjön, which allows us to draw the following conclusions.
-
We constrained the controls on the isotopic composition of biogenic carbonates in Lake Locknesjön. The large differences between species are mainly caused by species-specific vital offsets. Additional differences are caused by the timing and length of the calcification periods of the respective species, being exposed to seasonally changing water temperatures. In contrast, seasonal and interannual variations in lake water δ18O are small, at least over the time span of observation (2013–2014 and 2018). The influence of changing lake water δ18O on carbonate δ18O is outweighed by the opposing effect of changing water temperature (see Fig. 1 for different influences of temperature on carbonate δ18O).
-
Based on the understanding of these environmental controls, it is possible to estimate seasonal water temperature changes from the δ18O of lake water and of specific biogenic carbonates, provided that components formed during different seasons but sampled from the same sediment layer are analyzed individually. This approach is particularly applicable for groundwater-fed lakes with long residence times like Lake Locknesjön, where lake water δ18O varies within a relatively narrow range. Under such conditions, seasonal changes in lake water temperature are clearly reflected in the δ18O of multiple biogenic carbonates through the temperature effect on isotope fractionation between water and carbonate. Water temperature changes could also exert the dominant control on carbonate δ18O on longer (decadal to millennial) time scales, where significant changes in air temperature can be expected. However, in that case additional factors must be considered, such as the effects of changes in δ18O of atmospheric precipitation brought about by related or independent climate dynamics, or potential variations in evaporative enrichment in the δ18O of the lake water caused by a changing ratio of evaporation to inflow.
-
Our results have implications for the design of subsampling strategies aiming at reconstructions of past changes in environment and climate based on isotopic analyses of sediment cores. The intraspecies variability in δ18O and δ13C of biogenic carbonates highlights that care must be taken to obtain representative subsamples of a species for each time interval, especially if working with shallow water environments where water temperature can change rapidly over a short time. The interspecies variability observed in Lake Locknesjön adds to the growing body of evidence demonstrating the importance of performing measurements on specific carbonate components of lake sediments rather than on bulk carbonate. Sampling strategies involving multiple specific biogenic carbonate components are likely to produce more detailed and comprehensive environmental reconstructions, including records of seasonal water temperature variability.
Water isotope data obtained in this study are available at https://doi.org/10.1594/PANGAEA.942628 (Labuhn et al., 2022a). Carbonate isotope data are available at https://doi.org/10.1594/PANGAEA.942627 (Labuhn et al., 2022b).
A video showing the movement of sediment on the lake floor at a groundwater inflow is available at https://doi.org/10.5446/56815 (Labuhn et al., 2021).
IL and UvG designed the study and coordinated the field work. Samples were prepared by FT and UvG. Water isotope measurements were performed by BM, carbonate isotope measurements by HK. FT and IL created the figures. IL and FT wrote the paper, with contributions from DH, UvG and HK. All co-authors contributed to the interpretation and discussion of the results. DH and UvG were principal investigators of the project within which this study was conducted.
The contact author has declared that neither they nor their co-authors have any competing interests.
Publisher’s note: Copernicus Publications remains neutral with regard to jurisdictional claims in published maps and institutional affiliations.
This study would not have been possible without the scientific diving team of the Limnological Research Station Iffeldorf, Technical University of Munich, Germany: Benedikt Beck, Matthias Galm, Jan Leidholdt and Stefan Zimmermann. The authors are grateful to Östersund Diving School for arranging the diving equipment, to Malin Bernhardsson at the County Administration Board of Jämtland for providing a boat as well as water temperature and pH data from the Triple Lakes project, to Anna Eklund at the Swedish Meteorological and Hydrological Institute for providing archived water temperature data and to Sten-Åke Jonsson for collecting water samples. Wolfgang Bevern, Birgit Meyer and Maike Steinkamp are thanked for helping with the carbonate isotope measurements.
This study was funded by the Swedish Research Council as part of the French-Swedish Common Research and Training Programme on Climate and the Environment (grant no. 349-2012-6291) and by the Central Research Development Fund of the University of Bremen (grant no. 2018/ZF08/FB8/Labuhn).
The article processing charges for this open-access publication were covered by the University of Bremen.
This paper was edited by Ny Riavo G. Voarintsoa and reviewed by Ola Kwiecien and Karina Apolinarska.
Andersson, S., Rosqvist, G., Leng, M. J., Wastegård, S., and Blaauw, M.: Late Holocene climate change in central Sweden inferred from lacustrine stable isotope data, J. Quaternary Sci., 25, 1305–1316, https://doi.org/10.1002/jqs.1415, 2010. a, b, c
Andrews, J. E., Coletta, P., Pentecost, A., Riding, R., Dennis, S., Dennis, P. F., and Spiro, B.: Equilibrium and disequilibrium stable isotope effects in modern charophyte calcites: implications for palaeoenvironmental studies, Palaeogeogr. Palaeocl., 204, 101–114, https://doi.org/10.1016/S0031-0182(03)00725-9, 2004. a, b
Apolinarska, K., Pełechaty, M., and Pronin, E.: Discrepancies between the stable isotope compositions of water, macrophyte carbonates and organics, and mollusc shells in the littoral zone of a charophyte-dominated lake (Lake Lednica, Poland), Hydrobiologia, 768, 1–17, https://doi.org/10.1007/s10750-015-2524-6, 2016. a, b, c
Beck, W. C., Grossman, E. L., and Morse, J. W.: Experimental studies of oxygen isotope fractionation in the carbonic acid system at 15∘, 25∘, and 40 ∘C, Geochim. Cosmochim. Ac., 69, 3493–3503, https://doi.org/10.1016/j.gca.2005.02.003, 2005. a
Bova, S., Rosenthal, Y., Liu, Z., Godad, S. P., and Yan, M.: Seasonal origin of the thermal maxima at the Holocene and the last interglacial, Nature, 589, 548–553, https://doi.org/10.1038/s41586-020-03155-x, 2021. a
Bright, J., Kaufman, D. S., Forester, R. M., and Dean, W. E.: A continuous 250,000 yr record of oxygen and carbon isotopes in ostracode and bulk-sediment carbonate from Bear Lake, Utah-Idaho, Quaternary Sci. Rev., 25, 2258–2270, https://doi.org/10.1016/j.quascirev.2005.12.011, 2006. a
Bäckström, A.: A Study of Impact Fracturing and Electric Resistivity Related to the Lockne Impact Structure, Sweden, in: Impact Tectonics, edited by: Koeberl, C. and Henkel, H., Impact Studies, 389–404, Springer, Berlin, Heidelberg, https://doi.org/10.1007/3-540-27548-7_15, 2005. a
Börner, N., De Baere, B., Akita, L. G., Francois, R., Jochum, K. P., Frenzel, P., Zhu, L., and Schwalb, A.: Stable isotopes and trace elements in modern ostracod shells: implications for reconstructing past environments on the Tibetan Plateau, China, J. Paleolimnol., 58, 191–211, https://doi.org/10.1007/s10933-017-9971-1, 2017. a, b
Caisova, L. and Gabka, M.: Charophytes (Characeae, Charophyta) in the Czech Republic: taxonomy, autecology and distribution, Fottea, 9, 1–43, https://doi.org/10.5507/fot.2009.001, 2009. a
Cerling, T. E., Solomon, D. K., Quade, J., and Bowman, J. R.: On the isotopic composition of carbon in soil carbon dioxide, Geochim. Cosmochim. Ac., 55, 3403–3405, https://doi.org/10.1016/0016-7037(91)90498-T, 1991. a
Chivas, A., De Deckker, P., Wang, S., and Cali, J.: Oxygen-isotope systematics of the nektic ostracod australocypris robusta, Geoph. Monog. Series, 131, 301–313, https://doi.org/10.1029/131GM16, 2002. a
Clark, I. D. and Fritz, P.: Environmental Isotopes in Hydrogeology, Lewis Publishers, Boca Raton, London, New York, https://doi.org/10.1007/s00254-002-0679-8, 1997. a, b
Coletta, P., Pentecost, A., and Spiro, B.: Stable isotopes in charophyte incrustations: relationships with climate and water chemistry, Palaeogeogr. Palaeocl., 173, 9–19, https://doi.org/10.1016/S0031-0182(01)00305-4, 2001. a, b, c
Coplen, T. B., Kendall, C., and Hopple, J.: Comparison of stable isotope reference samples, Nature, 302, 236–238, https://doi.org/10.1038/302236a0, 1983. a
Craig, H.: Isotopic Variations in Meteoric Waters, Science, 133, 1702–1703, https://doi.org/10.1126/science.133.3465.1702, 1961. a
Craig, H. and Gordon, L. I.: Deuterium and oxygen 18 variations in the ocean and marine atmosphere, in: Stable Isotopes in Oceanographic Studies and Paleotemperatures, edited by: Tongiogi, E., Consiglio nazionale delle richerche, Laboratorio de geologia nucleare, 9–130, Pisa, 1965. a, b
Daëron, M., Drysdale, R. N., Peral, M., Huyghe, D., Blamart, D., Coplen, T. B., Lartaud, F., and Zanchetta, G.: Most Earth-surface calcites precipitate out of isotopic equilibrium, Nat. Commun., 10, 429, https://doi.org/10.1038/s41467-019-08336-5, 2019. a
Dahlqvist, P., Thorsbrink, M., and Sturkell, E.: Grundvattenmagasinet Lockne (Groundwater reservoir Lockne), Tech. Rep. K 612, Sveriges geologiska undersökning, ISBN 978-91-7403-435-6, 2018. a, b, c
Decrouy, L., Vennemann, T. W., and Ariztegui, D.: Controls on ostracod valve geochemistry, Part 1: Variations of environmental parameters in ostracod (micro-)habitats, Geochim. Cosmochim. Ac., 75, 7364–7379, https://doi.org/10.1016/j.gca.2011.09.009, 2011a. a, b
Decrouy, L., Vennemann, T. W., and Ariztegui, D.: Controls on ostracod valve geochemistry: Part 2. Carbon and oxygen isotope compositions, Geochim. Cosmochim. Ac., 75, 7380–7399, https://doi.org/10.1016/j.gca.2011.09.008, 2011b. a, b, c
Devriendt, L. S., McGregor, H. V., and Chivas, A. R.: Ostracod calcite records the ratio of the bicarbonate and carbonate ions in water, Geochim. Cosmochim. Ac., 214, 30–50, https://doi.org/10.1016/j.gca.2017.06.044, 2017. a
Eklund, A.: Vattentemperaturer i sjöar, sommar och vinter – resultat från SMHls mätningar [Water temperature in lakes, summer and winter – results from SMHI's measurements], Tech. Rep. 74, SMHI Swedish Meteorological and Hydrological Institute, ISSN 0282-7722, 1998. a, b, c
Gat, J. R.: Oxygen and hydrogen isotopes in the hydrologic cycle, Annu. Rev. Earth Pl. Sc., 24, 225–262, https://doi.org/10.1146/annurev.earth.24.1.225, 1996. a
Grossman, E. L. and Ku, T.-L.: Oxygen and carbon isotope fractionation in biogenic aragonite: Temperature effects, Chemical Geology: Isotope Geoscience section, 59, 59–74, https://doi.org/10.1016/0168-9622(86)90057-6, 1986. a, b
Hammarlund, D. and Buchardt, B.: Composite stable isotope records from a Late Weichselian lacustrine sequence at Grrenge, Lolland, Denmark: evidence of Allerød and Younger Dryas environments, Boreas, 25, 8–22, https://doi.org/10.1111/j.1502-3885.1996.tb00831.x, 1996. a, b
Hammarlund, D. and Keen, D. H.: A late Weichselian stable isotope and Molluscan Stratigraphy from Southern Sweden, GFF, 116, 235–248, https://doi.org/10.1080/11035899409546189, 1994. a, b
Hammarlund, D., Aravena, R., Barnekow, L., Buchardt, B., and Possnert, G.: Multi-component carbon isotope evidence of early Holocene environmental change and carbon-flow pathways from a hard-water lake in northern Sweden, J. Paleolimnol., 18, 219–233, 1997. a
Hammarlund, D., Barnekow, L., Birks, H. J. B., Buchardt, B., and Edwards, T. W. D.: Holocene changes in atmospheric circulation recorded in the oxygen-isotope stratigraphy of lacustrine carbonates from northern Sweden, Holocene, 12, 339–351, https://doi.org/10.1191/0959683602hl548rp, 2002. a, b
Hammarlund, D., Björck, S., Buchardt, B., Israelson, C., and Thomsen, C. T.: Rapid hydrological changes during the Holocene revealed by stable isotope records of lacustrine carbonates from Lake Igelsjön, southern Sweden, Quaternary Sci. Rev., 22, 353–370, https://doi.org/10.1016/S0277-3791(02)00091-4, 2003. a
Hammer, O., Harper, D. A. T., and Ryan, P. D.: PAST: Paleontological Statistics Software Package for Education and Data Analysis, Palaeontologia Electronica, 4, 1–9, 2001. a
Herbst, A., Tümpling, W. V., and Schubert, H.: The seasonal effects on the encrustation of charophytes in two hard‐water lakes, J. Phycol., 54, 630–637, https://doi.org/10.1111/jpy.12772, 2018. a
Hertzberg, J.: A seasonal solution to a palaeoclimate puzzle, Nature, 589, 521–522, https://doi.org/10.1038/d41586-021-00115-x, 2021. a
Holmes, J. A., Leuenberger, M., Molloy, K., and O'Connell, M.: Younger Dryas and Holocene environmental change at the Atlantic fringe of Europe derived from lake-sediment stable-isotope records from western Ireland, Boreas, 49, 233–247, https://doi.org/10.1111/bor.12425, 2020. a
Holopainen, I. and Jónasson, P.: Bathymetric Distribution and Abundance of Pisidium (Bivalvia: Sphaeriidae) in Lake Esrom, Denmark, from 1954 to 1988, OIKOS, 55, 324–334, 1989. a
IAEA/WMO: Global Network of Isotopes in Precipitation. The GNIP Database, https://nucleus.iaea.org/wiser (last access: 19 February 2021), 2019. a, b, c
Ito, E.: Application of Stable Isotope Techniques to Inorganic and Biogenic Carbonates, in: Tracking environmental change using lake sediments. Volume 2: Physical and Geochemical Methods, 351–371, Kluwer Academic Publishers, Dordrecht, the Netherlands, 2001. a
Jonsson, C. E., Andersson, S., Rosqvist, G. C., and Leng, M. J.: Reconstructing past atmospheric circulation changes using oxygen isotopes in lake sediments from Sweden, Clim. Past, 6, 49–62, https://doi.org/10.5194/cp-6-49-2010, 2010. a
Keatings, K. W., Heaton, T. H. E., and Holmes, J. A.: Carbon and oxygen isotope fractionation in non-marine ostracods: results from a “natural culture” environment, Geochim. Cosmochim. Ac., 66, 1701–1711, https://doi.org/10.1016/S0016-7037(01)00894-8, 2002. a
Kim, S.-T. and O'Neil, J. R.: Equilibrium and nonequilibrium oxygen isotope effects in synthetic carbonates, Geochim. Cosmochim. Ac., 61, 3461–3475, https://doi.org/10.1016/S0016-7037(97)00169-5, 1997. a, b, c, d
Kim, S.-T., O'Neil, J. R., Hillaire-Marcel, C., and Mucci, A.: Oxygen isotope fractionation between synthetic aragonite and water: Influence of temperature and Mg2+ concentration, Geochim. Cosmochim. Ac., 71, 4704–4715, https://doi.org/10.1016/j.gca.2007.04.019, 2007. a, b
Labuhn, I., Hammarlund, D., Chapron, E., Czymzik, M., Dumoulin, J., Nilsson, A., Régnier, E., Robygd, J., and von Grafenstein, U.: Holocene Hydroclimate Variability in Central Scandinavia Inferred from Flood Layers in Contourite Drift Deposits in Lake Storsjön, Quaternary, 1, 2, https://doi.org/10.3390/quat1010002, 2018. a
Labuhn, I., Tell, F., von Grafenstein, U., Hammarlund, D., Kuhnert, H., and Minster, B.: Groundwater inflow causes sediment movement on the lake floor of Locknesjön, Sweden, TIB [video], https://doi.org/10.5446/56815, 2021. a, b
Labuhn, I., Tell, F., von Grafenstein, U., Hammarlund, D., Kuhnert, H., and Minster, B.: Stable isotopes in water from Lake Locknesjön, Sweden collected between 2013 and 2018, PANGAEA [data set], https://doi.org/10.1594/PANGAEA.942628, 2022a. a
Labuhn, I., Tell, F., von Grafenstein, U., Hammarlund, D., Kuhnert, H., and Minster, B.: Stable isotopes in recent biogenic carbonates from Lake Locknesjön, Sweden collected in 2018, PANGAEA [data set], https://doi.org/10.1594/PANGAEA.942627, 2022b. a
Li, X. and Liu, W.: Oxygen isotope fractionation in the ostracod Eucypris mareotica: results from a culture experiment and implications for paleoclimate reconstruction, J. Paleolimnol., 43, 111, https://doi.org/10.1007/s10933-009-9317-8, 2010. a
Mann, H. B. and Whitney, D. R.: On a Test of Whether one of Two Random Variables is Stochastically Larger than the Other, Ann. Math. Stat., 18, 50–60, 1947. a
Martin, G., Torn, K., Blindow, I., Schubert, H., Munsterhjelm, R., and Henricson, C.: Introduction to charophytes, in: Charophytes of the Baltic Sea, edited by: Schubert, H. and Blindow, I., Gantner, Ruggell, Königstein, Germany, The Baltic Marine Biologists Publication, No. 19, ISBN 978-3-906166-06-3, 2003. a
Mayr, C., Lücke, A., Stichler, W., Trimborn, P., Ercolano, B., Oliva, G., Ohlendorf, C., Soto, J., Fey, M., Haberzettl, T., Janssen, S., Schäbitz, F., Schleser, G. H., Wille, M., and Zolitschka, B.: Precipitation origin and evaporation of lakes in semi-arid Patagonia (Argentina) inferred from stable isotopes (δ18O, δ2H), J. Hydrol., 334, 53–63, https://doi.org/10.1016/j.jhydrol.2006.09.025, 2007. a
Mayr, C., Laprida, C., Lücke, A., Martín, R. S., Massaferro, J., Ramón-Mercau, J., and Wissel, H.: Oxygen isotope ratios of chironomids, aquatic macrophytes and ostracods for lake-water isotopic reconstructions – Results of a calibration study in Patagonia, J. Hydrol., 529, 600–607, https://doi.org/10.1016/j.jhydrol.2014.11.001, 2015. a
McConnaughey, T.: 13C and 18O isotopic disequilibrium in biological carbonates: II. In vitro simulation of kinetic isotope effects, Geochim. Cosmochim. Ac., 53, 163–171, https://doi.org/10.1016/0016-7037(89)90283-4, 1989. a, b
McConnaughey, T. A. and Falk, R. H.: Calcium-Proton Exchange During Algal Calcification, Biol. Bull., 180, 185–195, https://doi.org/10.2307/1542440, 1991. a
McCormack, J. and Kwiecien, O.: Coeval primary and diagenetic carbonates in lacustrine sediments challenge palaeoclimate interpretations, Scientific Reports, 11, 7935, https://doi.org/10.1038/s41598-021-86872-1, 2021. a
McCormack, J., Nehrke, G., Jöns, N., Immenhauser, A., and Kwiecien, O.: Refining the interpretation of lacustrine carbonate isotope records: Implications of a mineralogy-specific Lake Van case study, Chem. Geol., 513, 167–183, https://doi.org/10.1016/j.chemgeo.2019.03.014, 2019. a
Meisch, C.: Freshwater Ostracoda of Western and Central Europe, Süßwasserfauna von Mitteleuropa, Spektrum Akademischer Verlag, Heidelberg, Berlin, ISBN 978-3-82741-001-6, 2000. a
Meyer, J., Wrozyna, C., Gross, M., Leis, A., and Piller, W. E.: Morphological and geochemical variations of Cyprideis (Ostracoda) from modern waters of the northern Neotropics, Limnology, 18, 251–273, https://doi.org/10.1007/s10201-016-0504-9, 2017. a
Mischke, S., Zhang, C., and Börner, A.: Bias of ostracod stable isotope data caused by drying of sieve residues from water, J. Paleolimnol., 40, 567–575, https://doi.org/10.1007/s10933-007-9160-8, 2007. a
Ormö, J., Hill, A. C., and Self‐Trail, J. M.: A chemostratigraphic method to determine the end of impact-related sedimentation at marine-target impact craters (Chesapeake Bay, Lockne, Tvären), Meteorit. Planet. Sci., 45, 1206–1224, https://doi.org/10.1111/j.1945-5100.2010.01084.x, 2010. a, b
Pearson, K.: Note on Regression and Inheritance in the Case of Two Parents, P. R. Soc. Lond. Ser.-I, 58, 240–242, https://doi.org/10.1098/rspl.1895.0041, 1895. a
Pentecost, A., Andrews, J. E., Dennis, P. F., Marca-Bell, A., and Dennis, S.: Charophyte growth in small temperate water bodies: Extreme isotopic disequilibrium and implications for the palaeoecology of shallow marl lakes, Palaeogeogr. Palaeocl., 240, 389–404, https://doi.org/10.1016/j.palaeo.2006.02.008, 2006. a
Pełechaty, M., Pukacz, A., Apolinarska, K., Pełechata, A., and Siepak, M.: The significance of Chara vegetation in the precipitation of lacustrine calcium carbonate, Sedimentology, 60, 1017–1035, https://doi.org/10.1111/sed.12020, 2013. a
Pronin, E., Pełechaty, M., Apolinarska, K., and Pukacz, A.: Oxygen stable isotope composition of carbonate encrustations of two modern, widely distributed, morphologically different charophyte species, Hydrobiologia, 809, 41–52, https://doi.org/10.1007/s10750-017-3444-4, 2018. a
R Core Team: R: A Language and Environment for Statistical Computing, https://www.r-project.org (last access: 2 October 2018), 2018. a
Rozanski, K., Araguás-Araguás, L., and Gonfiantini, R.: Relation between long-term trends of oxygen-18 isotope composition of precipitation and climate, Science, 258, 981–985, https://doi.org/10.1126/science.258.5084.981, 1992. a, b
Schwalb, A.: Lacustrine ostracodes as stable isotope recorders of late-glacial and Holocene environmental dynamics and climate, J. Paleolimnol., 29, 267–351, 2003. a
Shapiro, S. S. and Wilk, M. B.: An Analysis of Variance Test for Normality (Complete Samples), Biometrika, 52, 591–611, https://doi.org/10.2307/2333709, 1965. a
SMHI (Swedish Meteorological and Hydrological Institute): Vattenwebb, https://vattenwebb.smhi.se/, last access: 15 October 2020a. a, b, c, d, e, f
SMHI (Swedish Meteorological and Hydrological Institute): Öppna data, https://www.smhi.se/data/oppna-data/ (last access: 19 June 2020), 2020b. a, b
Spearman, C.: The Proof and Measurement of Association between Two Things, Am. J. Psychol., 15, 72–101, https://doi.org/10.2307/1412159, 1904. a
Spero, H. J., Bijma, J., Lea, D. W., and Bemis, B. E.: Effect of seawater carbonate concentration on foraminiferal carbon and oxygen isotopes, Nature, 390, 497–500, https://doi.org/10.1038/37333, 1997. a
Stroeven, A. P., Hättestrand, C., Kleman, J., Heyman, J., Fabel, D., Fredin, O., Goodfellow, B. W., Harbor, J. M., Jansen, J. D., Olsen, L., Caffee, M. W., Fink, D., Lundqvist, J., Rosqvist, G. C., Strömberg, B., and Jansson, K. N.: Deglaciation of Fennoscandia, Quaternary Sci. Rev., 147, 91–121, https://doi.org/10.1016/j.quascirev.2015.09.016, 2015. a
Sturkell, E., Ormö, J., and Lepinette, A.: Early modification stage (preresurge) sediment mobilization in the Lockne concentric, marine‐target crater, Sweden, Meteorit. Planet. Sci., 48, 321–338, https://doi.org/10.1111/maps.12058, 2013. a
Teranes, J. L., McKenzie, J. A., Bernasconi, S. M., Lotter, A. F., and Sturm, M.: A study of oxygen isotopic fractionation during bio-induced calcite precipitation in eutrophic Baldeggersee, Switzerland, Geochim. Cosmochim. Ac., 63, 1981–1989, https://doi.org/10.1016/S0016-7037(99)00049-6, 1999. a, b
von Grafenstein, U. and Labuhn, I.: Air-Interface: δ18O Records of Past Meteoric Water Using Benthic Ostracods from Deep Lakes, in: Paleoclimatology, edited by: Ramstein, G., Landais, A., Bouttes, N., Sepulchre, P., and Govin, A., 179–195, Springer International Publishing, https://doi.org/10.1007/978-3-030-24982-3_15, 2021. a, b, c
von Grafenstein, U., Erlenkeuser, H., Müller, J., and Kleinmann-Eisenmann, A.: Oxygen isotope records of benthic ostracods in Bavarian lake sediments, Naturwissenschaften, 79, 145–152, 1992. a
von Grafenstein, U., Erlenkeuser, H., Brauer, A., Jouzel, J., and Johnsen, S.: A Mid-European Decadal Isotope-Climate Record from 15,500 to 5000 Years B.P., Science, 284, 1654–1657, https://doi.org/10.1126/science.284.5420.1654, 1999a. a, b
von Grafenstein, U., Erlernkeuser, H., and Trimborn, P.: Oxygen and carbon isotopes in modern fresh-water ostracod valves: assessing vital offsets and autecological effects of interest for palaeoclimate studies, Palaeogeogr. Palaeocl., 148, 133–152, https://doi.org/10.1016/S0031-0182(98)00180-1, 1999b. a, b, c, d, e, f, g, h, i, j
von Grafenstein, U., Eicher, U., Erlenkeuser, H., Ruch, P., Schwander, J., and Ammann, B.: Isotope signature of the Younger Dryas and two minor oscillations at Gerzensee (Switzerland): palaeoclimatic and palaeolimnologic interpretation based on bulk and biogenic carbonates, Palaeogeogr. Palaeocl., 159, 215–229, https://doi.org/10.1016/S0031-0182(00)00086-9, 2000. a
Xia, J., Engstrom, D. R., and Ito, E.: Geochemistry of ostracode calcite: Part 2. The effects of water chemistry and seasonal temperature variation on Candona rawsoni, Geochim. Cosmochim. Ac., 61, 383–391, https://doi.org/10.1016/S0016-7037(96)00354-7, 1997a. a
Xia, J., Ito, E., and Engstrom, D. R.: Geochemistry of ostracode calcite: Part 1. An experimental determination of oxygen isotope fractionation, Geochim. Cosmochim. Ac., 61, 377–382, https://doi.org/10.1016/S0016-7037(96)00351-1, 1997b. a