the Creative Commons Attribution 4.0 License.
the Creative Commons Attribution 4.0 License.
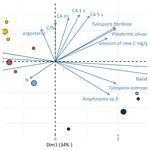
Nitrophobic ectomycorrhizal fungi are associated with enhanced hydrophobicity of soil organic matter in a Norway spruce forest
Nicholas P. Rosenstock
Susanne K. Woche
Georg Guggenberger
Håkan Wallander
In boreal forests an important part of the photo assimilates are allocated belowground to support symbiosis of ectomycorrhizal fungi (EMF). The production of EMF extramatrical mycelium can contribute to carbon (C) sequestration in soils, but the extent of this contribution depends on the composition of the EMF community. Some species can decrease soil C stocks by degrading soil organic matter (SOM), and certain species may enhance soil C stocks by producing hydrophobic mycelia which can reduce the rate of SOM decomposition. To test how EMF communities contribute to the development of hydrophobicity in SOM, we incubated sand-filled fungal-ingrowth mesh bags amended with maize compost for one, two or three growing seasons in non-fertilized and fertilized plots in a young Norway spruce (Picea abies) forest. We measured hydrophobicity as determined by the contact angle and the ratios in the mesh bags contents along with the amount of new C entering the mesh bags from outside (determined by C3 input to C4 substrate), and related that to the fungal community composition. The proportion of EMF species increased over time to become the dominant fungal guild after three growing seasons. Fertilization significantly reduced fungal growth and altered EMF communities. In the control plots the most abundant EMF species was Piloderma olivaceum, which was absent in the fertilized plots. The hydrophobicity of the mesh bag contents reached the highest values after three growing seasons only in the unfertilized controls plots and was positively related to the abundance of P. olivaceum, the ratios of the mesh bag contents and the amount of new C in the mesh bags. These results suggest that some EMF species are associated with higher hydrophobicity of SOM and that EMF community shifts induced by fertilization may result in reduced hydrophobicity of soil organic matter, which in turn may reduce C sequestration rates.
- Article
(1821 KB) - Full-text XML
- BibTeX
- EndNote
Fertilization of forests has been suggested as a way to increase C sequestration to mitigate climate change (Jörgensen et al., 2021). In support of this, Bergh et al. (2008) found more than doubling of aboveground growth of young Norway spruce forests in response to yearly additions of a complete fertilizer in experimental sites in Sweden. A major part of gross primary production, between 25 % and 63 % according to Litton et al. (2007), is however allocated belowground to roots and associated ectomycorrhizal fungi, and this portion usually declines in response to fertilization (Högberg et al., 2010). In support of this, reduced growth of mycelium of ectomycorrhizal fungi (EMF) was found in the young fertilized Norway spruce stands studied by Bergh et al. (2008) (Wallander et al., 2011).
EMF species form extensive mycelial networks, which efficiently distribute C in the soil (Smith and Read, 2008), and this mycelium turns into necromass when the mycelium dies. Necromass from different EMF species decomposes at different rates (Koide and Malcolm, 2009). Melanin content appears to have a negative influence on necromass decomposition, but physical protection is also an important factor to reduce decomposition according to Fernadez and Kennedy (2016). Soil organic matter (SOM) can be protected from decomposition in aggregates where hydrophobic coatings of mineral particles change the physical properties of the particles, reduce water films around them and limit water penetration inside the aggregates. This affects the mobility of microbial decomposers and extracellular enzymes from the soil solution and reduces organic matter decomposition (Leelamanie and Liyanage, 2016; Goebel et al., 2011; von Lützow et al., 2008), and hydrophobic SOM generally decomposes more slowly than hydrophilic SOM (Nguyen and Harvey, 2003, 2001). Since some EMF species form hydrophobic, while others form hydrophilic, mycelia (Unestam and Sun, 1995), the composition of the EMF community may thus have fundamental importance for the SOM properties and subsequently for carbon sequestration rates in the soil.
In contrast to carbon-accumulating activities by EMF species, certain species may also reduce soil C stocks by oxidizing organic matter to release nitrogen and phosphorus. Some EMF species use “brown-rot” Fenton chemistry and some use “white-rot” peroxidases to decompose SOM (Shah et al., 2015; Lindahl and Tunlid, 2015; Bödeker et al., 2014). This can result in a 30 % decrease in SOM according to Lindahl et al. (2021). Ectomycorrhizal fungi may thus have opposing effects on the amount of SOM, and differences in community composition were proposed as one explanation for different C accumulation rates in boreal forests in northern Sweden (Clemmensen et al., 2015, 2013): later successional stages that accumulated more C were dominated by ericoid mycorrhizal fungi with recalcitrant necromass, while younger successional stages that accumulated less C were dominated by EMF species of long-distance-exploration types with a high capacity to degrade soil organic matter. Certain EMF species may have exceptional importance for organic matter degradation as the presence of Cortinarius acutus (which has retained the enzymatic capability to break down SOM to access nutrients) was linked to 33 % lower C storage in the organic topsoils in 359 investigated stands in boreal forests in Sweden (Lindahl et al., 2021).
It is well known that fertilization with N has a strong impact on growth and composition of EMF species (Lilleskov et al., 2011; Wallenda and Kottke, 1988). Lilleskov et al. (2011) demonstrated that EMF species sensitive to N (e.g., Cortinarius, Tricholoma, Suillus and Piloderma) usually produce hydrophobic mycelia, while N-tolerant species often produce hydrophilic mycelia (e.g., Laccaria). Loss of hydrophobic EMF species at high N input could thus have consequences for SOM formation and C sequestration rates, but it is not well known to what extent EMF abundance has a significant effect on the overall hydrophobicity of SOM.
In our study with young Norway spruce forests reported above (Wallander et al., 2011), we used mesh bags amended with maize compost (C4 plant material enriched in 13C) to estimate EMF growth in control and fertilized plots. In the present study we analyzed the fungal communities as well as the hydrophobicity of the same mesh bag contents. The mesh bags were harvested after one, two or three growing seasons in order to follow fungal succession and development of hydrophobicity over time. All samples were subjected to 454 sequencing in order to characterize the fungal communities. We expected community composition to be influenced by fertilization and hydrophobicity to increase over time when EMF biomass and necromass accumulates. We also expected more N to be removed by EMF species from the mesh bags in the control than in the fertilized treatment. In addition, we expected higher hydrophobicity in control versus fertilized plots due to a higher proportion of hydrophobic species.
2.1 Study site
The experimental forest was located close to Ebbegärde in southeastern Sweden (56∘53′ N, 16∘15′ E) in a 10-year-old Norway spruce forest at time of sampling. The soil is a podzol on coarse sandy glacial till (site index G29), and the depth of the humus layer varied between 3 and 8 cm.
The treatments were designed in randomized block design with three fertilization treatments and three blocks per treatment (n=3). The plot size was 40×40 m. The fertilization treatments were the unfertilized control plots and two fertilization regimes. In the fertilization treatments specific amounts of nitrogen (N) (ammonium and nitrate) were applied to optimize plant growth without inducing leaching. The amount of N addition was based on needle N determinations and monitoring of N in soil water (Bergh et al., 2008). Thus, the fertilization was applied by hand as 50–100 kg N ha−1 every year for the first fertilization regime and as 100–150 kg N ha−1 every second year in the second fertilization regime (fertilization began in 2002). To avoid nutrient imbalance caused by fertilization, the quantity of micronutrients was adjusted to optimum nutrient proportions for Picea abies (as calculated by Ingestad, 1979). For a more detailed description of the fertilization regime see Linder (1995) and Bergh et al. (2008). For this study both fertilization regimes were treated as one fertilization treatment.
2.2 Experimental design
We used triangular-shaped ingrowth bags made of nylon mesh (50 µm mesh size, 10 cm side, ∼ 1 cm thick) to capture fungi growing in the soil. This mesh size allows the ingrowth of fungal hyphae but not of roots (Wallander et al., 2001). The mesh bags were filled with 30 g of acid-washed quartz sand (0.36–2.0 mm, 99.6 % SiO2, Ahlsell AB, Sweden) heated to a temperature of 600 ∘C overnight to remove all organic carbon. The sand was then mixed with 0.8 % () maize compost. Maize compost was used since it has a unique C isotopic signature, which makes it possible to estimate C influx into the mesh bags. Results from these measurements are presented in Wallander et al. (2011). Maize compost was produced by cutting maize leaves into small pieces and composting them in an isolated plastic compost bin for 12 months. After that the compost was kept at +4 ∘C. Fresh compost was forced through a 2 mm mesh and then mixed with dry sand to make a uniform mixture. The sand maize mixture had a carbon content of 0.4 %. The bags were buried at approximately 5 cm depth in the interface between the organic horizon and the mineral soil where ectomycorrhizal fungi are abundant (Lindahl et al., 2007). The first harvest was done in November 2007, after 8 months of incubation. The second harvest was done in November 2008, and the third harvest was done in November 2009. Four mesh bags were pooled to make one composite sample for each block, year and treatment. In the laboratory the mesh bags were opened and the contents from the four replicate mesh bags from each experimental plot were carefully pooled and mixed. Subsamples were taken for subsequent analyses (ergosterol, hydrophobicity, C and N content, fungal community) and immediately frozen.
The abundance of δ13C as well as total C and N content was analyzed using an elemental analyzer (model EuroEA3024; Eurovector, Milan, Italy) connected to an Isoprime isotope-ratio mass spectrometer (Isoprime, Manchester, UK) as described by Wallander et al. (2011). The isotopic shift that occurred when 13C-depleted C (mainly EMF mycelia) entered the bags from outside was used to calculate the amount of new C in the mesh bags. To estimate ectomycorrhizal growth, the fungal cell membrane compound ergosterol was measured as a biomarker of fungal biomass. Ergosterol was extracted from 5 g of the pooled sand–maize mixture from the mesh bag. Briefly the sample was subjected to saponification using a solution of 10 % KOH in methanol, and the non-polar phase (where the ergosterol is present) was separated using cyclohexane. The ergosterol was quantified by a high-performance liquid chromatograph (Hitachi model L2130) and a UV detector (Hitachi model L2400). For more detail regarding the protocol see Wallander et al. (2011).
2.3 DNA extraction, PCR and 454 sequencing
A total of 10 g of the sand–maize mixture from the composite samples was homogenized using a ball mill without a ball (Retsch, Haan, Germany). DNA was extracted from the homogenized samples by adding CTAB buffer (2 % cetyltrimetylammoniumbromid, 2 mM EDTA, 150 mM tris HCl, pH 8), vortexing and then incubating at 65 ∘C for 1.5 h, followed by chloroform addition, vortexing, supernatant removal, and isopropanol and ethanol precipitation. The pellet was resuspended in 50 µL of Milli-Q water (Millipore) and further cleaned using a Wizard DNA cleanup kit (Promega, Madison, WI, USA).
PCR was carried out for each sample in three triplicate 25 µL reactions, using the fungal-specific primers ITS1-F (Gardes and Bruns, 1993) and ITS4 (White, 1990). Each primer was elongated with adaptors required for 454 pyrosequencing (ITS1-F/A adaptor and ITS4/B adaptor). The ITS4 also contained a sample-specific tag consisting of eight bases; ITS1-F/A 5′-CCTATCCCCTGTGTGCCTTGGCAGTCTCAGCTTGGTCATTTAGAGGAAGTAA-3′ and ITS4/B 5′-CCATCTCATCCCTGCGTGTCTCCGACTCAGXXXXXXXXTCCTCCGCTTATTGATATGC-3′. PCR products were purified with Agencourt AMPure kit (Agencourt Bioscence Corporation, Beverly, MA, USA) in order to remove residual salts, primers and primer dimers. The concentration of the purified PCR products was measured with the PicoGreen dsDNA quantification kit (Molecular Probes, Eugene, OR, USA) on a FLUOstar OPTIMA (BMG Labtech Gmbh, Ortenberg, Germany). Equal amounts of DNA from each sample were pooled into one single pool and submitted for 454 pyrosequencing. Sequencing was performed on an FLX 454 (Roche Applied Biosystems) using the Lib-L chemistry at the pyrosequencing facility at Lund University, Lund, Sweden.
2.4 Bioinformatic analysis
After sequencing, sequences were trimmed and filtered using Mothur v1.34 (Schloss et al., 2009). The trim sequence operation was run with the following exclusion parameters: all sequences that mismatched the sample ID barcode at more than one position, those that mismatched the primers at more than two positions, those that had homopolymers longer than 10 bp, those that were shorter than 150 bp or those that had an average base call quality score below 20 over a moveable window of 40 bases.
Sequences outside the ITS2 region and chimeric sequences were removed using ITSx extractor v1.5.0 (Bengtsson-Palme et al., 2013). After filtering, a Bayesian clustering was applied to the sequences using the Gaussian mixture model CROP (Hao et al., 2011) at 97 % sequence similarity, and a set of operational taxonomic units (OTUs) was thus obtained. Clusters that were only found in one mesh bag sample (one PCR reaction) were excluded, further reducing the possibility that any chimeric sequences were used in our analysis. Search for sequence identities were performed by iteratively BLASTing (BLAST denotes “basic local alignment search tool”) against two different sequence databases: the first was the UNITE (Koljalg et al., 2005, http://unite.ut.ee/index.php, last access: 10 August 2022) reference/representative sequence database (21 000 sequences, dynamic taxa threshold, release date 9 February 2014), and the second was the full UNITE+INSD sequence database (377 000 sequences, dynamic threshold, release date 15 February 2014) (Karsch-Mizrachi et al., 2012). The UNITE and INSD databases were purged of all sequences, nearly 25 % of the total, that did not have any taxonomic information, primarily environmental samples from soils and roots using Boolean terms (excluding Environmental, uncultured, root endophyte, unidentified). Sequences were assigned to species when there was at least 97 % similarity between the query sequence and top hit. Sequences that failed to match at this threshold were excluded. Separate clusters that matched the same database sequence were subsequently lumped into one OTU.
Using names and taxonomy associated with the OTUs, the total fungal community was divided by both phylum (Basidiomycota, Ascomycota, Mucoromycota, Zoopagomycota and Chytridiomycota) and function (known ectomycorrhizal fungi, unknown ectomycorrhizal status and saprotrophic fungi); OTUs were considered known ectomycorrhizal fungi based on the knowledge of the ecology of known close relatives (genera or below) according to Tedersoo et al. (2010).
After filtering, each sample was rarified to the median number of reads using the “rrarefy” function in the vegan package (Oksanen et al., 2017) in R (R Core Team, 2013). For community comparison (total or for ectomycorrhizal fungi), all read abundances were converted to relative abundance such that the read abundances for all OTUs for each sample totaled 1.
2.5 Hydrophobicity
The hydrophobicity was evaluated in terms of contact angle (CA) with the sessile drop method (Bachmann et al., 2003), using a CCD-equipped CA microscope (OCA 15, DataPhysics, Filderstadt, Germany). Here the angle a drop of water forms at the solid–liquid–vapor interphase is measured. This contact angle is used to describe the wettability of the surface; CA ≥ 90∘ indicates a hydrophobic and a zero CA a hydrophilic surface. A CA > 0 and < 90∘ indicates subcritical water repellency.
For measurement, material from the mesh bags contents was fixed on a glass slide with double-sided adhesive tape in an ideally one-grain layer. Placement of a water drop is recorded and the initial CA evaluated after ending of mechanical disturbances by drop shape analysis (ellipsoidal fit) and fitting tangents on the left and right side of the drop, using the software SCA 20 (DataPhysics, Filderstadt, Germany; Goebel et al., 2013). CA is given as the mean CA of the left and right side of the drop. For an estimate about CA stability, CA was evaluated again after 1 s (denoted as CA1 s) and after 5 s (denoted as CA5 s; Bachmann et al., 2021).
Three replicates from each treatment (control or fertilized) and each incubation period (2007, 2008, 2009) were used in the measurements. One slide per replicate was prepared, and for each slide six drops were placed and averaged to obtain one CA per replicate (n=6). Two slides containing the non-incubated sand–maize compost mix were also analyzed as a non-treated reference material. Due to the coarse texture of the mesh bag material, the drop volume was 6 µL.
2.6 Statistical analysis
The statistical analyses for the fungal communities were performed using the vegan package (Oksanen et al., 2017) in R (R Core Team, 2013). Fungal communities were visualized with ordination using non-parametric multidimensional scaling (NMDS) using the metaMDS function. Differences in community structure were visually compared with centroids and the associated 95 % confidence interval associated with a t distribution around the standard error of the centroid. To detect if the fungal communities were significantly influenced by the treatments (fertilization and incubation periods), permutational multivariate analysis of variance (PERMANOVA; Anderson, 2017) was performed. Pairwise comparisons between treatments were tested using the pairwise Adonis test.
To test for differences in hydrophobicity (contact angle), ratios, new C inside the mesh bags and ergosterol, ANOVA and two-way ANOVA were performed using the car package (Fox and Weisberg, 2019) in R (R Core Team, 2013). To test for differences in the relative abundance of EMF species between the treatments, Dunn's test for non-parametrical samples was performed (Dinno, 2015).
Principal component analysis (PCA) was used to analyze the relationships between the most abundant fungal species and the properties of the mesh bag contents (hydrophobicity (contact angle), ratios, new carbon inside the mesh bags, ergosterol) using the package FactoMineR (Lê et al., 2008) in R (R Core Team, 2013).
3.1 Fungal biomass
The concentration of ergosterol, as an estimate of fungal biomass, in the mesh bags has been reported earlier (Wallander et al., 2011) and is summarized in Table 1. In brief, ergosterol content increased from a starting value of 0.7 (original maize compost) to 2.2 mg g−1 in the mesh bags after incubation for one growing season in control plots. After this the concentration did not change significantly over the following 2 years. In fertilized plots the concentration was significantly lower than in the control plots (ANOVA, F=13.4, p<0.01).
Table 1Average and standard error of the ergosterol concentrations, total C %, ratio, amount of new carbon (C3 mainly from EMF species), percentage of EMF DNA reads and contact angle determined 5 s after placement of water droplets placed on mesh bags material amended with maize compost (CA5 s; estimation of contact angle stability). Low score letters refer to statistical differences according to the post hoc Tukey test and pairwise Dunn test. Asterisks correspond to statistical differences for CA after 5 s between the mesh bag contents and the non-incubated reference material.
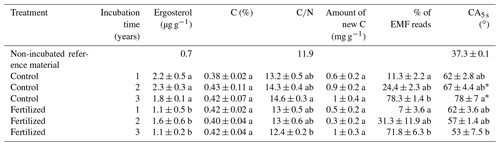
3.2 Hydrophobicity, C content and ratio of SOM
Incubation in the field significantly increased hydrophobicity of the mesh bag contents in the unfertilized control plots as indicated by CA1 s and CA5 s (ANOVA, F=6.2 and p<0.05 and ANOVA, F=10.2 and p<0.01, respectively). CA of the control plots increased with incubation time, but only CA1 s and CA5 s were significantly different from the reference material (non-incubated sand–maize compost mix); i.e., stability of CA was increased (Fig. 1a).
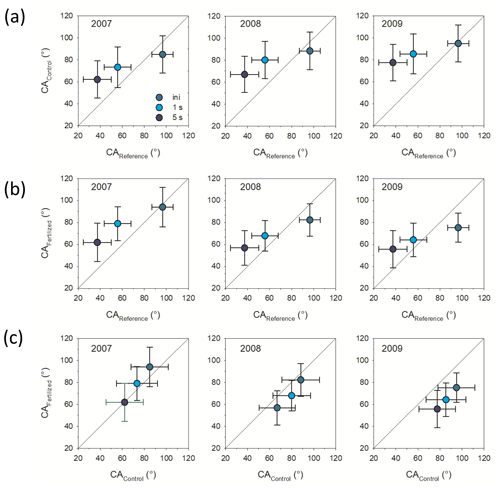
Figure 1Contact angle (CA) comparisons between (a) control treatment and reference material, (b) fertilization treatment and reference material, and (c) control and fertilized treatments. Shown is the initial CA (ini), determined directly after placement of the water drop and CA determined 1 and 5 s after placement of the water drop. Bars represent standard deviation (n=3).
Incubation in the field also affected hydrophobicity of the mesh bag contents in the fertilized plots as indicated by the initial CA and CA1 s and CA5 s (ANOVA, F=5.2 and p<0.05; ANOVA, F=4.1 and p=0.06; and ANOVA, F=3.9 and p=0.05, respectively). The CA stability (CA1 s and CA5 s) was increased compared to the reference material only in the 1-year-incubation mesh bags. As the time of incubation in the soil increased, however, CA decreased. After 3 years of incubation the initial CA became significantly smaller in comparison with the reference material (Fig. 1b). There were significant differences in the CA (initial, CA1 s and CA5 s) between mesh bags from the control and fertilized plots in the 3-year-incubation bags with smaller CA (initial, CA1 s and CA5 s) for the fertilized plots compared to the control (2009; Fig. 1c; ANOVA, F=3.2 and p<0.05; F=3.1 and p=0.05, and F=2.8 and p=0.06, respectively) but not for the first and second incubation year (2007, 2008)
The concentration of C in the mesh bags was not influenced by time or fertilization, but the amount of new C (C3 C presumably from EMF species) in the mesh bags was significantly affected by fertilization and was higher in the control plots than in the fertilized plots according to the two-way ANOVA (F=5.3, p<0.05). The amount of new C tended to increase with incubation time in the control plots (Table 1). The interaction between fertilization and incubation time was not significant.
There was a positive correlation between the amount of new C and the hydrophobicity for both CA1 s and CA5 s (Pearson, T=2, p=0.06, Cor = 0.45) (Fig. 2a and b, respectively). When breaking down the data by fertilization regime, there was a positive correlation between amount of new C and the hydrophobicity for the CA5 s in the control plots (Pearson, T=2.2, p=0.06, Cor = 0.63) (Fig. 2c), but the correlation tended to be negative in the fertilized plots (Fig. 2d).
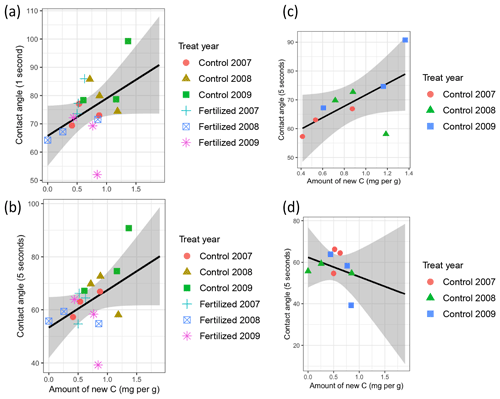
Figure 2Correlation between the amount of new C and the hydrophobicity of the mesh bag contents measured as the contact angle (CA). The CA was determined directly after placement of the water drop at (a) 1 s and (b) 5 s. (c) Correlation between the amount of new C and the hydrophobicity of the mesh bag at 5 s in the control plots. (d) Correlation between the amount of new C and the hydrophobicity of the mesh bag at 5 s in the fertilized plots.
The ratio of the mesh bag content was 11.9 in the initial material, which increased to an average of 14.6 and 12.2 after 3 years of incubation in the control and fertilized plots, respectively (Table 1). According to the two-way ANOVA, fertilization had a significant effect on the ratios of the mesh bags (ANOVA, F=6.1, p<0.05). The impact of incubation time or the interaction between fertilization and incubation was not significant. During the first 2 incubation years (2007, 2008) there were no differences between the ratios in the control and fertilized samples. During the third incubation year (2009) the ratios in the control samples were significantly higher than the ratios in the fertilized samples.
3.3 Effects of fertilization and incubation time on fungal community composition
After all bioinformatic processing and quality filtering, followed by rarefaction to a maximum of 1200 sequence reads per sample (minimum 612) and elimination of all operational taxonomic units (OTUs) that were only found in one sample, 26 943 sequence reads were recovered that were apportioned to 146 OTUs.
The total fungal communities were significantly influenced by incubation time and by fertilization according to the PERMANOVA analysis (p<0.001 and F=5.4 and p<0.001 and F=8.4, respectively) (Fig. 3a).
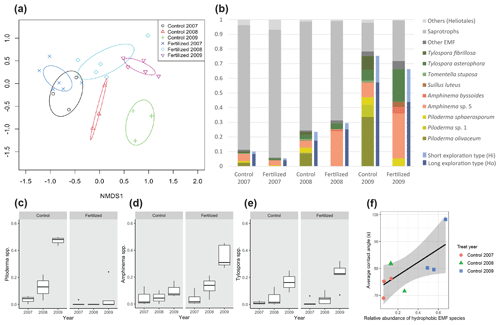
Figure 3Response of the fungal communities in the mesh bags to the fertilization treatment and incubation time. (a) NMDS ordination analysis of the fungal communities. (b) Relative abundance of the different fungal species and their corresponding mycelium exploration type and hydrophobic (Ho) or hydrophilic (Hi) properties. (c) Relative abundance of the genus Piloderma. (d) Relative abundance of the genus Amphinema. (e) Relative abundance of the genus Tylospora. (f) Correlation between the proportion hydrophobic EMF species and the averaged contact angle (initial CA, CA at 1 s and CA at 5 s) in the control plots.
Fertilization had no significant effect on the total fungal community during the first year, but during the second year and third year the fertilization effect was found to be significant (pairwise Adonis, p=0.06 and F=2 and p=0.02 and F=5.3, respectively).
The proportion of EMF sequences increased significantly over time in the mesh bags (Dunn test, χ2=18, p<0.0001) (Fig. 3b). Of the sequences, 11 % and 7 % were EMF species during the first growing period in the control and fertilized plots, respectively. These values increased to 24 % and 31 % after 2 years of incubation in the control and fertilized plots, respectively, and to 78 % and 72 % after three growing seasons in the control and fertilized plots, respectively (Table 1). The number of EMF reads was significantly correlated with the new C in the mesh bags (Pearson, T=2.4, p<0.05, Cor = 0.46).
The more abundant hydrophobic EMF genera were Piloderma and Amphinema (Fig. 3c and d, respectively), while the more abundant hydrophilic genus was Tylospora (Fig. 3e).
The proportion of hydrophobic EMF species (the sum of the relative abundance of fungal reads belonging to hydrophobic EMF species) tended to be higher in the control plots (up to 57 % of the total fungal reads) in comparison with the fertilized plots (up to 44 % of the total fungal reads) in the 3-year-incubation bags, but this increase was not significant. Additionally, the proportion of hydrophobic EMF species in relation to hydrophilic EMF species in the control plots tended to be higher than in the fertilized plots in the 3-year bags, but this was not significant. When both treatments (control and fertilization) where analyzed together, there was no correlation between the proportion of hydrophobic species and the contact angle. The proportion of hydrophobic EMF species was positively correlated with the averaged contact angle (initial CA, CA at 1 s and CA at 5 s) in the control plots (Pearson, T=2.9, p<0.04, Cor = 0.68) (Fig. 3f) but not in the fertilized plots.
Piloderma increased in abundance over time in the control plots to become the dominating genus (up to 47 % of the relative abundance) after 3 years of incubation (Fig. 3c). The most dominant species in the control plots was Piloderma olivaceum, which was reduced to 0 % in the fertilized plots independent of incubation time (Fig. 3b). Tylospora fibrillosa was also reduced in response to fertilization (Dunn test, χ2=13.4, p<0.0001), while T. asterophora showed an opposite trend (Dunn test, χ2=4.4, p<0.05) (Fig. 3b). Amphinema sp. 5 was the most abundant species in the fertilized plots and was enhanced by fertilization (Dunn test, χ2=3.8, p<0.05) (Fig. 3b).
3.4 Principal component analysis
The principal component analysis (Fig. 4) separated the samples by incubation time along principal component 1. This component explained 34 % of the variance. Samples belonging to the 3-year-incubation bags were ordinated to the right of principal component 2. Along principal component 2 (PC2) the samples were separated by the fertilization treatment. This component explained 25.7 % of the variance. Samples belonging to the unfertilized controls were ordinated above principal component 1 (PC1). The linear model showed that the fertilization/incubation treatments were significantly associated PC1 (F=8.3, p<0.01) and PC2 (F=18, p<0.0001). The proportion of EMF species and Basidiomycota increased over time while the proportion of saprotrophic fungi and Ascomycota decreased with increasing incubation time. The EMF species Tylospora fibrillosa and Piloderma olivaceum were positively related to the CA (initial CA, CA1 s, CA5 s), with the ratios and with the amount of new carbon inside the mesh bags; and their vectors were directed towards longer incubation time and the opposite to the fertilization treatments.
4.1 Effect of incubation and fertilization on the total fungal communities
As expected, the fungal communities were influenced by the fertilization and by incubation time and there was a significant increase in the percentage of EMF reads over time. It should, however, be noted that the ingrowth of EMF species in relation to other fungal groups was surprisingly low during the first growing season (< 12 % of the fungal sequences), which is much lower than what has been found in earlier studies (Parrent and Vilgalys, 2007; Wallander et al., 2010). Some of this variation may be due to different weather conditions – the first year was wetter than normal, while the third was close to normal in precipitation (Wallander et al., 2011) – or due to larger belowground carbon allocation when the trees approach canopy closure during the third year, as discussed in Wallander et al. (2010). Whether shifts in EMF species were due to selection of later succession fungal taxa or variation in climatic conditions remains unclear but is ultimately not particularly important in terms of understanding how shifts in EMF species relate to soil organic matter cycling. Thus, the EMF abundance was highest during the third year, and this increase was associated with higher ratios and hydrophobicity in the control plots and higher input of new C in the control and fertilized plots. This suggests a strong relation between EMF species and the changes in the properties of the organic material in the mesh bags.
The most dominant EMF genera in our study were Amphinema, Piloderma and Tylospora, which are also common in other studies of EMF communities in coniferous forests (Almeida et al., 2019; Walker et al., 2014; Tedersoo et al., 2008). In the control plots, the most dominant species was P. olivaceum, which did not colonize the mesh bags collected from fertilized plots. Piloderma is a common genus in boreal forests and is reported to be more abundant in soils rich in organic N (Heinonsalo et al., 2015; Lilleskov et al., 2002b) and to decline in response to inorganic N fertilization (Teste et al., 2012) and elevated N deposition (Kjöller et al., 2012; Lilleskov et al., 2011, 2002a; Taylor et al., 2000). The decline in Piloderma in the fertilized plots in the present study is not surprising since this genus produces abundant hydrophobic rhizomorphs that might constitute a large C cost for the host (Defrenne et al., 2019), which is not economical for symbiosis at high mineral N concentrations. Other more direct effects of the fertilizer on the growth of Piloderma mycelium are also possible. The increase in the ratios of the mesh bag substrates from the control treatment might thus be an effect of biomass accumulation of Piloderma species, since EMF species in general have a higher ratio than maize compost (Wallander et al., 2003). Additionally, it has been shown that P. olivaceum produces proteases that improve the ability of the host trees to utilize N from organic compounds (Heinonsalo et al., 2015). Therefore, N released from the maize compost by this fungus could have been transferred to the host plants, which would contribute to the increase in ratios in the control plots in comparison with the fertilized plots. This explanation is consistent with results described by Nicolás et al. (2017), who used FTIR and NEXAFS to analyze chemical changes of similar maize compost incubated in mesh bags over one growing season in a Norway spruce forest in southwestern Sweden. They found that heterocyclic-N compounds declined in mesh bags in comparison with non-incubated reference material, which was interpreted as an effect of removal by EMF species and transfer to the host trees. This decline was higher in the unfertilized control plots compared with fertilized plots. In the fertilized plots of the present study, the amount of new C tended to increase in the 3-year-incubation bags where the ratios reached the lowest values, indicating limited N removal by the EMF species colonizing these bags.
Amphinema sp. 5 responded positively to fertilization in our study, which is supported by a study by Kranabetter (2009), who found a strong increase in the abundance of Amphinema-colonized root tips along productivity gradients in Canada. While a reduced abundance of T. fibrillosa was observed in the fertilized plots, T. asterophora responded positively. Similarly contrasting effects between these two species were found in other studies as well (Teste et al., 2012; Kjöller et al., 2012; Toljander et al., 2006). In an N deposition gradient Kjøller et al. (2012) found increased abundance of Tylospora asterophora in areas with high N throughfall, while T. fibrillosa abundance decreased with higher N deposition. Reduction in T. fibrillosa in response to fertilization may be a result of C starvation since it has been shown that this species is more dependent on C transferred from a living host in order to colonize new seedlings on a clear-cut compared to Amphinema sp., which readily colonized saplings on clear-cuts (Walker and Jones, 2013).
4.2 Effect of incubation and fertilization on hydrophobicity
As expected, hydrophobicity increased over time in respect to the reference material (non-incubated maize–sand mixture), and this increase occurred only in the unfertilized controls at the last sampling when the fungal communities in the mesh bags were dominated by EMF species. This increase in hydrophobicity was expected to be an effect of the accumulation of fungal biomass and necromass over time as it has been shown that organic C (Woche et al., 2017; Mataix-Solera and Doerr, 2004; Chenu et al., 2000) and microbial biomass and necromass contribute to the hydrophobicity of soils (Schurig et al., 2013; Šimon et al., 2009; Capriel, 1997). However, the total amount of C was similar for all the incubation times and was not affected by fertilization, indicating that C content alone could not explain the variations in hydrophobicity. Instead, the amount of new C entering the mesh bags from outside was found to be significantly correlated with hydrophobicity (CA1 s and CA5 s). This new C is expected to be of EMF origin as discussed by Wallander et al. (2011). Since saprotrophic fungi utilize the maize compost material as their C source, it is expected that new C inputs come from plant photoassimilates and are brought by EMF species (Wallander et al., 2011). Therefore, these results suggest that the accumulation of biomass and necromass of EMF origin over time might contribute to the buildup of hydrophobicity in SOM in the control plots.
Our results show that fertilization reduced ergosterol concentration in the mesh bags in comparison with the control samples (Wallander et al., 2011) and this coincided with a decrease in the hydrophobicity over time in comparison with the unfertilized controls and the non-incubated reference material. It has been shown that fungi may enhance soil water repellency of soil particles since some filamentous fungi produce insoluble substances like ergosterol and hydrophobins (Mao et al., 2019; Rillig et al., 2010). For instance, Hallet et al. (2001) found that soil hydrophobicity decreased when fungi were killed after fungicide additions. Therefore, it is possible that the lower fungal biomass in the fertilized plots in our study led to a decrease in hydrophobicity as incubation time in the soil increased. However the concentration of ergosterol in the mesh bags from the control plots did not increase with incubation time and even tended to decline in the last incubation sampling when hydrophobicity increased, indicating that ergosterol alone is not a good predictor of hydrophobicity. It is possible that high ergosterol values after one growing season was an effect of high abundance of yeast like Guehomyces, Cryptococcus, Rhodotorula and Candida, which are unlikely to contribute to hydrophobicity but dominated the fungal communities of the mesh bags during the first growing seasons. These fungi decreased drastically in abundance in the 3-year-incubation bags. The ergosterol content per dry mass of yeasts is much higher than in filamentous fungi (Pasanen et al., 1999), which might explain the high ergosterol values in the first incubation periods. From these results we conclude that hydrophobicity is more associated with EMF colonization (measured as the amount of new C) than with total fungal biomass (measured by ergosterol). It should also be noted that we cannot rule out the possibility that other compounds from the soil entered the mesh bags during the underground incubation. In soils, polymeric substances coming from SOM, root or microbial exudates can have hydrophobic properties (Vogelmann et al., 2013). Hence, the hydrophobic changes in the material could be partly explained by sources other than EMF mycelium. However, the significant correlation between the new carbon in the bags and the EMF reads and the negative effect of fertilization on the CA might suggest that hydrophobicity changes in the mesh bag content are caused mainly by EMF species.
Given the apparent association of EMF colonization with higher hydrophobicity over time, some EMF species may be expected to be more important than others for this process. We expected higher hydrophobicity in the control plots in response to a higher proportion of hydrophobic long-distance-exploration-type species. Indeed, the proportion of hydrophobic EMF species in the control plots tended to be higher in comparison with the fertilized plots in the mesh bags incubated for 3 years. From the hydrophobic species in the control plots, Piloderma spp. constituted the majority of fungal species with up to 47 % of the total fungal reads. The presence of Piloderma species like P. olivaceum, known to form hydrophobic mycelia (Lilleskov et al., 2011; Agerer, 2001) and which was totally absent in the fertilized plots, is likely to contribute significantly to hydrophobicity of SOM. In the fertilized plots there was also an increase over time in the proportion of hydrophobic EMF species (Amphinema being the most abundant hydrophobic genus), which was not accompanied by an increase in hydrophobicity. This may suggest that necromass from Amphinema does not accumulate to the same extent as for Piloderma and is probably not associated with the hydrophobicity in the mesh bags. These findings suggest that hydrophobicity of living mycelium might not necessary influence the water retention of the organic material to a large extent. This is consistent with the findings of Zheng et al. (2014), who found that the hydrophobicity of EMF mycelium does not necessary enhance soil water repellency. They tested how different EMF strains were inoculated on Pinus sylvestris-affected water repellency of sandy loamy soil. The mycelium hydrophobicity of the fungal strains used in their experiment was previously tested by drop immersion on fungal mycelium growing on pure cultures. The authors found that the mycelium from hydrophobic species generally enhanced water repellency but not all hydrophobic isolates had a positive effect on soil hydrophobicity. It was suggested that beside mycelium hydrophobicity, other species-dependent factors like growth patterns, the degree of soil particles coverage or the quantity of hydrophobic substances produced by the fungus might influence soil water repellency. In the present study the difference in hydrophobicity between treatments might be related to not only the exploration types of the abundant species but also species-dependent features. For example, the characteristic yellow color of Piloderma comes from an insoluble pigment called corticrocin (Gray and Kernaghan, 2020; Schreiner et al., 1998). Moreover, the hyphae of Piloderma are reported to be coated with calcium oxalate crystals (Arocena et al., 2001), probably as a strategy against grazers or to repel water to avoid microbial predation (Gray and Kernaghan, 2020; Whitney and Arnott, 1987). Thus, these particular features of Piloderma make it a good candidate to explain the enhanced hydrophobicity of the material in the control mesh bags, which is supported by the association between the abundance of this fungus, the new C in the mesh bags and the CA.
4.3 Ecological significance
The effect of fertilization on fungal communities and its significance for C sequestration has been largely discussed (see Jörgensen et al., 2021; Almeida et al., 2019; Högberg et al., 2010; Janssens et al., 2010; Treseder, 2004). Additions of inorganic N may have a strong positive effect on plant net primary production (Binkley and Högberg, 2016) but have also been shown to decrease belowground C allocation (Högberg et al., 2010) and consequently decrease EMF biomass (Almeida et al., 2019; Bahr et al., 2015; Högberg et al., 2007, 2010; Nilsson and Wallander, 2003), which will reduce the input of C to the soils and may reduce C sequestration. However, Bödeker et al. (2014), for example, showed that addition of inorganic N significantly decreased the abundance of Cortinarius acutus, a species that can enhance SOM decomposition in order to take up N (Lindahl et al., 2021). The decrease in Cortinarius sp. was accompanied by a decrease in the enzymatic oxidation in the humus layer of the soil. Therefore, it has been suggested that fertilization might improve C sequestration by suppressing SOM decomposition by some key EMF genera like Cortinarius (Lindahl and Tunlid, 2015; Bödeker et al., 2014). In the current study we show that Piloderma, another common species from northern-forested ecosystems, is negatively affected by fertilization and that its decrease might be associated with a decrease in the organic-material hydrophobicity. These findings suggest that even if fertilization could reduce the abundance of EMF species with decomposer capabilities, it may also reduce the accumulation of hydrophobic fungal mycelium that could enhance SOM formation and C sequestration rates. Therefore, the role of different abundant EMF genera like Piloderma and Cortinarius in boreal forests for establishment and destruction of hydrophobicity and the effect of fertilization on them warrant further research.
The pipelines and software used to analyze the data in this study are described in the references provided in Sect. 2.4 and 2.6 of this article.
For further information on the data, the readers should contact the corresponding author of the article.
JPA contributed in terms of conceptualization of the research goals and aims, data curation and analysis, and manuscript writing. NPR contributed in terms of data acquisition, curation and analysis. SKW contributed in terms of data acquisition, curation and analysis. GG contributed in terms of conceptualization and development of the methodology. HW contributed in terms of conceptualization and development of the methodology, research goals and aims and manuscript writing.
The contact author has declared that none of the authors has any competing interests.
Publisher’s note: Copernicus Publications remains neutral with regard to jurisdictional claims in published maps and institutional affiliations.
This article is part of the special issue “Global change effects on terrestrial biogeochemistry at the plant–soil interface”. It is not associated with a conference.
We would like to thank the Swedish Research Council for Sustainable Development (Formas) for financial support.
This research was supported by a grant from Formas (grant no. 2018-00634) for Håkan Wallander.
This paper was edited by Serita Frey and reviewed by Mark Anthony, Christopher Fernandez, and one anonymous referee.
Agerer, R.: Exploration types of ectomycorrhizae: A proposal to classify ectomycorrhizal mycelial systems according to their patterns of differentiation and putative ecological importance, Mycorrhiza, 11, 107–114, https://doi.org/10.1007/s005720100108, 2001.
Almeida, J. P., Rosenstock, N. P., Forsmark, B., Bergh, J., and Wallander, H.: Ectomycorrhizal community composition and function in a spruce forest transitioning between nitrogen and phosphorus limitation, Fungal Ecol., 40, 20–31, https://doi.org/10.1016/j.funeco.2018.05.008, 2019.
Anderson, M. J.: Permutational Multivariate Analysis of Variance (PERMANOVA), Wiley StatsRef Stat. Ref. Online, John Wiley & Sons, 1–15, https://doi.org/10.1002/9781118445112.stat07841, 2017.
Arocena, J. M., Glowa, K. R., and Massicotte, H. B.: Calcium-rich hypha encrustations on Piloderma, Mycorrhiza, 10, 209–215, https://doi.org/10.1007/s005720000082, 2001.
Bachmann, J., Woche, S. K., Goebel, M. O., Kirkham, M. B., and Horton, R.: Extended methodology for determining wetting properties of porous media, Water Resour. Res., 39, 1–14, https://doi.org/10.1029/2003WR002143, 2003.
Bachmann, J., Söffker, S., Sepehrnia, N., Goebel, M. O., and Woche, S. K.: The effect of temperature and wetting–drying cycles on soil wettability: Dynamic molecular restructuring processes at the solid–water–air interface, Eur. J. Soil Sci., 72, 2180–2198, https://doi.org/10.1111/ejss.13102, 2021.
Bahr, A., Ellström, M., and Bergh, J.: Nitrogen leaching and ectomycorrhizal nitrogen retention capacity in a Norway spruce forest fertilized with nitrogen and phosphorus, Plant Soil, 390, 323–335, https://doi.org/10.1007/s11104-015-2408-6, 2015.
Bengtsson-Palme, J., Ryberg, M., Hartmann, M., Branco, S., Wang, Z., Godhe, A., De Wit, P., Sánchez-García, M., Ebersberger, I., de Sousa, F., Amend, A., Jumpponen, A., Unterseher, M., Kristiansson, E., Abarenkov, K., Bertrand, Y. J. K., Sanli, K., Eriksson, K. M., Vik, U., Veldre, V., and Nilsson, R. H.: Improved software detection and extraction of ITS1 and ITS2 from ribosomal ITS sequences of fungi and other eukaryotes for analysis of environmental sequencing data, Methods Ecol. Evol., 4, 914–919, https://doi.org/10.1111/2041-210X.12073, 2013.
Bergh, J., Nilsson, U., Grip, H., Hedwall, P. O., and Lundmark, T.: Effects of frequency of fertilisation on production, foliar chemistry and nutrient leaching in young Norway spruce stands in Sweden, Silva Fenn., 42, 721–733, https://doi.org/10.14214/sf.225, 2008.
Binkley, D. and Högberg, P.: Tamm Review: Revisiting the influence of nitrogen deposition on Swedish forests, Forest Ecol. Manage., 368, 222–239, https://doi.org/10.1016/j.foreco.2016.02.035, 2016.
Bödeker, I. T. M., Clemmensen, K. E., de Boer, W., Martin, F., Olson, Å., and Lindahl, B. D.: Ectomycorrhizal Cortinarius species participate in enzymatic oxidation of humus in northern forest ecosystems, New Phytol., 203, 245–256, https://doi.org/10.1111/nph.12791, 2014.
Capriel, P.: Hydrophobicity of organic matter in arable soils: Influence of management, Eur. J. Soil Sci., 48, 457–462, https://doi.org/10.1111/j.1365-2389.1997.tb00211.x, 1997.
Chenu, C., Le Bissonnais, Y., and Arrouays, D.: Organic Matter Influence on Clay Wettability and Soil Aggregate Stability, Soil Sci. Soc. Am. J., 64, 1479–1486, https://doi.org/10.2136/sssaj2000.6441479x, 2000.
Clemmensen, K. E., Bahr, A., Ovaskainen, O., Dahlberg, A., Ekblad, A., Wallander, H., Stenlid, J., Finlay, R. D., Wardle, D. A., and Lindahl, B. D.: Roots and associated fungi drive long-term carbon sequestration in boreal forest, Science, 340, 1615–1618, https://doi.org/10.1126/science.1231923, 2013.
Clemmensen, K. E., Finlay, R. D., Dahlberg, A., Stenlid, J., Wardle, D. A., and Lindahl, B. D.: Carbon sequestration is related to mycorrhizal fungal community shifts during long-term succession in boreal forests, New Phytol., 205, 1525–1536, https://doi.org/10.1111/nph.13208, 2015.
Defrenne, C. E., Philpott, T. J., Guichon, S. H. A., Roach, W. J., Pickles, B. J., and Simard, S. W.: Shifts in ectomycorrhizal fungal communities and exploration types relate to the environment and fine-root traits across interior douglas-fir forests of western Canada, Front. Plant Sci., 10, 1–16, https://doi.org/10.3389/fpls.2019.00643, 2019.
Dinno, A.: Nonparametric pairwise multiple comparisons in independent groups using Dunn's test, Stata J., 15, 292–300, https://doi.org/10.1177/1536867x1501500117, 2015.
Doerr, S. H., Shakesby, R. A., and Walsh, R. P. D.: Soil water repellency: Its causes, characteristics and hydro-geomorphological significance, Earth Sci. Rev., 51, 33–65, https://doi.org/10.1016/S0012-8252(00)00011-8, 2000.
Fernandez, C. W. and Kennedy, P. G.: Revisiting the “Gadgil effect”: Do interguild fungal interactions control carbon cycling in forest soils?, New Phytol., 209, 1382–1394, https://doi.org/10.1111/nph.13648, 2016.
Fox, J. and Weisberg, S.: An R Companion to Applied Regression, 3rd Edn., Thousand Oaks CA, Sage, https://socialsciences.mcmaster.ca/jfox/Books/Companion/ (last access: 10 August 2022), 2019.
Gardes, M. and Bruns, T. D.: ITS primers with enhanced specificity for basidiomycetes – application to the identification of mycorrhizae and rusts, Mol. Ecol., 2, 113–118, https://doi.org/10.1111/j.1365-294X.1993.tb00005.x, 1993.
Gray, L. and Kernaghan, G.: Fungal Succession During the Decomposition of Ectomycorrhizal Fine Roots, Microb. Ecol., 79, 271–284, https://doi.org/10.1007/s00248-019-01418-3, 2020.
Goebel, M. O., Bachmann, J., Reichstein, M., Janssens, I. A., and Guggenberger, G.: Soil water repellency and its implications for organic matter decomposition – is there a link to extreme climatic events?, Glob. Change Biol., 17, 2640–2656, https://doi.org/10.1111/j.1365-2486.2011.02414.x, 2011.
Goebel, M. O., Woche, S. K., Abraham, P. M., Schaumann, G. E., and Bachmann, J.: Water repellency enhances the deposition of negatively charged hydrophilic colloids in a water-saturated sand matrix, Colloid Surface A, 431, 150–160, https://doi.org/10.1016/j.colsurfa.2013.04.038, 2013.
Hallett, P. D., Baumgartl, T., and Young, I. M.: Subcritical Water Repellency of Aggregates from a Range of Soil Management Practices, Soil Sci. Soc. Am. J., 65, 184–190, https://doi.org/10.2136/sssaj2001.651184x, 2001.
Hao, X., Jiang, R., and Chen, T.: Clustering 16S rRNA for OTU prediction: A method of unsupervised Bayesian clustering, Bioinformatics, 27, 611–618, https://doi.org/10.1093/bioinformatics/btq725, 2011.
Heinonsalo, J., Sun, H., Santalahti, M., Bäcklund, K., Hari, P., and Pumpanen, J.: Evidences on the ability of mycorrhizal genus Piloderma to use organic nitrogen and deliver it to Scots pine, PLoS One, 10, 1–17, https://doi.org/10.1371/journal.pone.0131561, 2015.
Högberg, M. N., Högberg, P., and Myrold, D. D.: Is microbial community composition in boreal forest soils determined by pH, C-to-N ratio, the trees, or all three?, Oecologia, 150, 590–601, https://doi.org/10.1007/s00442-006-0562-5, 2007.
Högberg, M. N., Briones, M. J. I., Keel, S. G., Metcalfe, D. B., Campbell, C., Midwood, A. J., Thornton, B., Hurry, V., Linder, S., Näsholm, T., and Högberg, P.: Quantification of effects of season and nitrogen supply on tree below-ground carbon transfer to ectomycorrhizal fungi and other soil organisms in a boreal pine forest, New Phytol., 187, 485–493, https://doi.org/10.1111/j.1469-8137.2010.03274.x, 2010.
Ingestad, T.: Mineral nutrient requirements of Pinus silvestris and Picea abies seedlings, Physiol. Plant, 45, 373–380, https://doi.org/10.1016/0378-1127(92)90488-U, 1979.
Janssens, I. A., Dieleman, W., Luyssaert, S., Subke, J., Reichstein, M., Ceulemans, R., Ciais, P., Dolman, A. J., Grace, J., Matteucci, G., Papale, D., Piao, S. L., Schulze, E., Tang, J., and Law, B. E.: Reduction of forest soil respiration in response to nitrogen deposition, Nat. Geosci., 3, 315–322, https://doi.org/10.1038/ngeo844, 2010.
Jörgensen, K., Granath, G., Lindahl, B. D., and Strengbom, J.: Forest management to increase carbon sequestration in boreal Pinus sylvestris forests, Plant Soil, 466, 165–178, https://doi.org/10.1007/s11104-021-05038-0, 2021.
Karsch-Mizrachi, I., Nakamura, Y., and Cochrane, G.: The international nucleotide sequence database collaboration, Nucleic Acids Res., 40, 33–37, https://doi.org/10.1093/nar/gkr1006, 2012.
Kjøller, R., Nilsson, L. O., Hansen, K., Schmidt, I. K., Vesterdal, L., and Gundersen, P.: Dramatic changes in ectomycorrhizal community composition, root tip abundance and mycelial production along a stand-scale nitrogen deposition gradient, New Phytol., 194, 278–286, https://doi.org/10.1111/j.1469-8137.2011.04041.x, 2012.
Koide, R. T. and Malcolm, G. M.: N concentration controls decomposition rates of different strains of ectomycorrhizal fungi, Fungal Ecol., 2, 197–202, https://doi.org/10.1016/j.funeco.2009.06.001, 2009.
Kranabetter, J. M., Durall, D. M., and MacKenzie, W. H.: Diversity and species distribution of ectomycorrhizal fungi along productivity gradients of a southern boreal forest, Mycorrhiza, 19, 99–111, https://doi.org/10.1007/s00572-008-0208-z, 2009.
Kõljalg, U., Larsson, K. H., Abarenkov, K., Nilsson, R. H., Alexander, I. J., Eberhardt, U., Erland, S., Høiland, K., Kjøller, R., Larsson, E., Pennanen, T., Sen, R., Taylor, A. F. S., Tedersoo, L., Vrålstad, T., and Ursing, B. M.: UNITE: A database providing web-based methods for the molecular identification of ectomycorrhizal fungi, New Phytol., 166, 1063–1068, https://doi.org/10.1111/j.1469-8137.2005.01376.x, 2005.
Lê, S., Josse, J., and Husson, F.: FactoMineR: An R package for multivariate analysis, J. Stat. Softw., 25, 1–18, https://doi.org/10.18637/jss.v025.i01, 2008.
Leelamanie, D. A. L. and Liyanage, T. D. P.: Water repellent effects of manure amended soils on organic matter decomposition, C retention, and respired CO2-C, Biol., 71, 996–1001, https://doi.org/10.1515/biolog-2016-0127, 2016.
Linder, S.: Foliar analysis for detecting and correcting nutrient imbalances in Norway spruce, Ecol. Bull., 44, 178–190, 1995.
Lilleskov, E. A., Hobbie, E. A., and Fahey, T. J.: Ectomycorrhizal fungal taxa differing in response to nitrogen deposition also differ in pure culture organic nitrogen use and natural abundance of nitrogen isotopes, New Phytol., 154, 219–231, https://doi.org/10.1046/j.1469-8137.2002.00367.x, 2002a.
Lilleskov, E. A., Fahey, T. J., Horton, T. R., and Lovett, G. M.: Belowground ectomycorrhizal fungal community change over a nitrogen deposition gradient in alaska, Ecology, 83, 104–115, https://doi.org/10.1890/0012-9658(2002)083[0104:BEFCCO]2.0.CO;2, 2002b.
Lilleskov, E. A., Hobbie, E. A., and Horton, T. R.: Conservation of ectomycorrhizal fungi: Exploring the linkages between functional and taxonomic responses to anthropogenic N deposition, Fungal Ecol., 4, 174–183, https://doi.org/10.1016/j.funeco.2010.09.008, 2011.
Lindahl, B. D. and Tunlid, A.: Ectomycorrhizal fungi – potential organic matter decomposers, yet not saprotrophs, New Phytol., 205, 1443–1447, https://doi.org/10.1111/nph.13201, 2015.
Lindahl, B. D., Ihrmark, K., Boberg, J., Trumbore, S. E., Högberg, P., Stenlid, J., and Finlay, R. D.: Spatial separation of litter decomposition and mycorrhizal nitrogen uptake in a boreal forest, New Phytol., 173, 611–620, https://doi.org/10.1111/j.1469-8137.2006.01936.x, 2007.
Lindahl, B. D., Kyaschenko, J., Varenius, K., Clemmensen, K. E., Dahlberg, A., Karltun, E., and Stendahl, J.: A group of ectomycorrhizal fungi restricts organic matter accumulation in boreal forest, Ecol. Lett., 24, 1341–1351, https://doi.org/10.1111/ele.13746, 2021.
Litton, C. M., Raich, J. W., and Ryan, M. G.: Carbon allocation in forest ecosystems, Glob. Change Biol., 13, 2089–2109, https://doi.org/10.1111/j.1365-2486.2007.01420.x, 2007.
Mao, J., Nierop, K. G. J., Dekker, S. C., Dekker, L. W., and Chen, B.: Understanding the mechanisms of soil water repellency from nanoscale to ecosystem scale: a review, J. Soils Sediments, 19, 171–185, https://doi.org/10.1007/s11368-018-2195-9, 2019.
Mataix-Solera, J. and Doerr, S. H.: Hydrophobicity and aggregate stability in calcareous topsoils from fire-affected pine forests in southeastern Spain, Geoderma, 118, 77–88, https://doi.org/10.1016/S0016-7061(03)00185-X, 2004.
Nilsson, L. O. and Wallander, H.: Production of external mycelium by ectomycorrhizal fungi in a norway spruce forest was reduced in response to nitrogen fertilization, New Phytol., 158, 409–416, https://doi.org/10.1046/j.1469-8137.2003.00728.x, 2003.
Nguyen, R. T. and Harvey, H. R.: Preservation of protein in marine system: Hydrophobic and other noncovalent associations as major stabilizing forces, Geochim. Cosmochim. Ac., 65, 1467–1480, https://doi.org/10.1016/S0016-7037(00)00621-9, 2001.
Nguyen, R. T. and Harvey, H. R.: Preservation via macromolecular associations during Botryococcus braunii decay: Proteins in the Pula Kerogen, Org. Geochem., 34, 1391–1403, https://doi.org/10.1016/S0146-6380(03)00154-2, 2003.
Nicolás, C., Almeida, J. P., Ellström, M., Bahr, A., Bone, S. E., Rosenstock, N. P., Bargar, J. R., Tunlid, A., Persson, P., and Wallander, H.: Chemical changes in organic matter after fungal colonization in a nitrogen fertilized and unfertilized Norway spruce forest, Plant Soil, 419, 113–126, https://doi.org/10.1007/s11104-017-3324-8, 2017.
Oksanen, A. J., Blanchet, F. G., Friendly, M., Kindt, R., Legendre, P., Mcglinn, D., Minchin, P. R., Hara, R. B. O., Simpson, G. L., Solymos, P., Stevens, M. H. H., and Szoecs, E.: Package “ vegan”, https://CRAN.R-project.org/package=vegan (last access: 10 August 2022), 2017.
Pasanen, A. L., Yli-Pietilä, K., Pasanen, P., Kalliokoski, P., and Tarhanen, J.: Ergosterol content in various fungal species and biocontaminated building materials, Appl. Environ. Microb., 65, 138–142, https://doi.org/10.1128/aem.65.1.138-142.1999, 1999.
Parrent, J. L. and Vilgalys, R.: Biomass and compositional responses of ectomycorrhizal fungal hyphae to elevated CO2 and nitrogen fertilization, New Phytol., 176, 164–174, https://doi.org/10.1111/j.1469-8137.2007.02155.x, 2007.
R Core Team: R: A language and environment for statistical Computing, R Foundation for Statistical Computing, Vienna, Austria, https://www.R-project.org/ (last access: 10 August 2022), 2013.
Rillig, M. C., Mardatin, N. F., Leifheit, E. F., and Antunes, P. M.: Mycelium of arbuscular mycorrhizal fungi increases soil water repellency and is sufficient to maintain water-stable soil aggregates, Soil Biol. Biochem., 42, 1189–1191, https://doi.org/10.1016/j.soilbio.2010.03.027, 2010.
Schurig, C., Smittenberg, R. H., Berger, J., Kraft, F., Woche, S. K., Goebel, M. O., Heipieper, H. J., Miltner, A., and Kaestner, M.: Microbial cell-envelope fragments and the formation of soil organic matter: A case study from a glacier forefield, Biogeochemistry, 113, 595–612, https://doi.org/10.1007/s10533-012-9791-3, 2013.
Shah, F., Schwenk, D., Nicolás, C., Persson, P., Hoffmeister, D., and Tunlid, A.: Involutin is an Fe3+ reductant secreted by the ectomycorrhizal fungus Paxillus involutus during Fenton-based decomposition of organic matter, Appl. Environ. Microb., 81, 8427–8433, https://doi.org/10.1128/AEM.02312-15, 2015.
Schloss, P. D., Westcott, S. L., Ryabin, T., Hall, J. R., Hartmann, M., Hollister, E. B., Lesniewski, R. A., Oakley, B. B., Parks, D. H., Robinson, C. J., Sahl, J. W., Stres, B., Thallinger, G. G., Van Horn, D. J., and Weber, C. F.: Introducing mothur: Open-source, platform-independent, community-supported software for describing and comparing microbial communities, Appl. Environ. Microb., 75, 7537–7541, https://doi.org/10.1128/AEM.01541-09, 2009.
Schreiner, T., Hildebrandt, U., Bothe, H., and Marner, F. J.: Chemical and biological characterization of corticrocin, a yellow pigment formed by the ectomycorrhizal fungus Piloderma croceum, Z. Naturforsch. C, 53, 4–8, https://doi.org/10.1515/znc-1998-1-203, 1998.
Šimon, T., Javůrek, M., Mikanová, O., and Vach, M.: The influence of tillage systems on soil organic matter and soil hydrophobicity, Soil Till. Res., 105, 44–48, https://doi.org/10.1016/j.still.2009.05.004, 2009.
Smith, S. E. and Read, D. J.: Mycorrhizal Symbiosis, 3rd Edn., Academic Press, London, https://doi.org/10.1016/B978-0-12-370526-6.X5001-6, 2008.
Taylor, A. F. S., Martin, F., and Read, D. J.: Fungal Diversity in Ectomycorrhizal Communities of Norway Spruce [Picea abies (L.) Karst.] and Beech (Fagus sylvatica L.) Along North-South Transects in Europe, Carbon and Nitrogen Cycling in European Forest Ecosystems, 142, 343–365, https://doi.org/10.1007/978-3-642-57219-7_16, 2000.
Tedersoo, L., Suvi, T., Jairus, T., and Kõljalg, U.: Forest microsite effects on community composition of ectomycorrhizal fungi on seedlings of Picea abies and Betula pendula, Environ. Microbiol., 10, 1189–1201, https://doi.org/10.1111/j.1462-2920.2007.01535.x, 2008.
Tedersoo, L., May, T. W., and Smith, M. E.: Ectomycorrhizal lifestyle in fungi: global diversity, distribution, and evolution of phylogenetic lineages, Mycorrhiza, 20, 217–263, https://doi.org/10.1007/s00572-009-0274-x, 2010.
Teste, F. P., Lieffers, V. J., and Strelkov, S. E.: Ectomycorrhizal community responses to intensive forest management: Thinning alters impacts of fertilization, Plant Soil, 360, 333–347, https://doi.org/10.1007/s11104-012-1231-6, 2012.
Toljander, J. F., Eberhardt, U., Toljander, Y. K., Paul, L. R., and Taylor, A. F. S.: Species composition of an ectomycorrhizal fungal community along a local nutrient gradient in a boreal forest, New Phytol., 170, 873–884, https://doi.org/10.1111/j.1469-8137.2006.01718.x, 2006.
Treseder, K. K.: A meta-analysis of mycorrhizal responses to nitrogen, phosphorus, and atmospheric CO2 in field studies, New Phytol., 164, 347–355, https://doi.org/10.1111/j.1469-8137.2004.01159.x, 2004.
Unestam, T. and Sun, Y. P.: Extramatrical structures of hydrophobic and hydrophilic ectomycorrhizal fungi, Mycorrhiza, 5, 301–311, https://doi.org/10.1007/BF00207402, 1995.
Vogelmann, E. S., Reichert, J. M., Prevedello, J., and Awe, G. O.: Hydro-physical processes and soil properties correlated with origin of soil hydrophobicity, Ciênc. Rural, 43, 1582–1589, https://doi.org/10.1590/s0103-84782013005000107, 2013.
Walker, J. K. M. and Jones, M. D.: Little evidence for niche partitioning among ectomycorrhizal fungi on spruce seedlings planted in decayed wood versus mineral soil microsites, Oecologia, Botany, 173, 1499–1511, https://doi.org/10.1007/s00442-013-2713-9, 2013.
Walker, J. K. M., Phillips, L. A., and Jones, M. D.: Ectomycorrhizal fungal hyphae communities vary more along a pH and nitrogen gradient than between decayed wood and mineral soil microsites, 92, 453–463, https://doi.org/10.1139/cjb-2013-0239, 2014.
Wallander, H., Nilsson, L. O., Hagerberg, D., and Bååth, E.: Estimation of the biomass and seasonal growth of external mycelium of ectomycorrhizal fungi in the field, New Phytol., 151, 753–760, https://doi.org/10.1046/j.0028-646x.2001.00199.x, 2001.
Wallander, H., Nilsson, L. O., Hagerberg, D., and Rosengren, U.: Direct estimates of C : N ratios of ectomycorrhizal mycelia collected from Norway spruce forest soils, Soil Biol. Biochem., 35, 997–999, https://doi.org/10.1016/S0038-0717(03)00121-4, 2003.
Wallander, H., Johansson, U., Sterkenburg, E., Brandström Durling, M., and Lindahl, B. D.: Production of ectomycorrhizal mycelium peaks during canopy closure in Norway spruce forests, New Phytol., 187, 1124–1134, https://doi.org/10.1111/j.1469-8137.2010.03324.x, 2010.
Wallander, H., Ekblad, A., and Bergh, J.: Growth and carbon sequestration by ectomycorrhizal fungi in intensively fertilized Norway spruce forests, Forest Ecol. Manage., 262, 999–1007, https://doi.org/10.1016/j.foreco.2011.05.035, 2011.
White, T. J.: Amplification and Direct Sequencing of Fungal Ribosomal RNA Genes for Phylogenetics, in: PCR Protocols, a Guide to Methods and Applications, edited by: Innis, M. A., Gelfand, D. H., Sninsky, J. J., and White, T. J., Academic press, Inc., New York, 315–322, https://doi.org/10.1016/B978-0-12-372180-8.50042-1, 1990.
Von Lützow, M., Kögel-Knabner, I., Ludwig, B., Matzner, E., Flessa, H., Ekschmitt, K., Guggenberger, G., Marschner, B., and Kalbitz, K.: Stabilization mechanisms of organic matter in four temperate soils: Development and application of a conceptual model, J. Plant Nutr. Soil Sc., 171, 111–124, https://doi.org/10.1002/jpln.200700047, 2008.
Wallenda, T. and Kottke, I.: Nitrogen deposition and ectomycorrhizas, New Phytol., 139, 169–187, https://doi.org/10.1046/j.1469-8137.1998.00176.x, 1998.
Whitney, K. D. and Arnott, H. J.: Calcium Oxalate Crystal Morphology and Development in Agaricus Bisporus, Mycologia, 79, 180–187, https://doi.org/10.1080/00275514.1987.12025696, 1987.
Woche, S. K., Goebel, M. O., Mikutta, R., Schurig, C., Kaestner, M., Guggenberger, G., and Bachmann, J.: Soil wettability can be explained by the chemical composition of particle interfaces-An XPS study, Sci. Rep.-UK, 7, 1–8, https://doi.org/10.1038/srep42877, 2017.
Zheng, W., Morris, E. K., and Rillig, M. C.: Ectomycorrhizal fungi in association with Pinus sylvestris seedlings promote soil aggregation and soil water repellency, Soil Biol. Biochem., 78, 326–331, https://doi.org/10.1016/j.soilbio.2014.07.015, 2014.