the Creative Commons Attribution 4.0 License.
the Creative Commons Attribution 4.0 License.
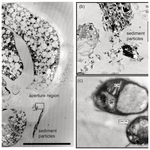
Deposit-feeding of Nonionellina labradorica (foraminifera) from an Arctic methane seep site and possible association with a methanotroph
Christiane Schmidt
Emmanuelle Geslin
Joan M. Bernhard
Charlotte LeKieffre
Mette Marianne Svenning
Helene Roberge
Magali Schweizer
Giuliana Panieri
Several foraminifera are deposit feeders that consume organic detritus (dead particulate organic material with entrained bacteria). However, the role of such foraminifera in the benthic food web remains understudied. Foraminifera feeding on methanotrophic bacteria, which are 13C-depleted, may cause negative cytoplasmic and/or calcitic δ13C values. To test whether the foraminiferal diet includes methanotrophs, we performed a short-term (20 h) feeding experiment with Nonionellina labradorica from an active Arctic methane-emission site (Storfjordrenna, Barents Sea) using the marine methanotroph Methyloprofundus sedimenti and analysed N. labradorica cytology via transmission electron microscopy (TEM). We hypothesised that M. sedimenti would be visible post-experiment in degradation vacuoles, as evidenced by their ultrastructure. Sediment grains (mostly clay) occurred inside one or several degradation vacuoles in all foraminifers. In 24 % of the specimens from the feeding experiment degradation vacuoles also contained bacteria, although none could be confirmed to be the offered M. sedimenti. Observations of the apertural area after 20 h incubation revealed three putative methanotrophs, close to clay particles, based on bacterial ultrastructural characteristics. Furthermore, we noted the absence of bacterial endobionts in all examined N. labradorica but confirmed the presence of kleptoplasts, which were often partially degraded. In sum, we suggest that M. sedimenti can be consumed via untargeted grazing in seeps and that N. labradorica can be generally classified as a deposit feeder at this Arctic site.
- Article
(10947 KB) - Full-text XML
-
Supplement
(1173 KB) - BibTeX
- EndNote
In methane seep sites, the upward migration of methane affects the pore-water chemistry of near-surface sediments, where benthic foraminifera live (e.g. Dessandier et al., 2019). Extremely light isotopic signals of δ13C have been measured in seep-associated foraminiferal calcite tests (Wefer et al., 1994; Rathburn et al., 2003; Hill et al., 2004b; Panieri et al., 2014). Studies specifically looking at living (rose bengal stained) foraminiferal tests support the hypothesis that the carbon isotopic composition is strongly influenced by the porewater dissolved inorganic carbon (DIC) (McCorkle et al., 1990). Interspecific δ13C differences between species with similar depth indicate sometimes taxon-specific “vital” effects (McCorkle et al., 1990). Those “vital” effects describe the biology of the different species, which could reflect different feeding patterns. It has been suggested that Nonionella auris is an indicator of methane release and possibly ingests 13C-depleted methane-oxidising bacteria (Wefer et al., 1994). Recently, Melonis barleeanus (Williamson, 1858) collected from an active methane seep site was found to be closely associated with putative methanotrophs (Bernhard and Panieri, 2018), providing impetus to examine feeding habits of foraminifera living in or around methane seeps.
Methanotrophs produce the biomarker diplopterol, which has an extremely light δ13C signature (−60 ‰) (Hinrichs et al., 2003). Our hypothesis is that if foraminifera ingest methanotrophs, δ13C values of foraminiferal cytoplasm should be altered by their diet. Experiments using a high-pressure culturing system revealed the difficulty of measuring the sensitive relationship between methane exposure and the foraminifera Cibicides wuellerstorfi. However, it was shown in one experiment using entire cores that a methane source was reflected in δ13C of foraminiferal calcite (Wollenburg et al., 2015). It is also not yet conclusive if diet can influence foraminiferal calcite as new calcite did not form during experiments (Mojtahid et al., 2011).
Another hypothesis to explain extremely light δ13C values recorded in benthic foraminiferal calcite is that foraminifera assimilate carbon as 13C-depleted methane-derived DIC, which would lead to extremely light δ13C values. The possibility that 13C-depleted DIC from the pore water can be assimilated by foraminifera is currently debated. Some studies suggest it is not possible (Herguera et al., 2014), while others assert the feasibility that foraminifera calcify close to seeps (Rathburn et al., 2003; Hill et al., 2004a; Panieri et al., 2014). The problem lies in the calcite tests and the difficulty of assessing the time of death of these protists in the sediment. Several studies found that the lightest isotopic δ13C values were measured in tests coated with methane-derived authigenic carbonate (MDAC) overgrowth, which happens after the death of the foraminifer (Torres et al., 2010; Panieri et al., 2014; Consolaro et al., 2015; Panieri et al., 2017; Schneider et al., 2017). However, light δ13C values remain in many tests after MDACs are removed (Panieri et al., 2014) and have been measured also in primary calcite, without MDACs, from tests in methane-rich environments (e.g. Mackensen, 2008; Dessandier et al., 2019). These observations again point to the role of food influencing the cytoplasmic δ13C.
Foraminifera play an important role in the carbon cycle on the deep seafloor (Nomaki et al., 2005) where feeding behaviour and food preference vary with species (Nomaki et al., 2006). Selected species of deep-sea benthic foraminifera have been shown to feed selectively on 13C-labelled algae from sedimentary organic matter but unselectively on 13C-labelled bacteria of the strain Vibrio (Nomaki et al., 2006). A study from the seafloor around Adriatic seeps suggested that δ13C of foraminiferal cytoplasm could be influenced by feeding on the sulfur-oxidising bacterium Beggiatoa, whose abundance was also positively correlated with foraminiferal densities (Panieri, 2006). Benthic foraminifera have a variety of different trophic strategies and consume many kinds of foods. There are deposit feeders, exclusively herbivorous or carnivorous species, and suspension feeders ingesting dissolved organic matter (DOM) (reviewed in Lipps, 1983). Deposit feeders are omnivorous, gathering fine-grained sediment (e.g. clay) and associated bacteria, organic detritus (dead particulate organic material), and, if present, diatom cells using their pseudopodia. Based on the ultrastructure of the diet found in vacuoles several species of foraminifera from different habitats have already been classified to be deposit feeders (Goldstein and Corliss, 1994).
Here we investigate if Nonionellina labradorica would feed in a short-term feeding experiment on the marine methanotroph Methyloprofundus sedimenti and compare its ultrastructure on experimental specimens and field specimens. Nonionellina labradorica is an abundant species in the North Atlantic (Cedhagen, 1991) and occurs together with N. digitata in Svalbard fjord sediments (Hald and Korsun, 1997; Shetye et al., 2011; Fossile et al., 2020). In addition to its wide distribution, it is an especially interesting experimental species for feeding studies because it hosts kleptoplasts, i.e. sequestered chloroplasts, of diatom origin inside its cytoplasm (Cedhagen, 1991; Jauffrais et al., 2019b). Nonionellina labradorica's aperture shows a specific ornamentation, possibly a morphological adaptation to this “predatory” mode of life for obtaining the kleptoplasts (Bernhard and Bowser, 1999). Denitrification has been speculated for N. labradorica (reviewed in Charrieau et al., 2019) because the foraminiferal genus Nonionella can denitrify, which was demonstrated on two species (Risgaard-Petersen et al., 2006; Choquel et al., 2021) but not yet on N. labradorica. Our study analysed contents of the degradation vacuoles of this species from an active methane-emitting site in the Arctic (Storfjordrenna, Barents Sea) before and after a feeding experiment.
2.1 Site description and sampling living foraminifera
The sampling site was located approx. 50 km south of Svalbard at 382 m water depth at the mouth of Storfjordrenna (Serov et al., 2017). The site is characterised by several large gas hydrate pingos (GHPs), which actively vent methane over an area of 2.5 km2. Our samples were taken at GHP3, which is referred to as an underwater gas-hydrate-bearing mound (Hong et al., 2017, 2018). GHP3 is a ∼ 500 m diameter and 10 m tall mound that actively vents methane (Fig. 1). Marine sediment samples were collected during CAGE cruise 18-05 supported by the research vessel Kronprins Haakon in October 2018 and sampled by the remotely operated vehicle (ROV) Ægir. A blade corer (BLC18; surface dimensions 27×19 cm, Fig. 1c) was used to retrieve marine sediment in the vicinity of bacterial mats (GPS 76∘6′23.7′′ N, 15∘58′1.7′′ E). Once on board the blade corer was immediately sampled to retrieve living (cytoplasm containing) foraminifera using a small aquarium hose targeting the first centimetre (∼ 0–1 cm). The sediment was collected in petri dishes and wet sieved to a size range of 250–500 µm. The species N. labradorica, which was abundant in that layer, was subsequently used for a feeding experiment described in detail below.
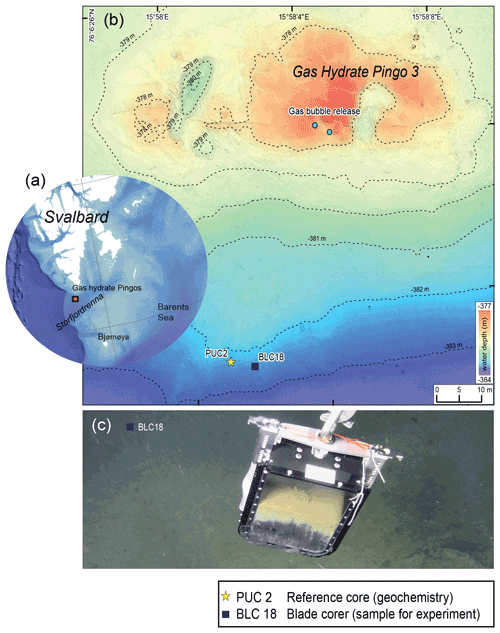
Figure 1Description of the sampling site Gas Hydrate Pingo 3 (GHP3), a gas-hydrate-bearing mound, located in Storfjordrenna, Barents Sea. (a) Map illustrating Svalbard archipelago and the sampling site, approx. 50 km offshore. (b) Map of sampling site GHP3; active gas bubble release is marked on the top of the underwater mount, the yellow star indicates location of push corer PUC2 (geochemical analyses), and the black square indicates location of BLC18 (sediment source for experiment). (c) Underwater image of retrieval of BLC18 taken by ROV camera illustrating the colouration of sediment with the sea floor visible in background.
2.2 Geochemistry of the study site
For geochemical analysis of the study site a push corer (PUC2; henceforth referred to as geochemistry core) was taken to obtain measurements of δ13CDIC and sulfate because the blade corer (BLC18) did not allow those measurements. PUC2 was taken in close vicinity to BLC18, ∼ 5 m apart (see Fig. S1 in the Supplement). Pore-water samples were taken from PUC2 using rhizons that were inserted through pre-drilled holes in the core tube at intervals of 1 cm (Table S1). Acid-washed 20 mL syringes were attached to the rhizons for pore-water collection. Depending on the amount of pore water collected, the samples were split for δ13CDIC and sulfate measurements. To the samples, 10 µL of saturated HgCl2 (aqueous) was added to stop microbial activity and stored in cold conditions (5 ∘C). A Thermo Scientific GasBench II coupled to a Thermo Scientific MAT 253 isotope-ratio mass spectrometer (IRMS) at the Stable Isotope Laboratory (SIL) at CAGE, UiT The Arctic University of Norway, was used to determine δ13CDIC of the pore water. Anhydrous phosphoric acid was added to small glass vials (volume 4.5 mL) that were closed and flushed with helium 5.0 gas before the pore-water sub-sample was measured. A porewater sub-sample (volume 0.5 mL) was then added through the septa with a syringe needle, followed by equilibration for 24 h at 24 ∘C to liberate the CO2 gas. Three solid calcite standards with a range of +2 to −49 ‰ were used for normalisation to δ13C Vienna Pee Dee Belemnite (VPDB). Correction of measured δ13C by −0.1 ‰ was done to account for fractionation between gas and aqueous in sample vials. Instrument precision for δ13C on a MAT253 IRMS was ±0.1 ‰ (SD). Sulfate was measured with a Metrohm ion chromatography instrument equipped with column Metrosep A Supp 4 and eluted with 1.8 mmol L−1 Na2CO3+1.7 mmol L−1 NaHCO3 at the University of Bergen.
2.3 Culturing of the marine methanotroph M. sedimenti
Methyloprofundus sedimenti PKF-14 had been previously isolated from a water-column sample collected at Prins Karls Forland, Svalbard, in the laboratory at UiT in Tromsø. Methyloprofundus sedimenti were cultured in 10 mL batches of a 35:65 mix of nitrate mineral salt medium (NMS) and sterile filtered seawater using 125 mL Wheaton® serum bottles with butyl septa and aluminium crimp caps (Teknolab®). Methane was injected to give a headspace of 20 % methane in air, and the bottles were incubated without shaking at 15 ∘C in darkness. Purity of the cultures and cell integrity was verified by microscopy and by absence of growth on agar plates with a general medium for heterotrophic bacteria (tryptone, yeast extract, glucose, and agar).
2.4 Experimental set-up
On the ship, N. labradorica (Fig. 2a, b) specimens showing dark greenish-brown cytoplasm were picked using sable artist brushes under a stereomicroscope immediately after wet sieving the sediment using natural seawater delivered from the ship pump. Living specimens had a partly inorganic covering surrounding the test, which was gently removed using fine artist brushes. Those so-called cysts are nothing unusual with many foraminiferan taxa (Heinz et al., 2005).
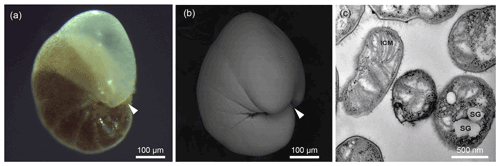
Figure 2Exemplary illustration of Nonionellina labradorica, utilised in this study. (a) Reflected light microscopy image from a specimen directly after sampling; the white arrowhead indicates aperture location. (b) Scanning electron image from a specimen before molecular analysis was performed; the white arrowhead indicates aperture location. (c) Transmission electron microscopy image of a culture of Methyloprofundus sedimenti, the marine methanotroph used in the feeding experiment. The characteristic features for methanotroph identification are the typical type I intracytoplasmic membranes (ICMs). Furthermore, other internal structures visible are storage granules (SGs) and a gram-negative cell wall (GNCW).
Our specimens were subsequently rinsed twice in filtered artificial seawater to remove any sediment before placing them into the experimental petri dishes. Care was taken that those were minimally exposed to light during preparation of the experiment as kleptoplasts are known to be highly light sensitive in this foraminifer (Jauffrais et al., 2019b).
The experiment with M. sedimenti was conducted for a total duration of 20 h to resemble previous experiments on N. labradorica using transmission electron microscopy (TEM) and nanometre-scale secondary ion mass spectrometry isotopic imaging (NanoSIMS) (Jauffrais et al., 2019b), and it included two more time points at 4 and 8 h. A short pre-experimental phase (2–4 h) was included before the start of the feeding experiment to allow specimens to acclimate. During the pre-experimental phase specimens were not fed, and they resided in the petri dishes to adjust to the experimental conditions. The feeding experiment consisted of several small petri dishes (3.5 cm ∅, 3 mL) each containing five N. labradorica in artificial seawater (ASW) at ambient salinity 35 (Red Sea Salt). Petri dishes were sealed with Parafilm® and covered with aluminium foil and placed inside the incubator in complete darkness. Temperature inside the chamber was maintained at 2–3 ∘C, which is within the range of the site's bottom-water temperature (−1.8–4.6 ∘C) (Hong et al., 2017). The feeding of M. sedimenti was performed once at the beginning of the experiment by adding 100 µL of culture to 3 mL of artificial seawater to produce a final concentration of ∼ 1×106 bacteria mL−1 in each petri dish. Previously conducted feeding studies were used as guides: Muller and Lee (1969) used 1×l04 bacteria mL−1 seawater and Mojtahid et al. (2011) used 4×108 bacteria mL−1 seawater.
Five foraminifera, which served as initial/field specimens (Table 1), were fixed without M. sedimenti incubation. The respective petri dishes were incubated for 4, 8, and 20 h to determine if incubation duration influenced response of the foraminifera to the methanotroph. One petri dish containing five foraminifera, which were un-fed and fixed at 20 h, served as a negative “control”. After the end of the respective incubation times, each foraminifer was picked with a sterilised fine artist brush, which was cleaned in 70 % ethanol between each specimen, and placed individually into a fixative solution (4 % glutaraldehyde and 2 % paraformaldehyde dissolved in ASW).
Table 1Summary of TEM observations of Nonionellina labradorica comparing field specimens and experimental specimens. Field specimens (initials) were not fed, and a non-fed control was not preserved after a 20 h incubation. The only putative methanotrophs were observed and imaged in specimens from the 20 h incubation. Bacteria of unknown origin were described as rod-shaped cells in the degradation vacuoles.
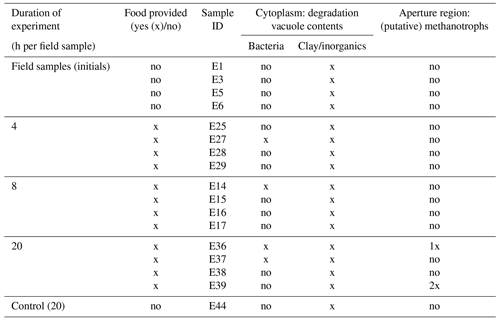
2.5 Transmission electron microscopy (TEM) preparation
Samples of N. labradorica preserved in fixative solution were transported to the University of Angers, where they were prepared for ultrastructural analysis using established protocols (Lekieffre et al., 2018). Four embedded foraminiferal cells per treatment were sectioned using an ultramicrotome (Leica® Ultracut S) equipped with a diamond knife (Diatome®, ultra 45∘). Grids were stained using UranyLess® EM Stain (EMS, USA). Ultra-thin sections (70 nm) were observed with a JEOL JEM-1400 TEM at the SCIAM facility, University of Angers.
To document the ultrastructure of M. sedimenti, a sub-sample of the culture used for experiments was imaged with TEM (Fig. 2c). To do so, an exponentially growing culture was collected, centrifuged, pre-fixed with 2.5 % () glutaraldehyde in growth medium overnight, washed in PBS (phosphate buffered saline), and then post-fixed with 1 % () aqueous osmium tetroxide for 1.5 h at room temperature. After dehydration in an ethanol series, the samples were embedded in an EPON-equivalent (SERVA) epoxy resin. Ultra-thin sections were cut on a Leica EM UC6 ultramicrotome and stained with 3 % () aqueous uranyl acetate followed by staining with lead citrate (Reynolds, 1963) at 20 ∘C for 4–5 min. The samples were examined with a JEOL JEM-1010 transmission electron microscope at an accelerating voltage of 80 kV with a Morada camera system at the Advanced Microscopy Core Facility (AMCF), Faculty of Health Science, UiT The Arctic University of Norway.
2.6 Foraminifera ultrastructural observation and image processing
Four specimens per experimental time point (initials, 4, 8, and 20 h) plus one un-fed (control) specimen were examined with the TEM. From each specimen, a minimum of 50 TEM images were taken, including images detailing the degradation vacuoles (approx. 5–27 images per specimen). Before the ultrastructure was examined in detail, an overview images was created of each section to illustrate the number of chambers and size of the specimen. Images were blended together using Photoshop CS5 (see Fig. 4–5a). Thereafter, the ultrastructure was examined at different parts of the cell: (a) in the interior to document vitality, (b) on degradation vacuoles to determine their contents, and (c) at the exterior to survey for microbes entrained in remnant “reticulopodial trunk” material. All images made during the observations of the TEM sections are deposited at Zenodo (https://doi.org/10.5281/zenodo.6941739; Schmidt et al., 2022).
2.7 Molecular genetics and morphology
DNA metabarcoding and morphological documentation were performed on 13 specimens of N. labradorica. Briefly, live specimens were dried on micropalaeontological slides and transported in a small container, cooled with ice-pads, to the University of Angers. All specimens were imaged for morphological analysis using a scanning electron microscope (SEM; EVOLS10, ZEISS, Fig. S1) followed by total DNA being individually extracted in dissolved organic carbon (DOC) buffer (Pawlowski, 2000). To amplify foraminiferal DNA, a hot start polymerase chain reaction (PCR; 2 min at 95 ∘C) was performed in a volume of 25 µL with 40 cycles of 30 s at 95 ∘C, 30 s at 50 ∘C, and 2 min at 72 ∘C, followed by 10 min at 72 ∘C for final extension. Primers s14F3 and sB were used for the first PCR and 30 cycles at an annealing temperature of 52 ∘C (other parameters unchanged) for the nested PCR with primers s14F1 and J2 (Pawlowski, 2000; Darling et al., 2016). Positive amplifications were sequenced directly with the Sanger method at Eurofins Genomics (Cologne, Germany). For taxonomic identification, DNA sequences were compared first with BLAST (basic local alignment search tool) (Altschul et al., 1997) and then within an alignment comprising other nonionids implemented in SeaView (Gouy et al., 2010) and corrected manually.
3.1 Sample description and geochemistry of the study site
The visual observation of the sediments within the blade corer BLC18 immediately after sampling (Fig. 1c) indicated that the sediment appeared light grey to yellowish in the upper part until approx. 13 cm and dark brown from approx. 13 cm to the bottom. The sulfate measured in the pore water of the geochemistry core (PUC2) declined from ∼ 2750 ppm at the sediment–water interface to ∼ 706 ppm at approximately 13 cm (see Fig. S1, Table S1). A decline in sulfate concentration indicates that the anaerobic oxidation of methane (AOM) occurred at approx. 13 cm depth. The sulfate–methane transition zone (SMTZ) characterised by a DIC value of −32 ‰ at approx. 13 cm sediment depth can be considered shallow based on the global average (Egger et al., 2018).
3.2 Ultrastructure of methanotroph culture used in the feeding experiment
Transmission electron microscopy was performed on culture aliquots to allow morphological comparison to previously published work (Tavormina et al., 2015). Methyloprofundus sedimenti strain PKF-14 cells are coccoid to slightly elongated in shape and are characterised by typical type I stacked intracytoplasmic membranes (ICMs) (Fig. 2c). It has storage granules (SGs) and a gram-negative cell wall (GNCW), which are not uniquely characteristic of methanotrophs (Fig. 2c). Additionally, 16S rRNA gene sequencing was performed (data not shown) to confirm it to be similar to the published M. sedimenti (Tavormina et al., 2015).
3.3 Foraminiferal ultrastructure from an Arctic seep environment
3.3.1 General ultrastructure
All 17 specimens examined for ultrastructure were considered living at the time of observation (Fig. 3) as the mitochondria had characteristic double membranes and occasionally visible cristae (Nomaki et al., 2016). Cytoplasm exhibited several vacuoles and kleptoplasts concentrated in the youngest chambers (Fig. 3a), and, in some specimens, a nucleus with nucleoli was visible (Fig. 3b). Kleptoplasts were numerous throughout the cytoplasm and occurred in the form of a single chloroplast (Fig. 3a–b) or as double chloroplasts (Fig. S2a–d). Not all kleptoplasts were intact; some showed peripheral degradation of the membranes indicated by an increasing number of white areas between pyrenoid, lamella, and thylakoids (Fig. S2a–d). The mitochondria occurred often in small clusters of two to five throughout the cytoplasm and were oval, round, or kidney-shaped in cross section (Fig. 3e–f). Peroxisomes in N. labradorica occurred mostly as pairs (Fig. 3c) or small clusters of three to four spherical organelles (Fig. S3a). Sometimes, but not always, peroxisomes were associated with endoplasmic reticulum (Fig. S3b) but could also occur alone. Golgi apparatus (Fig. 3d) had intact membranes, often occurring near mitochondria.
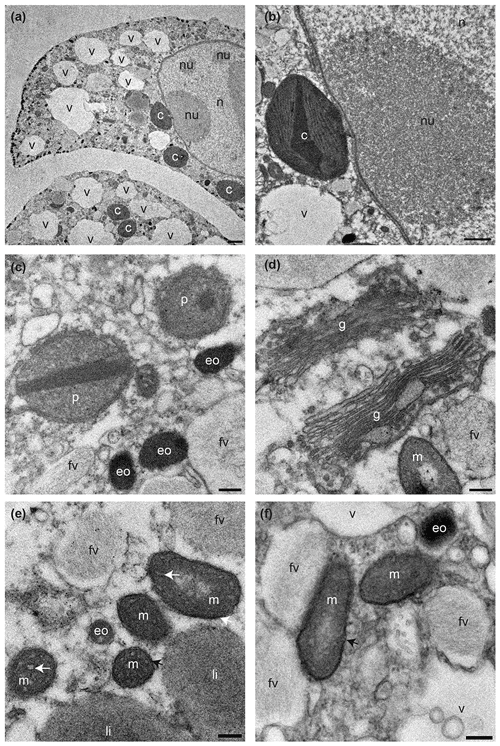
Figure 3Transmission electron micrographs showing cellular ultrastructure of N. labradorica. (a) Cytoplasm showing parts of two chambers, with nucleus with nucleoli, vacuoles, and several kleptoplasts, (b) nuclear envelope, nucleoli, and kleptoplasts, (c) peroxisomes and electron opaque bodies, (d) Golgi, and (e–f) mitochondria. V = vacuole, c = kleptoplast, nu = nucleoli, n = nucleus p = peroxisome, eo=electron opaque body, m = mitochondrion, fv = fibrillar vesicle, li = lipid droplet. Scales: (a) 2 µm, (b) 1 µm, (c–f) 200 nm.
3.3.2 Ultrastructure of aperture-associated bacteria
In total, three putative methanotrophs were identified in the vicinity of two specimens (sample E39, Fig. 4; E37, Fig. 5). These microbes were identified adjacent to reticulopodial remains (Fig. 4b). As an aid for identification of M. sedimenti we used the characteristics shown in the literature (Tavormina et al., 2015) and our own TEM observation obtained from M. sedimenti culture (Fig. 2c). As noted, M. sedimenti is characterised by a typical type I intracytoplasmic stacked membrane (ISM). Other characteristics, which are not specific for methanotrophs, included storage granules (SGs) and a typical gram-negative cell wall (GNCW) (Fig. 2c). On specimen E39 from the 20 h treatment, we found the methanotroph exhibiting the clearest internal structure, having both typical type I intracytoplasmic stacked membranes (ISM) and SGs (Fig. 4c).
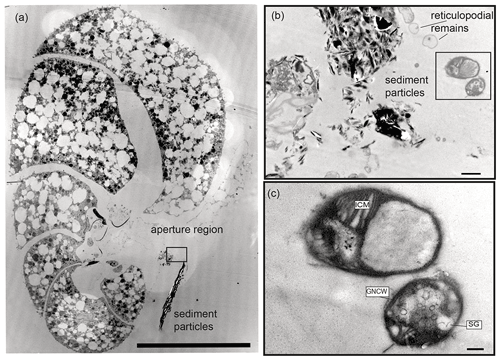
Figure 4Transmission electron micrographs of N. labradorica from 20 h treatment (sample E39). (a) Stitched cross section of TEM images showing location of methanotroph at the aperture region (black rectangle is the location of image shown in panel b). (b) Location of two putative methanotrophs next to sediment particles and putative reticulopodial remains (black rectangle is location of image shown in panel c). (c) Close-up of two putative methanotrophs revealing detailed features for identification, such as typical type I stacked intracytoplasmic membranes (ICMs), and other characteristics, such as storage granules (SGs) and gram-negative cell wall (GNCW). Scale bars: (a) 100 µm, (b) 1 µm, (c) 200 nm.
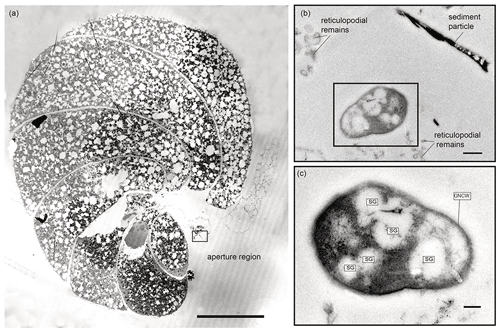
Figure 5Transmission electron micrographs of N. labradorica from 20 h treatment (sample E37). (a) Stitched cross section of TEM images showing location of putative methanotroph (black rectangle) at the aperture region. (b) Location of the putative methanotroph next to sediment particles and putative reticulopodial remains. (c) Close-up of putative methanotroph showing several storage granules (SGs) throughout its cell, which is surrounded by a gram-negative cell wall (GNCW). Scale bars: (a) 100 µm, (b) 0.5 µm, (c) 200 nm.
3.3.3 Contents of degradation vacuoles
Digestive vacuoles and food vacuoles are often summarised as degradation vacuoles in the literature (Lekieffre et al., 2018), and this makes sense for our study as well. A degradation vacuole is a vacuole where enzymatic activities degrade contents, often making them unidentifiable (Bé et al., 1982; Hemleben et al., 1989). Sediment particles were present in many degradation vacuoles. The sediment grains were easy to recognise in the TEM image as angular grains inside the vacuoles, next to organic debris, which can have many different shapes. Each specimen had at least one degradation vacuole and mostly several, which were filled with sediment particles (Table 1). If a sediment particle was visible, the vacuole was defined as a degradation vacuole (dv), and if it was not and empty, then it was defined as a standard vacuole (v) (Fig. 6). The observed entrained sediment particles were platelets, likely clay from the seafloor, and hence show that the vacuole must contain foreign objects, around which degradation processes have started. A total of 4 of 17 specimens examined (23 %) had one or more bacteria of various sizes inside their degradation vacuoles next to sediment particles (Fig. 6c, f).
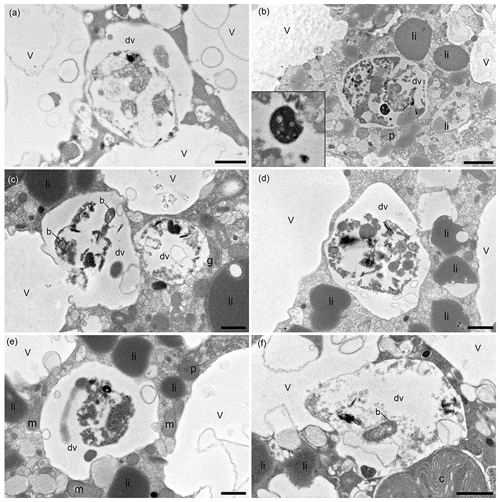
Figure 6TEM micrographs of N. labradorica showing degradation vacuoles containing miscellaneous items, including bacteria (b), inorganics (clay platelets), and unidentifiable remains after 4 h incubation, which are shown enlarged on the left side of the image in a zoomed-in window (a, b; specimens E27, E28, respectively), after 8 h incubation (c, d; specimen E14), and after 20 h incubation (e, f; specimens E36, E37, respectively). v = vacuole, dv = degradation vacuole, c = kleptoplast, p = peroxisome, m = mitochondrion, li = lipid, g = Golgi. Scales: (a, c–f) 1 µm, (b) 2 µm.
3.4 Foraminiferal genetics
A total of 6 of 13 specimens analysed for genetics were positively amplified and sequenced (Fig. S4). The sequences are deposited in GenBank under the accession numbers MN514777 to MN514782. When comparing them via BLAST, they were between 98.6 % and 99.6 % identical to published sequences belonging to foraminifera identified as the morphospecies N. labradorica from the Skagerrak, Svalbard, and the White Sea (Holzmann and Pawlowski, 2017; Jauffrais et al., 2019b). Sequences were also included in an alignment comprising other nonionids implemented in SeaView (not shown) and corrected manually to check the BLAST search. This step confirmed the BLAST identification.
4.1 Sampling site and geochemistry
The sampling site of blade corer BLC18 was in close proximity (∼ 50 m) to an active methane vent releasing methane bubbles at the gas hydrate pingo (GHP3) (Serov et al., 2017). At such sites with high methane fluxes, the SMTZ (sulfate–methane transition zone) is shallow as sulfate in the sediment is readily consumed in the first tens of centimetres (Barnes and Goldberg, 1976; Iversen and Jørgensen, 1993) by sulfate-reducing bacteria (SRB) (reviewed in Carrier et al., 2020). Geochemical analysis of PUC2 revealed an SMTZ at approx. 13 cm, which is rather shallow (Egger et al., 2018) as it can also be several metres deep in other sites (reviewed in Panieri et al., 2017). Similar geochemical characteristics can be considered at the sampling location of living specimens (BLC18) given the close proximity of the two locations. The geochemical data at PUC2 allow us conclude that the site where living foraminifera were collected can be classified as an active methane emission site.
4.2 Possible association with putative methanotrophs
The possible association of Nonioninella labradorica with methanotrophs was documented via presence of two putative methanotrophs, based on microbial ultrastructure (Tavormina et al., 2015). The documentation of this possible association with putative methanotrophs likely is due to the feeding experiment. However, there is a small possibility that the associated methanotrophs were field remains. Another benthic foraminifer, Melonis barleeanus, has been noted to have clumps of putative methanotrophs at the apertural opening of field-collected specimens (Bernhard and Panieri, 2018). However, the non-selective deposit-feeding behaviour of N. labradorica, which we describe for this species for the first time, shows that methanotrophs may be ingested via untargeted grazing.
4.3 Degradation vacuoles show a large number of sediment particles and few bacteria
Our results of the feeding experiment show that 23 % of the examined N. labradorica specimens contained bacteria inside their degradation vacuoles. That is not a large proportion compared to the presence of sediment particles, which occurred in 100 % of the examined foraminifers. From this result, however, we infer that N. labradorica at this site is a deposit feeder, feeding on organic detritus and associated bacteria. The bacteria observed in the degradation vacuoles resembled those from other deep-sea foraminifera (Globobulimina pacifica and Uvigerina peregrina) and the shallow-dwelling genus Ammonia (Goldstein and Corliss, 1994). Salt-marsh foraminifera also feed on bacteria and detritus, as observed in TEM studies (Frail-Gauthier et al., 2019). Scavenging on bacteria has also been observed by other foraminifera from intertidal environments such as Ammonia tepida or Haynesina germanica (Pascal et al., 2008) and is a logical consequence of detritus feeding. Certain foraminifera have been shown to selectively ingest algae/bacteria according to strain (Lee et al., 1966; Lee and Muller, 1973). From laboratory cultures we know that several foraminifera cultures require bacteria to reproduce, as antibiotics inhibited reproduction (Muller and Lee, 1969). Future studies will need to employ additional molecular tools to determine the food contents inside the cytoplasm (e.g. Salonen et al., 2019). For example, a recent study used metabarcoding to assess the contribution of eukaryotic operational taxonomic units (OTUs) associated with intertidal foraminifera, revealing that Ammonia sp. T6 preys on metazoans, whereas Elphidium sp. S5 and Haynesina sp. S16 were more likely to ingest diatoms (Chronopoulou et al., 2019).
4.4 General ultrastructure of N. labradorica collected in a seep environment
Our observations also included the intact nature of all major organelle types of this species as this was essential to conclude vitality after the experiment (Nomaki et al., 2016). Mitochondria and kleptoplasts were generally homogeneously distributed throughout the cytoplasm confirming previous observations of six N. labradorica from the Gullmar Fjord (Lekieffre et al., 2018; Jauffrais et al., 2019b). If mitochondria are concentrated predominately under pore plugs, it can be an indicator that the electron acceptor oxygen is scarce in their environment as the pores are the direct connection from the cell to the environment. This has been observed in several other studies in which mitochondria were accumulated under pores in N. stella (Leutenegger and Hansen, 1979) and Bolivina pacifica (Bernhard et al., 2010).
Even though our study did not focus on kleptoplasts, we could observe that kleptoplasts were occasionally degraded, which could have happened (a) during sampling, (b) due to exposure to microscope lights, or (c) due to the age and condition of kleptoplasts inside the host. Kleptoplasts in N. labradorica have been studied in detail describing their diatom origin (Cedhagen, 1991), sensitivity to light, and missing photosynthetic functionality (Jauffrais et al., 2019b).
Based on the content of degradation vacuoles, we conclude that N. labradorica from our study site, an active methane emitting site in the Barents Sea, is a deposit feeder. It ingests large amounts of sediment particles, together with bacteria. For two specimens of the feeding experiment, putative methanotrophs were observed near the N. labradorica aperture, suggesting ingestion of M. sedimenti via “untargeted grazing”. Further studies are needed on feeding strategies of other palaeo-oceanographically relevant foraminifera to detangle the relationship between δ13C of foraminiferal calcite, their cytoplasm, and their dietary composition.
Datasets containing TEM images are downloadable at Zenodo (https://doi.org/10.5281/zenodo.6941739, Schmidt et al., 2022). Molecular sequence data are deposited at GenBank under the accession numbers MN514777 to MN514782.
Samples are available upon request, and TEM thin sections are archived at the University of Angers.
The supplement related to this article is available online at: https://doi.org/10.5194/bg-19-3897-2022-supplement.
GP, EG, and CS designed the project and experiment; CS and EG collected samples; CS performed experiments; CS and HR prepared samples; CS, JMB, EG, and CL conducted TEM observations and interpretations; MS conducted molecular genetics; CS, GP, JMB, and EG wrote the paper; CL, MMS, MS, and HR provided critical review and comments on the manuscript; MMS, MS, and CL contributed reagents, materials, and analysis tools.
The contact author has declared that none of the authors has any competing interests.
Publisher’s note: Copernicus Publications remains neutral with regard to jurisdictional claims in published maps and institutional affiliations.
We thank the captains, crew members, and scientists of R/V Kronprins Haakon and ROV Ægir Team, Anne-Grethe Hestnes for culturing the methanotroph, Florence Manero, Romain Mallet, and Rodolphe Perrot (SCIAM microscopy facility, Univ Angers) for their TEM and SEM expertise, Sunil Vadakkepuliyambatta for assistance with mapping (Fig. 1), and Sophie Quinchard (LPG) for molecular analyses. Joan M. Bernhard was partially supported by US NSF 1634469, WHOI's Investment in Science Program, and the Région Pays de la Loire through the FRESCO Project.
This research has been supported by the French scientific programme MOPGA (Make our Planet Great Again) managed by the National Research Agency; the Norwegian Research Council through the Centre for Arctic Gas Hydrate, Environment and Climate (project number 223259); NORCRUST (project number 255250); and by the Deutsche Forschungsgemeinschaft (DFG, German Research Foundation) – 444059848.
The publication of this article was funded by the Open Access Fund of the Leibniz Association.
This paper was edited by Aninda Mazumdar and reviewed by Jutta Wollenburg and two anonymous referees.
Altschul, S. F., Madden, T. L., Schäffer, A. A., Zhang, J., Zhang, Z., Miller, W., and Lipman, D. J.: Gapped BLAST and PSI-BLAST: a new generation of protein database search programs, Nucl. Acid. Res., 25, 3389–3402, https://doi.org/10.1093/nar/25.17.3389, 1997.
Barnes, R. O. and Goldberg, E. D.: Methane production and consumption in anoxic marine sediments, Geology, 4, 297–300, https://doi.org/10.1130/0091-7613(1976)4<297:MPACIA>2.0.CO;2, 1976.
Bé, A. W. H., Spero, H. J., and Anderson, O. R.: Effects of symbiont elimination and reinfection on the life processes of the planktonic foraminifer Globigerinoides sacculifer, Mar. Biol., 70, 73–86, https://doi.org/10.1007/BF00397298, 1982.
Bernhard, J. M. and Bowser, S. S.: Benthic foraminifera of dysoxic sediments: chloroplast sequestration and functional morphology, Earth-Sci. Rev., 46, 149–165, https://doi.org/10.1016/s0012-8252(99)00017-3, 1999.
Bernhard, J. M. and Panieri, G.: Keystone Arctic paleoceanographic proxy association with putative methanotrophic bacteria, Sci. Rep.-Uk, 8, 10610, https://doi.org/10.1038/s41598-018-28871-3, 2018.
Bernhard, J. M., Goldstein, S. T., and Bowser, S. S.: An ectobiont-bearing foraminiferan, Bolivina pacifica, that inhabits microxic pore waters: cell-biological and paleoceanographic insights, Environ. Microbiol., 12, 2107–2119, https://doi.org/10.1111/j.1462-2920.2009.02073.x, 2010.
Carrier, V., Svenning, M. M., Gründger, F., Niemann, H., Dessandier, P.-A., Panieri, G., and Kalenitchenko, D.: The Impact of Methane on Microbial Communities at Marine Arctic Gas Hydrate Bearing Sediment, Front. Microbiol., 24, 11, https://doi.org/10.3389/fmicb.2020.01932, 2020.
Cedhagen, T.: Retention of chloroplasts and bathymetric distribution in the sublittoral foraminiferan Nonionellina labradorica, Ophelia, 33, 17–30, https://doi.org/10.1080/00785326.1991.10429739, 1991.
Charrieau, L. M., Ljung, K., Schenk, F., Daewel, U., Kritzberg, E., and Filipsson, H. L.: Rapid environmental responses to climate-induced hydrographic changes in the Baltic Sea entrance, Biogeosciences, 16, 3835–3852, https://doi.org/10.5194/bg-16-3835-2019, 2019.
Choquel, C., Geslin, E., Metzger, E., Filipsson, H. L., Risgaard-Petersen, N., Launeau, P., Giraud, M., Jauffrais, T., Jesus, B., and Mouret, A.: Denitrification by benthic foraminifera and their contribution to N-loss from a fjord environment, Biogeosciences, 18, 327–341, https://doi.org/10.5194/bg-18-327-2021, 2021.
Chronopoulou, P.-M., Salonen, I., Bird, C., Reichart, G.-J., and Koho, K. A.: Metabarcoding insights into the trophic behavior and identity of intertidal benthic foraminifera, Front. Microbiol., 10, 1169, https://doi.org/10.3389/fmicb.2019.01169, 2019.
Consolaro, C., Rasmussen, T. L., Panieri, G., Mienert, J., Bünz, S., and Sztybor, K.: Carbon isotope (Δ13C) excursions suggest times of major methane release during the last 14 kyr in Fram Strait, the deep-water gateway to the Arctic, Clim. Past, 11, 669–685, https://doi.org/10.5194/cp-11-669-2015, 2015.
Darling, K. F., Schweizer, M., Knudsen, K. L., Evans, K. M., Bird, C., Roberts, A., Filipsson, H. L., Kim, J.-H., Gudmundsson, G., Wade, C. M., Sayer, M. D. J., and Austin, W. E. N.: The genetic diversity, phylogeography and morphology of Elphidiidae (Foraminifera) in the Northeast Atlantic, Mar. Micropaleontol., 129, 1–23, https://doi.org/10.1016/j.marmicro.2016.09.001, 2016.
Dessandier, P.-A., Borrelli, C., Kalenitchenko, D., and Panieri, G.: Benthic Foraminifera in Arctic Methane Hydrate Bearing Sediments, Front. Mar. Sci., 6, 765, https://doi.org/10.3389/fmars.2019.00765, 2019.
Egger, M., Riedinger, N., Mogollón, J. M., and Jørgensen, B. B.: Global diffusive fluxes of methane in marine sediments, Nat. Geosci., 11, 421–425, https://doi.org/10.1038/s41561-018-0122-8, 2018.
Fossile, E., Nardelli, M. P., Jouini, A., Lansard, B., Pusceddu, A., Moccia, D., Michel, E., Péron, O., Howa, H., and Mojtahid, M.: Benthic foraminifera as tracers of brine production in the Storfjorden “sea ice factory”, Biogeosciences, 17, 1933–1953, https://doi.org/10.5194/bg-17-1933-2020, 2020.
Frail-Gauthier, J. L., Mudie, P. J., Simpson, A. G. B., and Scott, D. B.: Mesocosm and Microcosm Experiments On the Feeding of Temperate Salt Marsh Foraminifera, J. Foraminifer. Res., 49, 259–274, https://doi.org/10.2113/gsjfr.49.3.259, 2019.
Goldstein, S. T. and Corliss, B. H.: Deposit feeding in selected deep-sea and shallow-water benthic foraminifera, Deep Sea Res. Pt. I, 41, 229–241, https://doi.org/10.1016/0967-0637(94)90001-9, 1994.
Gouy, M., Guindon, S., and Gascuel, O.: SeaView version 4: a multiplatform graphical user interface for sequence alignment and phylogenetic tree building, Mol. Biol. Evol., 27, 221–224, https://doi.org/10.1093/molbev/msp259, 2010.
Hald, M. and Korsun, S.: Distribution of modern benthic foraminifera from fjords of Svalbard, European Arctic, J. Foramin. Res., 27, 101–122, https://doi.org/10.2113/gsjfr.27.2.101, 1997.
Heinz, P., Geslin, E., and Hemleben, C.: Laboratory observations of benthic foraminiferal cysts, Mar. Biol. Res., 1, 149–159, 2005.
Hemleben, C., Spindler, M., and Anderson, O. R.: Modern planktonic foraminifera , Springer, Berlin, 363 pp., https://doi.org/10.1007/978-1-4612-3544-6, 1989.
Herguera, J. C., Paull, C. K., Perez, E., Ussler Iii, W., and Peltzer, E.: Limits to the sensitivity of living benthic foraminifera to pore water carbon isotope anomalies in methane vent environments, Paleoceanography, 29, 273–289, https://doi.org/10.1002/2013PA002457, 2014.
Hinrichs, K.-U., Hmelo, L. R., and Sylva, S. P.: Molecular fossil record of elevated methane levels in late Pleistocene coastal waters, Science, 299, 1214–1217, https://doi.org/10.1126/science.1079601, 2003.
Hill, R., Schreiber, U., Gademann, R., Larkum, A. W. D., Kuhl, M., and Ralph, P. J.: Spatial heterogeneity of photosynthesis and the effect of temperature-induced bleaching conditions in three species of corals, Mar. Biol., 144, 633–640, https://doi.org/10.1007/s00227-003-1226-1, 2004a.
Hill, T. M., Kennett, J. P., and Valentine, D. L.: Isotopic evidence for the incorporation of methane-derived carbon into foraminifera from modern methane seeps, Hydrate Ridge, Northeast Pacific, Geochim. Cosmochim. Acta, 68, 4619–4627, https://doi.org/10.1016/j.gca.2004.07.012, 2004b.
Holzmann, M. and Pawlowski, J.: An updated classification of rotaliid foraminifera based on ribosomal DNA phylogeny, Mar. Micropaleontol., 132, 18–34, https://doi.org/10.1016/j.marmicro.2017.04.002, 2017.
Hong, W.-L., Torres, M. E., Carroll, J., Crémière, A., Panieri, G., Yao, H., and Serov, P.: Seepage from an arctic shallow marine gas hydrate reservoir is insensitive to momentary ocean warming, Nat. Commun., 8, 15745, https://doi.org/10.1038/ncomms15745, 2017.
Hong, W. L., Torres, M. E., Portnov, A., Waage, M., Haley, B., and Lepland, A.: Variations in gas and water pulses at an Arctic seep: fluid sources and methane transport, Geophys. Res. Lett., 45, 4153–4162, https://doi.org/10.1029/2018GL077309, 2018.
Iversen, N. and Jørgensen, B. B.: Diffusion coefficients of sulfate and methane in marine sediments: Influence of porosity, Geochim. Cosmochim. Acta, 57, 571–578, https://doi.org/10.1016/0016-7037(93)90368-7, 1993.
Jauffrais, T., LeKieffre, C., Schweizer, M., Geslin, E., Metzger, E., Bernhard, J. M., Jesus, B., Filipsson, H. L., Maire, O., and Meibom, A.: Kleptoplastidic benthic foraminifera from aphotic habitats: insights into assimilation of inorganic C, N and S studied with sub-cellular resolution, Environ. Microbiol., 21, 125–141, https://doi.org/10.1111/1462-2920.14433, 2019b.
Lee, J. J. and Muller, W. A.: Trophic dynamics and niches of salt marsh foraminifera, Am. Zool., 13, 215–223, 1973.
Lee, J. J., McEnery, M., Pierce, S., Freudenthal, H., and Muller, W.: Tracer experiments in feeding littoral foraminifera, J. Protozool., 13, 659–670, 1966.
LeKieffre, C., Bernhard, J. M., Mabilleau, G., Filipsson, H. L., Meibom, A., and Geslin, E.: An overview of cellular ultrastructure in benthic foraminifera: New observations of rotalid species in the context of existing literature, Mar. Micropaleontol., 138, 12–32, https://doi.org/10.1016/j.marmicro.2017.10.005, 2018.
Leutenegger, S. and Hansen, H. J.: Ultrastructural and radiotracer studies of pore function in foraminifera, Mar. Biol., 54, 11–16, https://doi.org/10.1007/BF00387046, 1979.
Lipps, J. H.: Biotic Interactions in Benthic Foraminifera, in: Biotic Interactions in Recent and Fossil Benthic Communities, edited by: Tevesz, M. J. S. and McCall, P. L., Springer US, Boston, MA, 331–376, https://doi.org/10.1007/978-1-4757-0740-3_8, 1983.
Mackensen, A.: On the use of benthic foraminiferal δ13C in palaeoceanography: constraints from primary proxy relationships, Geological Society, London, Special Publications, 303, 121–133, https://doi.org/10.1144/SP303.9, 2008.
McCorkle, D., Keigwin, L., Corliss, B. H., and Emerson, S. R.: The influence of microhabitats on the carbon isotopic composition of deep-sea benthic foraminifera, Paleoceanography, 5, 161–185, https://doi.org/10.1029/PA005i002p00161, 1990.
Mojtahid, M., Zubkov, M. V., Hartmann, M., and Gooday, A. J.: Grazing of intertidal benthic foraminifera on bacteria: Assessment using pulse-chase radiotracing, J. Exp. Mar. Biol. Ecol., 399, 25–34, https://doi.org/10.1016/j.jembe.2011.01.011, 2011.
Muller, W. A. and Lee, J. J.: Apparent Indispensability of Bacteria in Foraminiferan Nutrition, J. Protozool., 16, 471–478, https://doi.org/10.1111/j.1550-7408.1969.tb02303.x, 1969.
Nomaki, H., Heinz, P., Nakatsuka, T., Shimanaga, M., and Kitazato, H.: Species-specific ingestion of organic carbon by deep-sea benthic foraminifera and meiobenthos: In situ tracer experiments, Limnol. Oceanogr., 50, 134–146, https://doi.org/10.4319/lo.2005.50.1.0134, 2005.
Nomaki, H., Heinz, P., Nakatsuka, T., Shimanaga, M., Ohkouchi, N., Ogawa, N. O., Kogure, K., Ikemoto, E., and Kitazato, H.: Different ingestion patterns of C-13-labeled bacteria and algae by deep-sea benthic foraminifera, Mar. Ecol. Prog. Ser., 310, 95–108, https://doi.org/10.3354/meps310095, 2006.
Nomaki, H., Bernhard, J. M., Ishida, A., Tsuchiya, M., Uematsu, K., Tame, A., Kitahashi, T., Takahata, N., Sano, Y., and Toyofuku, T.: Intracellular Isotope Localization in Ammonia sp. (Foraminifera) of Oxygen-Depleted Environments: Results of Nitrate and Sulfate Labeling Experiments, Front. Microbiol., 7, 163, https://doi.org/10.3389/fmicb.2016.00163, 2016.
Panieri, G.: Foraminiferal response to an active methane seep environment: A case study from the Adriatic Sea, Mar. Micropaleontol., 61, 116–130, https://doi.org/10.1016/j.marmicro.2006.05.008, 2006.
Panieri, G., James, R. H., Camerlenghi, A., Westbrook, G. K., Consolaro, C., Cacho, I., Cesari, V., and Cervera, C. S.: Record of methane emissions from the West Svalbard continental margin during the last 23.500yrs revealed by δ13C of benthic foraminifera, Global Planet. Change, 122, 151–160, https://doi.org/10.1016/j.gloplacha.2014.08.014, 2014.
Panieri, G., Lepland, A., Whitehouse, M. J., Wirth, R., Raanes, M. P., James, R. H., Graves, C. A., Crémière, A., and Schneider, A.: Diagenetic Mg-calcite overgrowths on foraminiferal tests in the vicinity of methane seeps, Earth Planet. Sci. Lett., 458, 203–212, https://doi.org/10.1016/j.epsl.2016.10.024, 2017.
Pascal, P.-Y., Dupuy, C., Richard, P., and Niquil, N.: Bacterivory in the common foraminifer Ammonia tepida: Isotope tracer experiment and the controlling factors, J. Exp. Mar. Biol. Ecol., 359, 55–61, https://doi.org/10.1016/j.jembe.2008.02.018, 2008.
Pawlowski, J.: Introduction to the molecular systematics of foraminifera, Micropaleontology, 46, 1–12, 2000.
Rathburn, A. E., Pérez, M. E., Martin, J. B., Day, S. A., Mahn, C., Gieskes, J., Ziebis, W., Williams, D., and Bahls, A.: Relationships between the distribution and stable isotopic composition of living benthic foraminifera and cold methane seep biogeochemistry in Monterey Bay, California, Geochem. Geophy. Geosy., 4, 1220, https://doi.org/10.1029/2003GC000595, 2003.
Reynolds, E. S.: The use of lead citrate at high pH as an electron-opague stain in electron microscopy, J. Cell Biol., 17, 208–212, https://doi.org/10.1083/jcb.17.1.208, 1963.
Risgaard-Petersen, N., Langezaal, A. M., Ingvardsen, S., Schmid, M. C., Jetten, M. S. M., Op den Camp, H. J. M., Derksen, J. W. M., Piña-Ochoa, E., Eriksson, S. P., Peter Nielsen, L., Peter Revsbech, N., Cedhagen, T., and van der Zwaan, G. J.: Evidence for complete denitrification in a benthic foraminifer, Nature, 443, 93, https://doi.org/10.1038/nature05070, 2006.
Salonen, I. S., Chronopoulou, P.-M., Bird, C., Reichart, G.-J., and Koho, K. A.: Enrichment of intracellular sulphur cycle–associated bacteria in intertidal benthic foraminifera revealed by 16S and aprA gene analysis, Sci. Rep.-Uk, 9, 1–12, https://doi.org/10.1038/s41598-019-48166-5, 2019.
Schmidt, C., Geslin, E., Bernhard, J. M., LeKieffre, C., Svenning, M. M., Roberge, H., Schweizer, M., and Panieri, G.: Dataset of publication: Deposit-feeding of Nonionellina labradorica (foraminifera) from an Arctic methane seep site and possible association with a methanotroph, Zenodo [data set], https://doi.org/10.5281/zenodo.6941739, 2022.
Schneider, A., Crémière, A., Panieri, G., Lepland, A., and Knies, J.: Diagenetic alteration of benthic foraminifera from a methane seep site on Vestnesa Ridge (NW Svalbard), Deep Sea Res. Pt. I, 123, 22–34, https://doi.org/10.1016/j.dsr.2017.03.001, 2017.
Serov, P., Vadakkepuliyambatta, S., Mienert, J., Patton, H., Portnov, A., Silyakova, A., Panieri, G., Carroll, M. L., Carroll, J., Andreassen, K., and Hubbard, A.: Postglacial response of Arctic Ocean gas hydrates to climatic amelioration, P. Natl. Acad. Sci., 114, 6215–6220, https://doi.org/10.1073/pnas.1619288114, 2017.
Shetye, S., Mohan, R., Shukla, S. K., Maruthadu, S., and Ravindra, R.: Variability of Nonionellina labradorica Dawson in Surface Sediments from Kongsfjorden, West Spitsbergen, Acta Geologica Sinica – English Edition, 85, 549–558, https://doi.org/10.1111/j.1755-6724.2011.00450.x, 2011.
Tavormina, P. L., Hatzenpichler, R., McGlynn, S., Chadwick, G., Dawson, K. S., Connon, S. A., and Orphan, V. J.: Methyloprofundus sedimenti gen. nov., sp. nov., an obligate methanotroph from ocean sediment belonging to the `deep sea-1'clade of marine methanotrophs, Int. J. Syst. Evol. Microbiol., 65, 251–259, https://doi.org/10.1099/ijs.0.062927-0, 2015.
Torres, M. E., Martin, R. A., Klinkhammer, G. P., and Nesbitt, E. A.: Post depositional alteration of foraminiferal shells in cold seep settings: New insights from flow-through time-resolved analyses of biogenic and inorganic seep carbonates, Earth Planet. Sci. Lett., 299, 10–22, https://doi.org/10.1016/j.epsl.2010.07.048, 2010.
Wefer, G., Heinze, P. M., and Berger, W. H.: Clues to ancient methane release, Nature, 369, 282, https://doi.org/10.1038/369282a0, 1994.
Wollenburg, J. E., Raitzsch, M., and Tiedemann, R.: Novel high-pressure culture experiments on deep-sea benthic foraminifera – Evidence for methane seepage-related δ13C of Cibicides wuellerstorfi, Mar. Micropaleontol., 117, 47–64, 2015.