the Creative Commons Attribution 4.0 License.
the Creative Commons Attribution 4.0 License.
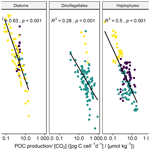
Physiological control on carbon isotope fractionation in marine phytoplankton
Karen M. Brandenburg
Björn Rost
Dedmer B. Van de Waal
Mirja Hoins
Appy Sluijs
One of the great challenges in biogeochemical research over the past half a century has been to quantify and understand the mechanisms underlying stable carbon isotope fractionation (εp) in phytoplankton in response to changing CO2 concentrations. This interest is partly grounded in the use of fossil photosynthetic organism remains as a proxy for past atmospheric CO2 levels. Phytoplankton organic carbon is depleted in 13C compared to its source because of kinetic fractionation by the enzyme RubisCO during photosynthetic carbon fixation, as well as through physiological pathways upstream of RubisCO. Moreover, other factors such as nutrient limitation, variations in light regime as well as phytoplankton culturing systems and inorganic carbon manipulation approaches may confound the influence of aquatic CO2 concentrations [CO2] on εp. Here, based on experimental data compiled from the literature, we assess which underlying physiological processes cause the observed differences in εp for various phytoplankton groups in response to C-demand/C-supply, i.e., particulate organic carbon (POC) production [CO2]) and test potential confounding factors. Culturing approaches and methods of carbonate chemistry manipulation were found to best explain the differences in εp between studies, although day length was an important predictor for εp in haptophytes. Extrapolating results from culturing experiments to natural environments and for proxy applications therefore require caution, and it should be carefully considered whether culture methods and experimental conditions are representative of natural environments.
- Article
(1057 KB) - Full-text XML
-
Supplement
(1026 KB) - BibTeX
- EndNote
Understanding of past climates, in particular variations in atmospheric CO2 concentrations and concomitant temperatures, may help to improve climate models and constrain the global temperature response to the projected CO2 rise (Rohling et al., 2012; Zhu et al., 2020; Tierney et al., 2020). Reconstructions of past CO2 concentrations [CO2] beyond the reach of ice cores rely on proxy estimates. These are based on biogeochemical relations between [CO2] in the atmosphere and the chemical or morphological properties of biogenic carbonates, other minerals, fossil leaves or various types of organic matter that can be found in sediments (Foster et al., 2017; Macdonald, 2020). All these proxies are based on assumptions and exhibit large uncertainties that are ideally constrained iteratively.
One line of proxies uses the CO2-dependence of 13C fractionation during photosynthetic carbon fixation in phytoplankton (O'Leary, 1984; Sharkey and Berry, 1985; Farquhar et al., 1989). Several components found in sediments have been proposed for this, including bulk organic matter (Hayes et al., 1999) and algae-derived molecules, such as porphyrins (Freeman and Hayes, 1992) and phytane (Bice et al., 2006) produced by all photosynthetic organisms. Also, the potential of more specific proxies has been tested, including alkenones originating from coccolithophores (Jasper and Hayes, 1990) and resting cysts produced by dinoflagellates (Hoins et al., 2015; Sluijs et al., 2018). The δ13C signal of various algal remains is thought to follow the δ13C signal of dissolved inorganic carbon (DIC), in particular the δ13C signal of CO2, modulated by CO2-dependent fractionation during photosynthetic carbon fixation. Therefore, ultimately, the δ13C signal in algal fossil remains may be used for estimating atmospheric CO2 levels through geological time. Accurate use of this proxy relies on the mechanistic understanding of carbon isotope fractionation (εp) in phytoplankton, which is obtained by different culturing approaches and assays targeting relevant physiological pathways.
Fractionation occurs during fixation of CO2 by ribulose-1,5-bisphosphate carboxylase/oxygenase (RubisCO) (Raven and Johnston, 1991), and is further dependent on the C-supply to this enzyme as well as the C-demand of the cells (Rau et al., 1996; Bidigare et al., 1997; Hoins et al., 2016a). RubisCO discriminates against the 13C isotope resulting in biomass being 13C-depleted relative to its source CO2. In higher plants, the intrinsic fractionation value of RubisCO (εf) is estimated to be 26 ‰–30 ‰ (Roeske and O'Leary, 1984; McNevin et al., 2007). However, εf can differ between phytoplankton taxa and species (e.g., Maberly et al., 1992; McNevin et al., 2007), and is indeed an important source of variation in 13C fractionation among phytoplankton groups. Several catalytically and phylogenetically distinct forms of RubisCO in phytoplankton exist, including forms IA, IB, ID and II (Whitney et al., 2011; Tabita et al., 2008). Direct in vitro measurements of εf yielded values of ∼ 11 ‰ for the haptophyte Emiliania huxleyi (Boller et al., 2011) and ∼ 18.5 ‰ for the diatom Skeletonema costatum (Boller et al., 2015). Much higher fractionation values have been estimated from in vivo experiments under nitrate-limited conditions, with values as high as ∼ 25 ‰ for the diatoms Phaeodactylum tricornutum and Porosira glacialis, and for E. huxleyi (Popp et al., 1998), and ∼ 27 ‰ for the dinoflagellate Alexandrium tamarense (Wilkes et al., 2017).
These large differences between phytoplankton groups and across treatments point towards physiological processes that can affect fractionation, notably those involved in so-called carbon concentrating mechanisms (CCMs). The CCMs have evolved over time as a response to declining atmospheric CO2 concentrations to ensure effective carboxylation in the vicinity of RubisCO in oxygenated waters (Giordano et al., 2005). Phytoplankton CCMs comprise a variety of physiological adaptations, and include active uptake of CO2 and HCO, the use of carbonic anhydrase (CA) to accelerate the interconversion between CO2 and HCO, and ways to minimize the CO2 efflux from the cell (Badger et al., 1998; Reinfelder, 2011; Rokitta et al., 2022). These processes can strongly influence 13C fractionation patterns of phytoplankton (Sharkey and Berry, 1985). For instance, HCO is enriched in 13C relative to CO2 (by ∼ 10 ‰), and a high uptake and assimilation of HCOcan therefore lower apparent 13C fractionation values. In addition, alterations in the CO2 efflux over total carbon uptake (i.e., leakage) also affect 13C fractionation, as faster replenishment of the intracellular CO2 pool prevents a build-up of 13CO2 and thus allows RubisCO to fully express its intrinsic fractionation. Different modes of CCMs are employed by different phytoplankton species, likely attributing to species-specific or group-specific differences in 13C fractionation (Badger et al., 1998; Van de Waal et al., 2019; Tortell, 2000).
The observed differences in εp between nutrient-limited and nutrient-replete cultures have been attributed to differences in the regulation of carbon uptake relative to carbon fixation (Laws et al., 2001). This variation may, at least partly, be caused by culturing methods, as chemostat cultures that were limited by nutrients or light showed similar responses of εp to changes in [CO2], while responses in light-controlled dilute batch cultures were markedly different (Laws et al., 2001). Likewise, discrepancies in measured δ13C values in different species of coccolithophores have been ascribed to varying culture methods, in particular to methods of CO2 manipulation (Liu et al., 2018; Hermoso et al., 2016). A recent study furthermore attributed differences in apparent fractionation to a regulatory CCM pathway upstream of RubisCO (Wilkes and Pearson, 2019). This pathway was suggested to alleviate excess photon flux when cells are nutrient-limited by shunting energy towards carbon uptake and hydroxylation reactions that increase εp.
Here, we aim to elucidate which underlying physiological processes cause the observed differences in 13C fractionation in phytoplankton under different [CO2], and how this is influenced by experimental settings. To this end, we collected data from all available culture studies, including a range of phytoplankton species from different groups, and evaluated systematic trends and offsets in 13C fractionation as a function of environmental, physiological, and experimental factors. This analysis compares the drivers behind phytoplankton 13C fractionation, assesses relations between 13C fractionation and culturing settings, and discusses implications for proxy development.
2.1 Literature review
We compiled data on 13C fractionation (εp) in phytoplankton species under a range of [CO2] and experimental conditions. A literature search was performed in Web of Science (https://www.webofknowledge.com/, last access: 25 February 2020) using the query “phytoplankton” OR “algae” OR “microalgae” OR “picoplankton”) AND “climate change” OR “ocean acidification” OR “CO2” OR “carbon dioxide” OR “global change” OR “pCO2” OR “carbonate chemistry” AND “13C fractionation” OR “εp” OR “carbon isotope” OR “isotope fractionation”. Data on 13C fractionation, growth rates (μ), and particulate organic carbon (POC) content under different experimental conditions and [CO2] were extracted using Engauge software when needed (Mitchell et al., 1991). To get an estimate of the carbon demands of the cells, we calculated POC production by multiplying the POC content with the instantaneous growth rate (μi). Using μi, we yield POC production that corresponds to the carbon fixation during the photoperiod (Riebesell et al., 2000a,b; Rost et al., 2002; Burkhardt et al., 1999a), and therefore corrects for difference in day length between studies. In addition, information was extracted on experimental settings (i.e., irradiance, light-dark cycle, salinity, temperature, nutrients), culturing approach (i.e., batch, chemostat, dilute batch, dilute chemostat), and type of carbonate chemistry manipulation resulting in different concentrations of dissolved inorganic carbon (DIC; i.e., aeration of culture with CO2, pre-aeration of culture medium with CO2) or total alkalinity (TA; i.e., acid or base addition). Under non-limiting growth conditions, δ13C of phytoplankton cells was measured during the exponential growth phase. The database includes only marine and estuarine phytoplankton species, with data acquired through single species culture experiments.
2.2 Statistical analyses
All analyses were performed in R version 4.0.3 (R Core Team, 2020). Significant differences in εp between different experimental conditions and culturing methods were calculated by means of a linear model followed by pairwise comparisons (Tukey method). To assess the relationship between εp and POC production [CO2], a linear model was fitted to the data for each of the distinct phytoplankton groups, and for each of the distinct species and study combinations. Data on POC production [CO2] was first log transformed, as this improved normality. To assess which of the influential conditions (i.e., nutrient conditions, carbonate chemistry manipulation method, culture approach, irradiance or light-dark cycle) could best explain the variation in εp, along with POC production/[CO2], we compared different models using the lmer function in R from the package “lme4” (Bates et al., 2015). In these models, POC production [CO2] and one of the influential conditions were fitted as fixed effects, including interaction terms, while species was fitted as a random intercept for each of the distinct phytoplankton groups (excluding cyanobacteria due to lack of data). Models were subsequently compared based on their Akaike Information Criterion (AIC) and Bayesian Information Criterion (BIC). For the different phytoplankton groups, we also tested how much explanatory power we could generate for the εp and POC production [CO2] relationship by including different environmental variables and using a multiple regression approach.
3.1 Dataset on 13C fractionation
The literature search yielded a total of 509 results, first titles and subsequently abstracts were reviewed, which led to a selection of 77 publications for screening. After careful screening for suitability, a total of 25 publications, containing 58 unique datasets, were included in our database. It contains data on four of the major marine phytoplankton groups, namely dinoflagellates (15 datasets), diatoms (24 datasets), haptophytes (17 datasets) and cyanobacteria (2 datasets).
Across all phytoplankton groups, there is a negative log-linear relationship between 13C fractionation (εp) and POC production over [CO2] (Fig. 1). This relationship is also apparent in each phytoplankton group (Fig. 2), although the slope of this curve varies between groups, and also strongly between species and studies (see also Fig. 3). As not all studies reported POC contents per cell, we also tested μi [CO2] to assess more species (especially for diatoms), finding similar pattern as POC production [CO2] (Fig. S1 in the Supplement). Across all phytoplankton groups, however, the explanatory power of the negative log-linear relationship between εp and μi/[CO2] was considerably smaller compared to the εp and POC production [CO2] relationship (Fig. S2). Within each phytoplankton group, the explanatory power of εp and μi [CO2] did hold up for diatoms and dinoflagellates but became insignificant for haptophytes (Fig. S3).
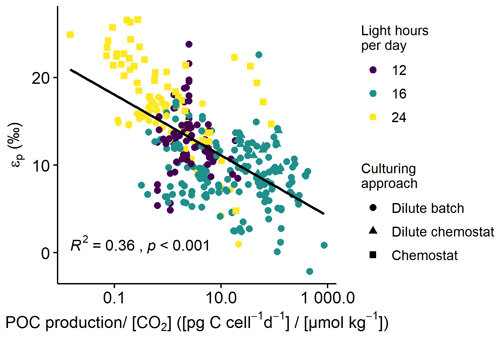
Figure 1εp against POC production [CO2] (C-demand/C-supply; log-transformed) across all phytoplankton groups. Colors indicate the light-dark cycle; marker shapes indicate the culturing approach. Black line illustrates the log-linear relationship (R2 and p-value indicated in the panel).
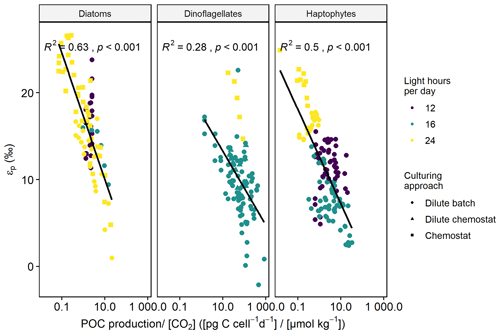
Figure 2εp against POC production CO2 (C-demand/C-supply; log-transformed) for the different phytoplankton groups, where the colored points indicate the respective light-dark cycle, and the shape of the points indicates the culturing approach. Black line illustrates the linear relationship (R2 and p-values indicated in the panels).
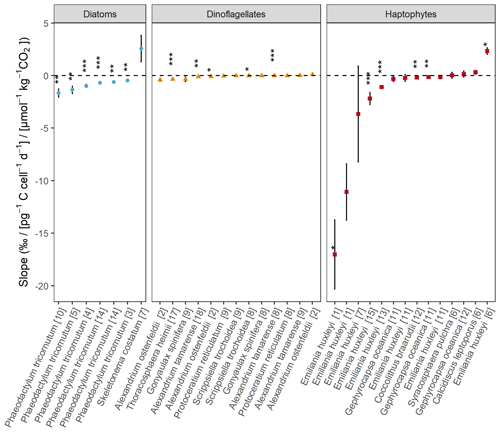
Figure 3Slopes of εp in response to POC production [CO2] for the different species and studies using a linear fit. Numbers between brackets refer to the different studies (Table S1). Blue dots represent diatoms, orange triangles dinoflagellates, and red squares haptophytes. Significance is indicated by the asterisks ( P < 0.001, P < 0.01, * P < 0.05).
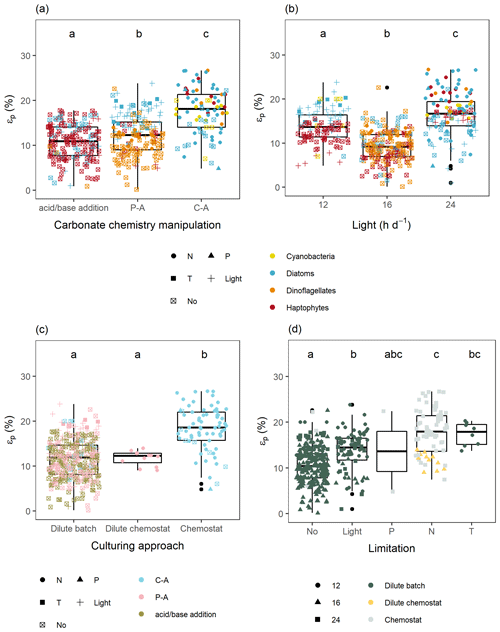
Figure 4Differences in εp between (a) type of carbonate chemistry manipulation (P-A is pre-aeration, and C-A is continuous aeration), (b) light-dark cycle, where colors indicate the different phytoplankton groups and shapes indicate type of limitation (N is nitrogen, P is phosphorus, T is temperature, and No is non-limited), (c) culturing approach, where colors indicate type of carbonate chemistry manipulations and shapes type of limitation again, and (d) type of limitation, where colors indicate culturing approach and shapes light hours per day. Significant differences between experimental conditions are indicated by the letters in the respective panels.
The explanatory power of the relationship between εp and POC production [CO2] within each phytoplankton group further increased when we included the amount of light hours per day, and the information whether there was nutrient limitation, yielding an R2 of 0.63 for diatoms (p < 0.001), 0.41 for dinoflagellates (p < 0.001), and 0.77 for haptophytes (p < 0.001).
3.2 Experimental settings and 13C fractionation
Some of the variation in εp can be explained by the different experimental settings between the studies (Fig. 4). For instance, phytoplankton grown under nitrogen limitation and lower temperatures show higher εp than those grown under light-controlled or non-limiting growth conditions (Fig. 4d). However, 13C fractionation also varied across the different types of carbonate chemistry manipulations and culturing approaches (Fig. 4a, c). Closed systems (i.e., pre-aeration with CO2 and acid or base addition) had lower εp values than open systems (continuous aeration with CO2), and cultures that were grown in chemostats with high biomass had higher overall εp values than those grown in dilute cultures. Moreover, light-dark cycle also strongly influences 13C fractionation, with cultures that experience continuous irradiation having higher εp values than cultures that are exposed to a dark-cycle (Fig. 4b). This was especially apparent for haptophytes and dinoflagellates, where cultures with continuous light grown in nitrogen-limited chemostats had higher εp values than those with a dark-cycle grown under replete dilute batch conditions (Fig. 2).
Some confounding experimental conditions across studies appear in our database. Notably, nutrient limitation experiments are almost always performed in chemostats with continuous aeration and without a light-dark cycle. In addition, non-limiting or light-controlled culture studies, with a light-dark cycle, are almost entirely performed using a dilute batch, with pre-aeration or acid or base addition (Fig. 4). To tease apart which of these confounding factors (i.e., nutrient conditions, type of carbonate chemistry manipulation, culturing approach, and light-dark cycle) can best explain the differences in εp, besides C-demand/C-supply, we compared different models including POC production [CO2] and one of the variables for each of the distinct phytoplankton groups (Tables S2–S4). For haptophytes, inclusion of light-dark cycle could best explain the data (AIC 711 and BIC 738), while the culturing approach yielded the best results for dinoflagellates (AIC 490 and BIC 520), and for diatoms this was either the culturing approach (AIC 600) or the method of carbonate chemistry manipulation (BIC 623).
In our analyses of the current literature on εp responses, we observed a high dependence on C-demand/C-supply (i.e., POC production [CO2]) across and within different phytoplankton groups (Figs. 1, 2), where the inclusion of light regime and nutrient limitation further increased the explanatory power of this relationship. The correction step for C-demand (as compared to growth rate) is essential, as already identified in previous work, because different growth rates and cellular carbon contents reflect the different C requirements of phytoplankton cells (Rau et al., 1996; Bidigare et al., 1997; Hoins et al., 2016b). Moreover, estimating C requirements based on instantaneous growth rates alone is not sufficient to reflect C-demand, especially for haptophytes (Figs. S2, S3). Variation in the εp relationship with POC production [CO2] was, however, observed between the different species and studies (Fig. 3). Next to species-specific differences, this may be attributed to the contrasting experimental settings and culture methods. In the following, we discuss the variation in fractionation patterns between the phytoplankton species and groups, highlighting the potential role of CCMs, how different experimental settings may result in isotopic disequilibrium conditions, and the implications for CO2 proxies based on carbon isotope fractionation.
4.1 Fractionation patterns and underlying processes
Across and within phytoplankton groups, the relationship between εp and POC production [CO2] follows a decay function (i.e., see untransformed data Fig. S4), which highlights the active role of CCMs in C uptake in all groups. If species relied on diffusive CO2 uptake alone, a more linear relationship can be expected. The presence of CCMs is further supported by some of the low εp signals (Fig. 1), indicating a higher contribution of HCO to C fixation and/or decreased leakage. Intrinsic RubisCO fractionation values (εf) of 18 ‰ and 11 ‰ were measured in diatoms and haptophytes, respectively (Boller et al., 2011, 2015). The εp values exceeding εf for both of these groups, and possibly also for dinoflagellates, therefore remain puzzling and indicate fractionation steps occurring upstream of RubisCO (Wilkes and Pearson, 2019).
In cyanobacteria, CO2 fixation by RubisCO takes place in the carboxysome, which is a distinct cellular compartment. The membrane of this compartment prevents diffusion of CO2, while it is permeable for HCO which is converted to CO2 via carboxysomal CA, thereby accumulating CO2 in the vicinity of RubisCO (Espie and Kimber, 2011; Dou et al., 2008; Price et al., 2008). To prevent CO2 efflux out of the cell, and likewise facilitate diffusive CO2 uptake, cytosolic CO2 is actively converted to HCO by the NAD(P)H dehydrogenase (NDH) complex in the cytoplasm (Price et al., 2002; Maeda et al., 2002). It was proposed that these specific processes modify and in fact raise εp values in cyanobacteria. A strong disequilibrium in the cytosol may, for instance, favor a unidirectional conversion of CO2 to HCO that would result in an additional fractionation step of at least ∼ 13 ‰ (O'Leary et al., 1992), and potentially up to 20 ‰–33 ‰ (Zeebe and Wolf-Gladrow, 2001; Zeebe, 2014; Siegenthaler and Münnich, 1981; Clark and Lauriol, 1992). If this conversion step is furthermore mediated by NDH, this enzyme will likely discriminate against 13C resulting in additional fractionation (Eichner et al., 2015). Overall, this “internal C-cycling” around NDH would yield higher εp values than otherwise expected based on the CO2 and HCO fluxes over the plasma membrane assuming equilibrium (Eichner et al., 2015; Sharkey and Berry, 1985).
Similar strategies may be present in the other algal groups that increase εp. Effective CO2 fixation in diatoms relies on biophysical CCMs that facilitate or actively transport CO2 and HCO3 through a 4-layered chloroplast membrane system (Keeling, 2013), which principally makes the uptake more challenging but also confers additional control on the DIC fluxes (Matsuda et al., 2017; Nakajima et al., 2013). Numerous subcellular localized CAs are present in diatoms, which accelerate the interconversion of CO2 and HCO, also within the pyrenoid, where RubisCO is localized (Tachibana et al., 2011; Kikutani et al., 2016; Samukawa et al., 2014). Chemical disequilibrium environments between compartments, unidirectional conversion of CAs, or 13C discrimination associated with HCO transporters (solute carrier type transporters; SLC) may represent additional, but likely small sources of 13C fractionation for diatoms.
No internal membrane systems with localized CAs associated to C fixation as present in diatoms have been recognized in haptophytes and dinoflagellates (Rokitta et al., 2022). In fact, some dinoflagellate species even lack the pyrenoid compartment, where RubisCO is located in most eukaryotic algae (Ratti et al., 2007). The contribution of HCO to photosynthesis is high in both groups (Rost et al., 2006; Rokitta and Rost, 2012; Bach et al., 2013; McClelland et al., 2017), and fractionation due to chemical disequilibria within the cell can therefore occur to some degree, e.g., by favoring unidirectional conversion of CO2 to HCO and vice versa. However, stronger internal C-cycling to maintain high CO2 accumulation in the proximity of RubisCO by decreasing CO2 leakage from the cell (Cassar et al., 2006; Schulz et al., 2007; Eichner et al., 2015; Hoins et al., 2016a) and higher contribution of HCO to net C fixation generally lead to higher build-up of 13C within the cell (i.e., stronger internal Rayleigh fractionation) and consequently lower εp values. Thus, while described modes of CCMs for the different groups are mostly in line with observed fractionation patterns (Schulz et al., 2007; Eichner et al., 2015; Hoins et al., 2016a; McClelland et al., 2017), εp values exceeding the intrinsic fractionation of RubisCO remain puzzling.
Wilkes and Pearson (2019) recently proposed that certain components of the CCM are differently regulated in nutrient-limited, light-replete cultures compared to light-controlled cultures, which could explain the often observed differences in εp patterns between chemostat and dilute batch cultures (Fig. 4), and likewise reconcile why εp values can exceed εf estimates under some conditions. More specifically, the authors suggested that when cells are nutrient-limited they can experience excess photon flux, which may be alleviated through fueling photocatalytic dehydration reactions of HCO by internal CAs localized in the thylakoid lumen. The acidic environment in the thylakoid favors the unidirectional conversion of HCO to CO2, while the alkaline environment in the chloroplast favors the unidirectional conversion of CO2 to HCO. Light-induced stimulation of these processes may increase fractionation due to unidirectional hydration of CO2 and dehydration of HCO (up to ∼ 25 ‰ and ∼ 34 ‰, respectively; Wilkes and Pearson, 2019). However, the proposed εp difference between light-limited and nutrient-limited cultures was not consistently found (Fig. 4; Laws et al., 2001; Hoins et al., 2016a). Moreover, our results suggest this “light-driven CCM” activity stems from the absence of a light-dark cycle during culture growth rather than from nutrient or light limitation (Figs. 1, 4b). This was especially the case for haptophytes and dinoflagellates, where εp values were consistently elevated under continuous irradiance (Fig. 2). In addition to differences in light-dark cycle, other culturing variables also differed between the studies reviewed by Wilkes and Pearson (2019).
4.2 Experimental settings and resulting isotopic disequilibria
Studies yielding exceptionally high εp values (apparently higher than εf) were, next to nutrient limitation and continuous irradiance, also performed in high-biomass chemostats under continuous aeration with CO2 (Fig. 4). In haptophytes, the light-dark cycle could, next to POC production [CO2], best explain differences in εp (Table S2). The important role of light on fractionation was already discussed by Rost et al. (2002), and more recently highlighted by Phelps et al. (2021). They found that in coccolithophores, εp depended more strongly on light intensity and light-dark cycle than on CO2 concentrations (higher εp values with more light exposure), even when corrected for C-demands (Phelps et al., 2021; Rost et al., 2002). While continuous light led to higher εp also in one dinoflagellate and several diatoms (Burkhardt et al., 1999b; Wilkes et al., 2017), the light-dependency of εp seems strongest in haptophytes and may thus relate to changes in C flow between photosynthetic C fixation and calcification under changing light conditions (Krumhardt et al., 2017; Bolton and Stoll, 2013; Phelps et al., 2021). For instance, day length had a significant influence on εp of E. huxleyi (up to 8 ‰), as the preferred carbon source shifted from CO2 under continuous light to HCO uptake under light-dark cycles (Rost et al., 2002, 2006). However, not all phytoplankton species' fractionation responds similarly to changes in day length. For two diatoms and one dinoflagellate species, for instance, εp values were similar for cultures grown under continuous light or light-dark cycles (Burkhardt et al., 1999b).
In diatoms and dinoflagellates, the culturing approach or method of carbonate chemistry manipulation was, next to POC production [CO2], the best predictor of changes in εp (Tables S3, S4). Rost et al. (2008) pointed out that, next to aspects of the CCM itself, different carbonate chemistry manipulations and culturing methods can lead to different CO2-dependencies between studies and different experimental set-ups.
Importantly, in the calculations for the δ13C of CO2 and thus also εp, chemical and isotopic equilibrium is assumed. In “open” carbonate chemistry systems with a continuous supply of CO2, however, an equilibrium situation may not yet be reached before phytoplankton assimilate carbon (Zeebe et al., 1999; Rost et al., 2008), which is even more so in the case of high-biomass cultures. Recent work from Zhang et al. (2022) showed that it takes much longer (several hours to days) for an isotopic equilibrium to be reached in empty algal culturing vessels than a chemical equilibrium and that this should be considered in εp calculations. This discrepancy could lead to an overestimation of εp in “open” carbonate chemistry systems compared to “closed” carbonate chemistry systems, which has been set up by pre-aeration with a certain [CO2] or by acid or base additions, such as observed in Fig. 4c. This may especially be true for cultures with high CO2 treatments (high carbon supply) and high overall carbon demands (high biomass), as both favor disequilibrium situations (see Fig. S5 for example of the dinoflagellate Alexandrium tamarense). In chemostats that were run with low cell densities, on the other hand, isotopic disequilibria may not play a role and therefore yield similar εp values comparable to dilute batch studies (Fig. 4c). Hence, biases in εp values introduced by isotopic disequilibria can be misinterpreted as treatment effects, e.g., as an effect of nitrate-limited vs. light-limited growth. Moreover, chemostat systems that are maintained with high biomass, even though they are meant to mimic oligotrophic systems, are not representative for natural environments as these systems support only low biomass concentrations (Van de Waal et al., 2014).
4.3 Implications for proxies
The extent to which experiments reflect natural conditions is important regarding proxy development, as they feed the mechanistic model of CO2-dependent carbon isotope fractionation and confounding factors. A more standardized approach in performing these types of experiments, e.g. representative of natural light settings, using only one type of carbonate chemistry manipulation, and maintaining cultures at low biomass, would already substantially reduce variation in the CO2-dependent εp responses between studies (Fig. 3). Both species-specific differences and the effects of drivers (nutrient limitation, temperature conditions, etc.) would then be more straightforward to distinguish, as the study design would not interfere as a concomitant source of εp variation (Fig. 4). We note, however, that even when studies use comparable methods, findings can still vary. For example, not all phytoplankton strains tested showed a negative relationship between εp and POC production [CO2] (Fig. 3), meaning that other environmental factors can mask the CO2 dependence, which urges for caution when using 13C fractionation during photosynthetic C fixation as a CO2 proxy. Quantitative constraints on these confounding factors are crucial to guarantee that reconstructed signals exceed the related uncertainties.
Quantitative constraints on physiological variables, implied to be growth rate and cell geometry, but also membrane permeability to CO2, and the boundary layer thickness dependent on temperature, pH, and salinity, are in place in CO2 proxy work with a catch-all term called b (Popp et al., 1998; Rau et al., 1996; Jasper and Hayes, 1990; Bolton et al., 2016; Stoll et al., 2019). The b value is often linearly correlated with modern dissolved reactive phosphate concentration in surface seawater (Bice et al., 2006; Bidigare et al., 1997; Pagani et al., 2005), as phosphate is a major nutrient that often co-limits with other important micronutrients such as iron, zinc, and cobalt, which affect phytoplankton growth rate and cell size (Bidigare et al., 1997). However, this is quite a simplistic view and b can vary substantially over time and between locations (Zhang et al., 2019).
Better constraints on b may further advance CO2 proxy development based on specific algal biomarkers, although this remains a challenge due to the sparsity of useful parameters on confounding factors in the paleo-environment. This is especially true for biomarker proxies, such as phytane and alkenones, as these biomarkers are produced by multiple species, certainly through geological time (Witkowski et al., 2018). These species may have had different modes of carbon acquisition, growth rates and cellular carbon contents, and discrepancies between alkenone proxy and ice core records were also attributed to CCM activity (Badger et al., 2019; Badger, 2021). The preference for more specific proxy work is also needed as the analyzed phytoplankton groups show different slopes for the εp versus POC production [CO2] relationship (Fig. 2). However, a better selection of study sites (i.e., located in more productive ocean regions with possibly similarly responsive species as well) can reproduce CO2 estimates that are in agreement with the ice core records even with constant b values (Zhang et al., 2019). Moreover, including estimations of light regime and nutrient status may further improve CO2 estimates based on algal proxies.
Another phytoplankton group and even species-specific line of CO2 proxies under development is the 13C fractionation of dinoflagellate resting cysts (Hoins et al., 2015; Sluijs et al., 2018). Single cysts can be analyzed with a recent analytical set-up (van Roij et al., 2017), which provides the advantage that specific species can be selected so that an estimate of cell size can be made. Vegetative cell sizes of dinoflagellates generally correspond to sizes of their resting cysts (Finkel et al., 2007). Cyst size may be used to infer cellular carbon contents, and together with phosphate concentrations for growth rate, can give a better estimate for carbon demands and therefore improve the constraints of b in this line of proxies.
Our results illustrate that the POC production [CO2]-dependency of εp can vary significantly between different phytoplankton species and groups, but also as a result of different culturing methods and differences in day length, especially for haptophytes. Extrapolating results to natural environments and for proxy applications therefore requires caution, and it should be carefully considered if culture methods and experimental conditions are representative of natural environments. Better approximations for carbon demands (described by μi and POC contents) in εp-based CO2 proxies could also greatly improve their estimates. This will be challenging in the paleo-environment, especially with proxies that rely on biomarkers. Alternatively, careful selection of sites with more similar environments and phytoplankton species could also further improve proxy estimates.
All data presented in this study are available in the open data repository Dryad (https://doi.org/10.5061/dryad.hmgqnk9k8, Brandenburg, 2022).
The supplement related to this article is available online at: https://doi.org/10.5194/bg-19-3305-2022-supplement.
All authors contributed to the study design. Preliminary data for the study were collected by MH. KMB collected additional data, performed statistical analyses and wrote the first draft. All authors discussed the results and AS, MH, DBvdW, and BR provided feedback on the manuscript.
The contact author has declared that neither they nor their co-authors have any competing interests.
Publisher’s note: Copernicus Publications remains neutral with regard to jurisdictional claims in published maps and institutional affiliations.
The authors thank Gert-Jan Reichart for constructive discussions that helped shape the presented ideas and are grateful for the constructive comments received from two reviewers. Appy Sluijs thanks the European Research Council for Consolidator Grant 771497.
This research has been supported by the H2020 European Research Council (SPANC, grant no. 771497).
This paper was edited by Tina Treude and reviewed by two anonymous referees.
Bach, L. T., MacKinder, L. C. M., Schulz, K. G., Wheeler, G., Schroeder, D. C., Brownlee, C., and Riebesell, U.: Dissecting the impact of CO2 and pH on the mechanisms of photosynthesis and calcification in the coccolithophore Emiliania huxleyi, New Phytol., 199, 121–134, 2013.
Badger, M. P. S.: Alkenone isotopes show evidence of active carbon concentrating mechanisms in coccolithophores as aqueous carbon dioxide concentrations fall below 7 µmol L−1, Biogeosciences, 18, 1149–1160, https://doi.org/10.5194/bg-18-1149-2021, 2021.
Badger, M. P. S., Chalk, T. B., Foster, G. L., Bown, P. R., Gibbs, S. J., Sexton, P. F., Schmidt, D. N., Pälike, H., Mackensen, A., and Pancost, R. D.: Insensitivity of alkenone carbon isotopes to atmospheric CO2 at low to moderate CO2 levels, Clim. Past, 15, 539–554, https://doi.org/10.5194/cp-15-539-2019, 2019.
Badger, M. R., Andrews, T. J., Whitney, S. M., Ludwig, M., Yellowlees, D. C., Leggat, W., and Price, G. D.: The diversity and coevolution of Rubisco, plastids, pyrenoids, and chloroplast-based CO2-concentrating mechanisms in algae, Can. J. Botany, 76, 1052–1071, 1998.
Bates, D., Machler, M., Bolker, B., and Walker, S.: Fitting linear mixed-effects models using lme4, J. Stat. Softw., 67, 1–48, 2015.
Bice, K. L., Birgel, D., Meyers, P. A., Dahl, K. A., Hinrichs, K. U., and Norris, R. D.: A multiple proxy and model study of Cretaceous upper ocean temperatures and atmospheric CO2 concentrations, Paleoceanography, 21, 1–17, 2006.
Bidigare, R., Freeman, H., Hanson, L., Hayes, M., Jasper, P., King, L., Millero, J., Popp, N., Steinberg, A., and Wakeham, G.: Consistent fractionation of 13C in nature and in the laboratory: growth-rate effects in some haptophyte algae, Global Biogeochem. Cy., 11, 279–292, 1997.
Brandenburg, K. M.: Physiological control on carbon isotope fractionation in marine phytoplankton, Dryad [data set], https://doi.org/10.5061/dryad.hmgqnk9k8, 2022.
Boller, A. J., Thomas, P. J., Cavanaugh, C. M., and Scott, K. M.: Low stable carbon isotope fractionation by coccolithophore RubisCO, Geochim. Cosmochim. Ac., 75, 7200–7207, 2011.
Boller, A. J., Thomas, P. J., Cavanaugh, C. M., and Scott, K. M.: Isotopic discrimination and kinetic parameters of RubisCO from the marine bloom-forming diatom, Skeletonema costatum, Geobiology, 13, 33–43, 2015.
Bolton, C. T. and Stoll, H. M.: Late Miocene threshold response of marine algae to carbon dioxide limitation, Nature, 500, 558–562, 2013.
Bolton, C. T., Hernández-Sánchez, M. T., Fuertes, M. Á., González-Lemos, S., Abrevaya, L., Mendez-Vicente, A., Flores, J. A., Probert, I., Giosan, L., Johnson, J., and Stoll, H. M.: Decrease in coccolithophore calcification and CO2 since the middle Miocene, Nat. Commun., 7, 10284, https://doi.org/10.1038/ncomms10284, 2016.
Burkhardt, S., Riebesell, U., and Zondervan, I.: Effects of growth rate, CO2 concentration, and cell size on the stable carbon isotope fractionation in marine phytoplankton, Geochim. Cosmochim. Ac., 63, 3729–3741, 1999a.
Burkhardt, S., Riebesell, U., and Zondervan, I.: Stable carbon isotope fractionation by marine phytoplankton in response to daylength, growth rate, and CO2 availability, Mar. Ecol.-Prog. Ser., 184, 31–41, 1999b.
Cassar, N., Laws, E. A., and Popp, B. N.: Carbon isotopic fractionation by the marine diatom Phaeodactylum tricornutum under nutrient- and light-limited growth conditions, Geochim. Cosmochim. Ac., 70, 5323–5335, 2006.
Clark, I. D. and Lauriol, B.: Kinetic enrichment of stable isotopes in cryogenic calcites, Chem. Geol., 102, 217–228, 1992.
Dou, Z., Heinhorst, S., Williams, E. B., Murin, C. D., Shively, J. M., and Cannon, G. C.: CO2 fixation kinetics of Halothiobacillus neapolitanus mutant carboxysomes lacking carbonic anhydrase suggest the shell acts as a diffusional barrier for CO2, J. Biol. Chem., 283, 10377–10384, 2008.
Eichner, M., Thoms, S., Kranz, S. A., and Rost, B.: Cellular inorganic carbon fluxes in Trichodesmium: Aa combined approach using measurements and modelling, J. Exp. Bot., 66, 749–759, 2015.
Espie, G. S. and Kimber, M. S.: Carboxysomes: cyanobacterial RubisCO comes in small packages, Photosynth. Res., 109, 7–20, 2011.
Farquhar, G. D., Ehleringer, J. R., and Hubick, K. T.: Carbon isotope discrimination and photosynthesis, Annu. Rev. Plant Phys., 40, 503–537, 1989.
Finkel, Z. V., Sebbo, J., Feist-Burkhardt, S., Irwin, A. J., Katz, M. E., Schofield, O. M. E., Young, J. R., and Falkowski, P. G.: A universal driver of macroevolutionary change in the size of marine phytoplankton over the Cenozoic, P. Natl. Acad. Sci. USA, 104, 20416–20420, 2007.
Foster, G. L., Royer, D. L., and Lunt, D. J.: Future climate forcing potentially without precedent in the last 420 million years, Nat. Commun., 8, 1–8, 2017.
Freeman, H. and Hayes, J. M.: Fractionation of carbon isotopes by phytoplankton and estimates of ancient CO2 levels, Global Biogeochem. Cy., 6, 185–198, 1992.
Giordano, M., Beardall, J., and Raven, J. A.: CO2 concentrating mechanisms in algae: mechanisms, environmental modulation, and evolution, Annu. Rev. Plant Biol., 56, 99–131, 2005.
Hayes, J. M., Strauss, H., and Kaufman, A. J.: The abundance of 13C in marine organic matter and isotopic fractionation in the global biogeochemical cycle of carbon during the past 800 Ma, Chem. Geol., 161, 103–125, 1999.
Hermoso, M., Chan, I. Z. X., McClelland, H. L. O., Heureux, A. M. C., and Rickaby, R. E. M.: Vanishing coccolith vital effects with alleviated carbon limitation, Biogeosciences, 13, 301–312, https://doi.org/10.5194/bg-13-301-2016, 2016.
Hoins, M., Van de Waal, D. B., Eberlein, T., Reichart, G. J., Rost, B., and Sluijs, A.: Stable carbon isotope fractionation of organic cyst-forming dinoflagellates: evaluating the potential for a CO2 proxy, Geochim. Cosmochim. Ac., 160, 267–276, 2015.
Hoins, M., Eberlein, T., Van de Waal, D. B., Sluijs, A., Reichart, G. J., and Rost, B.: CO2-dependent carbon isotope fractionation in dinoflagellates relates to their inorganic carbon fluxes, J. Exp. Mar. Biol. Ecol., 481, 9–14, 2016a.
Hoins, M., Eberlein, T., Großmann, C. H., Brandenburg, K., Reichart, G.-J., Rost, B., Sluijs, A., and Van De Waal, D. B.: Combined effects of ocean acidification and light or nitrogen availabilities on 13C fractionation in marine dinoflagellates, PLoS One, 11, e0154370, https://doi.org/10.1371/journal.pone.0154370, 2016b.
Jasper, J. P. and Hayes, J. M.: A carbon isotope record of CO2 levels during the late Quaternary, Nature, 347, 462–464, 1990.
Keeling, P. J.: The number, speed, and impact of plastid endosymbioses in eukaryotic evolution, Annu. Rev. Plant Biol., 64, 583–607, 2013.
Kikutani, S., Nakajima, K., Nagasato, C., Tsuji, Y., Miyatake, A., and Matsuda, Y.: Thylakoid luminal Θ-carbonic anhydrase critical for growth and photosynthesis in the marine diatom Phaeodactylum tricornutum, P. Natl. Acad. Sci. USA, 113, 9828–9833, 2016.
Krumhardt, K. M., Lovenduski, N. S., Iglesias-Rodriguez, M. D., and Kleypas, J. A.: Coccolithophore growth and calcification in a changing ocean, Prog. Oceanogr., 159, 276–295, 2017.
Laws, E. A., Popp, B. N., Bidigare, R. R., Riebesell, U., Burkhardt, S., and Wakeham, S. G.: Controls on the molecular distribution and carbon isotopic composition of alkenones in certain haptophyte algae, Geochem. Geophy. Geosy., 2, 2000GC000057, https://doi.org/10.1029/2000GC000057, 2001.
Liu, Y. W., Eagle, R. A., Aciego, S. M., Gilmore, R. E., and Ries, J. B.: A coastal coccolithophore maintains pH homeostasis and switches carbon sources in response to ocean acidification, Nat. Commun., 9, 1–12, 2018.
Maberly, S. C., Raven, J. A., and Johnston, A. M.: Discrimination between 12C and 13C by marine plants, Oecologia, 91, 481–492, 1992.
Macdonald, F. A.: Deep-time paleoclimate proxies, AGU Adv., 1, 1–3, 2020.
Maeda, S. I., Badger, M. R., and Price, G. D.: Novel gene products associated with NdhD3/D4-containing NDH-1 complexes are involved in photosynthetic CO2 hydration in the cyanobacterium, Synechococcus sp. PCC7942, Mol. Microbiol., 43, 425–435, 2002.
Matsuda, Y., Hopkinson, B. M., Nakajima, K., Dupont, C. L., and Tsuji, Y.: Mechanisms of carbon dioxide acquisition and CO2 sensing in marine diatoms: a gateway to carbon metabolism, Philos. T. R. Soc. B, 372, 20160403, https://doi.org/10.1098/rstb.2016.0403, 2017.
McClelland, H. L. O., Bruggeman, J., Hermoso, M., and Rickaby, R. E. M.: The origin of carbon isotope vital effects in coccolith calcite, Nat. Commun., 8, 14511, https://doi.org/10.1038/ncomms14511, 2017.
McNevin, D. B., Badger, M. R., Whitney, S. M., Von Caemmerer, S., Tcherkez, G. G. B., and Farquhar, G. D.: Differences in carbon isotope discrimination of three variants of D-ribulose-1,5-bisphosphate carboxylase/oxygenase reflect differences in their catalytic mechanisms, J. Biol. Chem., 282, 36068–36076, 2007.
Mitchell, M., Muftakhidinov, B., and Winchen, T.: Engauge Digitizer 4.1, Zenodo, https://doi.org/10.5281/zenodo.3941227, 2020.
Nakajima, K., Tanaka, A., and Matsuda, Y.: SLC4 family transporters in a marine diatom directly pump bicarbonate from seawater, P. Natl. Acad. Sci. USA, 110, 1767–1772, 2013.
O'Leary, M. H.: Measurement of the isotope fractionation associated with diffusion of carbon dioxide in aqueous solution, J. Phys. Chem., 88, 823–825, 1984.
O'Leary, M. H., Madhavan, S., and Paneth, P.: Physical and chemical basis of carbon isotope fractionation in plants, Plant. Cell Environ., 15, 1099–1104, 1992.
Pagani, M., Zachos, J. C., Freeman, K. H., Tipple, B., and Bohaty, S.: Atmospheric science: marked decline in atmospheric carbon dioxide concentrations during the Paleogene, Science, 309, 600–603, 2005.
Phelps, S. R., Hennon, G. M. M., Dyhrman, S. T., Hernández Limón, M. D., Williamson, O. M., and Polissar, P. J.: Carbon isotope fractionation in Noelaerhabdaceae algae in culture and a critical evaluation of the alkenone paleobarometer, Geochem. Geophy. Geosy., 22, 1–20, 2021.
Popp, B. N., Laws, E. A., Bidigare, R. R., Dore, J. E., Hanson, K. L., and Wakeham, S. G.: Effect of phytoplankton cell geometry on carbon isotopic fractionation, Geochim. Cosmochim. Ac., 62, 69–77, 1998.
Price, G. D., Maeda, S. I., Omata, T., and Badger, M. R.: Modes of active inorganic carbon uptake in the cyanobacterium, Synechococcus sp. PCC7942, Funct. Plant Biol., 29, 131–149, 2002.
Price, G. D., Badger, M. R., Woodger, F. J., and Long, B. M.: Advances in understanding the cyanobacterial CO2-concentrating- mechanism (CCM): Functional components, Ci transporters, diversity, genetic regulation and prospects for engineering into plants, J. Exp. Bot., 59, 1441–1461, 2008.
Ratti, S., Giordano, M., and Morse, D.: CO2-concentrating mechanisms of the potentially toxic dinoflagellate Protoceratium reticulatum (Dinophyceae, Gonyaulacales), J. Phycol., 43, 693–701, 2007.
Rau, G. H., Riebesell, U., and Wolf-Gladrow, D.: A model of photosynthetic 13C fractionation by marine phytoplankton based on diffusive molecular CO2 uptake, Mar. Ecol.-Prog. Ser., 133, 275–285, 1996.
Raven, J. A. and Johnston, A.: Mechanisms of inorganic-carbon acquisition in marine phytoplankton and their implications for the use of other resources, Limnol. Oceanogr., 36, 1701–1714, 1991.
R Core Team: R: A language and environment for statistical computing. R Foundation for Statistical Computing, Vienna, Austria, https://www.R-project.org/ (last access: January 2022), 2020.
Reinfelder, J. R.: Carbon concentrating mechanisms in eukaryotic marine phytoplankton, Ann. Rev. Mar. Sci., 3, 291–315, 2011.
Riebesell, U., Burkhardt, S., Dauelsberg, A., and Kroon, B.: Carbon isotope fractionation by a marine diatom: dependence on the growth-rate-limiting resource, Mar. Ecol.-Prog. Ser., 193, 295–303, 2000a.
Riebesell, U., Revill, A. T., Holdsworth, D. G., and Volkman, J. K.: The effects of varying CO2 concentration on lipid composition and carbon isotope fractionation in Emiliania huxleyi, Geochim. Cosmochim. Ac., 64, 4179–4192, 2000b.
Roeske, C. A. and O'Leary, M. H.: Carbon isotope effects on the enzyme-catalyzed carboxylation of ribulose bisphosphate, Biochemistry, 23, 6275–6284, 1984.
Rohling, E. J., Sluijs, A., Dijkstra, H. A., Köhler, P., Van De Wal, R. S. W., Von Der Heydt, A. S., Beerling, D. J., Berger, A., Bijl, P. K., Crucifix, M., Deconto, R., Drijfhout, S. S., Fedorov, A., Foster, G. L., Ganopolski, A., Hansen, J., Hönisch, B., Hooghiemstra, H., Huber, M., Huybers, P., Knutti, R., Lea, D. W., Lourens, L. J., Lunt, D., Masson-Demotte, V., Medina-Elizalde, M., Otto-Bliesner, B., Pagani, M., Pälike, H., Renssen, H., Royer, D. L., Siddall, M., Valdes, P., Zachos, J. C., and Zeebe, R. E.: Making sense of palaeoclimate sensitivity, Nature, 491, 683–691, 2012.
Rokitta, S. D. and Rost, B.: Effects of CO2 and their modulation by light in the life-cycle stages of the coccolithophore Emiliania huxleyi, Limnol. Oceanogr., 57, 607–618, 2012.
Rokitta, S. D., Kranz, S. A., and Rost, B.: Inorganic carbon acquisition by aquatic primary producers, in: Blue planet, red and green photosynthesis, edited by: Maberly, S. and Gontero, B., ISTE Ltd, London, ISBN 9-781-78945-082-8, 2022.
Rost, B., Zondervan, I., and Riebesell, U.: Light-dependent carbon isotope fractionation in the coccolithophorid Emiliania huxleyi, Limnol. Oceanogr., 47, 120–128, 2002.
Rost, B., Riebesell, U., and Sültemeyer, D.: Carbon acquisition of marine phytoplankton: effect of photoperiod length, Limnol. Oceanogr., 51, 12–20, 2006.
Rost, B., Zondervan, I., and Wolf-Gladrow, D.: Sensitivity of phytoplankton to future changes in ocean carbonate chemistry: current knowledge, contradictions and research directions, Mar. Ecol.-Prog. Ser., 373, 227–237, 2008.
Samukawa, M., Shen, C., Hopkinson, B. M., and Matsuda, Y.: Localization of putative carbonic anhydrases in the marine diatom, Thalassiosira pseudonana, Photosynth. Res., 121, 235–249, 2014.
Schulz, K. G., Rost, B., Burkhardt, S., Riebesell, U., Thoms, S., and Wolf-Gladrow, D. A.: The effect of iron availability on the regulation of inorganic carbon acquisition in the coccolithophore Emiliania huxleyi and the significance of cellular compartmentation for stable carbon isotope fractionation, Geochim. Cosmochim. Ac., 71, 5301–5312, 2007.
Sharkey, T. D. and Berry, J. A.: Carbon isotope fractionation of algae as influenced by an inducible CO2 concentrating mechanism, in: Inorganic Carbon Uptake by Aquatic Photosynthetic Organisms, edited by: Lucas, W. J. and Berry, J. A., American Society of Plant Physiologists, 389–401, ISBN 10 0943088054, ISBN 13 9780943088051, 1985.
Siegenthaler, U. and Münnich, K. O.: 12C 13C fractionation during CO2 transfer from air to sea, Carbon Cycle Model., 249–251, 1981.
Sluijs, A., van Roij, L., Frieling, J., Laks, J., and Reichart, G. J.: Single-species dinoflagellate cyst carbon isotope ecology across the Paleocene-Eocene Thermal Maximum, Geology, 46, 79–82, 2018.
Stoll, H. M., Guitian, J., Hernandez-Almeida, I., Mejia, L. M., Phelps, S., Polissar, P., Rosenthal, Y., Zhang, H., and Ziveri, P.: Upregulation of phytoplankton carbon concentrating mechanisms during low CO2 glacial periods and implications for the phytoplankton pCO2 proxy, Quaternary Sci. Rev., 208, 1–20, 2019.
Tabita, F. R., Satagopan, S., Hanson, T. E., Kreel, N. E., and Scott, S. S.: Distinct form I, II, III, and IV Rubisco proteins from the three kingdoms of life provide clues about Rubisco evolution and structure/function relationships, J. Exp. Bot., 59, 1515–1524, 2008.
Tachibana, M., Allen, A. E., Kikutani, S., Endo, Y., Bowler, C., and Matsuda, Y.: Localization of putative carbonic anhydrases in two marine diatoms, Phaeodactylum tricornutum and Thalassiosira pseudonana, Photosynth. Res., 109, 205–221, 2011.
Tierney, J. E., Poulsen, C. J., Montañez, I. P., Bhattacharya, T., Feng, R., Ford, H. L., Hönisch, B., Inglis, G. N., Petersen, S. V., Sagoo, N., Tabor, C. R., Thirumalai, K., Zhu, J., Burls, N. J., Foster, G. L., Goddéris, Y., Huber, B. T., Ivany, L. C., Turner, S. K., Lunt, D. J., McElwain, J. C., Mills, B. J. W., Otto-Bliesner, B. L., Ridgwell, A., and Zhang, Y. G.: Past climates inform our future, Science, 370, eaay3701, https://doi.org/10.1126/science.aay3701, 2020.
Tortell, P. D.: Evolutionary and ecological perspectives on carbon acquisition in phytoplankton, Limnol. Oceanogr., 45, 744–750, 2000.
Van De Waal, D. B., Eberlein, T., Bublitz, Y., John, U., and Rost, B.: Shake it easy: a gently mixed continuous culture system for dinoflagellates, J. Plankton Res., 36, 889–894, 2014.
Van de Waal, D. B., Brandenburg, K. M., Keuskamp, J., Trimborn, S., Rokitta, S., Kranz, S., and Rost, B.: Highest plasticity of carbon concentrating mechanisms in earliest evolved phytoplankton, Limnol. Oceanogr. Lett., 4, 37–43, 2019.
van Roij, L., Sluijs, A., Laks, J. J., and Reichart, G. J.: Stable carbon isotope analyses of nanogram quantities of particulate organic carbon (pollen) with laser ablation nano combustion gas chromatography/isotope ratio mass spectrometry, Rapid Commun. Mass Sp., 31, 47–58, 2017.
Whitney, S. M., Houtz, R. L., and Alonso, H.: Advancing our understanding and capacity to engineer nature's CO2-sequestering enzyme, Rubisco, Plant Physiol., 155, 27–35, 2011.
Wilkes, E. B. and Pearson, A.: A general model for carbon isotopes in red-lineage phytoplankton: interplay between unidirectional processes and fractionation by RubisCO, Geochim. Cosmochim. Ac., 265, 163–181, 2019.
Wilkes, E. B., Carter, S. J., and Pearson, A.: CO2-dependent carbon isotope fractionation in the dinoflagellate Alexandrium tamarense, Geochim. Cosmochim. Ac., 212, 48–61, 2017.
Witkowski, C. R., Weijers, J. W. H., Blais, B., Schouten, S., and Sinninghe Damsté, J. S.: Molecular fossils from phytoplankton reveal secular P trend over the phanerozoic, Sci. Adv., 4, 1–8, 2018.
Zeebe, R. E.: Kinetic fractionation of carbon and oxygen isotopes during hydration of carbon dioxide, Geochim. Cosmochim. Ac., 139, 540–552, 2014.
Zeebe, R. E. and Wolf-Gladrow, D. A.: CO2 in Seawater: Equilibrium, Kinetics, Isotopes, Elsevier Science Publishing Co., Amsterdam, the Netherlands, ISBN 9780444509468, 2001.
Zeebe, R. E., Wolf-Gladrow, D. A., and Jansen, H.: On the time required to establish chemical and isotopic equilibrium in the carbon dioxide system in seawater, Mar. Chem., 65, 135–153, 1999.
Zhang, H., Torres-Romero, I., Anjewierden, P., Jaggi, M., and Stoll, H. M.: The DIC carbon isotope evolutions during CO2 bubbling: implications for ocean acidification laboratory culture, Chem. Geol., 2022.
Zhang, Y. G., Pearson, A., Benthien, A., Dong, L., Huybers, P., Liu, X., and Pagani, M.: Refining the alkenone-pCO2 method I: Lessons from the Quaternary glacial cycles, Geochim. Cosmochim. Ac., 260, 177–191, 2019.
Zhu, J., Poulsen, C. J., and Otto-Bliesner, B. L.: High climate sensitivity in CMIP6 model not supported by paleoclimate, Nat. Clim. Chang., 10, 378–379, 2020.