the Creative Commons Attribution 4.0 License.
the Creative Commons Attribution 4.0 License.
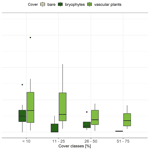
Pioneer biocrust communities prevent soil erosion in temperate forests after disturbances
Corinna Gall
Martin Nebel
Dietmar Quandt
Thomas Scholten
Steffen Seitz
Soil erosion continues to be one of the most serious environmental problems of our time and is exacerbated by progressive climate change. Until now, forests have been considered an ideal erosion control. However, even minor disturbances of the forest floor, for example, from heavy vehicles used for timber harvesting, can cause substantial sediment transport. An important countermeasure is the quick restoration of the uncovered soil surface by vegetation. To date, very little attention has been paid to the development of nonvascular plants, such as bryophytes, in disturbed areas of temperate forests and their impact on soil erosion. This study examined the natural succession of pioneer vegetation in skid trails on four soil substrates in a central European temperate forest and investigated their influence on soil erosion. For this purpose, rainfall simulations were conducted on small-scale runoff plots, and vegetation was continuously surveyed during the same period, primarily to map the development of bryophytes and the occurrence of biological soil crusts (biocrusts).
Biocrusts appeared immediately after disturbance, consisting primarily of bryophyte protonemata and cyanobacteria as well as coccoid and filamentous algae that lost their biocrust characteristics as succession progressed. They were present from April to July 2019, with a particular expression in the skid trail that was on shale clay (Psilonotenton Formation) and silty clay loam substrate. In general, skid trails on clayey substrates showed considerably higher bryophyte cover and species richness. Although bryophytes were subsequently overtopped by vascular plants, they managed to coexist until their growth was restricted due to leaf litter fall. Brachythecium rutabulum and Oxyrrhynchium hians were the most important and persistent pioneer bryophyte species, while Dicranella schreberiana and Pohlia lutescens were volatile and quickly disappeared after spreading in the summer. Sediment discharge was 22 times higher on disturbed bare soil compared with undisturbed forest soil and showed the largest sediment removal in the wheel tracks. Counteracting this, soil erosion decreased with the recovery of surface vegetation and was particularly reduced with growing pioneer biocrusts in summer, but it again increased in winter, when vascular vegetation became dominant. This leads to the conclusion that the role of bryophyte-dominated biocrusts in forests has been underestimated so far, and they can contribute more to soil conservation at specific times of succession than vascular plants.
- Article
(6588 KB) - Full-text XML
- BibTeX
- EndNote
For decades, soil erosion has been a major environmental problem, as it degrades the most productive soil layers, which threatens, among other things, food production worldwide. Although these effects have long been known, there are still a variety of challenges to mitigating soil erosion in different ecosystems. As climate change progresses, the risk of soil loss increases, particularly due to increased rainfall intensities, making the preparation of effective solutions an urgent matter (Olsson et al., 2019; Scholten and Seitz, 2019). The most prominent soil loss occurs in agricultural environments, and thus, considerable relevant research has been conducted in these habitats (Morgan, 2005; Maetens et al., 2012). Soil erosion in forests has received comparably less attention, as undisturbed forest ecosystems generally exhibit the lowest soil erosion among all land-use types (Blanco and Lal, 2008; Maetens et al., 2012; Panagos et al., 2015b) and are seen as a successful countermeasure to prevent the soil from being eroded (Panagos et al., 2015a; Wiśniewski and Märker, 2019).
However, soil erosion in forestlands can be locally severe, due in part to management intensity and tree species composition, for example, in subtropical forest ecosystems (Goebes et al., 2015; Seitz et al., 2016). Even forest disturbances on smaller scales, such as human-induced felling and skidding of individual trees or the construction of forest trail systems on sloped terrain, have the potential to drastically increase soil loss (Blanco and Lal, 2008). Sheridan and Noske (2007) showed that unsealed forest roads accounted for 4.4 % of the total annual sediment load from a forest, even though they represented only 0.023 % of the catchment. The most important reason for this is soil compaction and reduced infiltration rates caused by heavy machines used for timber harvesting (Foltz et al., 2009; Jordán-López et al., 2009; Wemple et al., 2018; Kastridis, 2020). For instance, results from Demir et al. (2007) revealed a significantly higher soil bulk density on skid trails, where soil compaction is caused by direct overpassing with forestry equipment. In this context, Zemke (2016) measured 58 times higher erosion rates on unfortified forest roads (272.2 g m−2) compared with undisturbed forest floor (4.7 g m−2) in a temperate forest in western Germany. Also, already vegetated wheel tracks of skid trails showed 5-fold higher soil erosion, up to 21.4 g m−2. Similarly, Safari et al. (2016) reported an increase in erosion rates of a factor of 14 for bare wheel tracks of skid trails relative to the undisturbed forest floor.
The findings of Li et al. (2019), Seitz et al. (2016), and Shinohara et al. (2019) suggest that it is not primarily the forest canopy that protects the soil against erosion but an intact forest floor. Several studies have also confirmed that soil erosion on skid trails was highest in the first year after logging and decreased significantly thereafter, mainly due to revegetation (Baharuddin et al., 1995; Jourgholami et al., 2017). Thus, the most important measure to counteract negative effects of soil erosion on the upper soil layer after skidding is a quick restoration of the soil surface by vegetation (Zemke, 2016; McEachran et al., 2018). These protective soil covers consist of either leaf and conifer litter from surrounding trees (Li et al., 2014; Seitz et al., 2015) or understorey vascular vegetation on the forest soil (Miyata et al., 2009; Liu et al., 2018). They also include a cryptogamic cover of bryophytes, lichens, fungi, algae, cyanobacteria, and various other bacteria lineages within or on top of the first millimetres of the soil, referred to as biological soil crust (biocrust; Weber et al., 2016, 2022). Especially when vascular-plant growth is limited by soil conditions such as high acidity or low nutrient and water availability, biocrusts play a vital role as pioneer soil colonizers and stabilizers (Corbin and Thiet, 2020) and can persist even in temperate climates due to these harsh environmental conditions (Szyja et al., 2018).
In mesic environments not necessarily constrained by harsh soil conditions, biocrusts occur primarily as an intermediate state of succession following disturbances such as deforestation (Seppelt et al., 2016), although they may redevelop seasonally if disturbances continue (Szyja et al., 2018; Kurth et al., 2021; Weber et al., 2022). The definition of biocrusts first provided by Belnap et al. (2003) referred to organisms that are in close contact with the soil surface and form a coherent hardening layer. In this context, all organisms with a substantial part of their biomass above the ground are excluded, e.g. large cryptogamic mats consisting of bryophytes or lichens, which are common in temperate coniferous forests. However, especially in temperate climates, the boundaries are fluid, so the distinction between biocrusts and cryptogamic covers is not always easy to make. Consequently, evidence of the occurrence of biocrusts in temperate forests is rare (Glaser et al., 2018; Corbin and Thiet, 2020).
Biocrusts in general, and especially bryophyte-dominated biocrusts, are known for their influence on hydrological processes (Eldridge et al., 2020) such as reducing surface runoff (Bu et al., 2015; Xiao et al., 2015) and, thus, decreasing sediment discharge (Silva et al., 2019). Such mitigation of soil erosion is also reported by cryptogamic covers consisting of bryophytes (Pan et al., 2006; Parsakhoo et al., 2012), which is inevitably related to their impressive water storage capacity, since bryophytes are able to absorb up to 20 times their dry weight (Proctor et al., 1998), with some Sphagnum species even reaching more than 50 times their dry weight (Wang and Bader, 2018). These mechanisms of water storage capacity are influenced by the complex 3D structure of bryophytes; the composition of a variety of individual functional traits, e.g. leaf area, leaf frequency, leaf area per shoot length, leaf area index, total surface area, shoot length, and shoot density; and their ability to form dense colony-level cushions (Elumeeva et al., 2011; Glime, 2021; Thielen et al., 2021). As most studies investigating the impact of biocrusts on soil erosion have been conducted in arid and semiarid regions, their influence in humid and temperate climates is largely unknown (Weber et al., 2016; Eldridge et al., 2020). Previous studies in subtropical China proved an important erosion-reducing effect of bryophyte-dominated biocrusts within early-stage forest plantations after clear-cutting (Seitz et al., 2017). It can be assumed that similar effects also occur in humid and temperate forest conditions; however, evidence for these effects is missing.
Pioneer biocrust communities could be particularly important as erosion-controlling agents in recently disturbed forest areas, such as along skid trails, where vascular plants are presumed to grow slowly due to harsh soil conditions. To date, few studies have addressed natural plant succession and its influencing factors in skid trail recovery (DeArmond et al., 2021), and of these, the majority relate exclusively to vascular plants (Buckley et al., 2003; Wei et al., 2015). For example, Mercier et al. (2019) observed on skid trails of different forest types in southern Germany that the species composition of vascular plants and bryophytes differed markedly from the forest interior. Furthermore, these vegetation surveys showed that vascular-plant species richness benefited from soil compaction in the skid trails, while bryophyte species richness was unaffected. Overall, there are still a variety of unresolved questions regarding the temporal development of species composition; species richness; and coverage of bryophytes in temperate forest disturbance zones and how they are affected by soil properties such as soil texture, bulk density, pH, and carbon and nitrogen content. With respect to these research gaps, it is of great interest to determine at what time and under what conditions biocrust communities naturally develop after the passing over by forestry machinery and when they transition to a more developed bryophyte cover. It is also important to investigate the functional role of these temperate successional stages of bryophyte cover in soil erosion. The knowledge gained from this study can be used to implement more targeted measures of good forestry practice to prevent soil erosion, for example, by enhancing the recovery of cryptogamic vegetation in skid trails.
This study examined the natural succession of pioneer vegetation with a focus on bryophytes and the occurrence of biocrusts in skid trails at four different sites with varying substrates and soil properties in a central European temperate forest. Moreover, it investigated the influence of bryophytes and biocrusts on soil erosion processes measured in small-scale runoff plots (ROPs) with rainfall simulations while also considering the position of the tracks within the skid trails. We tested the following hypotheses:
-
Species composition of bryophytes varies depending on individual skid trails.
-
Bryophyte cover and species richness are highest in wheel tracks, and total vegetation cover and vascular-plant species richness are highest in centre tracks, but each differs depending on the individual skid trail.
-
Soil erosion is reduced with increasing vegetation cover and is higher in wheel tracks than in centre tracks.
-
Bryophytes and early-successional bryophyte-dominated biocrusts are a major factor in mitigating soil losses following disturbances in temperate forests.
2.1 Study site
This study took place in Schönbuch Nature Park in southwestern Germany (Fig. A1 in the Appendix), which is situated in Triassic hills consisting of sandstones, marlstones, and claystones with abundant limestones and a few Lower Jurassic shales, sandstones, and limestones on the hilltops. The Lower Jurassic plateaus are often covered with a loess layer (Einsele and Agster, 1986). Schönbuch Nature Park represents a low-altitude (the highest peak, Bromberg, is 583 m above sea level), hilly (69 % with slopes ≤3∘ and 14 % with slopes >15∘), and almost completely forested (86 %) area in the sub-Atlantic temperate climate zone (Einsele and Agster, 1986; Arnold, 1986). While the mean annual temperature is 8.3 ∘C, the average precipitation is 740 mm (mean annual values from 1979 to 1984 at the climate station in Herrenberg; DWD Climate Data Center, 2021a), which is comparable to the long-term average for Germany (DWD Climate Data Center, 2021c, d).
For this research, four newly established (winter 2018/19) and unfortified skid trails in Schönbuch Nature Park with different parent materials, soil properties, and vegetation characteristics were selected (Table A1 in the Appendix). All four skid trails consisted of two wheel tracks and a centre track in between. They were created during logging operations conducted by the state forestry service of Baden-Württemberg (ForstBW) and represented an initial point of vegetation development when this study commenced.
The four skid trails were differentiated by their parent material and named according to the geological formation of the parent material: Angulatensandstein (AS), Psilonotenton (PT), Löwenstein (LS), and Trossingen (TS). AS consists of thin, platy, fine-grained sandstones containing limestone in an unweathered state; PT is composed of pyrite-bearing shale clay interstratified by beds of limestone; TS consists of firm, fractured, unstratified claystones with lime nodules; and LS forms medium- to coarse-grained banked sandstones interrupted by reddish marls (Einsele and Agster, 1986). The AS skid trail was located next to a loess deposition, which also determines soil properties. Since Schönbuch Nature Park was formed by extensive periglacial processes, the geological formation does not represent the parent rock of soil formation in every case (Bibus, 1986).
In the surroundings of LS, a reforested conifer stand was determined with approximately 70-year-old Pinus sylvestris and 50-year-old Picea abies, where the former occurred with 50 % cover and the latter with 40 % cover in the highest tree layer. Furthermore, in a second tree layer, about 20-year-old Fagus sylvatica and Carpinus betulus had colonized, covering the forest floor with leaf litter over the entire area such that a herb layer of about 10 %–20 % was formed, which was mainly restricted to sparse areas and dominated by grasses such as Carex sylvatica and Brachypodium sylvaticum. Additionally, a soil survey was carried out based on the classification system of the German soil mapping guideline (Bodenkundliche Kartieranleitung, KA5; Ad-hoc-AG Boden, 2005), and subsequently, the soil types according to the World Reference Base for Soil Resources (WRB; IUSS Working Group WRB, 2015) were derived using the WRB Tool for German Soil Data (Eberhardt et al., 2019). For LS, a Eutric Cambisol (Ochric) with typical moder was identified, and the soil surface was covered with a moss layer up to 5 % in total.
In comparison, the natural habitat of TS was dominated by young Picea abies (approx. 30-year-old), with 90 %–100 % of the soil surface covered with moss, and in 5 %–10 % of the area, a herb layer was formed. The soil survey revealed a Eutric Cambisol (Geoabruptic, Clayic, Ochric, Raptic, Protovertic), which was much deeper than the wheel track in the skid trail and covered with a mull-like moder humus layer.
The other two sites were characterized by deciduous tree species: while PT was formed primarily by beech trees (Fagus sylvatica) at different ages, developing a sparse tree layer and a very dense shrub layer, in AS, a sparse tree layer of approximately 100-year-old Quercus petraea and a second level of younger Fagus sylvatica and Carpinus betulus were found. In PT, a soil survey revealed a Eutric Calcaric Amphistagnic Cambisol (Loamic, Ochric) with a mull-like moder humus layer, and in the vegetation survey, a herb layer with a cover rate of less than 5 % was determined. In contrast, AS had a 20 % herb layer formed almost exclusively by Quercus petraea and Carpinus betulus seedlings, and the soil type was identified as Dystric Stagnic Regosol (Ochric) with L mull.
2.2 Field and laboratory methods
To test for particular impacts of early-successional post-disturbance forest floor vegetation on sediment discharge, rainfall simulations with micro-scale ROPs (0.4×0.4 m; cf. Seitz, 2015) were performed at four different times (March 2019, July 2019, October 2019, and February 2020). ROPs are stainless-steel metal frames connected with a triangular surface runoff gutter and are used to measure interrill erosion processes (Seitz, 2015; Zemke, 2016; Seitz et al., 2019), which is the discharge of sediment in thin sheets between rills due to shallow surface runoff (Blanco and Lal, 2008). A total of 4 ROPs were placed in each right wheel track, and 4 were in the centre track at each of the 4 skid trails, for a total of 32 ROPs. Two ROPs were placed in the undisturbed forest soil adjacent to every skid trail (n=8). While rainfall simulations in the skid trails were conducted for each of the four measurement times (n=128), in the undisturbed forest soil, they were reduced to measurements in October 2019 and February 2020 (n=16), yielding a total of 144 measurements.
Rainfall simulations were conducted with the Tübingen rainfall simulator (Iserloh et al., 2013; Seitz, 2015) that was equipped with a Lechler 460.788.30 nozzle and adjusted to a falling height of 3.5 m. Mean rainfall intensity was set at 60 mm h−1, applied over a duration of 30 min. This rainfall intensity refers to a regional rainfall event with a recurrence interval of 20 years (DWD Climate Data Center, 2021b). In each run, two ROPs (wheel and centre track) were irrigated simultaneously, with surface runoff and sediment collected in sample bottles (1 L). An overview of the experimental setup is available in Fig. A2. Prior to each rainfall simulation, soil moisture was determined next to every ROP using a ThetaProbe ML2 in combination with an HH2 Moisture Meter (Delta-T Devices, Cambridge, UK).
After soil erosion measurements, the total surface runoff for each ROP was gathered from the associated sample bottles marked with a millilitre measuring scale. To ascertain sediment discharge, the sample bottles were dried at 40 ∘C in a compartment drier and weighed in a dry state. To determine basic soil properties, bulk soil samples of the topsoil (0–5 cm) were collected in the surroundings of every ROP. While aggregate size was obtained by wet sieving, which served as a basis for the calculation of the mean weight diameter (MWD) of soil aggregates (van Bavel, 1950), grain size distribution was determined with an X-ray particle size analyser (SediGraph III, Micromeritics, Norcross, GA, US). Soil pH was measured with a pH meter and SenTix 81 electrodes (WTW, Weilheim, Germany) in 0.01 M CaCl2 solution. Additionally, soil organic carbon (SOC) and total nitrogen (Nt) were determined with an elemental analyser (vario EL III, Elementar Analysensysteme GmbH, Hanau, Germany). Core samples (100 cm3) were taken to determine soil bulk density in the topsoil using the mass-per-volume method (Blake and Hartge, 1986). Slope was measured on both sides of every ROP using an inclinometer, while aspect for the entire sites was derived from a digital elevation model (DEM, Geobasisdaten © Landesamt für Geoinformation und Landentwicklung Baden-Württemberg, https://www.lgl-bw.de, last access: 7 July 2022) using a geographical information system (QGIS version 3.16.13 Hannover; QGIS Development Team, 2020). Furthermore, skid trails were examined for water repellency by applying the water drop penetration time (WDPT) test (Dekker et al., 2009).
To investigate the development of vegetation cover on the forest floor surface in every ROP, sampling campaigns took place at five measurement times (April 2019, June 2019, July 2019, October 2019, and February 2020) synchronized with in situ soil erosion measurements. Vascular plants and bryophytes were classified by eye and identified by morphological characteristics using a stereomicroscope (SteREO Discovery.V8, Carl Zeiss Microscopy Deutschland GmbH, Oberkochen, Germany) and a microscope (Leitz SM-Lux, Ernst Leitz GmbH, Wetzlar, Germany). Classification was carried out to the species level (Tables 1 and 2), wherever possible, using the following plant identification literature: Jäger (2005), Nebel et al. (2000, 2001, 2005), and Moser (1963). In addition, total vegetation and bryophyte cover were surveyed for each ROP, while the Braun-Blanquet cover-abundance scale was used to determine coverages at the species level (Braun-Blanquet, 1964). Due to further use of the TS skid trail after the rainfall simulation in March 2019, it was not possible to survey the vegetation in the centre track in April 2019. Vascular-plant cover was calculated as the difference between total vegetation cover and bryophyte cover. Furthermore, perpendicular photographs were taken of each ROP with a digital compact camera (Panasonic DC-TZ91, Osaka, Japan) to additionally assess total vegetation cover with a photogrammetric survey and were processed with the grid quadrat method and using a digital grid overlay with 100 subdivisions (Belnap et al., 2001). Bare soil and vegetation covers were separated by hue distinction.
2.3 Statistics
All analyses were conducted with R 4.0.4 (R Core Team, 2021) on the level of individual samples. To screen for significant differences, Kruskal–Wallis tests were used in combination with post hoc Wilcoxon rank-sum tests for independent measurements and Wilcoxon signed-rank tests for related measurements (using the R package “stats”). To test for significant differences between cover types, we classified ROPs as bare, bryophyte, and vascular-plant ROPs. In bare ROPs, there was neither bryophyte nor vascular-plant cover; bryophyte ROPs were mainly covered by bryophytes; and vascular-plant ROPs were mainly covered by vascular plants, and, at the same time, bryophyte cover was lower than or equal to 10 %. A nonparametric analysis of covariance comparing nonparametric regression curves was performed to determine if there was a significant difference between vascular-plant ROPs and bryophyte ROPs in terms of sediment discharge (R package “sm”; Bowman and Azzalini, 2021). To determine whether bryophyte species composition differed significantly in the individual skid trails, an analysis of similarity (ANOSIM) with 999 permutations from the R package “vegan” was used (Oksanen et al., 2020). Additionally, generalized additive models (GAMs) with restricted maximum likelihood and smoothing parameters selected by an unbiased risk estimator (UBRE) criterion were performed to assess the effect of environmental parameters on soil erosion, total vegetation coverage, bryophyte coverage, and bryophyte species richness (R package “mgcv”; Wood, 2020). Prior to all statistical tests, normality was proved with the Shapiro–Wilk test, while homoscedasticity was verified using Levene's test. Significance was assessed as p<0.05 in all cases. For all mean values described, the standard error was also given (mean ± standard error). The selected colours for Fig. 1 are from the R package “wesanderson” (Karthik et al., 2018), and for Figs. 3, 4, 5, and 6 they are from the R package “RColorBrewer” (Neuwirth, 2022).
3.1 Bryophyte species composition
3.1.1 General succession of bryophyte species composition
Within the vegetation survey at five measurement times, a total of 24 moss, 2 liverwort, and 2 fungi species were found in the skid trails (Table 1), while 13 moss species occurred in the undisturbed forest soil (Table 2). The first bryophyte species to recolonize the skid trails in April 2019 after skidding were Brachythecium rutabulum (53.1 % of ROPs) and Oxyrrhynchium hians (37.5 % of ROPs). Protonemata of various species, the earliest stage of bryophyte development consisting of green cell filaments, were observed in 25 % of the ROPs. In June 2019, the percentage of ROPs occupied by Brachythecium rutabulum and Oxyrrhynchium hians increased to 75 % and 40.6 %, respectively, while protonemata were found in 31.3 % of the ROPs. Furthermore, Plagiomnium undulatum occurred in 25 % of the ROPs, and Thuidium tamariscinum occurred in 18.8 %. When the first bryophyte shoots developed from protonemata in July 2019, many occurrences could be assigned to the species Pohlia lutescens, Dicranella schreberiana, and Trichodon cylindricus. From July 2019 to February 2020, Oxyrrhynchium hians, Brachythecium rutabulum, and Plagiomnium undulatum remained the most abundant bryophyte species, and the quantity of different species increased. In comparison, 13 moss species occurred in the undisturbed forest soil (Table 2), 8 of which were also present in the skid trails.
Table 1Percentage occurrence of bryophyte and fungi species for a total of 32 runoff plots distributed in four skid trails in Schönbuch Nature Park in southwestern Germany, based on five vegetation surveys from April 2019 to February 2020.
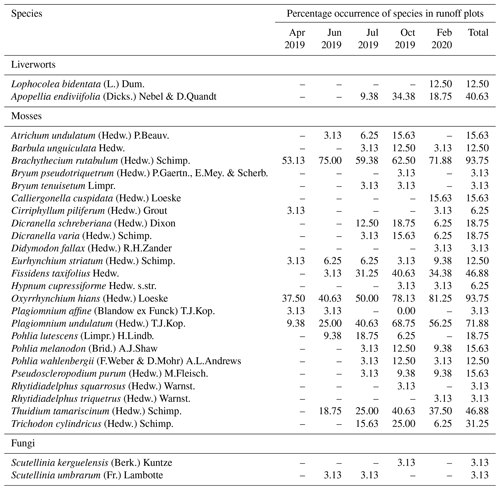
Table 2Percentage occurrence of bryophyte species for a total of eight runoff plots in undisturbed forest soil in Schönbuch Nature Park in southwestern Germany, based on one vegetation survey in February 2020.
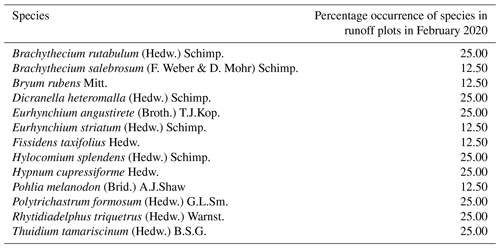
In our study area, the occurrence of cyanobacteria as well as coccoid and filamentous algae (e.g. Chlorophyceae and Xanthophyceae) plus bryophyte protonemata and the subsequent very early developmental stage of bryophyte shoots fulfilled the definition of biocrusts by Belnap et al. (2003) and Weber et al. (2022) and occurred from April to July 2019. Since the species Pohlia lutescens, Dicranella schreberiana, and Trichodon cylindricus have predominantly evolved from protonemata and formed only a minor part of their biomass above the soil surface in their early developmental stages, we include these species here among the temperate biocrust species. According to the biocrust definition of Belnap et al. (2003), we can also include the thallose liverwort Apopellia endiviifolia among the temperate biocrust species in our study area. Furthermore, Brachythecium rutabulum and Oxyrrhynchium hians have emerged as the most important pioneer species. Both species are widespread in Baden-Württemberg, Germany (Nebel et al., 2001), and are known to colonize a wide range of habitats (Nebel et al., 2001; Atherton et al., 2010). While Brachythecium rutabulum is particularly common on wood and stones, growing also on soil and gravelly ground, the habitat of Oxyrrhynchium hians is preferentially restricted to bare base-rich soils (Atherton et al., 2010), which renders both pioneer-friendly mosses (Nebel et al., 2001). Due to its competitive strength and broader distribution, Brachythecium rutabulum was even more frequent in the skid trails than Oxyrrhynchium hians. At a more advanced stage of succession, Plagiomnium undulatum and Thuidium tamariscinum also occurred, both of which grow mainly on forest soils (Nebel et al., 2001; Atherton et al., 2010). Furthermore, a clearly different species composition was found in the undisturbed forest soil compared with the skid trails. There, species composition showed an increased occurrence of more specialized species common in acidic woodlands, such as Hylocomium splendens, Polytrichastrum formosum, and Dicranella heteromalla (Atherton et al., 2010), which can be attributed to the lower pH in the undisturbed forest soil (mean pH = 4.54±0.07) compared with the skid trails (mean pH = 6.19±0.07). Mercier et al. (2019) also observed a different species composition in skid trails of different forest types in northern Bavaria compared with the forest interior during their vegetation surveys of vascular plants and bryophytes, indicating that skid trails can contribute to higher species diversity in managed forests.
3.1.2 Succession of bryophyte species composition in different skid trails
The vegetation succession developed differently in the four skid trails (see Figs. 1 and 2) in terms of species composition (p=0.001). At the beginning of vegetation succession after the disturbance due to skidding, we observed the development of protonemata in AS and PT. Whereas protonemata occurred in AS from April to July 2019 in 50 % of the ROPs, it was less common in PT but reached 50 % coverage in two ROPs in June 2019. These protonemata and their early-successional stages of Pohlia lutescens, Dicranella schreberiana, and Trichodon cylindricus are classified as biocrusts, which appeared in both PT and AS in April 2019 after the disturbance occurred and persisted in both skid trails until July 2019. The most abundant pioneer species were Brachythecium rutabulum and Oxyrrhynchium hians in all skid trails, but Oxyrrhynchium hians was absent in TS. TS was clearly dominated by Brachythecium rutabulum, which occurred in almost every ROP, with the coverage being up to 50 % in centre tracks, increasing constantly during the vegetation survey. Brachythecium rutabulum was present in all other skid trails but with less than 5 % coverage. Furthermore, Thuidium tamariscinum occurred in TS in almost every ROP and in centre track plots, also with a considerably high coverage of up to 25 % in October 2019 and February 2020; it did not colonize PT or AS, but it was also abundant in LS, with cover up to 5 %. Liverwort species developed most notably in October 2019 in PT, LS, and TS, with Apopellia endiviifolia occurring in PT and LS and Lophocolea bidentata found only in TS. While Plagiomnium undulatum did not occur in AS, it was very common in all other skid trails, with mostly low coverage (around 5 %). Generally, Plagiomnium undulatum development started early in summer (June or July 2019) in PT and LS and exclusively in autumn in TS. Especially in July and October 2019, Dicranella schreberiana was abundant in PT and in some ROPs, up to a coverage of 50 %, while it did not grow in all other skid trails. Furthermore, Oxyrrhynchium hians achieved high coverage rates of up to 25 % in PT.
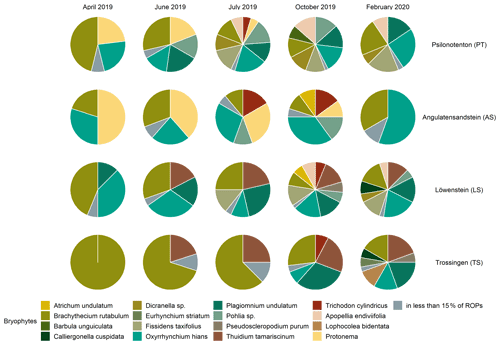
Figure 1Bryophyte species composition in the different skid trails for each time of vegetation survey. Species from the same genera are grouped together, and species which occur in less than 15 % of the runoff plots are listed in one group.
Pioneer biocrust species were found in the three skid trails in AS, PT, and LS. It was particularly interesting that the related moss species Dicranella schreberiana and Pohlia lutescens were more volatile than expected, spreading only during the summer and disappearing again at the beginning of autumn. Temporally, the liverwort biocrust species Apopellia endiviifolia appeared just when the moss biocrusts disappeared. As noted by Düll (1991), Apopellia endiviifolia is exclusively distributed at sites with neutral-to-alkaline pH, which is why it occurred in PT and LS in our study area but not in the other two skid trails. Brachythecium rutabulum occurred in all skid trails as a pioneer species; however, while in PT, AS, and LS it was associated with other moss species as succession progressed, in TS it was dominant in terms of coverage. Since Brachythecium rutabulum is known to be stimulated in growth by eutrophication (Nebel et al., 2001), high Nt in TS could be a possible explanation for its dominant occurrence there. In addition, TS was the only skid trail in which Oxyrrhynchium hians did not occur. On the one hand, this can be attributed to the fact that Brachythecium rutabulum is very competitive, especially on eutrophic sites, and suppresses other species (Nebel et al., 2001). On the other hand, TS had a low pH of 5.4±0.11, and since Oxyrrhynchium hians grows on base-rich soils, TS is not the preferred growing location. The absence of Plagiomnium undulatum in AS can be attributed to the fact that AS was clearly drier than the other sites, and according to Nebel et al. (2001), Plagiomnium undulatum is a permanent moisture indicator. This moisture requirement is also shown by the fact that Plagiomnium undulatum occurred comparatively late in the year in TS. We assume that only the formation of a closed vegetation cover of vascular plants at this site developed a sufficiently shady and humid microclimate for Plagiomnium undulatum to establish itself there. In this context, Sedia and Ehrenfeld (2003) and Ingerpuu et al. (2005) demonstrated that vascular plants can promote a microhabitat that is more hospitable for moss growth. Thuidium tamariscinum occurred exclusively in skid trails surrounded by coniferous forests, which corresponds to its preferential distribution area (Nebel et al., 2001).
3.2 Coverage and species richness
3.2.1 Bryophyte and total vegetation coverage
For all skid trails and vegetation surveys, bryophyte coverage was, on average, higher in centre tracks (12.01 ± 1.95 %) than in wheel tracks (7.15 ± 1.45 %; p<0.001), which was also true for total vegetation coverage (centre track: 60.49 ± 3.78 %; wheel track: 24.00 ± 3.73 %; p<0.001). With respect to the individual skid trails, the extent of bryophyte cover varied widely (Fig. 3). In AS and LS, bryophyte coverage averaged no more than 12.00 %, while in PT it peaked at 33.33 ± 6.67 % in July 2019, and TS achieved 34.64 ± 11.95 % in February 2020, with considerable variation in cover between wheel and centre tracks in the last two skid trails. PT showed a more pronounced development of bryophyte cover in wheel tracks (up to 40 % from June to October 2019), opposite to the preferential colonization of centre tracks in TS (up to 60 % in February 2020). While bryophyte cover in PT decreased between October 2019 and February 2020, this effect did not occur in TS. Calculated in a GAM that explained 80.3 % of the deviation of bryophyte cover, pH (p<0.001), SOC (p<0.001), sand content (p<0.001), total vegetation coverage (p<0.001), and Nt (p<0.05) were significant.
Generally, total vegetation and bryophyte cover developed with a higher coverage rate in centre tracks, indicating inferior soil conditions in wheel tracks compared with centre tracks. In this context, we found higher pH values in wheel tracks than in centre tracks, with the difference being significant for AS (wheel track: 5.8±0.08; centre track: 5.3±0.13; p<0.05), TS (wheel track: 5.6±0.06; centre track: 5.1±0.12; p<0.05), and LS (wheel track: 7.0±0.04; centre track: 6.8±0.05; p<0.05). The importance of soil pH on the growth of vascular plants and bryophytes, as well as their composition and diversity, has also been highlighted in several studies (Löbel et al., 2006; Hydbom et al., 2012; Oldén et al., 2016). For example, Rola et al. (2021) showed that soils with a more acidic pH promoted larger bryophyte coverage, which could explain, among other things, the generally higher bryophyte cover in centre tracks in our study.
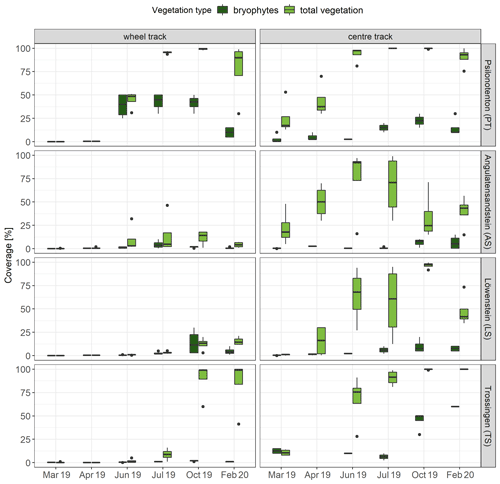
Figure 3Development of bryophyte (n=4) and total vegetation coverage (n=4) per runoff plot at the individual skid trails. The bottom and top of the box represent the first and third quartiles, and whiskers extend up to 1.5 times the interquartile range (IQR) of the data. Outliers are defined as more than 1.5 times the IQR and are displayed as dots.
In AS and LS, total vegetation coverage was lower than in PT (p<0.001), which was also the case for bryophyte cover (for AS and PT: p<0.001; for LS and PT: p<0.01). In comparison, PT and TS were rapidly overgrown by vascular plants; however, they did not displace bryophytes (see Fig. 3). This coexistence of vascular plants and bryophytes was also displayed in a positive correlation between their coverage rates (Spearman's correlation ρ = 0.38, p<0.001). Nevertheless, the overgrowth of bryophytes by vascular plants also marks the transition from biocrust to an evolved successional stage of bryophyte cover, characterized by a large proportion of the biomass being above the soil surface (Belnap et al., 2003). While closed vegetation cover developed in PT and TS until autumn in both centre and wheel tracks, no continuous pattern of growth emerged in AS and LS, with clear differences between centre and wheel tracks. AS and LS developed a very sparse total vegetation cover in wheel tracks (about 5 %) and revealed considerably higher coverage in centre tracks.
Biocrusts reached a more developed successional stage as bryophyte cover when they were overgrown by vascular plants. This bryophyte cover could be established even with high total vegetation cover, which contradicts observations that vascular plants limit bryophyte growth in different ecosystems (Bergamini et al., 2001; Fojcik et al., 2019; Corbin and Thiet, 2020). For instance, Fojcik et al. (2019) found a negative relationship between bryophyte cover and the coverage of vascular plants in a temperate forest ecosystem, which they attributed to competition between bryophytes and vascular plants. Bergamini et al. (2001) also discovered one such negative relationship and explained it primarily in terms of light availability, with a combination of optimal radiation and moisture conditions depending on the extent of vascular-plant cover. In contrast, Ingerpuu et al. (2005) verified in a grassland experiment that vascular plants could actually facilitate bryophyte growth, explaining this by the fact that vascular plants create a more favourable microclimate under their canopy. Likewise, positive correlations between vascular plants and bryophyte cover have been reported for temperate forests, which are comparable to our results (Márialigeti et al., 2009; Rola et al., 2021). According to Rola et al. (2021), this relationship can be explained by the species composition (e.g. expansive grasses and sedges could easily eliminate bryophytes; Chmura and Sierka, 2007) and a relatively low vascular-plant cover. A decline in bryophyte cover was observed for the first time in autumn on deciduous forest sites but not on coniferous sites. For this reason, we assume that bryophyte growth in our study area was limited by leaf litter fall rather than suppression by vascular plants. A negative effect of leaf litter was also reported in several other studies (Márialigeti et al., 2009; Fojcik et al., 2019; Mercier et al., 2019; Alatalo et al., 2020; Wu et al., 2020).
3.2.2 Bryophyte and vascular-plant species richness
Regarding bryophyte and vascular-plant species richness, we observed that a greater number of vascular-plant species occurred in centre tracks (9.85±0.59) than in wheel tracks (4.85±0.53; p<0.001), while no significant difference between tracks was found for bryophyte species richness (Fig. 4). Furthermore, species richness varied in the skid trails: PT and LS showed, on average, considerably higher numbers of bryophyte species compared with AS and TS (p<0.01). Concerning vascular plants, the highest species richness was achieved in PT, which was significantly higher than in AS and TS but not much higher than in LS. In comparison, AS, TS, and LS exhibited no differences in vascular-plant species richness. While bryophyte species richness was positively correlated with pH (Spearman's correlation ρ = 0.40, p<0.001) and negatively correlated with silt content (Spearman's correlation ρ = −0.35, p<0.001), we could not find any clear associations between the soil parameters surveyed and vascular-plant species richness. A GAM was used to explain 70.9 % of the deviation of bryophyte species richness, with pH (p<0.001), bryophyte cover (p<0.001), SOC (p<0.01), and Nt (p<0.01) being significant.
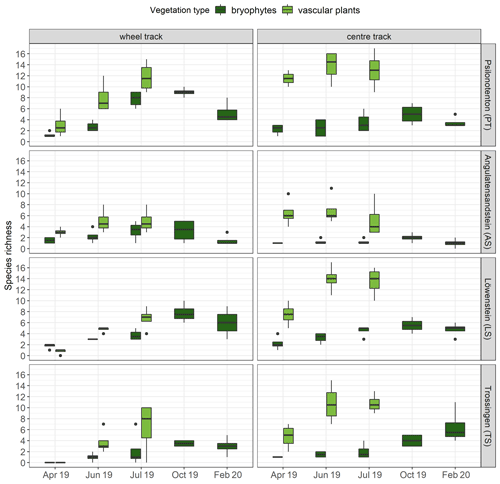
Figure 4Species richness of bryophytes (n=4) and vascular plants (n=4) per runoff plot at the individual skid trails. The bottom and top of the box represent the first and third quartiles, and whiskers extend up to 1.5 times the interquartile range (IQR) of the data. Outliers are defined as more than 1.5 times the IQR and are displayed as dots.
Our results revealed not only that development of total vegetation cover was slower and less pronounced in wheel tracks but also that fewer vascular-plant species could colonize there. Contrary to our expectations, bryophyte species richness was not affected by track position. In this context, Müller et al. (2013) found that experimentally induced disturbances had no impact on bryophyte species richness, whereas the diversity of annual plants benefited from disturbances. Minor disturbances, not exceeding 12 % bare ground, could still promote bryophyte species richness, while further disturbance was detrimental. Additionally, Mercier et al. (2019) discovered that soil compaction in skid trails had a positive effect on the species richness of vascular plants, while bryophyte species richness was not affected. AS and LS, which showed particularly low levels of coverage and species richness, exhibited a different underlying substrate (sandstone) from the other two skid trails (claystone), which was also why we found different soil conditions there. Regional variations in species richness of vascular plants and bryophytes due to different soil conditions have also been confirmed in a variety of studies (Löbel et al., 2006; Klaus et al., 2013; Müller et al., 2013; Filibeck et al., 2019), with pH in particular proving to be an important positive control variable for bryophyte species richness (Hydbom et al., 2012; Oldén et al., 2016; Tyler et al., 2018). Additionally, Tyler et al. (2018) discovered a significant influence of substrate type, soil depth, and grazing intensity on overall bryophyte species richness, with pH remaining the most important factor in this study also. Further factors influencing bryophyte species richness, such as light availability, the carbon-to-nitrogen ratio, and bark water capacity, were identified by Jagodziński et al. (2018) for 30-year-old reforested areas on lignite mining spoil heaps.
3.3 Soil erosion depending on site, track position, and vegetation cover
In total, mean sediment discharge in the wheel tracks reached 206.76 ± 24.53 g m−2 and 15.68 ± 3.84 g m−2 in the undisturbed forest soil (p<0.001), while centre tracks caused a sediment loss of 63.09 ± 10.28 g m−2, which was 4 times higher than the undisturbed forest soil (p<0.05). Considering ROPs with bare soil separately, an average soil erosion of 341.53 ± 68.20 g m−2 was achieved, which corresponds to a 22-fold enhancement compared with undisturbed forest soil. Additionally, sediment discharge in wheel tracks was increased by a factor of 3.3 compared with centre tracks. The main driver of sediment discharge was surface runoff (Spearman's correlation ρ = 0.80, p<0.001), and other important influencing soil characteristics were soil bulk density (Spearman's correlation ρ = 0.50, p<0.001), SOC and Nt (both with Spearman's correlation ρ = −0.46, p<0.001), and MWD (Spearman's correlation ρ = −0.46, p<0.001). Additionally, a negative correlation between soil erosion and clay content was identified (Spearman's correlation ρ = −0.42, p<0.001), and antecedent soil moisture and slope played a minor role in soil erosion. A GAM could explain 71.9 % of the deviation of sediment discharge, with runoff (p<0.001) and total vegetation cover (p<0.001) being significant.
These results show that skid trails are a major contributor to soil erosion in forest ecosystems and that compacted wheel tracks in particular significantly increased sediment discharge, which has also been demonstrated in previous studies (Safari et al., 2016; Zemke, 2016). In line with our results, Safari et al. (2016) highlighted soil texture, soil bulk density, SOC, and aggregate stability as the main soil parameters affecting runoff generation and soil erosion in skid trails. Based on these relationships, the significantly higher sediment discharge in skid trails is explained by the fact that the soil was disturbed and compacted by timber-harvesting machines, especially in wheel tracks, such that infiltration is reduced, which in turn leads to higher surface runoff and sediment transport (Zemke et al., 2019).
For all skid trails, sediment discharge was, on average, highest in March 2019 with a mean value of 201.80 ± 39.82 g m−2 and was considerably decreased in July 2019 to 74.13 ± 16.16 g m−2 (p<0.01). Subsequently, sediment discharge increased significantly in October 2019 (97.77 ± 21.16 g m−2; p<0.05) and rose again to 165.03 ± 29.75 g m−2 in February 2020 (p<0.001). Considering the time progression of soil erosion individually in the skid trails, different erosion mechanisms and sediment loads were evident (Fig. 5). Average sediment discharge was highest in AS with 243.63 ± 37.30 g m−2 and lowest in TS with 42.83 ± 10.34 g m−2, which represented a difference of a factor of 5.7 (p<0.001). While all skid trails differed from each other in terms of sediment discharge, no significant difference was detected between PT (151.62 ± 32.57 g m−2) and LS (99.26 ± 15.76 g m−2). With respect to the time progression of soil erosion in the skid trails, we found a difference between the measurement times for PT and LS but not for AS and TS. In both cases, sediment discharge was significantly reduced from the bare soil condition in March 2019 to an early-successional stage of biocrust and vascular-plant vegetation in July 2019: PT showed a decrease of 89 %, and LS had a reduction of 59 %. The same pattern of soil erosion over the year was also observed in AS but could not be statistically demonstrated. While the correlation between surface runoff and sediment discharge was particularly high on average for the first rainfall simulation (Spearman's correlation ρ = 0.89, p<0.001), the influence was distinctly reduced in the other simulations and especially in October 2019 (Spearman's correlation ρ = 0.51, p<0.01). In the subsequent rainfall simulations, vegetation cover was an additional factor influencing soil erosion: the negative relationship between total vegetation cover and sediment discharge increased considerably from the first to the third simulation in October 2019 (first simulation in March: Spearman's correlation ρ = −0.45, p<0.01; third simulation in October: Spearman's correlation ρ = −0.86, p<0.001), and the highest reduction in sediment discharge occurred in July 2019.
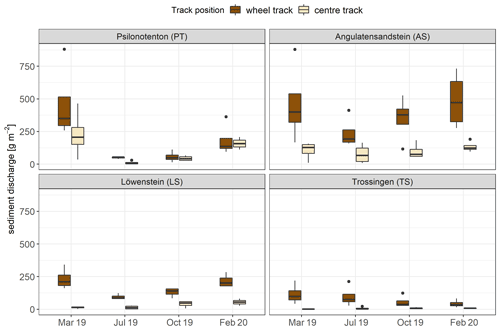
Figure 5Sediment discharge during simulated rainfall in the wheel track (n=4) and centre track (n=4) of the four skid trails for every rainfall simulation time. The bottom and top of the box represent the first and third quartiles, and whiskers extend up to 1.5 times the interquartile range (IQR) of the data. Outliers are defined as more than 1.5 times the IQR and are displayed as dots.
Overall, the amount of discharged sediment clearly depended on the particular site, likely indicating an important effect of parent material on soil properties and adjunct vegetation development and, thus, on soil erosion. A high influence of parent material on soil erosion was confirmed by Rodrigo-Comino et al. (2018). Regardless of the amount of sediment discharge, three skid trails showed comparable trends in soil erosion over time: in general, soil erosion was highest on bare soil; was reduced during the vegetation period, by the most with pioneer vegetation in July 2019, where biocrusts predominated; and then increased again in winter. This general trend was not observed in TS, which is probably related to the ecological structure of TS, since it was the only skid trail located in a clearing and was therefore clearly distinguished from the other skid trails in terms of vegetation succession. In addition, forest residues, such as bark, small branches, and needles were added to the topsoil in TS as a result of forestry use, which also had a stabilizing effect and certainly contributed to the low sediment discharge in this skid trail. The erosion-reducing effect of these types of mulching with forest residues has already been demonstrated in various studies (Prats et al., 2016; Prosdocimi et al., 2016), and Vinson et al. (2017) recently demonstrated that mulching strategies could also significantly reduce erosion rates in skid trails.
Several erosion studies on skid trails have already emphasized vegetation cover as one of the key control variables of soil erosion (Zemke, 2016; Malvar et al., 2017; McEachran et al., 2018). Soil erosion was often observed to be highest in the first year after skidding and decreased thereafter with increasing vegetation cover (Baharuddin et al., 1995; Jourgholami et al., 2017; Malvar et al., 2017). Martínez-Zavala et al. (2008) also reported a seasonality in their erosion measurements on forest road backslopes in southern Spain, with higher soil loss rates in winter despite vegetation cover, primarily attributed to higher soil moisture. However, they further found that this seasonal effect did not occur above a vegetation cover of 30 %. Thus, we hypothesize that, among other factors, higher soil moisture may have influenced increased winter soil erosion in our case as well, although we have not found significant correlations to support this theory.
3.4 Influence of bryophyte cover and early-successional bryophyte-dominated biocrusts on soil erosion
Sediment discharge was distinctly negatively affected by total vegetation cover (Spearman's correlation ρ = −0.61, p<0.001). Furthermore, we discovered a stronger negative correlation between bryophyte cover and sediment discharge (Spearman's correlation ρ = −0.54, p<0.001) than between vascular-plant cover and sediment discharge (Spearman's correlation ρ = −0.36, p<0.001). For these correlations, all undisturbed forest soil ROPs that were covered with leaf litter were extracted because we assume that litter-covered soils have a different protective mechanism than soils with bryophytes or vascular plants (Silva et al., 2019; Wang et al., 2020). All cover classes differed significantly from each other in terms of sediment discharge, with a reduction of 77 % being observed between bare ROPs and bryophyte ROPs (p<0.001) and a reduction of 59 % being observed between bare ROPs and vascular-plant ROPs (p<0.005). Bryophyte ROPs showed 44 % less sediment discharge than vascular-plant ROPs (p<0.05). When ROPs were categorized into different cover classes, there was a nonsignificant trend for bryophytes to result in less sediment discharge compared with vascular plants (Fig. 6). Especially with a cover of more than 50 %, the erosion-reducing effect of bryophytes was more pronounced compared with vascular plants; for example, the mean sediment discharge of bryophyte ROPs was 3.27 ± 1.50 g m−2, while vascular-plant ROPs still reached an average of 57.82 ± 12.47 g m−2, an 18-fold difference.
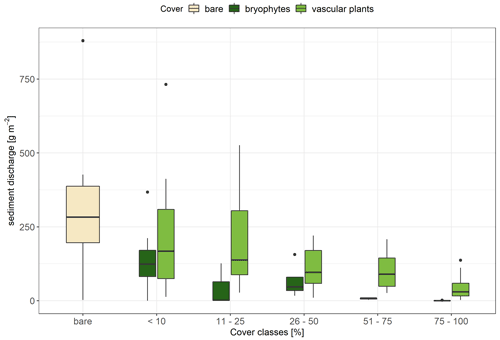
Figure 6Sediment discharge during simulated rainfall for bare (n=14), bryophyte (n=27), and vascular-plant (n=58) runoff plots (ROPs) categorized into cover classes. The bottom and top of the box represent the first and third quartiles, and whiskers extend up to 1.5 times the interquartile range (IQR) of the data. Outliers are defined as more than 1.5 times the IQR and are displayed as dots.
Bryophyte covers in temperate forest are known to stabilize soil surfaces and, thus, act as a protective agent against soil erosion (Mägdefrau and Wutz, 1951; Belnap and Büdel, 2016; Seitz et al., 2017). The same applies to covers of vascular plants (Zuazo Durán and Rodríguez Pleguezuelo, 2009); however, it is assumed that bryophyte communities have a stronger erosion-reducing effect than vascular plants (Casermeiro et al., 2004; Bu et al., 2015) due to their large water absorption capacity (Thielen et al., 2021) and the soil-stabilizing effect of their rhizoids (Mitchell et al., 2016). In this context, the biocrust characteristics were demonstrated in this study at the initial successional stage, with communities of bryophytes, their protonemata, cyanobacteria, and algae, for example, seeming to further enhance the erosion-reducing effect. Thus, the erosion-reducing effect appears to be stronger than that of communities dominated by vascular plants in the later stages (Fig. 5); this might be due to a combination of different complementary plant traits. Likewise, Seitz et al. (2017) attributed a positive effect to bryophyte protonemata in erosion control in mesic ecosystems. On the Loess Plateau in China, Bu et al. (2015) found that bryophyte-dominated biocrusts achieved a reduction in soil erosion of 81 % compared with bare soil, while a mixture of vascular plants and bryophytes contributed significantly less to erosion control (a 0.7 %–0.3 % reduction depending on plant species). Furthermore, Casermeiro et al. (2004) discovered during rainfall simulations in Spain that scrubs are more effective at mitigating soil erosion when they are underlain with a cover of bryophytes. However, contrasting results were reported for a very specific setup by Parsakhoo et al. (2012), who found that bryophyte-covered ROPs produced more sediment than ROPs with Rubus hyrcanus. Thus, there are still a number of unresolved questions regarding bryophyte–soil interactions on aspects such as water absorption, storage, and therefore erosion processes (Thielen et al., 2021), as well as on the development of biocrusts in mesic and forested areas, which need to be tackled in future research.
This study examined the initial development of pioneer nonvascular- and vascular-plant cover, composition, and species richness in temperate forest disturbance zones and their influence on soil erosion. Regarding our hypotheses, the following conclusions were drawn:
-
The succession of bryophytes and their composition varied at every site. Generally, Brachythecium rutabulum and Oxyrrhynchium hians were the most important and persistent pioneer bryophyte species, while Dicranella schreberiana and Pohlia lutescens formed covers that quickly disappeared after spreading in summer. Biocrust communities occurred immediately after disturbance from April to July 2019, consisting primarily of bryophyte protonemata and cyanobacteria as well as coccoid and filamentous algae.
-
Skid trails on clayey substrates showed considerably higher total vegetation cover and species richness, which applied to bryophytes and vascular plants. While vascular plants were more abundant in centre tracks than wheel tracks in terms of both cover and species richness, there was no clear difference in bryophyte species richness in this regard. Although bryophytes were quickly overtopped by vascular plants during vegetation succession, they managed to coexist until the end of the vegetation period and were then limited, most likely due to leaf litter fall.
-
The total amount of sediment discharge and the general mechanisms of soil erosion were clearly site dependent. Soil erosion was reduced, especially with the occurrence of pioneer biocrust vegetation in summer, and again increased in winter, when vascular vegetation became dominant. Sediment discharge was 13.2 times higher in wheel tracks than in undisturbed forest soil, and bare soil ROPs produced a 22-fold greater sediment discharge than undisturbed forest soil.
-
Bryophytes made a major contribution to erosion control after disturbances in this temperate forest ecosystem. They contributed more to mitigating soil erosion than vascular plants. Since soil erosion was especially low when bryophytes occurred within biocrusts, we assume that bryophyte-dominated biocrusts, in particular, are of utmost importance for preventing soil degradation, even in mesic environments.
Based on these results, artificial inoculation of bryophytes as erosion control on bare forest soils is assumed to be of particular interest for future research. In this context, Varela et al. (2021) recently published an approach to establish moss cultures from the laboratory, which could be applied for environmental studies. Moreover, the question arises as to whether bryophytes reduce soil erosion primarily through their protective-layer effect on splash and runoff or whether they also improve soil properties, such as aggregate stability, which further enhance erosion control (Riveras-Muñoz et al., 2022). Within this framework, it continues to be of special interest whether there are different mechanisms of erosion control depending on particular bryophyte species and which of their structural traits affect soil erosion patterns the most.
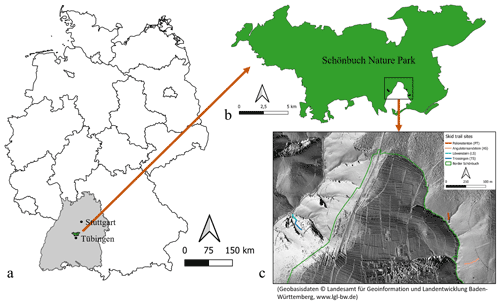
Figure A1Overview of the study area. (a) Location of Schönbuch Nature Park in Germany. (b) Location of the selected skid trails inside Schönbuch Nature Park. (c) Location of the four skid trails on a hillshade raster (Geobasisdaten © Landesamt für Geoinformation und Landentwicklung Baden-Württemberg, https://www.lgl-bw.de).
The codes used in this study are available upon request.
The dataset compiled and analysed in this study is available on figshare at https://doi.org/10.6084/m9.figshare.17206835.v3 (Gall et al., 2021).
StS, TS, DQ, and MN designed the experiment. CG and StS carried out field measurements, and CG was responsible for laboratory and data analyses. MN and CG conducted the vegetation surveys. CG and StS prepared the manuscript with contributions from all other co-authors.
The contact author has declared that none of the authors has any competing interests.
Publisher’s note: Copernicus Publications remains neutral with regard to jurisdictional claims in published maps and institutional affiliations.
This research would not have been possible without the exceptional support of Michael Sauer during vegetation surveys. His enthusiasm and knowledge of moss identification was an inspiration, and we thank him for his great contribution to this work. We would also like to express our sincere gratitude to Laura Bindereif, Lena Grabherr, Helena Obermeier, Philipp Gries, Delia Maas, Stefanie Gotterbarm, Giulia König, Nicolás Riveras-Muñoz, Sascha Scherer, Matthew A. Bowker, Daniel Schwindt, and all students of GEO51 in winter semester 2019/20 for their help with field and lab work. Many thanks also go to Sabine Flaiz, Rita Mögenburg, and Peter Kühn for always being available to help us with questions regarding lab work. Furthermore, we thank the state forestry service of Baden-Württemberg (ForstBW) for supporting the MesiCrust project. We are grateful to Bettina Weber, two further anonymous reviewers, and the students and tutor of the course “Critical Thinking in Ecological and Environmental Sciences” at the University of Edinburgh for a very constructive and helpful review.
This research has been supported by the Deutsche Forschungsgemeinschaft (grant no. SE 2767/2-1, “MesiCrust”).
This paper was edited by Steven Bouillon and reviewed by Bettina Weber and two anonymous referees.
Ad-hoc-AG Boden: Bodenkundliche Kartieranleitung, 5. verbesserte und erweiterte Auflage, Schweizerbart'sche Verlagsbuchhandlung, Stuttgart, ISBN 978-3-510-95920-4, 2005.
Alatalo, J. M., Jägerbrand, A. K., Erfanian, M. B., Chen, S., Sun, S.-Q., and Molau, U.: Bryophyte cover and richness decline after 18 years of experimental warming in alpine Sweden, AoB Plants, 12, 1–12, https://doi.org/10.1093/aobpla/plaa061, 2020.
Arnold, W.: Der Wald im Naturpark Schönbuch, in: Das landschaftsökologische Forschungsprojekt Naturpark Schönbuch: Wasser- und Stoffhaushalt, Bio-, Geo- und Forstwirtschaftliche Studien in Südwestdeutschland, edited by: Einsele, G., VCH Verlagsgesellschaft, Weinheim, ISBN 3-527-27122-8, 1986.
Atherton, I., Bosanquet, S., and Lawley, M. (Eds.): Mosses and Liverworts of Britian and Ireland – A field guide, British Bryological Society, Plymouth, ISBN: 978-0-956-13101-0, 2010.
Baharuddin, K., Mokhtaruddin, A. M., and Muhamad, M. N.: Surface runoff and soil loss from a skid trail and a logging road in a tropical forest, J. Trop. For. Sci., 7, 558–569, 1995.
Belnap, J. and Büdel, B.: Biological Soil Crusts as Soil Stabilizers, in: Biological soil crusts: An organizing principle in drylands, edited by: Weber, B., Büdel, B., and Belnap, J., Springer International Publishing, Cham, 305–320, https://doi.org/10.1007/978-3-319-30214-0_16, 2016.
Belnap, J., Kaltenecker, J. H., Rosentreter, R., Williams, J., Leonard, S., and Eldridge, D. (Eds.): Biological soil crusts: Ecology and management, US Department of the Interior – Bureau of Land Management and United States Geological Survey, https://ia802600.us.archive.org/16/items/biologicalsoilcr00beln/biologicalsoilcr00beln.pdf (last access: 6 July 2022), 2001.
Belnap, J., Büdel, B., and Lange, O. L.: Biological Soil Crusts: Characteristics and Distribution, in: Biological Soil Crusts: Structure, Function, and Management, edited by: Belnap, J. and Lange, O. L., Springer Berlin Heidelberg, Berlin, Heidelberg, 3–30, https://doi.org/10.1007/978-3-642-56475-8_1, 2003.
Bergamini, A., Pauli, D., Peintinger, M., and Schmid, B.: Relationships between productivity, number of shoots and number of species in bryophytes and vascular plants, J. Ecol., 89, 920–929, https://doi.org/10.1111/j.1365-2745.2001.00613.x, 2001.
Bibus, E.: Die Bedeutung periglazialer Deckschichten für Bodenprofil, Standort und junge Reliefentwicklung im Schönbuch bei Tübingen, in: Das landschaftsökologische Forschungsprojekt Naturpark Schönbuch: Wasser- und Stoffhaushalt, Bio-, Geo- und Forstwirtschaftliche Studien in Südwestdeutschland, edited by: Einsele, G., VCH Verlagsgesellschaft, Weinheim, ISBN 3-527-27122-8, 1986.
Blake, G. R. and Hartge, K. H.: Bulk density, in: Methods of Soil Analysis, Part 1: Physical and Mineralogical Methods, 2nd Edn., edited by: Arnold, K., American Society of Agronomy, Inc. and Soil Science Society of America, Inc., Madison, 363–375, https://doi.org/10.2136/sssabookser5.1.2ed.c13, 1986.
Blanco, H. and Lal, R.: Principles of Soil Conservation and Management, Springer, New York, ISBN 978-1-4020-8708-0, 2008.
Bowman, A. and Azzalini, A.: sm: Smoothing methods for nonparametric regression and density estimation (R package version 2.2-5.7), CRAN [code], http://www.stats.gla.ac.uk/~adrian/sm (last access: 6 July 2022), 2021.
Braun-Blanquet, J. (Ed.): Pflanzensoziologie. Grundzüge der Vegetationskunde, Springer Verlag, Wien, https://doi.org/10.1007/978-3-7091-8110-2, 1964.
Bu, C., Wu, S., Han, F., Yang, Y., and Meng, J.: The combined effects of moss-dominated biocrusts and vegetation on erosion and soil moisture and implications for disturbance on the Loess Plateau, China, PLOS ONE, 10, e0127394, https://doi.org/10.1371/journal.pone.0127394, 2015.
Buckley, D. S., Crow, T. R., Nauertz, E. A., and Schulz, K. E.: Influence of skid trails and haul roads on understory plant richness and composition in managed forest landscapes in Upper Michigan, USA, Forest Ecol. Manage., 175, 509–520, 2003.
Casermeiro, M. A., Molina, J. A., de la Cruz Caravaca, M. T., Hernando Costa, J., Hernando Massanet, M. I., and Moreno, P. S.: Influence of scrubs on runoff and sediment loss in soils of Mediterranean climate, Catena, 57, 91–107, https://doi.org/10.1016/S0341-8162(03)00160-7, 2004.
Chmura, D. and Sierka, E.: The invasibility of deciduous forest communities after disturbance: A case study of Carex brizoides and Impatiens parviflora invasion, Forest Ecol. Manage., 242, 487–495, https://doi.org/10.1016/j.foreco.2007.01.083, 2007.
Corbin, J. D. and Thiet, R. K.: Temperate biocrusts: Mesic counterparts to their better-known dryland cousins, Front. Ecol. Environ., 18, 456–464, https://doi.org/10.1002/fee.2234, 2020.
DeArmond, D., Ferraz, J., and Higuchi, N.: Natural recovery of skid trails. A review, Can. J. Forest Res., 51, 948–961, https://doi.org/10.1139/cjfr-2020-0419, 2021.
Dekker, L. W., Ritsema, C. J., Oostindie, K., Moore, D., and Wesseling, J. G.: Methods for determining soil water repellency on field-moist samples, Water Resour. Res., 45, 1–6, https://doi.org/10.1029/2008WR007070, 2009.
Demir, M., Makineci, E., and Yilmaz, E.: Investigation of timber harvesting impacts on herbaceous cover, forest floor and surface soil properties on skid road in an oak (Quercus petrea L.) stand, Build. Environ., 42, 1194–1199, https://doi.org/10.1016/j.buildenv.2005.11.008, 2007.
Düll, R.: Zeigerwerte von Laub- und Lebermoosen, in: Zeigerwerte von Pflanzen in Mitteleuropa: (Scripta Geobotanica, 18), edited by: Ellenberg, H., Verlag Erich Goltze KG, Göttingen, 175–214, ISBN 3-88452-518-2, 1991.
DWD Climate Data Center: Jahresmittel der Stationsmessungen der Lufttemperatur in 2 m Höhe in ∘C und Jahressumme der Niederschlagshöhe für Deutschland (Version v21.3), DWD Climate Data Center [data set], https://opendata.dwd.de/climate_environment/CDC/observations_germany/climate/annual/kl/historical/ (last access: 6 July 2022), 2021a.
DWD Climate Data Center: Grids of return periods of heavy precipitation (design precipitation) over Germany (KOSTRA-DWD) (version 2010R), DWD Climate Data Center [data set], https://opendata.dwd.de/climate_environment/CDC/grids_germany/return_periods/precipitation/KOSTRA/KOSTRA_DWD_2010R/asc/ (last access: 6 July 2022), 2021b.
DWD Climate Data Center: Annual regional averages of air temperature (annual mean) in ∘C (2 m above ground) (v19.3), DWD Climate Data Center [data set], https://opendata.dwd.de/climate_environment/CDC/regional_averages_DE/annual/air_temperature_mean/regional_averages_tm_year.txt (last access: 6 July 2022), 2021c.
DWD Climate Data Center: Annual regional averages of precipitation height (annual sum) in mm (v19.3), DWD Climate Data Center [data set], https://opendata.dwd.de/climate_environment/CDC/regional_averages_DE/annual/precipitation/regional_averages_rr_year.txt (last access: 6 July 2022), 2021d.
Eberhardt, E., Schad, P., Lehmann, C., and Harhoff, R.: WRB Tool for German Soil Data (Version 2.0 (09.01.2019)), Bundesanstalt für Geowissenschaften und Rohstoffe (BGR) [code], https://www.bgr.bund.de/EN/Themen/Boden/Projekte/Informationsgrundlagen_laufend/Uebersetzungsschluessel_en/UeSchluessel_AblSchluessel_en/AblS_KA5_WRB_LicenseTerms.html;jsessionid=6DDB24B66DEB03A844947F1AE57354F5.1_cid292?nn=1548376 (last access: 6 July 2022), 2019.
Einsele, G. and Agster, G.: Überblick zur Geologie und Morphologie des Schönbuchs, in: Das landschaftsökologische Forschungsprojekt Naturpark Schönbuch : Wasser- und Stoffhaushalt, Bio-, Geo- und Forstwirtschaftliche Studien in Südwestdeutschland, edited by: Einsele, G., VCH Verlagsgesellschaft, Weinheim, ISBN 3-527-27122-8, 1986.
Eldridge, D. J., Reed, S., Travers, S. K., Bowker, M. A., Maestre, F. T., Ding, J., Havrilla, C., Rodriguez-Caballero, E., Barger, N., Weber, B., Antoninka, A., Belnap, J., Chaudhary, B., Faist, A., Ferrenberg, S., Huber-Sannwald, E., Malam Issa, O., and Zhao, Y.: The pervasive and multifaceted influence of biocrusts on water in the world's drylands, Glob. Change Biol., 26, 6003–6014, https://doi.org/10.1111/gcb.15232, 2020.
Elumeeva, T. G., Soudzilovskaia, N. A., During, H. J., and Cornelissen, J. H.: The importance of colony structure versus shoot morphology for the water balance of 22 subarctic bryophyte species, J. Veg. Sci., 22, 152–164, https://doi.org/10.1111/j.1654-1103.2010.01237.x, 2011.
Filibeck, G., Sperandii, M. G., Bazzichetto, M., Mancini, L. D., Rossini, F., and Cancellieri, L.: Exploring the drivers of vascular plant richness at very fine spatial scale in sub-Mediterranean limestone grasslands (Central Apennines, Italy), Biodivers. Conserv., 28, 1–25, https://doi.org/10.1007/s10531-019-01788-7, 2019.
Fojcik, B., Wierzgoń, M., and Chmura, D.: Response of bryophytes to disturbances in managed forests. A case study from a Polish forest, Cryptogamie, Bryologie, 40, 105–118, https://doi.org/10.5252/cryptogamie-bryologie2019v40a10, 2019.
Foltz, R. B., Copeland, N. S., and Elliot, W. J.: Reopening abandoned forest roads in northern Idaho, USA: Quantification of runoff, sediment concentration, infiltration, and interrill erosion parameters, J. Environ. Manage., 90, 2542–2550, https://doi.org/10.1016/j.jenvman.2009.01.014, 2009.
Gall, C., Nebel, M., Sauer, M., Scholten, T., and Seitz, S.: Soil erosion and pioneer vegetation in temperate forests, figshare [data set], https://doi.org/10.6084/m9.figshare.17206835.v3, 2021.
Glaser, K., Baumann, K., Leinweber, P., Mikhailyuk, T., and Karsten, U.: Algal richness in BSCs in forests under different management intensity with some implications for P cycling, Biogeosciences, 15, 4181–4192, https://doi.org/10.5194/bg-15-4181-2018, 2018.
Glime, J. M.: Volume 1: Physiological Ecology, in: Bryophyte Ecology, edited by: Glime, J. M., Michigan Technological University, Michigan, https://digitalcommons.mtu.edu/oabooks/4 (last access: 6 July 2022), 2021.
Goebes, P., Bruelheide, H., Härdtle, W., Kröber, W., Kühn, P., Li, Y., Seitz, S., von Oheimb, G., and Scholten, T.: Species-specific effects on throughfall kinetic energy in subtropical forest plantations are related to leaf traits and tree architecture, PLoS ONE, 10, e0128084, https://doi.org/10.1371/journal.pone.0128084, 2015.
Hydbom, S., Ödman, A. M., Olsson, P. A., and Cronberg, N.: The effects of pH and disturbance on the bryophyte flora in calcareous sandy grasslands, Nord. J. Bot., 30, 446–452, https://doi.org/10.1111/j.1756-1051.2012.01463.x, 2012.
Ingerpuu, N., Liira, J., and Pärtel, M.: Vascular plants facilitated bryophytes in a grassland experiment, Plant Ecol., 180, 69–75, https://doi.org/10.1007/s11258-005-2508-0, 2005.
Iserloh, T., Ries, J., Arnáez, J., Boix-Fayos, C., Butzen, V., Cerdà, A., Echeverría, M., Fernández-Gálvez, J., Fister, W., and Geißler, C.: European small portable rainfall simulators: A comparison of rainfall characteristics, Catena, 110, 100–112, https://doi.org/10.1016/j.catena.2013.05.013, 2013.
IUSS Working Group WRB: World Reference Base for Soil Resources 2014, update 2015 International soil classification system for naming soils and creating legends for soil maps, World Soil Resources Reports No. 106 FAO, Rome, ISBN 978-92-5-108369-7, 2015.
Jäger, E. J. (Eds.): Rothmaler – Exkursionsflora von Deutschland, Band 4: Kritischer Band, Spektrum Akademischer Verlag, München, ISBN 978-3-8274-1496-0, 2005.
Jagodziński, A. M., Wierzcholska, S., Dyderski, M. K., Horodecki, P., Rusińska, A., Gdula, A. K., and Kasprowicz, M.: Tree species effects on bryophyte guilds on a reclaimed post-mining site, Ecol. Eng., 110, 117–127, https://doi.org/10.1016/j.ecoleng.2017.10.015, 2018.
Jordán-López, A., Martínez-Zavala, L., and Bellinfante, N.: Impact of different parts of unpaved forest roads on runoff and sediment yield in a Mediterranean area, Sci. Total Environ., 407, 937–944, https://doi.org/10.1016/j.scitotenv.2008.09.047, 2009.
Jourgholami, M., Labelle, E. R., and Feghhi, J.: Response of runoff and sediment on skid trails of varying gradient and traffic intensity over a two-year period, Forests, 8, 472, https://doi.org/10.3390/f8120472, 2017.
Karthik, R., Wickham, H., Richards, C., and Bagget, A.: wesanderson: A Wes Anderson palette generator (R package version 0.3.6), CRAN [code], https://CRAN.R-project.org/package=wesanderson (last access: 6 July 2022), 2018.
Kastridis, A.: Impact of forest roads on hydrological processes, Forests, 11, 1201, https://doi.org/10.3390/f11111201, 2020.
Klaus, V. H., Hölzel, N., Boch, S., Müller, J., Socher, S. A., Prati, D., Fischer, M., and Kleinebecker, T.: Direct and indirect associations between plant species richness and productivity in grasslands: Regional differences preclude simple generalization of productivity-biodiversity relationships, Preslia, 85, 97–112, 2013.
Kurth, J. K., Albrecht, M., Karsten, U., Glaser, K., Schloter, M., and Schulz, S.: Correlation of the abundance of bacteria catalyzing phosphorus and nitrogen turnover in biological soil crusts of temperate forests of Germany, Biol. Fert. Soils, 57, 179–192, https://doi.org/10.1007/s00374-020-01515-3, 2021.
Li, G., Wan, L., Cui, M., Wu, B., and Zhou, J.: Influence of canopy interception and rainfall kinetic energy on soil erosion under forests, Forests, 10, 509, https://doi.org/10.3390/f10060509, 2019.
Li, X., Niu, J., and Xie, B.: The effect of leaf litter cover on surface runoff and soil erosion in northern China, PLOS ONE, 9, e107789, https://doi.org/10.1371/journal.pone.0107789, 2014.
Liu, J., Gao, G., Wang, S., Jiao, L., Wu, X., and Fu, B.: The effects of vegetation on runoff and soil loss: Multidimensional structure analysis and scale characteristics, J. Geogr. Sci., 28, 59–78, https://doi.org/10.1007/s11442-018-1459-z, 2018.
Löbel, S., Dengler, J., and Hobohm, C.: Species richness of vascular plants, bryophytes and lichens in dry grasslands: The effects of environment, landscape structure and competition, Folia Geobot., 41, 377–393, https://doi.org/10.1007/BF02806555, 2006.
Maetens, W., Vanmaercke, M., Poesen, J., Jankauskas, B., Jankauskiene, G., and Ionita, I.: Effects of land use on annual runoff and soil loss in Europe and the Mediterranean: A meta-analysis of plot data, Prog. Phys. Geogr., 36, 599–653, https://doi.org/10.1177/0309133312451303, 2012.
Mägdefrau, K. and Wutz, A. (Eds.): Die Wasserkapazität der Moos- und Flechtendecke des Waldes, Veröffentlichung des Botanischen Instituts der Forstl. Forschungsanstalt München, https://doi.org/10.1007/BF01815956, 1951.
Malvar, M. C., Silva, F. C., Prats, S. A., Vieira, D. C. S., Coelho, C. O. A., and Keizer, J. J.: Short-term effects of post-fire salvage logging on runoff and soil erosion, Forest Ecol. Manag., 400, 555–567, https://doi.org/10.1016/j.foreco.2017.06.031, 2017.
Márialigeti, S., Németh, B., Tinya, F., and Ódor, P.: The effects of stand structure on ground-floor bryophyte assemblages in temperate mixed forests, Biodivers. Conserv., 18, 2223, https://doi.org/10.1007/s10531-009-9586-6, 2009.
Martínez-Zavala, L., Jordán López, A., and Bellinfante, N.: Seasonal variability of runoff and soil loss on forest road backslopes under simulated rainfall, Catena, 74, 73–79, https://doi.org/10.1016/j.catena.2008.03.006, 2008.
McEachran, Z. P., Slesak, R. A., and Karwan, D. L.: From skid trails to landscapes: Vegetation is the dominant factor influencing erosion after forest harvest in a low relief glaciated landscape, Forest Ecol. Manag., 430, 299–311, https://doi.org/10.1016/j.foreco.2018.08.021, 2018.
Mercier, P., Aas, G., and Dengler, J.: Effects of skid trails on understory vegetation in forests: a case study from Northern Bavaria (Germany), Forest Ecol. Manag., 453, 117579, https://doi.org/10.1016/j.foreco.2019.117579, 2019.
Mitchell, R. L., Cuadros, J., Duckett, J. G., Pressel, S., Mavris, C., Sykes, D., Najorka, J., Edgecombe, G. D., and Kenrick, P.: Mineral weathering and soil development in the earliest land plant ecosystems, Geology, 44, 1007–1010, https://doi.org/10.1130/G38449.1, 2016.
Miyata, S., Kosugi, K., Gomi, T., and Mizuyama, T.: Effects of forest floor coverage on overland flow and soil erosion on hillslopes in Japanese cypress plantation forests, Water Resour. Res., 45, 1–17, https://doi.org/10.1029/2008WR007270, 2009.
Morgan, R. P. C. (Ed.): Soil Erosion and Conservation, 3rd Edn., Blackwell Publishing, Oxford, ISBN 1-40511-781-8, 2005.
Moser, M.: Ascomyceten: (Schlauchpilze) in: Kleine Kryptogamenflora, Band 2: Pilze, edited by: Gams, H., Fischer, Stuttgart, 1963.
Müller, J., Heinze, J., Joshi, J., Boch, S., Klaus, V. H., Fischer, M., and Prati, D.: Influence of experimental soil disturbances on the diversity of plants in agricultural grasslands, J. Plant Ecol., 7, 509–517, https://doi.org/10.1093/jpe/rtt062, 2013.
Nebel, M., Philippi, G., Ahrens, M., Ingo, H., Michael, S., and Georg, S.: Die Moose Baden-Württembergs, Band 1: Bryophytina I, Andreaeales bis Funariales, edited by: Nebel, M. and Philippi, G., Eugen Ulmer Verlag, Stuttgart, ISBN 3-80013-527-2, 2000.
Nebel, M., Philippi, G., Ahrens, M., Sauer, M., Schäfer-Verwimp, A., and Schoepe, G.: Die Moose Baden-Württembergs, Band 2: Bryophytina II, Schistostegales bis Hypnobryales, edited by: Nebel, M. and Philippi, G., Eugen Ulmer Verlag, Stuttgart, 1–529, ISBN 3-80013-530-2, 2001.
Nebel, M., Philippi, G., Ahrens, M., Hölzer, A., Holz, I., Sauer, M., and Schoepe, G.: Die Moose Baden-Württembergs, Band 3: Bryophyta: Sphagnopsida, Marchantiophyta, Anthocerotophyta, edited by: Nebel, M. and Philippi, G., Eugen Ulmer Verlag, Stuttgart, ISBN 3-80013-278-8, 2005.
Neuwirth, E.: RColorBrewer: ColorBrewer Palettes (R package version 1.1-3), CRAN [code], https://CRAN.R-project.org/package=RColorBrewer, last access: 6 July 2022.
Oksanen, J., Blanchet, F. G., Friendly, M., Kindt, R., Legendre, P., McGlinn, D., Minchin, P. R., O'Hara, R. B., Simpson, G. L., Solymos, P., Stevens, M. H. H., Szoecs, E., and Wagner, H.: vegan: Community Ecology Package (R package version 2.5-7), CRAN [code], https://CRAN.R-project.org/package=vegan (last access: 6 July 2022), 2020.
Oldén, A., Raatikainen, K. J., Tervonen, K., and Halme, P.: Grazing and soil pH are biodiversity drivers of vascular plants and bryophytes in boreal wood-pastures, Agr. Ecosyst. Environ., 222, 171–184, https://doi.org/10.1016/j.agee.2016.02.018, 2016.
Olsson, L., Barbosa, H., Bhadwal, S., Cowie, A., Delusca, K., Flores-Renteria, D., Hermans, K., Jobbagy, E., Kurz, W., Li, D., Sonwa, D. J., and Stringer, L.: Land degradation, in: Climate Change and Land: An IPCC special report on climate change, desertification, land degradation, sustainable land management, food security, and greenhouse gas fluxes in terrestrial ecosystems, edited by: Shukla, P. R., Skea, J., Calvo Buendia, E., Masson-Delmotte, V., Pörtner, H.-O., Roberts, D. C., Zhai, P., Slade, R., Connors, S., van Diemen, R., Ferrat, M., Haughey, E., Luz, S., Neogi, S., Pathak, M., Petzold, J., Portugal Pereira, J., Vyas, P., Huntley, E., Kissik, K., Belkameci, M., and Malley, J., in press, 2019.
Pan, C., Shangguan, Z., and Lei, T.: Influences of grass and moss on runoff and sediment yield on sloped loess surfaces under simulated rainfall, Hydrol. Process., 20, 3815–3824, https://doi.org/10.1002/hyp.6158, 2006.
Panagos, P., Borrelli, P., Meusburger, K., Alewell, C., Lugato, E., and Montanarella, L.: Estimating the soil erosion cover-management factor at the European scale, Land Use Policy, 48, 38–50, https://doi.org/10.1016/j.landusepol.2015.05.021, 2015a.
Panagos, P., Borrelli, P., Poesen, J., Ballabio, C., Lugato, E., Meusburger, K., Montanarella, L., and Alewell, C.: The new assessment of soil loss by water erosion in Europe, Environ. Sci. Policy., 54, 438–447, https://doi.org/10.1016/j.envsci.2015.08.012, 2015b.
Parsakhoo, A., Lotfalian, M., Kavian, A., Hosseini, S., and Demir, M.: The effects of Rubus hyrcanus L. and Philonotis marchica (Hedw.) Brid. on soil loss prevention from cutslopes of a forest road, Journal of Forest Science, 58, 337–344, https://doi.org/10.17221/9/2012-JFS, 2012.
Prats, S. A., Wagenbrenner, J. W., Martins, M. A. S., Malvar, M. C., and Keizer, J. J.: Mid-term and scaling effects of forest residue mulching on post-fire runoff and soil erosion, Sci. Total Environ., 573, 1242–1254, https://doi.org/10.1016/j.scitotenv.2016.04.064, 2016.
Proctor, M. C. F., Nagy, Z., Csintalan, Z., and Takács, Z.: Water-content components in bryophytes: Analysis of pressure-volume relationships, J. Exp. Bot., 49, 1845–1854, https://doi.org/10.1093/jxb/49.328.1845, 1998.
Prosdocimi, M., Tarolli, P., and Cerdà, A.: Mulching practices for reducing soil water erosion: A review, Earth-Sci. Rev., 161, 191–203, https://doi.org/10.1016/j.earscirev.2016.08.006, 2016.
QGIS Development Team: QGIS Geographic Information System. Open Source Geospatial Foundation Project (3.16.13-Hannover) [code], https://qgis.org/de/ (last access: 6 July 2022), 2020.
R Core Team: R: A language and environment for statistical computing, R Foundation for Statistical Computing [code], https://www.r-project.org/ (last access: 6 July 2022), 2021.
Riveras-Muñoz, N., Seitz, S., Witzgall, K., Rodríguez, V., Kühn, P., Mueller, C. W., Oses, R., Seguel, O., Wagner, D., and Scholten, T.: Biocrust-linked changes in soil aggregate stability along a climatic gradient in the Chilean Coastal Range, SOIL Discuss. [preprint], https://doi.org/10.5194/soil-2021-141, in review, 2022.
Rodrigo-Comino, J., Novara, A., Gyasi-Agyei, Y., Terol, E., and Cerdà, A.: Effects of parent material on soil erosion within Mediterranean new vineyard plantations, Eng. Geol., 246, 255–261, https://doi.org/10.1016/j.enggeo.2018.10.006, 2018.
Rola, K., Plášek, V., Rożek, K., and Zubek, S.: Effect of tree species identity and related habitat parameters on understorey bryophytes – interrelationships between bryophyte, soil and tree factors in a 50-year-old experimental forest, Plant Soil, 466, 613–630, https://doi.org/10.1007/s11104-021-05074-w, 2021.
Safari, A., Kavian, A., Parsakhoo, A., Saleh, I., and Jordán, A.: Impact of different parts of skid trails on runoff and soil erosion in the Hyrcanian forest (northern Iran), Geoderma, 263, 161–167, https://doi.org/10.1016/j.geoderma.2015.09.010, 2016.
Scholten, T. and Seitz, S.: Soil erosion and land degradation, Soil Syst., 3, 68, https://doi.org/10.3390/soilsystems3040068, 2019.
Sedia, E. G. and Ehrenfeld, J. G.: Lichens and mosses promote alternate stable plant communities in the New Jersey Pinelands, Oikos, 100, 447–458, https://doi.org/10.1034/j.1600-0706.2003.12058.x, 2003.
Seitz, S.: Mechanisms of soil erosion in subtropical chinese forests – Effects of species diversity, species identity, functional traits and soil fauna on sediment discharge, Universitätsbibliothek Tübingen, https://doi.org/10.15496/publikation-8010, 2015.
Seitz, S., Goebes, P., Zumstein, P., Assmann, T., Kühn, P., Niklaus, P. A., Schuldt, A., and Scholten, T.: The influence of leaf litter diversity and soil fauna on initial soil erosion in subtropical forests, Earth Surf. Proc. Land., 40, 1439–1447, https://doi.org/10.1002/esp.3726, 2015.
Seitz, S., Goebes, P., Song, Z., Bruelheide, H., Härdtle, W., Kühn, P., Li, Y., and Scholten, T.: Tree species and functional traits but not species richness affect interrill erosion processes in young subtropical forests, SOIL, 2, 49–61, https://doi.org/10.5194/soil-2-49-2016, 2016.
Seitz, S., Nebel, M., Goebes, P., Käppeler, K., Schmidt, K., Shi, X., Song, Z., Webber, C. L., Weber, B., and Scholten, T.: Bryophyte-dominated biological soil crusts mitigate soil erosion in an early successional Chinese subtropical forest, Biogeosciences, 14, 5775–5788, https://doi.org/10.5194/bg-14-5775-2017, 2017.
Seitz, S., Goebes, P., Puerta, V. L., Pereira, E. I. P., Wittwer, R., Six, J., van der Heijden, M. G., and Scholten, T.: Conservation tillage and organic farming reduce soil erosion, Agron. Sustain. Dev., 39, 1–10, https://doi.org/10.1007/s13593-018-0545-z, 2019.
Seppelt, R. D., Downing, A. J., Deane-Coe, K. K., Zhang, Y., and Zhang, J.: Bryophytes Within Biological Soil Crusts, in: Biological Soil Crusts: An Organizing Principle in Drylands, edited by: Weber, B., Büdel, B., and Belnap, J., Springer International Publishing, Cham, 101–120, https://doi.org/10.1007/978-3-319-30214-0_6, 2016.
Sheridan, G. J. and Noske, P. J.: Catchment-scale contribution of forest roads to stream exports of sediment, phosphorus and nitrogen, Hydrol. Process., 21, 3107–3122, https://doi.org/10.1002/hyp.6531, 2007.
Shinohara, Y., Misumi, Y., Kubota, T., and Nanko, K.: Characteristics of soil erosion in a moso-bamboo forest of western Japan: Comparison with a broadleaved forest and a coniferous forest, Catena, 172, 451–460, https://doi.org/10.1016/j.catena.2018.09.011, 2019.
Silva, F. C., Vieira, D. C., van der Spek, E., and Keizer, J. J.: Effect of moss crusts on mitigation of post-fire soil erosion, Ecol. Eng., 128, 9–17, https://doi.org/10.1016/j.ecoleng.2018.12.024, 2019.
Szyja, M., Büdel, B., and Colesie, C.: Ecophysiological characterization of early successional biological soil crusts in heavily human-impacted areas, Biogeosciences, 15, 1919–1931, https://doi.org/10.5194/bg-15-1919-2018, 2018.
Thielen, S. M., Gall, C., Ebner, M., Nebel, M., Scholten, T., and Seitz, S.: Water's path from moss to soil: A multi-methodological study on water absorption and evaporation of soil-moss combinations, J. Hydrol. Hydromech., 69, 421–435, https://doi.org/10.2478/johh-2021-0021, 2021.
Tyler, T., Bengtsson, F., Dahlberg, C. J., Lönnell, N., Hallingbäck, T., and Reitalu, T.: Determinants of bryophyte species composition and diversity on the Great Alvar of Öland, Sweden, J. Bryol., 40, 12–30, https://doi.org/10.1080/03736687.2017.1412387, 2018.
van Bavel, C. H. M.: Mean Weight-Diameter of Soil Aggregates as a Statistical Index of Aggregation, Soil Sci. Soc. Am. J., 14, 20–23, https://doi.org/10.2136/sssaj1950.036159950014000C0005x, 1950.
Varela, Z., Real, C., Branquinho, C., do Paço, T. A., and Cruz de Carvalho, R.: Optimising artificial moss growth for environmental studies in the Mediterranean area, Plants, 10, 2523, https://doi.org/10.3390/plants10112523, 2021.
Vinson, J. A., Barrett, S. M., Aust, W. M., and Bolding, M. C.: Evaluation of bladed skid trail closure methods in the ridge and valley region, Forest Sci., 63, 432–440, https://doi.org/10.5849/FS.2016-030R1, 2017.
Wang, L., Zhang, G., Zhu, P., and Wang, X.: Comparison of the effects of litter covering and incorporation on infiltration and soil erosion under simulated rainfall, Hydrol. Process., 34, 2911–2922, https://doi.org/10.1002/hyp.13779, 2020.
Wang, Z. and Bader, M. Y.: Associations between shoot-level water relations and photosynthetic responses to water and light in 12 moss species, AoB Plants, 10, ply034, https://doi.org/10.1093/aobpla/ply034, 2018.
Weber, B., Büdel, B., and Belnap, J. (Eds.): Biological Soil Crusts: An Organizing Principle in Drylands, Ecological studies, Vol. 226, Springer, Dordrecht, https://doi.org/10.1007/978-3-319-30214-0, 2016.
Weber, B., Belnap, J., Büdel, B., Antoninka, A. J., Barger, N. N., Chaudhary, V. B., Darrouzet-Nardi, A., Eldridge, D. J., Faist, A. M., Ferrenberg, S., Havrilla, C. A., Huber-Sannwald, E., Malam Issa, O., Maestre, F. T., Reed, S. C., Rodriguez-Caballero, E., Tucker, C., Young, K. E., Zhang, Y., Zhao, Y., Zhou, X., and Bowker, M. A.: What is a biocrust? A refined, contemporary definition for a broadening research community, Biol. Rev., https://doi.org/10.1111/brv.12862, online first, 2022.
Wei, L., Villemey, A., Hulin, F., Bilger, I., Yann, D., Chevalier, R., Archaux, F., and Gosselin, F.: Plant diversity on skid trails in oak high forests: A matter of disturbance, micro-environmental conditions or forest age?, Forest Ecol. Manag., 338, 20–31, https://doi.org/10.1016/j.foreco.2014.11.018, 2015.
Wemple, B. C., Browning, T., Ziegler, A. D., Celi, J., Chun, K. P., Jaramillo, F., Leite, N. K., Ramchunder, S. J., Negishi, J. N., Palomeque, X., and Sawyer, D.: Ecohydrological disturbances associated with roads: Current knowledge, research needs, and management concerns with reference to the tropics, Ecohydrology, 11, e1881, https://doi.org/10.1002/eco.1881, 2018.
Wiśniewski, P. and Märker, M.: The role of soil-protecting forests in reducing soil erosion in young glacial landscapes of Northern-Central Poland, Geoderma, 337, 1227–1235, https://doi.org/10.1016/j.geoderma.2018.11.035, 2019.
Wood, S.: mgcv: Mixed GAM computation vehicle with automatic smoothness estimation (R package version 1.8-33), CRAN [code], https://cran.r-project.org/web/packages/mgcv/ (last access: 6 July 2022), 2020.
Wu, G.-L., Zhang, M.-Q., Liu, Y., and López-Vicente, M.: Litter cover promotes biocrust decomposition and surface soil functions in sandy ecosystem, Geoderma, 374, 114429, https://doi.org/10.1016/j.geoderma.2020.114429, 2020.
Xiao, B., Zhao, Y., Wang, Q., and Li, C.: Development of artificial moss-dominated biological soil crusts and their effects on runoff and soil water content in a semi-arid environment, J. Arid Environ., 117, 75–83, https://doi.org/10.1016/j.jaridenv.2015.02.017, 2015.
Zemke, J. J.: Runoff and soil erosion assessment on forest roads using a small scale rainfall simulator, Hydrology, 3, 25, https://doi.org/10.3390/hydrology3030025, 2016.
Zemke, J. J., Enderling, M., Klein, A., and Skubski, M.: The influence of soil compaction on runoff formation. A case study focusing on skid trails at forested andosol sites, Geosciences, 9, 204, https://doi.org/10.3390/geosciences9050204, 2019.
Zuazo Durán, V. H. and Rodríguez Pleguezuelo, C. R.: Soil-erosion and runoff prevention by plant covers: A review, in: Sustainable Agriculture, edited by: Lichtfouse, E., Navarrete, M., Debaeke, P., Véronique, S., and Alberola, C., Springer Netherlands, Dordrecht, 785–811, https://doi.org/10.1051/agro:2007062, 2009.