the Creative Commons Attribution 4.0 License.
the Creative Commons Attribution 4.0 License.
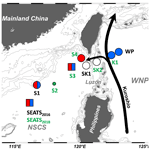
The response of diazotrophs to nutrient amendment in the South China Sea and western North Pacific
Zuozhu Wen
Thomas J. Browning
Rongbo Dai
Wenwei Wu
Weiying Li
Xiaohua Hu
Wenfang Lin
Lifang Wang
Zhimian Cao
Haizheng Hong
Dalin Shi
The availability of iron (Fe) and phosphorus (P) has been shown to be a key factor regulating rates of nitrogen fixation in the western subtropical Pacific. However, the relative importance of Fe and P at finer spatial scales between the northern South China Sea (NSCS) and the western boundary of the North Pacific is poorly constrained. Furthermore, nutrient limitation of specific diazotroph types has not yet been assessed. Here we investigated these unknowns by (i) carrying out measurements of finer-scale spatial variabilities in N2 fixation rates and diazotroph nifH gene abundances throughout these regions and (ii) conducting eight additional Fe and phosphate addition bioassay experiments where both changes in N2 fixation rates and the nifH gene abundances of specific diazotrophs were measured. Overall, nitrogen fixation rates and nifH gene abundances were lower in the NSCS than around the Luzon Strait and the western North Pacific. The nutrient addition bioassay experiments demonstrated that N2 fixation rates in the central NSCS were co-limited by Fe and P, whereas at the western boundary of the North Pacific they were P-limited. Changes in the abundances of nifH in response to nutrient addition varied in how well they correlated with changes in N2 fixation rates, and in six out of eight experiments the largest responses in nifH gene abundances were dominated by either Trichodesmium or UCYN-B (unicellular diazotrophic cyanobacteria group B). In general, nutrient addition had a relatively restricted impact on the composition of the six phylotypes that we surveyed apart from on UCYN-B. This unicellular cyanobacterium group showed increased contribution to the total nifH gene abundance following P addition at sites where N2 fixation rates were P-limited. Our study provides comprehensive evidence of nutrient controls on N2 fixation biogeography in the margin of the western North Pacific. Future research that more accurately constrains nutrient supply rates to this region would be beneficial for resolving what controls diazotroph community structure.
- Article
(3739 KB) - Full-text XML
-
Supplement
(1110 KB) - BibTeX
- EndNote
Nitrogen fixation by diazotrophic bacteria converts abundant dinitrogen (N2) gas into ammonia, providing nearly half of the ocean's bioavailable nitrogen (N) (Gruber and Galloway, 2008), which goes on to support > 30 % of carbon export from surface to deep waters in the N-limited ocean (Böttjer et al., 2016; Wang et al., 2019). A diverse community of diazotrophs has been described across the oligotrophic ocean that includes Trichodesmium, unicellular cyanobacteria (UCYN-A and Crocosphaera, also referred to as UCYN-B), the heterocystous symbiont Richelia associated with diatoms (DDAs, diatom–diazotroph associations) and noncyanobacterial diazotrophs (NCDs, heterotrophic or photoheterotrophic bacteria) (Zehr and Capone, 2020). However, there is still a lack of knowledge on what controls diazotrophic distribution, activity and community structure in the current ocean.
Iron (Fe) and phosphorus (P) are believed to be key factors controlling the biogeographic distribution of marine N2 fixation (Sohm et al., 2011; Zehr and Capone, 2020; Wen et al., 2022). Fe is particularly important for N2 fixers as a cofactor for the FeS-rich nitrogenase enzyme (Berman-Frank et al., 2001), whereas P is also required for genetic information storage, cellular structure and energy generation. A number of nutrient addition bioassay experiments conducted in the field have shown that N2 fixation in the oligotrophic oceans can be limited by Fe or P or co-limited by both nutrients at the same time (Mills et al., 2004; Needoba et al., 2007; Grabowski et al., 2008; Watkins-Brandt et al., 2011; Langlois et al., 2012; Turk-Kubo et al., 2012; Dekaezemacker et al., 2013; Krupke et al., 2015; Tanita et al., 2021; Wen et al., 2022). However, few studies have quantified how the supply of Fe and/or P impacts the abundance of individual diazotrophic phylotypes and their community structure (Langlois et al., 2012; Moisander et al., 2012; Turk-Kubo et al., 2012). Experiments conducted so far that have investigated this were located in the South Pacific and North Atlantic, and they have found diverse responses among diazotrophic phylotypes to the addition of Fe and/or P. Furthermore, the responses of total diazotroph abundances assessed from nifH gene quantifications did not qualitatively match the responses of bulk N2 fixation rates (Langlois et al., 2012; Moisander et al., 2012; Turk-Kubo et al., 2012). Resolution of the specific types of diazotrophs responding to nutrient supply, in addition to overall N2 fixation rates, is potentially crucial for understanding their biogeography, which in turn could be important for their biogeochemical function. For example, the presence of large Trichodesmium filaments is expected to have a different fate in the microbial food web and contribute differently to the sinking flux of carbon than that of small unicellular species (Bonnet et al., 2016).
The northern South China Sea (NSCS) and the neighboring western boundary of the North Pacific are interacting waterbodies, with the major western-boundary Kuroshio Current intruding into the NSCS across the Luzon Strait, generating frontal zones with unique physical and biogeochemical characteristics (Du et al., 2013; Guo et al., 2017; Xu et al., 2018; Huang et al., 2019; Lu et al., 2019; Li et al., 2021). Common to the full regime, however, are surface waters that are warm, stratified and N-depleted but subject to elevated dust input from the Gobi Desert (Duce et al., 1991; Jickells et al., 2005). These conditions potentially provide an ideal habitat for diazotrophs (Chen et al., 2003; Wu et al., 2003). Investigations in these regions have shown high variability in diazotroph abundances and N2 fixation rates (Chen et al., 2003, 2008, 2014; Wu et al., 2018; Lu et al., 2019), which overall increased from the NSCS basin to the western boundary of the North Pacific (Wen et al., 2022). Along this gradient in N2 fixation, the dominant diazotroph types switched from Trichodesmium in the NSCS to UCYN-B at the western boundary of the North Pacific (Wen et al., 2022). Several studies have hypothesized that these gradients of diazotroph abundances and N2 fixation rates were regulated by nutrient availability (specifically, Fe, P and N; Wu et al., 2003; Chen et al., 2003, 2008; Shiozaki et al., 2014a, 2015a). More recent observational and experimental evidence has supported the hypothesis that Fe : N supply ratios are the main drivers of the abundance of diazotrophs and N2 fixation rates across the western North Pacific (Wen et al., 2022). With an increasing supply ratio of Fe : N from the North Equatorial Current (NEC) to the Philippine Sea, Wen et al. (2022) found that diazotroph abundances and N2 fixation rates increased, and bioassay experiments demonstrated evidence for N2 fixation rates switching from Fe to P limitation or to nutrient-replete conditions. In the NSCS, Wen et al. (2022) found N2 fixation rates fell in between NEC and Kuroshio values and bioassay experiments demonstrated rates were co-limited by Fe and P, which they hypothesized was due to intermediate Fe : N supply ratios (Wen et al., 2022).
Although this previous study has outlined the broad spatial pattern of nutrient regulation of marine N2 fixation throughout the western subtropical Pacific (Wen et al., 2022), important questions remain. Two specific examples are that (i) the Kuroshio intrusion generates a frontal zone with a unique diazotrophy regime in the NSCS (Lu et al., 2019), and thus the relatively lower spatial resolution of the experiments in Wen et al. (2022) and other studies (Shiozaki et al., 2014b; Chen et al., 2019) remains insufficient to delineate Fe and P controls at finer spatial scales between the neighboring NSCS and the western boundary of the North Pacific, and (ii) in addition to controls on N2 fixation rates, broad-scale differences in the types of diazotrophs dominating the N2 fixer community were not concretely associated with environmental drivers in experimental tests for nutrient limitation because changes in type-specific diazotroph abundances following nutrient addition were not measured (Shiozaki et al., 2014b; Chen et al., 2019; Wen et al., 2022). Therefore, in the present study we extend the findings of Wen et al. (2022) and others by carrying out additional, higher-spatial-resolution observations of volumetric N2 fixation rates and measurements of the nifH gene abundances of key diazotrophic phylotypes from the NSCS basin to the western boundary of the North Pacific (including the upstream Kuroshio) between 2016 and 2018 (Fig. 1). These new observations were supplemented by eight further, high-volume (10 L) nutrient amendment bioassay experiments throughout the transect to directly test the response of both (i) N2 fixation rates and (ii) nifH gene abundances to the supply of potentially limiting nutrients (Fe, P and Fe + P).
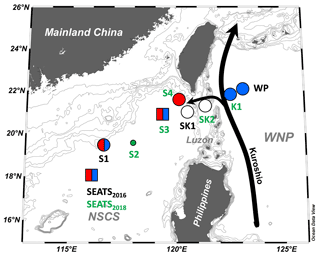
Figure 1Sampling and nutrient amendment experiment locations in the northern South China Sea and the western boundary of the North Pacific. One station (SEATS2016) was sampled in 2016, three (S1, SK1, WP) in 2017, and six (stations with green labels) in 2018. Nutrient amendment experiments were conducted at 8 of 10 stations. Symbols summarize the nutrient limitation of N2 fixation rates found at each site: red, Fe limitation; blue, P limitation; split red–blue, Fe–P co-limitation; white, nutrient replete. The co-limitation type is indicated by the symbol type (square, independent co-limitation; circle, simultaneous co-limitation). WNP, the western North Pacific. Black arrows indicate the Kuroshio Current and its branch.
2.1 Sample collection
Investigations and bioassay experiments were conducted on three cruises to the NSCS (stations SEATS and S1 to S4), the Luzon Strait (stations SK1 and SK2), the upstream Kuroshio (station K1), and the western boundary of the North Pacific (station WP) (Fig. 1) between May 2016 and June 2018 on board the RV Dong Fang Hong 2 and RV Tan Kah Kee. At each station (except station SK2 where no hydrological data are available), temperature and salinity were recorded by a Seabird 911plus CTD. Water samples were collected using Niskin-X bottles at five or six depths (except at SK2, where only surface waters were sampled) throughout the upper 150 m for the determination of N2 fixation and primary production rates. Seawaters from each depth were also sampled for the analysis of nifH gene abundance. Samples for nutrient analysis were also collected. Seawater for the bioassay experiments (at 8 of 10 stations) was collected using a trace-metal-clean towed sampling device located at around 2–5 m depth with suction provided by a Teflon bellows pump. Seawaters were sampled in a dedicated trace-metal-clean laminar flow hood maintained over-pressurized by HEPA-filtered air. During the cruise in 2018 (stations with green labels in Fig. 1), surface waters were sampled under trace-metal-clean conditions for the determination of total particulate Fe concentration.
2.2 N2 fixation and primary production rate measurements
N2 fixation rates were determined by the 15N2 gas dissolution method (Mohr et al., 2010), combined with a primary production assay using NaH13CO3 (99 at. % (where “at.” denotes atom) 13C, Cambridge Isotope Laboratories). Briefly, 0.22 µm filtered surface seawater was degassed using a STERAPORE membrane unit (20M1500A: Mitsubishi Rayon Co., Ltd., Tokyo, Japan) as described in Shiozaki et al. (2015b). After that, 20 mL of 98.9 at. % pure 15N2 gas (Cambridge Isotope Laboratories) was injected into a gastight plastic bag (Tedlar® PVF, Dalian Delin Gas Packing Co., Ltd.) containing 2 L of the degassed seawater and was allowed to fully equilibrate before use. The N2 fixation and primary production incubations were conducted in duplicate 4.3 L Nalgene polycarbonate bottles. Samples were spiked with 100 mL of 15N2-enriched filtered seawater from the same site and incubated on deck for 24 h. The final 15N2-enriched seawater concentration in the incubation bottles was not measured directly during this study. We thus employed a 15N2 at. % of 1.40±0.08 at. % (ranges from 1.28 at. % to 1.56 at. %, n=17) measured in a following cruise in 2020 (Wen et al., 2022), during which the N2 fixation incubations were conducted using the same approach, reagents and equipment as for the study described here. For primary production measurements, NaH13CO3 solution was added to a final amended concentration of 100 µM. After that, the bottles were covered with a neutral-density screen to adjust the light to the levels at sampling depths, and then they were incubated for 24 h in an on-deck incubator continuously flushed with surface seawater. Incubated samples were filtered onto pre-combusted (450 ∘C, 4 h) GF/F filters, and the particulate organic matter from each depth was also collected to determine background particulate organic carbon (POC) and particulate organic nitrogen (PON) concentrations and their natural 13C and 15N abundances.
All filter samples were acid-fumed to remove the inorganic carbon and then analyzed using an elemental analyzer coupled to a mass spectrometer (EA-IRMS, Thermo Scientific Flash 2000 HT–Delta V plus). The N2 fixation and primary production rates were then calculated according to Montoya et al. (1996) and Hama et al. (1983), respectively. The detection limits of N2 fixation rates were then calculated according to Montoya et al. (1996), taking 4 ‰ as the minimum acceptable change in the δ15N of particulate nitrogen. All parameters involved in N2 fixation rate calculation are shown in the Supplement. To represent the inventories, the upper 150 m depth-integrated N2 fixation rate and primary production were calculated by the trapezoidal integration method.
2.3 nifH gene abundance
At each depth, 4.3 L seawater samples for DNA extraction were filtered onto 0.22 µm pore-sized membrane filters (Supor 200, Pall Gelman, NY, USA) and then frozen in liquid N2. To extract the DNA, membranes were cut into pieces under sterile conditions and then extracted using the QIAamp® DNA Mini Kit (Qiagen) following the manufacturer's protocol. The quantitative polymerase chain reaction (qPCR) analysis was targeted on the nifH phylotypes of Trichodesmium spp.; unicellular cyanobacterial UCYN-A1, UCYN-A2 and UCYN-B; Richelia spp. (het-1); and a gamma-proteobacterium (γ-24774A11), using previously designed primers and probe sets (Table S1 in the Supplement; Church et al., 2005a, b; Moisander et al., 2008; Thompson et al., 2014). A recent study suggested that the primers for UCYN-A2 also target UCYN-A3 and thus cannot be used to differentiate between these two phylotypes (Farnelid et al., 2016). Therefore, we used the convention UCYN-A2/A3 when referring to these two groups. The nifH standards were obtained by cloning the environmental sequences from previous samples collected from the South China Sea (SCS). qPCR analysis was carried out as described previously (Church et al., 2005a) with slight modifications. Triplicate qPCR reactions were run for each environmental DNA sample and for each standard on a CFX96 real-time system (Bio-Rad Laboratories). Standards corresponding to between 101 and 107 copies per well were amplified in the same 96-well plate. The amplification efficiencies of PCR were always between 90 %–105 %, with R2 values > 0.99. The quantification limit of the qPCR reactions was 10 nifH gene copies per reaction, and 1 µL from 100 or 150 µL template DNA was applied to the qPCR assay, which was equivalent to approximately ∼ 230–350 gene copies per liter of seawater sample filtered (4.3 L).
A previous study has reported that nifH gene polyploidy exists in Trichodesmium (Sargent et al., 2016), which may impact the estimates of diazotroph compositions. However, given that the degree of polyploidy can vary significantly (ranging from 1 to 1405; Sargent et al., 2016; White et al., 2018), with a potential dependence on the growth conditions, nutrient status, developmental stage and cell cycle (see references in Karlusich et al., 2021), we did not attempt to account or correct for this in calculations of proportions of the different diazotrophs.
2.4 Bioassay experiments
Acid-cleaned Nalgene polycarbonate carboys (10 L) were filled with near-surface seawater from the towed fish system. Trace-metal-clean techniques were strictly applied in the experimental setup and manipulations. All materials including the degas unit and Tedlar® PVF bags that came in contact with the incubation water were acid-washed in a Class-100 cleanroom before use. Nutrient amendments at all sites were Fe, P and Fe + P. Surface dissolved Fe and P concentrations previously reported in the NSCS were 0.17–1.01 and 5–20 nM, respectively (Wu et al., 2003; Zhang et al., 2019). In order to obtain a measurable response within the relatively short 72 h experimental period, 2 nM of Fe and 100 nM of P (chelexed and filter-sterilized) were added to each of the treatment bottles. Control bottles incubated with no nutrient treatment were included in all experiments. Treatments for seven out of eight experiments were conducted with three replicates. However, there were three cases when one of the triplicate samples was lost due to filtration errors (one +Fe + P carboy at station S1, one N2 fixation/primary production (NFR/PP) sample of +Fe + P at station WP, and one NFR/PP sample of +P sample at station S3). In addition, for the bioassay experiment at station SEATS2016, sufficient water was only available to conduct the experiment with two replicates for control and +Fe + P treatments, while +Fe and +P retained three replicates. All carboys were then incubated for 3 d in a screened on-deck incubator (a ∼ 400 L clear on-deck incubator with inflow and outflow) continuously flushed with surface seawater. After 72 h of pre-incubation, subsamples were collected for the determination of Chl a concentration, the N2 fixation rate and nifH gene abundance. The 15N2-enriched seawater was prepared as described above, except that all the materials coming into contact with the seawater were acid-cleaned before use.
2.5 Macronutrient and chlorophyll a analyses
Samples for macronutrient analyses were collected in 125 mL acid-washed high-density polyethylene (HDPE) bottles (Nalgene) and analyzed on board using a four-channel continuous-flow Technicon AutoAnalyzer III (AA3; Bran+Luebbe GmbH). The detection limits for NO + NO and PO were 0.1 and 0.08 µmol L−1, respectively. The nitracline was defined as the depth at which NOx concentration equaled 0.1 µmol L−1 (Le Borgne et al., 2002). Surface seawaters were additionally sampled for the measurement of low-level nutrient concentrations in the 2018 cruise, and NO + NO was determined following Zhang (2000), while PO was analyzed following Ma et al. (2008). Samples for chlorophyll a analysis were collected on nominal 0.7 µm pore-size GF/F filters (Whatman), extracted in 90 % acetone, and analyzed fluorometrically on a Turner Designs fluorometer (Welschmeyer, 1994).
2.6 Particulate Fe concentration
Total particulate Fe (PFetotal) and intracellular Fe (PFeintra) were sampled under a laminar flow hood. Briefly, 4–9 L of surface waters was filtered onto acid-cleaned 0.22 µm polycarbonate membrane filters. For PFeintra samples, in order to remove metal bound to the cell surface, cells were exposed twice to an oxalate–EDTA solution for 5 min and rinsed nine times with Chelex-cleaned 0.56 mol L−1 NaCl solution (Li et al., 2020). PFetotal and PFeintra concentrations were then determined by inductively coupled plasma mass spectrometry (ICP-MS 7700x, Agilent).
2.7 Statistical analysis
The significance of differences among nutrient treatments of bioassay experiments was tested by ANOVA followed by Fisher's protected least significant difference (PLSD) test, using R 4.1.2. Pairwise correlation between N2 fixation rates, diazotroph groups and environmental factors was analyzed using Pearson correlation. A significance level of p<0.05 was applied, except as noted where significance was even greater. It should be noted that those statistical results that were produced from only two replicates are not strictly statistically valid, but for completeness the post hoc test results are nevertheless still included here.
3.1 Spatial variations in N2 fixation rates and diazotroph composition
Our survey revealed substantial spatial variability in N2 fixation rates and nifH gene abundances across the study area (Figs. 2 and 3). Vertically, high N2 fixation rates were found in the upper 50 m (ranging from below the detection limit to 10.4±0.01 nmol N L−1 d−1), rates dropped rapidly at greater depths (Fig. 2), and surface rates were positively correlated with depth-integrated rates (Pearson r=0.68, p=0.043, Table S2). Horizontally, depth-integrated N2 fixation rates were generally low at the central NSCS basin stations (SEATS, S1 and S2, on average 86±33 µmol N m−2 d−1), elevated at stations close to the western edge of the Luzon Strait (S3 and S4, on average 214±47 µmol N m−2 d−1), and were highest at the Luzon Strait station (SK1, 437 µmol N m−2 d−1) and the western North Pacific boundary station (WP, 403 µmol N m−2 d−1) (Figs. 1 and 3 and Table 1).
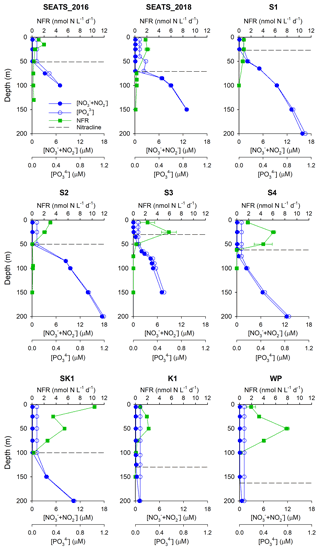
Figure 2Vertical profiles of N2 fixation rates. Green squares, N2 fixation rate (NFR, nmol N L−1 d−1); solid blue circles, NO + NO concentrations (µM); open blue circles, PO concentrations (µM). The dashed line indicates the nitracline depth. Note that no profile data were available at station SK2.
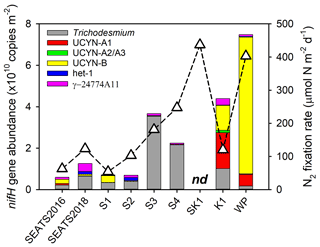
Figure 3Depth-integrated (upper 150 m) nifH gene abundances (bars) and N2 fixation rates (triangles). Note that depth-integrated N2 fixation rates and nifH gene abundances were not available at station SK2. nd, not determined.
Table 1Environmental conditions, N2 fixation and primary production rates: sea surface temperature (SST) and salinity (SSS), chlorophyll a concentration, surface dissolved inorganic nitrogen (SDIN) and surface dissolved inorganic phosphorus (SDIP), nitracline depth (DNitr), surface N2 fixation rate (SNF), upper 150 m depth-integrated N2 fixation rate (INF), and primary production (IPP) at each station. nd, not determined.
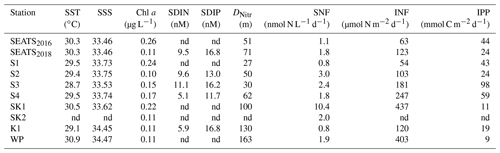
A significant positive correlation was found between the depth-integrated nifH gene abundance and N2 fixation rate (Pearson r=0.72, p=0.046, Table S2), demonstrating that the nifH gene abundances of these major diazotroph phylotypes that we surveyed well explained the major variability in measured rates. However, considerable spatial variation was found in the specific diazotrophs supporting N2 fixation (Fig. 3). Trichodesmium dominated the total nifH gene abundance throughout the water column of the NSCS (52 %–96 % of the total nifH gene abundance, excluding station SEATS2016). In contrast, at the Kuroshio station K1, unicellular diazotrophic cyanobacteria (UCYN-A and UCYN-B) were the most abundant nifH gene phylotypes, and at station WP, UCYN-B alone was dominant (Fig. 3 and Table S3). It should be noted that nifH gene polyploidy exists in Trichodesmium (Sargent et al., 2016), which may have an important impact on the estimates of both in situ and nutrient-treated diazotroph compositions (see Method).
3.2 Diazotroph response to Fe and P supply
To directly test which nutrients were limiting overall N2 fixation rates and the nifH gene abundances of individual diazotrophs, we conducted eight, ∼ 3 d nutrient addition bioassay experiments (Figs. 4 and 5). The responses of the N2 fixation rate to different combinations of Fe and P supply demonstrated a coherent geographic switch across the study area (Figs. 1, 4 and 5). At stations towards the NSCS basin (SEATS2016, S1 and S3), N2 fixation rates were co-limited by Fe and P. Two forms of this co-limitation were identified: (i) only simultaneous Fe and P addition stimulated N2 fixation rates (“simultaneous co-limitation”, station S1, Fig. 4); (ii) independent addition of either Fe or P alone or supply of Fe and P in combination enhanced N2 fixation rates (“independent co-limitation”, station SEATS2016, Fig. 4). For the experiment conducted at station S3, in which N2 fixation was also recognized to be independently co-limited, the rates in all nutrient-amended groups increased by 1.44–1.70 times compared to control values, although statistical significance was not observed in +P and +Fe + P treatments (Fig. 4). Further to the northeast, in contrast, N2 fixation rates were only stimulated by nutrient combinations containing Fe at S4 and by combinations containing P at K1 and WP, suggesting single limitation by Fe and P, respectively, at these sites (Fig. 4). Although Fe addition also appeared to stimulate N2 fixation rates at station K1, P was generally the major limiting nutrient at this station, taking into account the responses of both N2 fixation rates and nifH gene abundance (see below) (Figs. 4 and 5). At stations SK1 and SK2 in the Luzon Strait, mean N2 fixation rates and nifH gene abundances were not significantly affected by nutrient amendments, suggesting that both Fe availability and P availability were not limiting N2 fixation rates (Figs. 4 and 5).
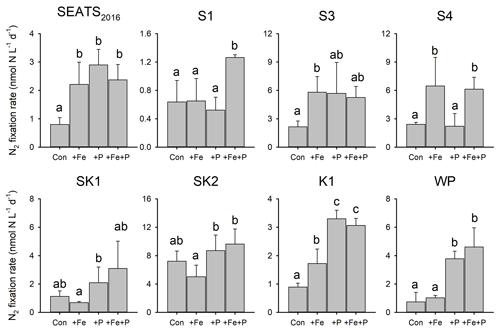
Figure 4Response of N2 fixation to nutrient amendment. Error bars represent the standard deviation of biological replicates (n=2 or 3). Different letters above error bars indicate statistically significant differences (p<0.05) between treatments (ANOVA followed by Fisher's PLSD test). Note that statistical results were produced from only two replicates for control and +Fe + P at station SEATS2016, for +Fe + P at stations S1 and WP, and for +P at station S3 and should thus be treated with caution.
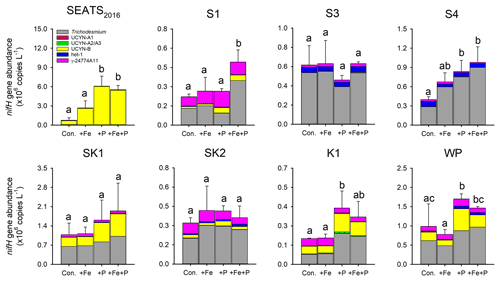
Figure 5Response of diazotroph phylotypes to nutrient amendment. Bar heights represent the mean total nifH concentration and error bars the standard deviation of biological replicates (n=2 or 3). Different letters above error bars indicate a statistically significant difference (p<0.05) between treatments (ANOVA followed by Fisher's PLSD test). Note that statistical results were produced from only two replicates for control and +Fe + P at station SEATS2016, for control and +Fe + P at station S1, and for +P and +Fe + P at station SK2 and should thus be treated with caution.
Further detail as to the drivers of the N2 fixation responses to Fe and P additions was provided by the species-level analysis of diazotroph nifH from the treatment bottles. In general, responses of total nifH gene abundance to Fe and P amendments were qualitatively consistent with N2 fixation rates at most sites; that is, the nutrient(s) limiting N2 fixation rates also limited the diazotroph nifH abundance (Figs. 4 and 5). The exceptions were at stations S3 and S4, where variability in nifH abundances was observed in response to nutrient treatment (station S3) or overall trends differed between nifH abundances and N2 fixation rates (station S4; enhanced nifH abundance in response to +P, whereas rates only responded to +Fe). Quantitatively, the responses of N2 fixation rates and nifH biomass to nutrient addition were not well correlated (total nifH abundance increase rate, calculated as ln(nifH_treatment nifH_control) incubation time, versus the N2 fixation increase rate following nutrient supply; R2=0.07, p=0.21; Fig. S1 in the Supplement), despite initial background nifH abundances and N2 fixation rates being well correlated (Pearson r=0.72, p=0.046, Table S1). This suggested a decoupling of the rates of change in biomass and N2 fixation rates following nutrient addition over the relatively short incubation timescales (∼ 3 d).
Overall, the compositions of the six diazotroph phylotypes that we surveyed were not greatly changed after nutrient amendments (Fig. 5). Trichodesmium and UCYN-B were the two most dominant nifH phylotypes in all experimental waters that contributed to the enhanced total nifH gene abundance after nutrient additions (Figs. 3, 5 and S1). Despite showing independent co-limitation in response to Fe and P supply at station SEATS2016 (Fig. 4), as reflected by equally responding N2 fixation rates, UCYN-B, the dominant nifH phylotype in non-amended control waters, increased 2-fold more following P addition in comparison to Fe addition (Figs. 5 and S2). Furthermore, no significant changes in nifH were observed at station S3, where N2 fixation rates were also independently Fe–P co-limited. More consistent between the N2 fixation rates and nifH biomass changes were the nifH responses at station S1, with overall nifH concentrations only responding to Fe + P additions, matching the N2 fixation response. This was mostly driven by co-limitation of Trichodesmium, whereas UCYN-B responded only to P supply (Figs. 5 and S2).
In contrast to the Fe limitation of N2 fixation rates found at station S4, nifH abundances showed the most significant responses to the combined supply of Fe and P. However, at sites where N2 fixation rates were P-limited (K1 and WP) overall nifH concentrations also responded most to P addition, with contributions from both Trichodesmium and UCYN-B (Fig. 5). In addition, het-1 also increased significantly with +P combinations at stations K1 and WP (Fig. S2). By contrast, γ-24774A11, which also accounted for a substantial fraction of the total nifH gene abundance (up to 31 %), did not show clear enhancement following nutrient additions (Fig. S2), suggesting that it was not Fe- and/or P-limited. Interestingly, UCYN-A disappeared in the nutrient amendment experiments, although they were abundant in the initial water at stations K1 and WP (Figs. 3 and 5), possibly due to an unconstrained bottle effect (Göran and Cooper, 2003).
In the present study, surface dissolved inorganic nitrogen (SDIN) and surface dissolved inorganic phosphorus (SDIP) concentrations in our study area were depleted (5.1–11.1 nM SDIN, 11.7–16.8 nM SDIP, Table 1). Furthermore, bioassay incubations showed no significant responses of Chl a concentration to the amendments of Fe and/or P (Fig. S3), together implying the overall phytoplankton community across the entire area was N-limited and the upper waters were favorable for N2 fixation. However, the rates and nifH gene abundances were much higher in the northeast region of our study area than in the NSCS basin (Fig. 3). Rates at stations SK1 and WP were comparable to those recently reported in this region (∼ 450 µmol N m−2 d−1) measured using the same 15N2 gas dissolution method (Lu et al., 2019; Wen et al., 2022). Although relatively low rates were measured at the Kuroshio Current station (K1) compared with previous observations (e.g., Wen et al., 2022), high nifH gene abundance was nevertheless observed at this site (Fig. 3 and Table S3). Therefore, our observations provide increasing evidence for this western (sub)tropical North Pacific boundary region containing important “hot spots” of N2 fixation (Shiozaki et al., 2010, 2015a; Wen et al., 2022). However, the elevated total nifH concentration at the western boundary of the North Pacific during our study was largely attributed to an increased nifH abundance of unicellular diazotrophs (UCYN-A and UCYN-B, Fig. 3) but not Trichodesmium as previously reported (Chen et al., 2003, 2008, 2014; Shiozaki et al., 2014a). Instead, we found that the Trichodesmium nifH gene was most abundant at stations (S3 and S4) close to the western edge of the Luzon Strait (Fig. 3 and Table S3), where Kuroshio intrusion water has been hypothesized to introduce Trichodesmium into a favorable biogeographic regime (Lu et al., 2019). Either this region is spatially and/or temporally heterogeneous with respect to the presence of unicellular versus Trichodesmium diazotrophs, or the environmental changes have led to a shift in diazotroph community structure (Gruber, 2011; Hutchins and Fu, 2017).
The depth-integrated N2 fixation rate and nifH gene abundance were not correlated with sea surface temperature (SST), but a significant positive correlation was found between nitracline depth and total nifH gene abundance (Pearson r=0.74, p=0.037, Table S2). This was suggestive of subsurface N supply into the euphotic zone, which is inversely related to nitracline depth, potentially being important in regulating diazotroph abundance in our study area, with lower N supply leading to enhanced diazotroph abundances (Chen et al., 2003; Shiozaki et al., 2014b). The presence of diazotrophs in the ocean will be a function of how well they can compete with non-diazotrophic phytoplankton for limiting resources (e.g., Fe and P) under grazing pressure (Ward et al., 2013; Dutkiewicz et al., 2014; Landolfi et al., 2021). Accordingly, because of the growth characteristics of diazotrophs in comparison to non-diazotrophs, in particular their lack of requirement for pre-fixed N but higher requirement for Fe and P, the relative supply rates of N, Fe and P are highly important in dictating where diazotrophs can succeed (Ward et al., 2013). Aligning with earlier global model predictions (Ward et al., 2013) and investigations in the (sub)tropical Atlantic (Schlosser et al., 2014), Wen et al. (2022) recently found that the Fe : N supply ratio (including subsurface and aerosol N and Fe supplies) was a robust predictor of diazotroph standing stock across the broader western North Pacific, including our study region.
By carrying out bioassay incubations, we observed that N2 fixation rates in the NSCS basin were either (i) simultaneously co-limited by Fe and P (identified at station S1), which represents a state where two, non-substitutable nutrients (in this case Fe and P) have been drawn down to equally limiting levels (Sperfeld et al., 2016), or (ii) independently co-limited (stations SEATS2016 and S3), which represents a state where the resources are substitutable at biochemical (Saito et al., 2008) or community levels (Arrigo, 2005). Previous studies reported relatively low surface Fe concentrations in the NSCS basin (0.2–0.3 nM; Wu et al., 2003; Wen et al., 2022), although Fe supply rates to this region are likely elevated via riverine, sedimental and aerosol inputs (Duce et al., 1991; Jickells et al., 2005; Zhang et al., 2019). Wu et al. (2003) hypothesized that the low Fe concentrations were due to a lack of Fe-binding organic ligands, which was subsequently restricting the growth of diazotrophs. We suggest that this may also be attributed to the rapid consumption of Fe (as well as P) by the faster-growing non-diazotrophs under elevated N supply (due to shallower nitraclines, alongside riverine and aerosol inputs). Thus we hypothesize that with the lower Fe : N supply ratio, diazotrophs in this region were outcompeted by non-diazotrophic phytoplankton and co-limited by both Fe and P (Fig. 4). These results add to increasing evidence for the potentially widespread Fe–P co-limitation of N2 fixation under elevated Fe supply (Mills et al., 2004; Snow et al., 2015; Cerdan-Garcia et al., 2022).
The measured contributions of individual diazotrophs to total nifH concentration in response to nutrient supply suggested that simultaneous Fe–P co-limitation of N2 fixation rates at station S1 was via regulation of Trichodesmium, which only responded to Fe + P addition (Fig. 5). The nifH responses also suggested that independent Fe–P co-limitation of N2 fixation rates at sites SEATS2016 and S3 was not operating at the community level (i.e., one diazotroph type limited by Fe and the other by P; Arrigo, 2005) as different qPCR-based diazotroph community structure responses to either Fe or P addition were not observed (Fig. 5). We suggest three possible causes for this observation: (i) co-limitation was at the biochemical rather than community level (i.e., either Fe or P could enhance the rates of processes ultimately driving elevated N2 fixation; Saito et al., 2008). For instance, in addition to serving as a cofactor in nitrogenase, Fe is also a cofactor in alkaline phosphatases (Rodriguez et al., 2014; Yong et al., 2014). Thus, the addition of Fe may allow for enhanced utilization of dissolved organic P (DOP) under depleted DIP (Browning et al., 2017). (ii) Other diazotrophs, which were not analyzed by the qPCR assay, may be responsible for the enhanced N2 fixation rates after nutrient additions. Or (iii) community co-limitation of N2 fixation rates for the measured groups was occurring, but, unlike the simultaneous co-limitation scenario at station S1, experimental durations were too short for this to be reflected in diazotroph biomass changes. Surprisingly, stations with independent co-limitation of N2 fixation rates by Fe and P (SEATS2016 and S3) were not additive (i.e., increases in N2 fixation rates in Fe + P treatments were not larger than Fe and P alone; Sperfeld et al., 2016). Although the available data do not allow us to provide a concrete reason for this, the absence of this additive response may reflect one or a combination of the following: (i) addition of Fe or P leading to the depletion of another secondary limitation nutrient (e.g., Ni); (ii) overall light levels setting an upper limit of N2 fixation rates, which prevented further enhancements after nutrient additions; or (iii) grazer regulation of diazotroph biomass accumulation.
In contrast to the more central NSCS, the deeper nitraclines at the western boundary of the North Pacific appeared more favorable for N2 fixation (Fig. 3 and Table 1). P limitation of N2 fixation at these sites implied that Fe supply (e.g., via aerosols) stimulated diazotroph growth (Fig. 3; Wen et al., 2022) and subsequently the drawdown of P to limiting levels (Table 1, Figs. 4 and 5; Hashihama et al., 2009; Ward et al., 2013; Wen et al., 2022). Additional Fe inputs other than aerosol deposition are also potentially important in supporting the elevated N2 fixation in the Luzon Strait. At station SK2, much higher surface particulate Fe concentrations (both intracellular and total forms) were observed (Table S4), implying supplementary Fe inputs potentially sourced from the adjacent islands and the surrounding shallow subsurface bathymetry (Shiozaki et al., 2014a, 2015a).
In addition to the Fe : N supply ratio regulating the total nifH gene abundance and activity (Wen et al., 2022), we also further hypothesize that overall Fe supply rates might be an important factor in determining the diazotroph community structure in our study area (Church et al., 2008; Langlois et al., 2008; Shiozaki et al., 2017). Specifically, the depth-integrated diazotroph compositions of the six phylotypes switched from being co-dominated by Trichodesmium and other diazotrophs in the central NSCS (SEATS, S1 and S2) and Trichodesmium-dominated in the more northern NSCS (S3 and S4) to finally becoming dominated by UCYN-B at the western boundary of the North Pacific (Fig. 3 and Table S3). Elevated Fe supply in the NSCS, particularly around the islands and shallow bathymetry of the Luzon Strait, might create a more favorable condition for Trichodesmium (Fig. 3 and Table S3), consistent with elevated Fe demands of this genus (Kustka et al., 2003; Kupper et al., 2008; Sohm et al., 2011), as well as its ability to use particulate Fe forms (Rubin et al., 2011), and in line with the elevated contribution of this genus found in other regions with enhanced Fe supply (e.g., the tropical North Atlantic and western South Pacific; Sañudo-Wilhelmy et al., 2001; Sohm et al., 2011; Bonnet et al., 2018; Stenegren et al., 2018). In fact, Fe stimulation of Trichodesmium nifH abundance was observed in the experiment conducted at station S4 (Fig. S2). At station S3, however, this was only observed for N2 fixation rates but not Trichodesmium nifH abundance (Fig. S2). We suggest this could reflect a variable decoupling of N2 fixation rates and diazotroph abundance, depending on other environmental and/or ecological conditions. Conversely, unicellular species may be more competitive than Trichodesmium in regions with lower Fe supply rates (Fig. 3). In addition to having a higher surface-to-volume ratio that favors Fe uptake (Hudson and Morel, 1990; Jacq et al., 2014), UCYN-B species such as Crocosphaera spp. have been reported to employ a repertoire of Fe-conservation strategies, e.g., daily synthesis and breakdown of metalloproteins to recycle Fe between the photosynthetic and N2 fixation metalloenzymes and increased expression of flavodoxin at night even under Fe-replete conditions (Saito et al., 2011). These strategies potentially explain why UCYN-B was less Fe-limited in the NSCS basin (stations SEATS2016 and S1; Figs. 5 and S2) and dominates the diazotroph community on the western Pacific side of the Luzon Strait (Fig. 3; Chen et al., 2019; Wen et al., 2022). Future work with paired measurements of Fe supply rates to surface waters and diazotroph community structure throughout the region would allow for more robust testing of this hypothesis.
Observations and experiments conducted in the NSCS and the western boundary of the North Pacific demonstrated that in the more central NSCS, Fe and P were co-limiting the lower overall observed N2 fixation rates, whereas P was limiting the higher rates on the western Pacific side of the Luzon Strait. This matched the expectation of higher Fe : N supply ratios in the western Pacific generating a more favorable niche for diazotrophs, leading to a drawdown of P. Trichodesmium and UCYN-B were the most dominant nifH phylotypes in the incubation waters, and both dominated the responses of the total nifH gene after nutrient amendments. In general, nutrient addition had a relatively restricted impact on qPCR-based diazotroph community structure apart from on UCYN-B, which showed increased contribution in the diazotroph community following P addition at sites where N2 fixation rates were P-limited. We hypothesize that overall switches in diazotroph community structure from Trichodesmium-dominated in the NSCS to single-celled UCYN-A/UCYN-B were related to declines in overall Fe supply rates and the different physiological strategies of these diazotrophs to obtain and use Fe. Future research that more accurately constrains nutrient supply rates to these different regions would be beneficial for further resolving this hypothesis.
All data needed to evaluate the conclusions in the paper are present in the paper and/or the Supplement. Additional data associated with the paper are available from the corresponding authors upon request.
The supplement related to this article is available online at: https://doi.org/10.5194/bg-19-5237-2022-supplement.
DS, HH and ZW designed the research. ZW, RD, WW, WeiL, XH, WenL and LW performed the experiments. ZW, DS, HH, TJB, XL and ZC analyzed the data. ZW, TJB, HH and DS wrote the manuscript. All authors discussed the results and commented on and edited the manuscript.
The contact author has declared that none of the authors has any competing interests.
Publisher's note: Copernicus Publications remains neutral with regard to jurisdictional claims in published maps and institutional affiliations.
The authors acknowledge the captains and crew of the RV Dong Fang Hong 2 and RV Tan Kah Kee for their help during the cruises.
This work was supported by the National Natural Science Foundation of China (grant nos. 41890802, 42076149, 41925026, 42106041 and 41721005), the “111” Project (grant no. BP0719030) and the Xplorer Prize from the Tencent Foundation to Dalin Shi.
This paper was edited by Koji Suzuki and reviewed by Meri Eichner and one anonymous referee.
Arrigo, K. R.: Marine microorganisms and global nutrient cycles, Nature, 437, 349–355, https://doi.org/10.1038/nature04158, 2005.
Berman-Frank, I., Cullen, J. T., Shaked, Y., Sherrell, R. M., and Falkowski, P.: Iron availability, cellular iron quotas, and nitrogen fixation in Trichodesmium, Limnol. Oceanogr., 46, 1249–1260, https://doi.org/10.4319/lo.2001.46.6.1249, 2001.
Bonnet, S., Baklouti, M., Gimenez, A., Berthelot, H., and Berman-Frank, I.: Biogeochemical and biological impacts of diazotroph blooms in a low-nutrient, low-chlorophyll ecosystem: synthesis from the VAHINE mesocosm experiment (New Caledonia), Biogeosciences, 13, 4461–4479, https://doi.org/10.5194/bg-13-4461-2016, 2016.
Bonnet, S., Caffin, M., Berthelot, H., Grosso, O., Benavides, M., Helias-Nunige, S., Guieu, C., Stenegren, M., and Foster, R. A.: In-depth characterization of diazotroph activity across the western tropical South Pacific hotspot of N2 fixation (OUTPACE cruise), Biogeosciences, 15, 4215–4232, https://doi.org/10.5194/bg-15-4215-2018, 2018.
Böttjer, D., Dore, J. E., Karl, D. M., Letelier, R. M., Mahaffey, C., Wilson, S. T., Zehr, J. P., and Church, M. J.: Temporal variability of nitrogen fixation and particulate nitrogen export at Station ALOHA, Limnol. Oceanogr., 62, 200–216, https://doi.org/10.1002/lno.10386, 2016.
Browning, T. J., Achterberg, E. P., Yong, J. C., Rapp, I., Utermann, C., Engel, A., and Moore, C. M.: Iron limitation of microbial phosphorus acquisition in the tropical North Atlantic, Limnol. Oceanogr. Lett., 8, 15465, https://doi.org/10.1038/ncomms15465, 2017.
Cerdan-Garcia, E., Baylay, A., Polyviou, D., Woodward, E. M. S., Wrightson, L., Mahaffey, C., Lohan, M. C., Moore, C. M., Bibby, T. S., and Robidart, J. C.: Transcriptional responses of Trichodesmium to natural inverse gradients of Fe and P availability, ISME J., 16, 1055–1064, https://doi.org/10.1038/s41396-021-01151-1, 2022.
Chen, M., Lu, Y., Jiao, N., Tian, J., Kao, S. J., and Zhang, Y.: Biogeographic drivers of diazotrophs in the western Pacific Ocean, Limnol. Oceanogr., 64, 1403–1421, https://doi.org/10.1002/lno.11123, 2019.
Chen, Y. L. L., Chen, H. Y., and Lin, Y. H.: Distribution and downward flux of Trichodesmium in the South China Sea as influenced by the transport from the Kuroshio Current, Mar. Ecol.-Prog. Ser., 259, 47–57, https://doi.org/10.3354/meps259047, 2003.
Chen, Y. L. L., Chen, H. Y., Tuo, S. H., and Ohki, K.: Seasonal dynamics of new production from Trichodesmium N2 fixation and nitrate uptake in the upstream Kuroshio and South China Sea basin, Limnol. Oceanogr., 53, 1705–1721, https://doi.org/10.4319/lo.2008.53.5.1705, 2008.
Chen, Y. L. L., Chen, H. Y., Lin, Y. H., Yong, T. C., Taniuchi, Y., and Tuo, S. H.: The relative contributions of unicellular and filamentous diazotrophs to N2 fixation in the South China Sea and the upstream Kuroshio, Deep-Sea Res. Pt. I, 85, 56–71, https://doi.org/10.1016/j.dsr.2013.11.006, 2014.
Church, M. J., Jenkins, B. D., Karl, D. M., and Zehr, J. P.: Vertical distributions of nitrogen fixing phylotypes at Stn ALOHA in the oligotrophic North Pacific Ocean, Aquat. Microb. Ecol., 38, 3–14, https://doi.org/10.3354/ame038003 2005a.
Church, M. J., Short, C. M., Jenkins, B. D., Karl, D. M., and Zehr, J. P.: Temporal patterns of nitrogenase gene (nifH) expression in the oligotrophic North Pacific Ocean, Appl. Environ. Microb., 71, 5362–5370, https://doi.org/10.1128/AEM.71.9.5362-5370.2005, 2005b.
Church, M. J., Björkman, K., and Karl, D.: Regional distributions of nitrogen-fixing bacteria in the Pacific Ocean, Limnol. Oceanogr., 53, 63–77, 2008.
Dekaezemacker, J., Bonnet, S., Grosso, O., Moutin, T., Bressac, M., and Capone, D. G.: Evidence of active dinitrogen fixation in surface waters of the eastern tropical South Pacific during El Niño and La Niña events and evaluation of its potential nutrient controls, Global Biogeochem. Cy., 27, 768–779, https://doi.org/10.1002/gbc.20063, 2013.
Du, C., Liu, Z., Dai, M., Kao, S.-J., Cao, Z., Zhang, Y., Huang, T., Wang, L., and Li, Y.: Impact of the Kuroshio intrusion on the nutrient inventory in the upper northern South China Sea: insights from an isopycnal mixing model, Biogeosciences, 10, 6419–6432, https://doi.org/10.5194/bg-10-6419-2013, 2013.
Duce, R. A., Liss, P. S., Merrill, J. T., Atlas, E. L., Buat-Menard, P., Hicks, B. B., Miller, J. M., Prospero, J. M., Arimoto, R., Church, T. M., Ellis, W., Galloway, J. N., Hansen, L., Jickells, T. D., Knap, A. H., Reinhardt, K. H., Schneider, B., Soudine, A., Tokos, J. J., Tsunogai, S., Wollast, R., and Zhou, M.: The atmospheric input of trace species to the world ocean, Global Biogeochem. Cy., 5, 193–259, https://doi.org/10.1029/91gb01778, 1991.
Dutkiewicz, S., Ward, B. A., Scott, J. R., and Follows, M. J.: Understanding predicted shifts in diazotroph biogeography using resource competition theory, Biogeosciences, 11, 5445–5461, https://doi.org/10.5194/bg-11-5445-2014, 2014.
Farnelid, H., Turk-Kubo, K., Muñoz-Marín, M. C., and Zehr, J. P.: New insights into the ecology of the globally significant uncultured nitrogen-fixing symbiont UCYN-A, Aquat. Microb. Ecol., 77, 125–138, https://doi.org/10.3354/ame01794, 2016.
Göran, E. and Cooper, S. D.: Scale effects and extrapolation in ecological experiments, Adv. Ecol. Res., 33, 161–213, https://doi.org/10.1016/S0065-2504(03)33011-9, 2003.
Grabowski, M. N. W., Church, M. J., and Karl, D. M.: Nitrogen fixation rates and controls at Stn ALOHA, Aquat. Microb. Ecol., 52, 175–183, https://doi.org/10.3354/ame01209, 2008.
Gruber, N.: Warming up, turning sour, losing breath: ocean biogeochemistry under global change, Philos. T. R. Soc. A, 369, 1980–1996, https://doi.org/10.1098/rsta.2011.0003, 2011.
Gruber, N. and Galloway, J. N.: An Earth-system perspective of the global nitrogen cycle, Nature, 451, 293–296, https://doi.org/10.1038/nature06592, 2008.
Guo, L., Xiu, P., Chai, F., Xue, H. J., Wang, D. X., and Sun, J.: Enhanced chlorophyll concentrations induced by Kuroshio intrusion fronts in the northern South China Sea, Geophys. Res. Lett., 44, 11565–11572, https://doi.org/10.1002/2017GL075336, 2017.
Hama, T., Miyazaki, T., Ogawa, Y., Iwakuma, T., Takahashi, M., Otsuki, A., and Ichimura, S.: Measurement of photosynthetic production of a marine phytoplankton population using a stable 13C isotope, Mar. Biol., 73, 31–36, https://doi.org/10.1007/BF00396282, 1983.
Hashihama, F., Furuya, K., Kitajima, S., Takeda, S., Takemura, T., and Kanda, J.: Macro-scale exhaustion of surface phosphate by dinitrogen fixation in the western North Pacific, Geophys. Res. Lett., 36, L03610, https://doi.org/10.1029/2008gl036866, 2009.
Huang, Y., Laws, E. A., Chen, B., and Huang, B.: Stimulation of heterotrophic and autotrophic metabolism in the mixing zone of the Kuroshio Current and northern South China Sea: Implications for export production, J. Geophys. Res.-Biogeo., 124, 2645–2661, https://doi.org/10.1029/2018jg004833, 2019.
Hudson, R. J. M. and Morel, F. M. M.: Iron transport in marine-phytoplankton – kinetics of cellular and medium coordination reactions, Limnol. Oceanogr., 35, 1002–1020, https://doi.org/10.4319/lo.1990.35.5.1002, 1990.
Hutchins, D. A. and Fu, F.: Microorganisms and ocean global change, Nat. Microbiol., 2, 17058, https://doi.org/10.1038/nmicrobiol.2017.58, 2017.
Jacq, V., Ridame, C., L'Helguen, S., Kaczmar, F., and Saliot, A.: Response of the unicellular diazotrophic cyanobacterium Crocosphaera watsonii to iron limitation, PLoS One, 9, e86749, https://doi.org/10.1371/journal.pone.0086749, 2014.
Jickells, T. D., An, Z. S., Andersen, K. K., Baker, A. R., Bergametti, G., Brooks, N., Cao, J. J., Boyd, P. W., Duce, R. A., Hunter, K. A., Kawahata, H., Kubilay, N., laRoche, J., Liss, P. S., Mahowald, N., Prospero, J. M., Ridgwell, A. J., Tegen, I., and Torres, R.: Global iron connections between desert dust, ocean biogeochemistry, and climate, Science, 308, 67–71, https://doi.org/10.1126/science.1105959, 2005.
Karlusich, J. J. P., Pelletier, E., Lombard, F., Carsique, M., Dvorak, E., Colin, S., Picheral, M., Cornejo-Castillo, F. M., Acinas, S. G., Pepperkok, R., Karsenti, E., de Vargas, C., Wincker, P., Bowler, C., Foster, R. A.: Global distribution patterns of marine nitrogen-fixers by imaging and molecular methods, Nat. Commun., 12, 4160, https://doi.org/10.1038/s41467-021-24299-y, 2021.
Krupke, A., Mohr, W., LaRoche, J., Fuchs, B. M., Amann, R. I., and Kuypers, M. M.: The effect of nutrients on carbon and nitrogen fixation by the UCYN-A-haptophyte symbiosis, ISME J., 9, 1635–1647, https://doi.org/10.1038/ismej.2014.253, 2015.
Kustka, A., Sañudo-Wilhelmy, S., Carpenter, E. J., Capone, D. G., and Raven, J. A.: A revised estimate of the iron use efficiency of nitrogen fixation, with special reference to the marine cyanobacterium Trichodesmium spp. (cyanophyta), J. Phycol., 39, 12–25, https://doi.org/10.1046/j.1529-8817.2003.01156.x, 2003.
Kupper, H., Setlik, I., Seibert, S., Prasil, O., Setlikova, E., Strittmatter, M., Levitan, O., Lohscheider, J., Adamska, I., and Berman-Frank, I.: Iron limitation in the marine cyanobacterium Trichodesmium reveals new insights into regulation of photosynthesis and nitrogen fixation, New Phytol., 179, 784–798, https://doi.org/10.1111/j.1469-8137.2008.02497.x, 2008.
Landolfi, A., Prowe, A. E. F., Pahlow, M., Somes, C. J., Chien, C. T., Schartau, M., Koeve, W., and Oschlies, A.: Can top-down controls expand the ecological niche of marine N2 fixers?, Front. Microbiol., 12, 690200, https://doi.org/10.3389/fmicb.2021.690200, 2021.
Langlois, R. J., Hummer, D., and LaRoche, J.: Abundances and distributions of the dominant nifH phylotypes in the Northern Atlantic Ocean, Appl. Environ. Microb., 74, 1922–1931, https://doi.org/10.1128/AEM.01720-07, 2008.
Langlois, R. J., Mills, M. M., Ridame, C., Croot, P., and LaRoche, J.: Diazotrophic bacteria respond to Saharan dust additions, Mar. Ecol.-Prog. Ser., 470, 1–14, https://doi.org/10.3354/meps10109, 2012.
Le Borgne, R., Barber, R. T., Delcroix, T., Inoue, H. Y., Mackey, D. J., and Rodier, M.: Pacific warm pool and divergence: Temporal and zonal variations on the equator and their effects on the biological pump, Deep-Sea Res. Pt. II, 49, 2471–2512, https://doi.org/10.1016/S0967-0645(02)00045-0, 2002.
Li, W., Sunda, W. G., Lin, W., Hong, H., and Shi, D.: The effect of cell size on cellular Zn and Cd and Zn-Cd-CO2 colimitation of growth rate in marine diatoms, Limnol. Oceanogr., 65, 2896–2911, https://doi.org/10.1002/lno.11561, 2020.
Li, X., Wu, K., Gu, S., Jiang, P., Li, H., Liu, Z., and Dai, M.: Enhanced biodegradation of dissolved organic carbon in the western boundary Kuroshio Current when intruded to the marginal South China Sea, J. Geophys. Res.-Oceans, 126, e2021JC017585, https://doi.org/10.1029/2021jc017585, 2021.
Lu, Y., Wen, Z., Shi, D., Lin, W., Bonnet, S., Dai, M., and Kao, S. J.: Biogeography of N2 fixation influenced by the western boundary current intrusion in the South China Sea, J. Geophys. Res.-Oceans, 124, 6983–6996, https://doi.org/10.1029/2018jc014781, 2019.
Ma, J., Yuan, D. X., Liang, Y., and Dai, M. H.: A modified analytical method for the shipboard determination of nanomolar concentrations of orthophosphate in seawater, J. Oceanogr., 64, 443–449, 2008.
Mills, M., Ridame, C., Davey, M., Roche, J. L., and Geider, R. J.: Iron and phosphorus co-limit nitrogen fixation in the eastern tropical North Atlantic, Nature, 429, 292–294, https://doi.org/10.1038/nature02550, 2004.
Mohr, W., Großkopf, T., Wallace, D. W., and LaRoche, J.: Methodological underestimation of oceanic nitrogen fixation rates, PLoS One, 5, e12583, https://doi.org/10.1371/journal.pone.0012583.g001, 2010.
Moisander, P. H., Beinart, R. A., Voss, M., and Zehr, J. P.: Diversity and abundance of diazotrophic microorganisms in the South China Sea during intermonsoon, ISME J., 2, 954–967, https://doi.org/10.1038/ismej.2008.51, 2008.
Moisander, P. H., Zhang, R., Boyle, E. A., Hewson, I., Montoya, J. P., and Zehr, J. P.: Analogous nutrient limitations in unicellular diazotrophs and Prochlorococcus in the South Pacific Ocean, ISME J., 6, 733–744, https://doi.org/10.1038/ismej.2011.152, 2012.
Montoya, J. P., Voss, M., Kähler, P., and Capone, D. G.: A simple, high-precision, high-sensitivity tracer assay for N2 fixation, Appl. Environ. Microb., 62, 986–993, https://doi.org/10.1128/AEM.62.3.986-993.1996, 1996.
Needoba, J. A., Foster, R. A., Sakamoto, C., Zehr, J. P., and Johnson, K. S.: Nitrogen fixation by unicellular diazotrophic cyanobacteria in the temperate oligotrophic North Pacific Ocean, Limnol. Oceanogr., 54, 1317–1327, https://doi.org/10.4319/lo.2007.52.4.1317, 2007.
Rodriguez, F., Lillington, J., Johnson, S., Timmel, C. R., Lea, S. M., and Berks, B. C.: Crystal structure of the bacillus subtilis phosphodiesterase PhoD reveals an iron and calcium-containing active site, J. Biol. Chem., 289, 30889–30899, https://doi.org/10.1074/jbc.M114.604892, 2014.
Rubin, M., Berman-Frank, I., and Shaked, Y.: Dust- and mineral-iron utilization by the marine dinitrogen-fixer Trichodesmium, Nat. Geosci., 4, 529–534, https://doi.org/10.1038/ngeo1181, 2011.
Saito, M. A., Goepfert, T. J., and Ritt, J. T.: Some thoughts on the concept of colimitation: Three definitions and the importance of bioavailability, Limnol. Oceanogr., 53, 276–290, https://doi.org/10.4319/lo.2008.53.1.0276, 2008.
Saito, M. A., Bertrand, E. M., Dutkiewicz, S., Bulygin, V. V., Moran, D. M., Monteiro, F. M., Follows, M. J., Valois, F. W., and Waterbury, J. B.: Iron conservation by reduction of metalloenzyme inventories in the marine diazotroph Crocosphaera watsonii, P. Natl. Acad. Sci. USA, 108, 2184–2189, https://doi.org/10.1073/pnas.1006943108, 2011.
Sañudo-Wilhelmy, S. A., Kustka, A. B., Gobler, C. J., Hutchins, D. A., Yang, M., Lwiza, K., Burns, J. A., Capone, D. G., Ravenk, J. A., and Carpenter, E. J.: Phosphorus limitation of nitrogen fixation by Trichodesmiun in the central Atlantic Ocean, Nature, 411, 66–69, https://doi.org/10.1038/35075041, 2001.
Sargent, E. C., Hitchcock, A., Johansson, S. A., Langlois, R., Moore, C. M., LaRoche, J., Poulton, A. J., and Bibby, T. S.: Evidence for polyploidy in the globally important diazotroph Trichodesmium, FEMS Microbiol. Lett., 363, https://doi.org/10.1093/femsle/fnw244, 2016.
Schlosser, C., Klar, J. K., Wake, B. D., Snow, J. T., Honey, D. J., Woodward, E. M. S., Lohan, M. C., Achterberg, E. P., and Moore, C. M.: Seasonal ITCZ migration dynamically controls the location of the (sub)tropical Atlantic biogeochemical divide, P. Natl. Acad. Sci. USA, 111, 1438–1442, https://doi.org/10.1073/pnas.1318670111, 2014.
Shiozaki, T., Furuya, K., Kodama, T., Kitajima, S., Takeda, S., Takemura, T., and Kanda, J.: New estimation of N2 fixation in the western and central Pacific Ocean and its marginal seas, Global Biogeochem. Cy., 24, GB1015, https://doi.org/10.1029/2009gb003620, 2010.
Shiozaki, T., Kodama, T., and Furuya, K.: Large-scale impact of the island mass effect through nitrogen fixation in the western South Pacific Ocean, Geophys. Res. Lett., 41, 2907–2913, https://doi.org/10.1002/2014GL059835, 2014a.
Shiozaki, T., Chen, Y. L. L., Lin, Y. H., Taniuchi, Y., Sheu, D. S., Furuya, K., and Chen, H. Y.: Seasonal variations of unicellular diazotroph groups A and B, and Trichodesmium in the northern South China Sea and neighboring upstream Kuroshio Current, Cont. Shelf Res., 80, 20–31, https://doi.org/10.1016/j.csr.2014.02.015, 2014b.
Shiozaki, T., Nagata, T., Ijichi, M., and Furuya, K.: Nitrogen fixation and the diazotroph community in the temperate coastal region of the northwestern North Pacific, Biogeosciences, 12, 4751–4764, https://doi.org/10.5194/bg-12-4751-2015, 2015a.
Shiozaki, T., Takeda, S., Itoh, S., Kodama, T., Liu, X., Hashihama, F., and Furuya, K.: Why is Trichodesmium abundant in the Kuroshio?, Biogeosciences, 12, 6931–6943, https://doi.org/10.5194/bg-12-6931-2015, 2015b.
Shiozaki, T., Bombar, D., Riemann, L., Hashihama, F., Takeda, S., Yamaguchi, T., Ehama, M., Hamasaki, K., and Furuya, K.: Basin scale variability of active diazotrophs and nitrogen fixation in the North Pacific, from the tropics to the subarctic Bering Sea, Global Biogeochem. Cy., 31, 996–1009, https://doi.org/10.1002/2017gb005681, 2017.
Snow, J. T., Schlosser, C., Woodward, E. M., Mills, M., Achterberg, E. P., Mahaffey, C., Bibby, T. S., and Moore, C. M.: Environmental controls on the biogeography of diazotrophy and Trichodesmium in the Atlantic Ocean, Global Biogeochem. Cy., 29, 865–884, https://doi.org/10.1002/2015GB005090, 2015.
Sohm, J. A., Webb, E. A., and Capone, D. G.: Emerging patterns of marine nitrogen fixation, Nature reviews, Microbiology, 9, 499–508, https://doi.org/10.1038/nrmicro2594, 2011.
Sperfeld, E., Raubenheimer, D., and Wacker, A.: Bridging factorial and gradient concepts of resource co-limitation: Towards a general framework applied to consumers, Ecol. Lett., 19, 201–215, https://doi.org/10.1111/ele.12554, 2016.
Stenegren, M., Caputo, A., Berg, C., Bonnet, S., and Foster, R. A.: Distribution and drivers of symbiotic and free-living diazotrophic cyanobacteria in the western tropical South Pacific, Biogeosciences, 15, 1559–1578, https://doi.org/10.5194/bg-15-1559-2018, 2018.
Tanita, I., Shiozaki, T., Kodama, T., Hashihama, F., Sato, M., Takahashi, K., and Furuya, K.: Regionally variable responses of nitrogen fixation to iron and phosphorus enrichment in the Pacific Ocean, J. Geophys. Res.-Biogeo., 126, e2021JG006542, https://doi.org/10.1029/2021jg006542, 2021.
Thompson, A. W., Carter, B. J., Turk-Kubo, K. A., Malfatti, F., Azam, F., and Zehr, J. P.: Genetic diversity of the unicellular nitrogen-fixing cyanobacteria UCYN-A and its prymnesiophyte host, Environ. Microbiol., 16, 3238–3249, https://doi.org/10.1111/1462-2920.12490, 2014.
Turk-Kubo, K. A., Achilles, K. M., Serros, T. R., Ochiai, M., Montoya, J. P., and Zehr, J. P.: Nitrogenase (nifH) gene expression in diazotrophic cyanobacteria in the Tropical North Atlantic in response to nutrient amendments, Front Microbiol., 3, 386, https://doi.org/10.3389/fmicb.2012.00386, 2012.
Wang, W. L., Moore, J. K., Martiny, A. C., and Primeau, F. W.: Convergent estimates of marine nitrogen fixation, Nature, 566, 205–211, https://doi.org/10.1038/s41586-019-0911-2, 2019.
Ward, B. A., Dutkiewicz, S., Moore, C. M., and Follows, M. J.: Iron, phosphorus, and nitrogen supply ratios define the biogeography of nitrogen fixation, Limnol. Oceanogr., 58, 2059–2075, https://doi.org/10.4319/lo.2013.58.6.2059, 2013.
Watkins-Brandt, K. S., Letelier, R. M., Spitz, Y. H., Church, M. J., Böttjer, D., and White, A. E.: Addition of inorganic or organic phosphorus enhances nitrogen and carbon fixation in the oligotrophic North Pacific, Mar. Ecol.-Prog. Ser., 432, 17–29, https://doi.org/10.3354/meps09147, 2011.
Welschmeyer, N. A.: Fluorometric analysis of chlorophyll-a in the presence of chlorophyll-B and pheopigments, Limnol. Oceanogr., 39, 1985–1992, 1994.
Wen, Z., Browning, T. J., Cai, Y., Dai, R., Zhang, R., Du, C., Jiang, R., Lin, W., Liu, X., Cao, Z., Hong, H., Dai, M., and Shi, D.: Nutrient regulation of biological nitrogen fixation across the tropical western North Pacific, Sci. Adv., 8, eabl7564, https://doi.org/10.1126/sciadv.abl7564, 2022.
White, A. E., Watkins-Brandt, K. S., and Church, M. J.: Temporal variability of Trichodesmium spp. and diatom-diazotroph assemblages in the North Pacific Subtropical Gyre, Front. Mar. Sci., 5, 27, https://doi.org/10.3389/fmars.2018.00027, 2018.
Wu, C., Fu, F. X., Sun, J., Thangaraj, S., and Pujari, L.: Nitrogen fixation by Trichodesmium and unicellular diazotrophs in the northern South China Sea and the Kuroshio in summer, Sci. Rep.-UK, 8, 2415, https://doi.org/10.1038/s41598-018-20743-0, 2018.
Wu, J., Chung, S. W., Wen, L. S., Liu, K. K., Chen, Y. L. L., Chen, H. Y., and Karl, D. M.: Dissolved inorganic phosphorus, dissolved iron, and Trichodesmium in the oligotrophic South China Sea, Global Biogeochem. Cy., 17, 1008, https://doi.org/10.1029/2002gb001924, 2003.
Xu, M. N., Zhang, W., Zhu, Y., Liu, L., Zheng, Z., Wan, X. H. S., Qian, W., Dai, M., Gan, J., Hutchins, D. A., and Kao, S. J.: Enhanced ammonia oxidation caused by lateral Kuroshio intrusion in the boundary zone of the northern South China Sea, Geophys. Res. Lett., 45, 6585–6593, https://doi.org/10.1029/2018gl077896, 2018.
Yong, S. C., Roversi, P., Lillington, J., Rodriguez, F., Krehenbrink, M., Zeldin, O. B., Garman, E. F., Lea, S. M., and Berks, B. C.: A complex iron-calcium cofactor catalyzing phosphotransfer chemistry, Science, 345, 1170–1173, https://doi.org/10.1126/science.1254237, 2014.
Zehr, J. P. and Capone, D. G.: Changing perspectives in marine nitrogen fixation, Science, 368, eaay9514, https://doi.org/10.1126/science.aay9514, 2020.
Zhang, J. Z.: Shipboard automated determination of trace concentrations of nitrite and nitrate in oligotrophic water by gas-segmented continuous flow analysis with a liquid waveguide capillary flow cell, Deep-Sea Res. Pt. I, 47, 1157–1171, https://doi.org/10.1016/S0967-0637(99)00085-0, 2000.
Zhang, R., Zhu, X., Yang, C., Ye, L., Zhang, G., Ren, J. L., Wu, Y., Liu, S. M., Zhang, J., and Zhou, M.: Distribution of dissolved iron in the Pearl River (Zhujiang) Estuary and the northern continental slope of the South China Sea, Deep-Sea Res. Pt. II, 167, 14–24, https://doi.org/10.1016/j.dsr2.2018.12.006, 2019.