the Creative Commons Attribution 4.0 License.
the Creative Commons Attribution 4.0 License.
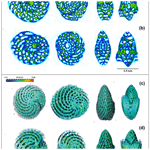
Acidification impacts and acclimation potential of Caribbean benthic foraminifera assemblages in naturally discharging low-pH water
Adina Paytan
Olga Maria Oliveira de Araújo
Ricardo Tadeu Lopes
Cátia Fernandes Barbosa
Ocean acidification (OA) is expected to negatively affect many ecologically important organisms. Here we report the response of Caribbean benthic foraminiferal assemblages to naturally discharging low-pH waters with a composition similar to that expected for the end of the 21st century. At low pH ∼ 7.8 and low saturation state with respect to calcite (Ωcalcite < 4), the relative abundance of hyaline, agglutinated, and symbiont-bearing species increased, indicating higher resistance to potential carbonate chemistry changes. Diversity and other taxonomical metrics (i.e., richness, abundance, and evenness) declined steeply with decreasing pH despite exposure of this ecosystem to low-pH conditions for millennia, suggesting that tropical foraminiferal communities will be negatively impacted under acidification scenarios SSP3-7.0 (Shared Socioeconomic Pathways) and SSP5-8.5. The species Archaias angulatus, a major contributor to sediment production in the Caribbean, was able to calcify at more extreme conditions (7.1 pH) than those projected for the late 21st century, but the calcified tests had a lower average density than those exposed to higher-pH conditions (7.96), indicating that reef foraminiferal carbonate production might decrease this century. Smaller foraminifera were particularly sensitive to low pH, and our results demonstrate their potential use to monitor OA conditions.
- Article
(8340 KB) - Full-text XML
-
Supplement
(342 KB) - BibTeX
- EndNote
With anthropogenic carbon dioxide (CO2) emissions having been steadily increasing since the beginning of the industrial age, levels are currently higher than they have been in the past 800 000 years (Petit et al., 1999; Lüthi et al., 2008). Global emissions are increasing annually and leading to a continued uptake of CO2 by the oceans. Consequently, surface ocean pH and saturation state decrease (−0.0181 ± 0.0001 per decade; Lida et al., 2021), a process commonly known as ocean acidification (OA) (Doney et al., 2020). Based on the Coupled Model Intercomparison Project Phase Six (CMIP6), a further decrease in surface ocean pH is expected for all Shared Socioeconomic Pathways (SSPs) at the end of the 21st century (Kwiatkowski et al., 2020; IPCC, 2021). Because the carbonate system has major control on biogenic calcification efficiency this process is expected to negatively affect many ecologically important calcifying organisms such as corals (Kroeker et al., 2013; Crook et al., 2013; Hughes et al., 2017), foraminifers (Uthicke et al., 2013; Kawahata et al., 2019), and coralline crustose algae (Penã et al., 2021).
Among these, foraminifera are dominant members of both planktonic and benthic communities with widespread distribution in the ocean. They are vital to calcium carbonate (CaCO3) cycling (Langer et al., 1997; Langer, 2008), and on a global scale, they are estimated to contribute a total of 14 × 109 t of CaCO3 per year, accounting for approximately 25 % of the current total CaCO3 production (Langer, 2008). Due to their ability to consume substantial amounts of organic matter, they are also relevant for organic carbon cycling (Moodley et al., 2000), and they constitute a key link in marine food webs. Once they die, their shells (also known as tests) become important contributors to sediment accumulation in many ecosystems (Yamano et al., 2000; Doo et al., 2016) and are hence relevant for the carbon burial flux in the ocean (Schiebel, 2002). With ongoing OA and future scenarios projecting further changes (Kwiatkowski et al., 2020; IPCC, 2021), it is vital for assessing biological feedbacks and changes in biochemical cycles to understand how foraminifera will be affected. Many studies under controlled conditions have documented the negative impact of lower pH on calcification, weight, size, and taxonomical metrics (Nehrke et al., 2013; Kawahata et al., 2019; Narayan et al., 2021, and references therein). However, some studies have also demonstrated either resilience (Engel et al., 2015; Pettit et al., 2015; Stuhr et al., 2021) or even positive effects on foraminifera, such as enhanced calcification (Fujita et al., 2011) or enzymatic calcification activity (Prazeres et al., 2015), demonstrating the complexity of foraminiferal responses to OA. Additionally, relatively little is known about how foraminifera respond in natural settings under low-pH, low-carbonate saturation conditions, which is crucial for determining if and how communities have the potential to acclimate.
In situ investigations have been performed in natural CO2 vents in the Mediterranean Sea (Dias et al., 2010; Pettit et al., 2015), Papua New Guinea (Uthicke et al., 2013), the northern Gulf of California (Pettit et al., 2013), and coastal springs in Puerto Morelos (PM), Mexico (Martinez et al., 2018). In the latter, recruitment and early succession (Crook et al., 2016), acclimatization potential (Crook et al., 2013), and the responses of calcifying communities were studied (Crook et al., 2012; Martinez et al., 2018), demonstrating that despite general deleterious effects, some organisms were able to calcify under OA conditions. A study focusing on large benthic foraminifera (LBF) reported that porcelaneous, chlorophyte-bearing foraminifera (e.g., Archaias angulatus) were relatively less impacted (Martinez et al., 2018). Study sites such as coastal springs allow the investigation of foraminiferal communities under projected future conditions more realistically, helping to decrease the uncertainty in global-scale models. However, a detailed survey considering community-wide responses (i.e., including smaller foraminifera) is necessary to ascertain a wider range of potential impacts.
As CO2 emissions continue to grow despite emerging climate policies (Peters et al., 2020), global awareness has demonstrated a strong interest in research focused on potential impacts for mitigative action. To build on and expand the findings at PM we aimed to (i) explore the mid-term (i.e., multidecadal) responses of foraminifera species to OA using total assemblages, (ii) investigate the effects of OA on assemblages of both large and small foraminifera for acidification scenarios projected to the end of the 21st century (Kwiatkowski et al., 2020; IPCC, 2021), (iii) explore the taphonomical and ecological implications of post mortem alterations for reef ecosystems, and (iv) investigate possible acclimation patterns in the shell structure of the species A. angulatus. Specifically, an examination of assemblage structure, taxonomic metrics, assemblage test size, preservation potential, and an X-ray micro computed tomography (micro-CT) analysis in the species A. angulatus were employed.
2.1 Study site and data retrieval
The Yucatán Peninsula is a karstic region in southern Mexico (Fig. 1a) where Tertiary limestones are underlain by an ejecta/evaporite complex. Several structural and tectonic features divide the area into six distinct physiographic regions (Back and Hanshaw, 1970). Among these, Puerto Morelos reef lagoon is part of the Holbox Fracture Zone–Xel-Ha region, which is characterized by a >100 km long chain of elongated depressions referred to as “sabanas” (Perry et al., 2002). In this area, rainwater infiltrates the porous karstic limestone (Fig. 1b) and flows towards the ocean through interconnected caves and fractures where the groundwater mixes with seawater in the underground aquifers before discharging between the shore and the offshore barrier reef (Beddows et al., 2007; Null et al., 2014). Flowing through the limestone and interacting with the strata through processes of dissolution, precipitation, and mixing, the groundwater conditions change, and the resulting water with low pH, low carbonate saturation state (Ω), and high inorganic C content discharges along the Mexican Caribbean coast (Back and Hanshaw, 1970; Perry et al., 2002; Crook et al., 2012, 2013, 2016; Martinez et al., 2018, 2019; Hernandez-Terrones et al., 2021). The submarine groundwater discharges at submarine springs, whose structure ranges from long “fractures” to small circular depression “seeps” (Fig. 1d; Agua spring) (Crook et al., 2012). The discharge of the springs is relatively constant throughout the year (Crook et al., 2016), and the lagoon circulation is not significantly affected by tides (avg. 17 cm) or currents due to the microtidal regime of the region (Coronado et al., 2007) and the springs' location in the protected back-reef. The waves overtopping the reef are the main driving factor of circulation, which is generally slow (avg. 2–3 cm s−1), with faster (avg. 20 cm s−1) flow restricted to the northern and southern channels where the water exits the lagoon (Coronado et al., 2007). At the springs, the discharged waters with slightly lower salinity mainly flow vertically and not towards the sediment due to the buoyancy effect. The beach sediments in the area are composed of coarse (∼ 0.258 mm) carbonate sands of biogenic origin (Escudero et al., 2020).
Surface sediment samples (<1 cm depth) were retrieved using a plastic spoon at various distances from the center of six submarine springs (Fig. 1c; Gorgos, Laja, Mini, Norte, Agua, and Pargos) in October 2011. In the laboratory, samples were stained (rose bengal, 1 g L−1 ethanol), weighed, washed with deionized water through a 63 µm sieve mesh, and dried at 50 ∘C for 24 h. Discrete water samples were also retrieved near the sites of sediment collection for chemical analysis. Carbonate chemistry, temperature, and salinity data from seven samples reported in Martinez et al. (2018) were complemented with 20 additional samples collected on the same day following the protocols described by the authors. Briefly, the samples were filtered (0.2 µm) and split into aliquots for the analysis of salinity, total inorganic carbon (CT), and total alkalinity (AT), following the protocols of Dickson et al. (2007). The CT was measured on a CM5011 carbon coulometer (UIC, Inc.; analytical measurement error: ± 3 µmol kg−1) and TA using an automated open-cell, potentiometric titrator (Orion model 950; analytical measurement error: ± 2 µmol kg−1). Salinity was measured using a portable salinometer (Portasal Model 8410, Guild Line). Seawater temperature was measured in situ with a handheld YSI micro-processor (Yellow Springs model 63). The pH (seawater scale), carbonate ion concentration (CO), and calcite saturation state (Ωcalcite) were calculated using the program CO2Sys (Pierrot et al., 2006), using the CO2 dissociation constants of Lueker et al. (2000), KHSO4 of Dickson et al. (2007), and B concentration of Uppström (1974). Certified CO2 reference material (from Andrew Dickson lab at UC San Diego, batch 112) was used to calibrate all instruments.
2.2 Foraminiferal analysis
Sediment dry weight was recorded, and samples were homogenized and split into several small aliquots to allow efficient picking. The specimens found in each pre-weighted sediment aliquot were counted under a Zeiss STEMI 2000 stereomicroscope until a minimum of 250 specimens were recorded for each sample. Foraminiferal tests were identified to the lowest possible taxonomic level and assigned to informal species categories for diversity analyses. The taxonomic classification was based on the specialized bibliography of Cushman (1931) and Jones (1994), as well as supplementary taxonomic studies (Milker and Schmiedl, 2012; Abu-Zied et al., 2016; Sariaslan and Langer, 2021). Each species and genus were verified against the World Register of Marine Species (WoRMS, 2022) to ensure the use of the most recent nomenclature.
The samples were stained in rose bengal to consider the living counts. While most tests were at least partially stained, the proportions of fully stained specimens were small (∼ 3 %); hence total (alive plus dead) assemblages were used. We expect that the sample represents accumulation over several decades. This approach allows us to assess the mid-term responses of foraminiferal assemblages since the generational accumulation of tests in sediments integrate the effects of stressors over time (Hallock et al., 2003). This smooths out any seasonal fluctuations and therefore allows the foraminifera responses to average environmental conditions to be used (Scott and Medioli, 1980). We note that the low living percentage is a common pattern as most reef-dwelling taxa tend to live on phytal or hard substrates rather than directly on the sediments (Martin, 1986; Barbosa et al., 2009, 2012; Stephenson et al., 2015). Shannon–Wiener diversity index (H′) and Pielou's evenness (J′) were calculated considering the standardized foraminifera density for a volume of 1 cm3 sediment. These taxonomic metrics were calculated as follows: Shannon–Wiener diversity index with the equation , where Pi is the proportion of individuals per species; Pielou's evenness with the equation , where H′ is the Shannon–Wiener diversity index and S the species richness. Assemblage distributions were assessed according to differences in functional groups, i.e., symbiont bearing and opportunistic, and test type groups, i.e., small miliolids, small rotaliids, and agglutinated that do not present an opportunistic behavior. This approach has been used by Amergian et al. (2022) in nearby settings, based on categories designed by Hallock et al. (2003) for sensitivity/stress-tolerance taxa and by Murray (2006) for different test compositions.
2.3 Taphonomy and assemblage test size analysis
To analyze alteration by taphonomy, foraminiferal tests were classified into three categories: “optimally” (i.e., pristine tests), “well” (i.e., tests with weak taphonomic signals), and “poorly” (i.e., strongly abraded or fragmented tests) preserved, following the descriptions of Yordanova and Hohenegger (2002). Discoloration patterns were analyzed to detect any vertical mixing and exposure of relict tests. In general, if colored black (with iron/manganese sulfides), the tests indicate relict sediments deposited under reducing conditions, whereas a brown coloration indicates the oxygenation of iron through the reworking of the sediments (Maiklem, 1967); white tests indicate a lack of significant sediment burial and alteration. For a complete survey of the assemblage test size distribution, the surface area of all individuals was calculated using the ImageJ software (Schneider et al., 2012). All specimens picked were placed on the dorsal side in common brass picking trays and photographed under the same magnification and camera settings using an adapter for a microscope camera (Prazeres et al., 2015), to trace surface area changes (i.e., gain or loss) in large benthic foraminiferal species under low-pH conditions. Specifically, the test area was defined according to the gray-scale differences between the surface of the individual test (white) and background (black). The surface area parameter was the most suitable for the analysis considering the high taxonomical and consequently morphological diversity of PM samples since it identifies the size of the foraminifera tests in a standard way.
2.4 X-ray micro-CT
An X-ray micro-CT (micro computed tomography) analysis was performed on four individuals from ambient (7.96) and from low-pH conditions (7.11). To ensure that the analyzed tests represent living conditions, only tests in excellent condition, and therefore not influenced by post mortem processes of dissolution and transport, were selected. For the X-ray micro-CT acquisition, a V/TOMEX/M (GE Measurement and Control Solutions, Wunstorf, Germany) was used. The micro-CT parameters for the acquisition included a voltage of 60 kV, current of 100 µA, five frames, and an Al filter with a thickness of 0.5 mm. The geometry had a magnification of 31.81 and pixel size of 6.28 µm. Certified calcite standards were used to calibrate the density for the analyzed samples. The 3D reconstructions were performed using the Phoenix datos|x reconstruction software, in which a slice-aligning, beam-hardening correction was implemented, and a mathematical edge-enhancement filter was applied to achieve a higher contrast at the edges of the chambers. For the 3D visualization, the VGSTUDIO MAX v 3.0 and Avizo 2020.3 software packages were used. For calcite density analysis, the CT Analyser v. 1.18.4.0 software was used. Calcite density was assessed by the calcite density distribution calculated from the CT number that was determined based on the X-ray attenuation coefficient of each sample (Iwasaki et al., 2019). In addition, estimations of morphometric parameters such as total volume and chamber wall thickness distribution were performed.
2.5 Statistical analysis
A BIO-ENV procedure (9999 permutations) and global BEST test (statistical significance) were used to identify the set of explanatory environmental parameters that produced a Euclidean matrix that best correlated (Spearman method) the species assemblage similarity matrix and normalized environmental variables. Polynomial models (second order) were performed to investigate the relationships between carbonate chemistry and the taxonomical metrics (n=26). They were compared according to their contribution to the model's Akaike information criterion (AIC), and the models with the lowest AIC values (i.e., highest fit) were selected for the analysis. For comparison of A. angulatus microstructure parameters between high and low pH, Student's t test (n=8) was used for variables with normal distributions and homogenous variances. When these conditions were not met, Welch's t test was performed. We used the Kruskal–Wallis test to assess differences between functional groups, taxonomic metrics, and assemblage test size. For the latter, the stations were separated into four pH groups: 8.1–8.05 pH representing present-day conditions (n=4); 8.01–7.9 pH aligned with low–intermediate acidification scenarios SSP1-2.6 and SSP2-4.5 (n=11); 7.8–7.7 representing high-acidification scenarios SSP3-7.0 and SSP5-8.5 (n=4); 7.6–7.2 representing acidification conditions beyond those predicted for the end of the 21st century (n=7). Data normality and variance homogeneity were tested using the Shapiro–Wilk and Levene tests. The BIO-ENV and global BEST procedures were performed in Primer v.6 software (Clarke and Gorley, 2006). Student's t test, Welch's t test, the Kruskal–Wallis test, and data visualization were performed using R software (version 4.0.2; http://www.Rproject.org, last access: 31 March 2022, R Core Team, 2020).
3.1 Water chemistry
Seawater carbonate chemistry (Table 1) differed significantly between samples. Obtained ranges were as follows: pH = 7.2–8.1, Ωcalcite=1.3–6.2, [CO] = 52–240 µmol kg−1, TA=2044–3108 µmol kg−1, and CT= 1725–3197 µmol kg−1. The temperature was similar between sites ranging from 26.1–27.9 ∘C, while salinity decreased with proximity to the springs, ranging from 37–28. The BIOENV analysis and global BEST test revealed that the best combination (p-value = 0.01) of environmental variables with species abundance was observed when considering pH, [CO], Ωcalcite, and T (ρ= 0.55), in which CO and pH were the environmental variables resulting in the best correlation (ρ= 0.5) and salinity (ρ= 0.33) and temperature (ρ= 0.038) the lowest. Concerning the taxonomic metrics, the multiple regression analysis presented similar results. For diversity, richness, and evenness the pH model presented the lowest AIC values (26.96, 196.65, −67,05, respectively), indicating the central influence of this variable on the communities, while salinity (43.77, 209.71, −54,79, respectively) and temperature (59.46, 224.28, −40.65, respectively) were less influential. Interestingly, TA and CT were the most important variables correlating to foraminiferal density (AIC = 401.79 and 401.99). The salinity (AIC = 406.34) and temperature (AIC = 409.03) were not significant variables affecting foraminifera density. Weighing by relative likelihood (Akaike weights), log-likelihood, significance, and level of variation explained by each of the environmental parameters (R2) are available in Table S1. Considering its predominant influence, pH will be used as the primary variable for discussion of the potential impacts of changing carbonate chemistry. We note, however, that pH, Ωcalcite, TA, [CO, and CT are all strongly related to each other as important components of carbonate chemistry.
3.2 Taphonomy and assemblage test size analysis
Along the gradient of changing carbonate chemistry, a significant change in foraminiferal test size was observed (Kruskal–Wallis, chi-squared = 16, df = 3, p-value ). A gradual decrease in the abundance of tests with smaller surface area and a relative increase in larger tests are observed towards low-pH sites (Fig. 2a; R2= 0.73, p-value 0.01), whereas an abrupt increase in test size was observed at 7.8 pH. The post hoc Dunn's test reveals that only differences between present-day and extremely low-pH conditions, which are beyond those predicted by the end of the 21st century, were significant (z = -2.7, p-value ). Specifically, average test size in the assemblage more than tripled when compared to present-day conditions (from 0.33 ± 0.2 to 0.87 ± 0.14 mm2). This large change can be visualized in Fig. 2a and is likely related to changes in faunal composition rather than interspecific changes in species size. As observed in the taphonomy analysis, linear correlation with respect to the dominant taxa coverage, i.e., the species A. angulatus, shows a high and significant correlation to changes in average assemblage test size (Fig. 2c; R2= 0.89, p-value ). Raw data of assemblage average test size and taxonomic metrics are available in Table S2.
Sites with the highest pH (∼ 8.1 pH ) at PM are relatively pristine with well-preserved tests representing approximately 80 % of the assemblage; however, this gradually changes as the effects of spring water increase (Fig. 2b). In general, dissolution was not homogenous between species but mainly associated with the occurrence of LBF, specifically, Archaias angulatus, which was able to individually explain 73 % of highly dissolved test occurrence (R2= 0.73; Fig. 2c). The small, less robust calcifiers (e.g., Rosalina spp., Elphidium spp.) were rare, but when found, they were mostly in pristine conditions. Regarding color patterns (Table S2), only two specimens with brown color were found at Laja spring, indicating little reworking of sediments and therefore mixing of pristine and relict tests. Overall, we observed that the specimens were in good condition, composed of well-preserved time-averaged assemblages, which thus provide a good representation of the present-day biocoenosis (Yordanova and Hohenegger, 2002). However, at 7.7 pH and lower, high levels of taphonomical alteration started to occur (Fig. 2b–c, dashed lines), when poorly preserved tests comprised ∼ 50 % of total assemblages.
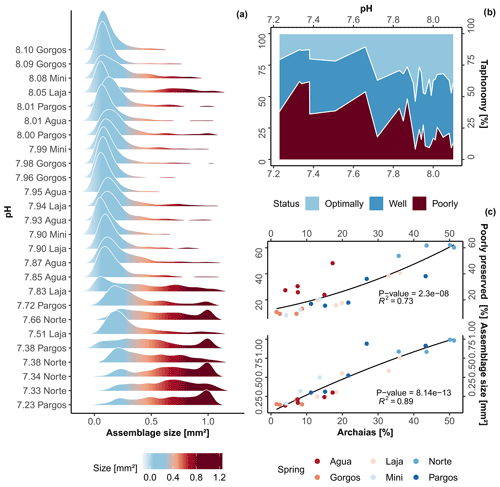
Figure 2(a) Density plot of assemblage test size, (b) area plot of foraminifera taphonomical status against pH, and (c) variation in poorly preserved tests and average assemblage test size against Archaias angulatus relative contribution. The black lines represent the second-order polynomial model fits along with the R2 value and p-value (c).
3.3 Foraminiferal analysis
The assemblages (alive + dead; Table S3) found at PM exhibit a similar composition to previous studies conducted in nearby coastal settings (Gischler and Möder, 2009), eastern Caribbean islands (Wilson and Wilson, 2011), and the Gulf of Mexico (Stephenson et al., 2015; Amergian et al., 2022). A total of 8564 foraminifera from 141 species were identified, belonging to 4 orders, 37 families, and 73 genera. Agglutinated species contributed 6.4 % (9 species), porcelaneous 61 % (86 species), and hyaline 32.6 % (45 species) of the total species richness. For total assemblages the species A. angulatus (9.4 %), Rotorbinella rosea (9.3 %), Asterigerina carinata (6.9 %), and the Rotorbis auberii (4.7 %) were the most important contributing taxa, whereas for living counts Rosalina globularis was the most important taxon (11 %). Species that contributed at least 3 % of total abundance are shown in Fig. 3.
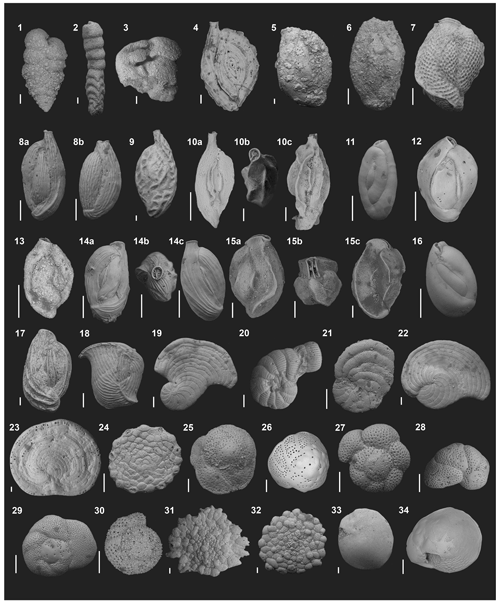
Figure 3Electron micrographs of foraminifera species from Puerto Morelos reef lagoon springs constituting at least 3 % of the assemblage. All scale bars represent 100 µm. (1) Textularia agglutinans, lateral view. (2) Clavulina angularis, lateral view. (3) Valvulina oviedoiana, lateral view. (4) Spiroloculina corrugata, lateral view. (5) Agglutinella compressa, lateral view. (6) Schlumbergerina alveoliniformis, lateral view. (7) Lachlanella carinata, lateral view. (8) Quinqueloculina subpoeyana, lateral view. (9) Quinqueloculina tricarinata, lateral view. (10a, 10c) Quinqueloculina cf. Quinqueloculina distorqueata, lateral views. (10b) Quinqueloculina conf. Quinqueloculina distorqueata, apertural view. (11) Quinqueloculina bosciana, lateral view. (12) Quinqueloculina disparilis, lateral view. (13) Quinqueloculina conf. berthelotiana, lateral view. (14a, 14c) Quinqueloculina carinatastriata, lateral views. (14b) Quinqueloculina carinatastriata, apertural view. (15a, 15c) Affinetrina quadrilateralis, apertural views. (15b) Affinetrina quadrilateralis, apertural view. (16) Miliolinella elongata, lateral view. (17) Pseudotriloculina linneiana, lateral view. (18) Articulina pacifica, lateral view. (19) Laevipeneroplis proteus, lateral view. (20) Peneroplis pertustus, lateral view. (21) Peneroplis planatus, lateral view. (22) Archaias angulatus, lateral view. (23) Cyclorbiculina compressa, lateral view. (24) Sorites marginalis, lateral view. (25) Rotorbis auberii, spiral view. (26) Rotorbinella rosea, spiral view. (27) Trochulina sp., spiral view. (28) Rosalina cf. floridana, spiral view. (29) Rosalina globularis, spiral view. (30) Cibicidoides sp, spiral view. (31) Planorbulina mediterranensis, lateral view. (32) Planogypsina acervalis, lateral view. (33) Amphistegina gibbosa, lateral view. (34) Asterigerina carinata, lateral view.
In general, the species Quinqueloculina tricarinata, A. angulatus, Amphistegina gibbosa, Valvulina oviedoiana, and Ciclorbiculina compressa increased towards low pH, high CT, and high TA values, presenting an increased relative abundance and lower sensitivity to OA. In contrast, highly sensitive species include Thochulina sp., Sorites marginalis, Quinqueloculina subpoeyana, and R. auberii. The species Rotorbinella rosea, Clavulina angularis, Quinqueloculina disparilis, Lachlanella carinata, and Schlumbergerina alveoliniformis decrease in abundance towards low pH at a lower rate compared to the highly sensitive species suggesting higher tolerance to lower saturation states.
The symbiont-bearing taxa (Fig. 4a; R2= 0.59, p-value 0.01) presented lower sensitivity to OA conditions, increasing in relative abundance towards low-pH conditions. The small miliolids (Fig. 4c; R2= 0.42, p-value 0.01), opportunistic taxa (Fig. 4d; R2= 0.28, p-value 0.01), and small rotaliid taxa (Fig. 4e; R2= 0.36, p-value 0.01) decreased in relative abundance towards low-pH conditions, indicating higher sensitivity. Kruskal–Wallis analysis reveals that the observed variation was statistically significant for most functional groups: symbiont-bearing (chi-squared = 13, df = 3, p-value 0.01), small miliolids (chi-squared = 12, df = 3, p-value 0.01), opportunistic (chi-squared = 16, df = 3, p-value 0.01), and small rotaliids (chi-squared = 9, df = 3, p-value 0.01). A post hoc Dunn test reveals that significant changes occurred predominantly between present-day (∼ 8.1 pH) and extremely low-pH conditions (≤ 7.6 pH), representing conditions beyond those predicted for the end of the 21st century: symbiont-bearing (z 2.38, p-value = 0.01), small miliolids (z = 2.7, p-value 0.01), and opportunistic (z = 2.4, p-value = 0.01). For small rotaliid taxa the significance was observed between low–intermediate acidification scenarios (∼ 7.9 pH), in which the group presented a higher contribution, and extremely low-pH conditions (≤ 7.6 pH), under which a strong decrease was observed (z = 1.7, p-value 0.01). No significance in abundance between sites was observed for agglutinated foraminifera (chi-squared = 2, df = 3, p-value = 0.5), which also did not present significant correlation with changing pH (Fig. 4b; R2= 0.11, p-value = 0.1). Raw data of the functional and the test type groups are given in Table S4 and the distribution of functional groups against changing pH in Fig. 4.
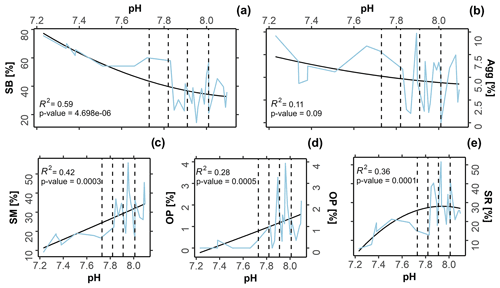
Figure 4Variation in functional groups versus pH. The black line represents the second-order polynomial model fits along with the R2 value, and the blue line represents the raw values obtained from in situ assemblages. Dashed lines demark predicted pH values at the end of this century following the Coupled Model Intercomparison Project Phase Six (CMIP6) predictions for Shared Socioeconomic Pathways (SSP1-2.6: 8.01 pH; SSP2-4.5: 7.91 pH; SSP3-7.0: 7.82 pH, and SSP4: 7.73 pH). SB = symbiont bearing; Agg = agglutinated; SM = small miliolids; OP = opportunistic; SR = small rotaliids.
All taxonomic metrics presented a gradual decrease towards the springs (Fig. 5a–d). On average, H′ ranged from 3.9 to 1.6 (Fig. 5a; R2= 0.72, p-value = 4.8−08), S from 71 to 11 (Fig. 5b; R2 = 0.67, p-value = 3.3−07), J′ from 0.9 to 0.6 (Fig. 5c; R2= 0.64, p-value = 9.5−07), and foraminiferal density from 2167 to 36 ind. cm−3 (Fig. 5d; R2= 0.22, p-value = 0.02). Kruskal–Wallis analysis revealed that the observed variation was statistically significant for all taxonomic metrics: N (chi-squared = 14.5, df = 3, p-value = ≤ 0.01), S (chi-squared = 20, df = 3, p-value 0.01), J′ (chi-squared = 15, df = 3, p-value 0.01), and H (chi-squared = 19, df = 3, p-value 0.01). However, as observed for the functional and test type groups, the post hoc Dunn test revealed that changes were most significant between present-day and extremely low-pH conditions: N (z = 2.2, p-value = 0.02), S (z = 3.4, p-value 0.01), J (z = 3.1, p-value 0.01), and H (z = 3.4, p-value 0.01). No significant difference was observed for any taxonomic metric at low–intermediate acidification scenarios (SSP1-2.6 and SSP2-4.5), and only S differed significantly between present-day and high-acidification scenarios (Fig. 5b; SSP3-7.0 and SSP5-8.5, z = 2.1, p-value = 0.03).
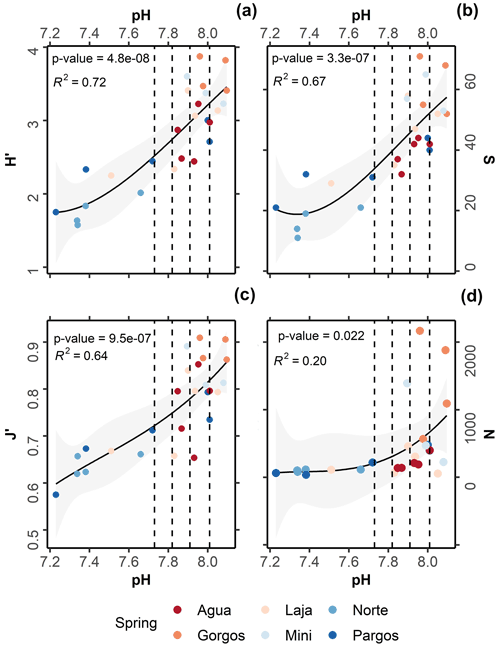
Figure 5Relationships between pH and (a) Shannon–Wiener diversity index (H′), (b) species richness (S), (c) Pielou's evenness (J′), and (d) foraminiferal density (N). The black lines represent second-order polynomial model fits, and grey areas mark 95 % confidence intervals. Dashed lines demark predicted pH values at the end of this century following the Coupled Model Intercomparison Project Phase Six (CMIP6) predictions for Shared Socioeconomic Pathways (SSP1-2.6: 8.01 pH; SSP2-4.5: 7.91 pH; SSP3-7.0: 7.82 pH, and SSP4: 7.73 pH).
Data analyses indicate that under the most conservative projections (SSP1-2.6; SSP2-4.5) foraminiferal assemblages did not display considerable changes in taxonomic metrics, relative to assemblages living under present-day conditions. For projections SSP3-7.0 and SSP5-8.5 the analyzed assemblages presented a significant decrease in richness S, indicating that foraminifera assemblages are likely to be affected under high-acidification scenarios. At the species level, agglutinated foraminifera were not measurably influenced by changes in pH, while the small rotaliids and symbiont-bearing taxa presented relatively higher resistance. For conditions beyond those predicted for the late 21st century, foraminifera density decreased abruptly and high taphonomical alteration was observed.
3.4 X-ray micro-CT
The X-ray micro-CT (Fig. 6a–d) analysis revealed that despite having a similar size (0.80 ± 0.05 mm3) and volume (0.06 ± 0.02 mm3), specimens present at low-pH conditions (7.1 pH) were on average 46 % less dense (2.4 ± 0.2 to 1.30 ± 0.03 g cm−3) than the specimens present at high-pH conditions (Welch two sample t test, t = 8.1204, df = 3.0808, p-value = 0.0035). Yet, no significant (two sample t test, t = −1.4378, df = 6, p-value = 0.2) difference in chamber wall thickness was observed (0.050 ± 0.006 mm). The differences in internal density (Fig. 6a and b) represent two specimens, one living in high-pH and the other in low-pH conditions, respectively. The external differences in these same individuals are represented in the 3D volume image in Fig. 6c and d. Raw data of test density, chamber wall thickness, test volume, and test diameter measured in A. angulatus individuals are listed in Table S5.
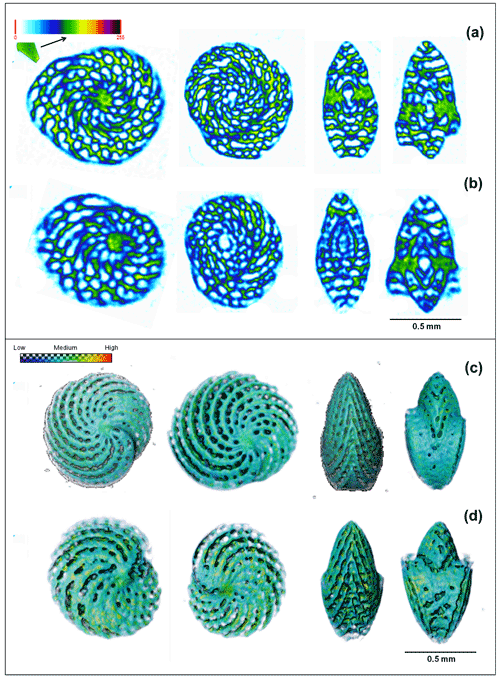
Figure 6Comparison between X-ray micro-CT images with color code as a function of calcite density. The specimen living in ∼ 7.96 pH (a) presents a higher calcite density (greener) when compared with the individual at the low ∼ pH 7.11 (bluer) (b). The 3D volume rendering as a function of calcite density for the same individuals living at the high- (c) and low-pH conditions (d). Note that the individual at “d” living in low pH presents a test with incomplete parts and blurred edges, which demonstrates a lower density.
4.1 Foraminiferal resistance to intermediate-pH conditions
Under the two most conservative acidification projections (Fig. 5a–d) foraminifera assemblages in PM did not display considerable changes, while for high-acidification scenarios a significant decrease in species richness was observed. These results indicate that benthic foraminifera are unlikely to be affected by pH decreases of ∼ 0.2 but certainly respond adversely to higher acidification levels (∼ 0.4 pH). These findings are generally consistent with previous observations from other naturally high-pCO2 sites in which taxonomic metrics decreased significantly with declining pH (Bernhard et al., 2009; Dias et al., 2010; Pettit et al., 2015; Dong et al., 2019, 2020). It is noteworthy, however, that changes in assemblage composition did not follow the same pattern observed in these previous studies. Whereas the proportion of calcareous species usually declines with decreasing pH, they remained dominant in PM (∼ 90 %, mainly SB; Fig. 4a) under all projections.
Considering the mid-range pH (∼ 7.9 pH), small rotaliids are more resilient (Fig. 4e); the chemical conditions at PM, along with the physiology of calcification in foraminifera, may explain the lack of sensitivity of these species. Recent calcification models demonstrate that hyaline foraminifera can manipulate pH to control the speciation of inorganic carbon parameters during calcification (De Nooijer et al., 2009; Toyofuku et al., 2017; De Goeyse et al., 2021; Geerken et al., 2022). Specifically, the proton-pumping-based model (Toyofuku et al., 2017) shows that a decrease in pH (∼ 6.9 pH) in the environment induces the transformation of CO and bicarbonate (HCO3−) into CO2, whereas at the site of calcification the elevated pH (∼ 9 pH) results in the opposite shift converting to CO. As foraminifera induce pH changes exceeding those predicted from SSP1-2.6 and SSP2-4.5, intermediate acidification scenarios are unlikely to impair foraminiferal calcification. In fact, the higher abundance of small rotaliids (Fig. 4e) and resistance of SB genera (e.g., Amphistegina) support the hypothesis that they might, at least to a certain extent, benefit from the extra dissolved inorganic carbon (Toyofuku et al., 2017; De Goeyse et al., 2021). This interpretation is consistent with the observation that carbonic anhydrase plays a key role in the biomineralization process of some rotaliids, possibly concentrating inorganic carbon for calcification by converting HCO into CO2 (De Goeyse et al., 2021). As such, these models suggest that increased CO2 might favor foraminifera calcification by increasing CT, which is notably higher towards the springs in PM (Table 1).
The higher CT and TA may also induce CO2 fertilization effects in SB species, increasing the activity of symbionts (Fujita et al., 2011; Uthicke and Fabricius, 2012; Martinez et al., 2018). This could explain why symbiont-bearing species, including A. angulatus (chlorophyte-bearing), increased in relative abundance from 11 %–15 % to 21 % from ∼ 8 to 7.72 pH (e.g., Pargos spring). This behavior was also observed for Amphistegina gibbosa (diatom-bearing), increasing from 16 %–19 % to 23 %, and Cyclorbiculina compressa (chlorophyte-bearing) that contributed only ∼ 1 % at high-pH stations but increased in relative contribution to 3.2 % at intermediate pH. These findings are supported by laboratory-controlled experiments demonstrating that both A. angulatus (Stuhr et al., 2021) and Amphistegina sp. (McIntyre-Wressnig et al., 2013; Prazeres et al., 2015) can calcify and live under relatively low-pH conditions (∼ 7.6 pH).
This behavior, however, was not observed for all SB foraminifera. For example, the species H. depressa was documented to be resilient in laboratory-controlled conditions (Vogel and Uthicke et al., 2012; Schmidt et al., 2014), but in PM it showed a strong decline towards low-pH waters. A possible explanation is that although salinity is considered to not be a significant factor controlling the overall foraminiferal communities (AIC, BIO-ENV and global BEST analysis), salinity may have specifically affected the occurrence of select stenohaline (30–45) species, e.g., larger rotaliids (Hallock, 1986). However, this is unlikely important since salinity at the springs is >30 over 90 % of the time and does not drop below 27 (Crook et al., 2012; Martinez et al., 2018). Other parameters, such as heavy metals could also influence the abundance of certain species, but concentrations of the metals (Paytan, unpublished) were not significantly higher at the springs when compared to sites not influenced by spring water discharge (>1 m away from the discharge sites). Hence, we do not attribute the changes in foraminifera assemblages to impacts of heavy metals. We note that all of the samples collected and analyzed here are just a few meters apart; hence other parameters such as light, eutrophication, and pollution are identical.
The high CT and TA might also raise local pH and carbonate saturation during photosynthesis, even if only on the specimen scale (i.e., at the foraminiferal shell surface). A diffusive boundary layer of high pH (up to 8.9) has been documented on the underlying surface of symbiont-bearing foraminifera (Koehler-Rink and Kuehl, 2000; Glas et al., 2012), and although insufficient to compensate future decreases in ambient seawater pH, it might increase the symbiont-bearing species' resistance to (periodical) lowered saturation states. Correspondently, the symbiosis between seagrasses and foraminifera has also been suggested as a key factor in the resilience of epiphytic species (e.g., A. angulatus, C. compressa, and A. gibbosa). Although no significant effect has been reported for some species (Fabricius et al., 2011; Pettit et al., 2015), Marginopora vertebralis was observed to maintain its growth when associated with its common algal host, Laurencia intricata, in laboratory conditions (Doo et al., 2020). With respect to observations in the present study, the epiphytic species R. globularis was the abundant taxon in the living community (11 %), and although not the primary objective of the present study, it gives important insights about short-term foraminifera responses. Specifically, this finding agrees with the observed resilient behavior of rosalinids in the natural, low-pH venting sites of Panarea (Di Bella et al., 2022). Considering the low occurrence of fully stained tests, future analysis on phytal substrates in PM would be necessary to confirm this trend. Lastly, the ability of foraminifera to function and calcify near the springs may also be related to the site-specific natural pH variability to which the species are exposed. For many coastal and transitional areas characterized by high pCO2 variability, foraminifera seem to be more resilient and acclimated to changing conditions including low pH (Haynert et al., 2012; Charrieau et al., 2018). By discharging low-pH waters for millennia (Back et al., 1979) the foraminifera living near the spring have experienced pH variability over a much longer time span than the life span of individual organisms (Martinez et al., 2018). Specifically, as reef-dwelling organisms, the foraminifera in PM experience a wide range of pH levels on daily and seasonal scales which might physiologically increase the species resilience at low-pH conditions (Price et al., 2012).
4.2 High-acidification scenarios
Previous data from recruitment and succession experiments in PM showed that foraminifera were able to calcify and increased in weight over the investigated period (14 months) at low-pH (∼ 7.8) conditions (data from Laja and Gorgos springs; Crook et al., 2016). Two years later, Martinez et al. (2018) documented the occurrence of calcareous tests at PM even at extreme acidification levels (∼ 7.1 pH). In agreement, we observed that despite the strong decrease in foraminifera density, calcareous foraminifera remained dominant in PM sediments (∼ 90 %, mainly A. angulatus and A. gibbosa) even at expected future conditions for the end of the 21st century and beyond.
For high-acidification scenarios (SSP3-7.0 and SSP5-8.5), the in situ occurrence of calcifying foraminifera has only been reported in the deep sea near extensive CO2 vents in the Wagner Basin (Pettit et al., 2013). At this site, a rich food supply and stable temperatures were hypothesized to compensate the effects of OA and explain the shift towards opportunistic assemblages. The springs from PM also have relatively high nutrient concentrations compared to the open waters in the region (Null et al., 2014; Crook et al., 2016); however, near the springs, assemblages did not change towards opportunistic-dominated assemblages, suggesting that the nutrient availability does not exert a major control at this site. Rather, the high-pH assemblages which were heavily dominated by small calcareous forms were replaced by larger symbiont-bearing species near the springs (Fig. 4a–e). Such species are known to be sensitive to high nutrient loading likely because of changes in turbidity and/or light regimes and their dependence on algal symbionts to enhance growth and calcification (Hallock et al., 2003; Prazeres et al., 2020; Girard et al., 2022). However, at PM despite higher nutrient levels the waters at the springs are clear, and light regimes are not reduced (water depth at the spring sites is 5–7 m).
To explore the causes for the resilience seen in certain taxa (Fig. 4a–d) and investigate possible acclimation patterns, we employed an X-ray micro-CT analysis in A. angulatus specimens from high- and low-pH conditions. The analysis (Fig. 6a–d) revealed that despite having a similar size, volume, and chamber wall thickness, the specimens found at low-pH conditions (7.1 pH) were on average 46 % less dense than the specimens present at high-pH conditions (7.96 pH). This demonstrates that this species is able to calcify in low-pH conditions beyond those predicted for the late 21st century, albeit with shells that have a lower average density. This indicates that Archaias individuals were not capable to acclimate sufficiently to maintain calcification efficiency similar to ambient present-day rates. These results agree with Knorr et al. (2015), who observed a 50 % decrease in A. angulatus size at 7.6 pH and a consequent decrease of 85 % in the production of high-Mg calcite by this species, as well as with other published results for SB species such as Peneroplis spp. (pH 7.4, approximately 25 % lower; Charrieau et al., 2022), and Amphistegina spp. (pH 7.6, approximately 20 % lower; Prazeres et al., 2015). We acknowledge that post mortem dissolution may also contribute to the observed lower density, but only the most pristine tests were analyzed, so this influence must be minimal. Future analysis of B isotopes and ratios could provide more information about the trends documented in the present study. Since A. angulatus showed lower density close to the low-pH springs and hence is negatively impacted by the low pH, the species increase in relative abundance towards the springs is probably associated with the high preservation potential of its tests. The tests of A. angulatus are large, thick, and reinforced by internal partitions (pillars) and are therefore more likely to be preserved in the sediment (Martin, 1986; Cottey and Hallock, 1988). This is confirmed by the performed regression analysis as the species relative contribution explains 88 % of assemblage test size and 73 % of high dissolved test occurrence in the samples (Fig. 2c). In fact, changes were so abrupt that shifts in the assemblage test size and functional groups were clearly observed at ∼ 7.7 pH (Fig. 2a), when the symbiont-bearing taxa relative contribution also started to increase (Fig. 4a). At this point preservation thresholds of smaller taxa seemed to be crossed, and their decrease in relative abundance near the springs is likely related to higher rates of breakage and dissolution (Present study, Martinez et al., 2018).
Considering foraminifera as a crucial component of reef sediment production (Langer et al., 1997; Langer, 2008), including A. angulatus in the Caribbean region, our results support previous findings that reef-building carbonate production and accumulation are likely to decrease under future OA scenarios, even in the tropics (Knorr et al., 2015; Eyre et al., 2018; Kuroyanagi et al., 2021). Specifically, we also observed a decrease in foraminifera test density (Fig.5d) and therefore in carbonate accumulation. As OA intensifies, symbiont-bearing taxa, which demonstrated higher resistance to low pH (>7.8 pH ), will likely still represent major contributors in the Caribbean and Gulf of Mexico to sediments where species like A. angulatus may dominate (Culver and Buzas, 1982). In contrast, the high sensitivity of Quinqueloculina spp., Triloculina spp., Articulina spp., and Miliolinella spp. to low pH highlighted their lower fitness in response to OA, demonstrating that changes in abundance of small taxa can be used as bioindicators to monitor the effects of OA.
The relative contribution of agglutinated foraminifera slightly increased towards the low-pH springs (Fig. 4b), but they did not fully compensate the decline in calcareous species (Fig. 4a–e). Since the particles available for the agglutinated tests in this region are made of calcium carbonate, under low-Ω waters these particles are also prone to dissolution, possibly affecting agglutinating species. Interestingly, agglutinated foraminifera also presented species-specific responses to acidification like calcareous foraminifera. For example, Valvulina oviedoiana increased in relative abundance towards low pH, while Textularia agglutinans presented a strong decrease. Since acidification is expected to have little direct effect on agglutinated foraminifera, the observed interspecific behavior is also probably associated with preservation potential. The variation in agglutinating material (e.g., mucopolysaccharide), structure (e.g., fibrous, strands, foam-like masses), and size of granular particles (e.g., fine, and coarser) is essential to determine the preservation and accumulation of agglutinated tests (Bender and Hemleben, 1988). The most important agglutinated species in our study, e.g., T. agglutinans, C. angulata, and V. oviedoiana, use calcite cement as the binding material of particles, which probably results in a higher resistance to dissolution (Bender, 1995). Among these, T. agglutinans's lower resistance is likely a response to its smaller size, which enhances dissolution (Bender, 1995). Altogether, we observe that between 8.1 and 7.8 pH, foraminifera physiology was a main driver of foraminifera distribution, whereas at ≤ 7.7 pH (Fig. 2b) the preservation potential became an important factor affecting the distribution of both calcareous and agglutinated tests.
We cannot exclude the possibility that the higher accumulation of A. angulatus tests could be responsible for an overestimation in symbiont-bearing taxa density. In this case, species richness would be more reliable to the interpretation of assemblage responses, which was the only parameter to decrease significantly at a pH below 7.7 (Fig. 5b), suggesting that overall foraminifera are less likely to acclimate under high-acidification scenarios. These results bring serious implications for foraminifera communities' resilience in this century as SSP3-7.0 and SSP5-8.5 scenarios also predict substantial increases in sea surface temperature (Kwiatkowski et al., 2020), which combined with surface OA might critically decrease the tolerance of foraminifera (reviewed in Kawahata et al., 2019). Recently, Bernhard et al. (2021) observed that foraminiferal assemblages presented the lowest number of species and abundances under a triple-stress treatment (low pH and O2 and high temperature) demonstrating the synergetic effects of these variables. As observed in PM, agglutinated foraminifera were relatively more resistant than calcareous taxa.
In general, for emissions beyond those predicted by the end of the 21st century (resulting in a pH below 7.7) all taxonomic metrics decreased significantly, and calcareous species with higher preservation potential like C. compressa and A. angulatus comprised up to 50 %–60 % of the total assemblage. This was expected since a drop in the Ω aragonite <3.2 would increase foraminifera dissolution (Yamamoto et al., 2012), but these calcareous taxa were still found at the center of discharge where the surface sediments were still composed of carbonate. We attribute this to high TA levels, which was suggested as a parameter that limits the dissolution rates of A. angulatus and other porcelaneous tests in the springs on the coast of Florida (Amergian et al., 2022). The high TA may specifically provide a calcification optimum within the polyhaline (22–30) waters both at the springs in Florida and in PM where a similar range of salinity was observed. This hypothesis could explain the observed resistance of A. angulatus in the present study and the higher association of foraminifera density to TA. We note that when restricting our analysis to pristine, well-preserved tests, the taxonomic metrics at 7.7–7.2 pH (Fig. 5) would be much lower and more like those presented by Uthicke et al. (2013), in which foraminifera were almost absent at sites with ≤ 7.9 pH.
Despite their life-long exposure to low-pH conditions, benthic tropical foraminifera species could be negatively affected under the high-acidification scenarios (SSP3-7.0 and SSP5-8.5) for the end of the 21st century. Species-specific responses in foraminiferal assemblages were observed, and as the oceans become more acidic, reef foraminiferal assemblages may gradually shift towards hyaline, symbiont-bearing, and agglutinating species. The species A. angulatus, which is known to be dominant in warm, oligotrophic areas of the Caribbean and the Gulf of Mexico, can calcify at pH conditions lower than those projected by SSP5-8.5; however, the observed lower density of the pristine tests suggests that reef carbonate budget may decrease as this species represents a major carbonate producer in these areas. Considering the observed trends of increasing average assemblage test size, our results demonstrate the key role smaller foraminifera have as bioindicators to monitor the effects of OA, as their high sensitivity to dissolution makes them first responders to ongoing OA.
All data related to this study are given in the Supplement data files accompanying this paper.
The supplement related to this article is available online at: https://doi.org/10.5194/bg-19-5269-2022-supplement.
DF, AP, and CFB conceived of and designed the study. DF performed the faunal and statistical analysis. OMOdA and RTL conducted the Micro-CT experiments. DF, AP, and CFB analyzed the data. DF, AP, and CFB prepared the original draft of the manuscript with writing, and OMOdA and RTL reviewed and edited it.
The contact author has declared that none of the authors has any competing interests.
Publisher’s note: Copernicus Publications remains neutral with regard to jurisdictional claims in published maps and institutional affiliations.
This study was funded by the National Science Foundation-1040952 (to Adina Paytan). Daniel François thanks the scholarship of the National Council for Scientific and Technological Development (CNPq) no. 132210/2020-7. Cátia Fernandes Barbosa acknowledges the Buzas Award for Travel (BAT) received from the Cushman Foundation for Foraminiferal Research. The funders had no role in the study design, data collection and analysis, decision to publish, or preparation of the paper. Daniel François thanks Pamela Hallock, Heitor Evangelista, Lennart de Nooijer, Sven Uthicke, and the anonymous reviewers for their helpful comments and suggestions.
This research has been supported by the National Science Foundation (grant no. OCE-1040952).
This paper was edited by Tyler Cyronak and reviewed by Sven Uthicke and two anonymous referees.
Abu-Zied, R. H., Al-Dubai, T. A., and Bantan, R. A.: Environmental conditions of shallow waters alongside the southern Corniche of Jeddah based on benthic foraminifera, physico-chemical parameters and heavy metals, J. Foramin. Res., 46, 149–170, https://doi.org/10.2113/gsjfr.46.2.149, 2016.
Amergian, K. E., Beckwith, S., Gfatter, C., Selden, C., and Hallock, P.: Can areas of high alkalinity freshwater discharge provide potential refugia for marine calcifying organisms?, J. Foramin. Res., 52, 63–76, https://doi.org/10.2113/gsjfr.52.1.60, 2022.
Back, W. and Hanshaw, B. B.: Comparison of chemical hydrogeology of the carbonate peninsulas of Florida and Yucatan, J. Hydrol., 10, 330–368, https://doi.org/10.1016/0022-1694(70)90222-2, 1970.
Back, W., Hanshaw, B. B., Pyle, T. E., Plummer, L. N., and Weidie, A. E.: Geochemical significance of groundwater discharge and carbonate solution to the formation of Caleta Xel Ha, Quintana Roo, Mexico, Water Resour. Res., 19, 1521–1535, https://doi.org/10.1029/WR015I006P01521, 1979.
Barbosa, C. F., Prazeres, M., Padovani, B., and Seoane, J. C. S.: Foraminiferal assemblage and reef check census in coral reef health monitoring of East Brazilian margin, Mar. Micropaleontol., 73, 62–69, https://doi.org/10.1016/j.marmicro.2009.07.002, 2009.
Barbosa, C. F., Ferreira, B. P., Seoane, J. C. S., Oliveira-Silva, P., Gaspar, A. L. B., Cordeiro, R. C., and Soares-Gomes, A.: Foraminifer-based coral reef health assessment for southwestern Atlantic offshore archipelagos, Brazil, J. Foramin. Res., 42, 169–183, https://doi.org/10.2113/gsjfr.42.2.169, 2012.
Beddows, P. A., Smart, P. L., Whitaker, F. F., and Smith, S. L.: Decoupled fresh – saline groundwater circulation of a coastal carbonate aquifer: Spatial patterns of temperature and specific electrical conductivity, J. Hydrol., 346, 18–32, https://doi.org/10.1016/j.jhydrol.2007.08.013, 2007.
Bender, H.: Test structure and classification in agglutinated Foraminifera, in: Proceedings of the Fourth International Workshop on Agglutinated Foraminifera, edited by: Kaminski, M. A., Geroch, S., and Gasiñski, M. A., Kraków Poland, September 12–19, 1993, 27–70, Grzybowski Foundation, Special Publication, 3, http://gf.tmsoc.org/Spec-Publ-3.html (last access: 17 November 2022), 1995.
Bender, H. and Hemleben, C.: Constructional aspects in test formation of some agglutinated foraminifera, Abh. Geol. B.-A., 41, 13–22, 1988.
Bernhard, J. M., Barry, J. P., Buck, K. R., and Starczak, V. R.: Impact of intentionally injected carbon dioxide hydrate on deep-sea benthic foraminiferal survival, Glob. Change Biol., 15, 2078–2088, https://doi.org/10.1111/j.1365-2486.2008.01822.x, 2009.
Bernhard, J. M., Wit, J. C., Starczak, V. R., Beaudoin, D. J., Phalen, W. G., and Mccorkle, D. C.: Impacts of multiple stressors on a benthic foraminiferal community: a long-term experiment assessing response to ocean acidification, hypoxia and warming, Front. Mar. Sci., 8, 1–18, https://doi.org/10.3389/fmars.2021.643339, 2021.
Charrieau, L. M., Filipsson, H. L., Nagai, Y., Kawada, S., Ljung, K., Kritzberg, E., and Toyofuku, T. Decalcification and survival of benthic foraminifera under the combined impacts of varying pH and salinity, Mar. Environ. Res., 138, 36–45, https://doi.org/10.1016/j.marenvres.2018.03.015, 2018.
Clarke, K. R. and Gorley, R. N.: PRIMER v6: User manual/tutorial, PRIMER-E Ltd., Plymouth Marine Laboratory, UK, 2006.
Coronado, C., Candela, J., Igresias-Prieto, R., Sheinbaum, J., López, M., and Ocampo-Torres, F. J.: On the circulation in the Puerto Morelos fringing reef lagoon, Coral Reefs, 26, 149–163, https://doi.org/10.1007/s00338-006-0175-9, 2008.
Cottey, T. L. and Hallock, P.: Test surface degradation in Archaias angulatus, J. Foramin. Res., 8, 187–202, https://doi.org/10.2113/gsjfr.18.3.187, 1988.
Crook, E. D., Potts, D., Hernandez, L., and Paytan, A.: Calcifying coral abundance near low-pH springs: implications for future ocean acidification, Coral Reefs, 31, 239–245, https://doi.org/10.1007/s00338-011-0839-y, 2012.
Crook, E. D., Cohen, A. L., Rebolledo-Vieyra, M., Hernandez, L., and Paytan, A.: Reduced calcification and lack of acclimatization by coral colonies growing in areas of persistent natural acidification, P. Natl. Acad. Sci. USA, 110, 11044–11049, https://doi.org/10.1073/pnas.1301589110, 2013.
Crook, E. D., Kroeker, K. J., Potts, D. C., and Rebolledo-Vieyra, M.: Recruitment and succession in a tropical benthic community in response to in-situ ocean acidification, PLoS ONE, 11, e0146707, https://doi.org/10.1371/journal.pone.0146707, 2016.
Culver, S. J. and Buzas, M. A.: Distribution of Recent benthic foraminifera in the Caribbean area, Smithsonian Institution Press, Washington, https://doi.org/10.5479/si.01960768.14.1, 1982.
Cushman, A.: The Foraminifera of the Atlantic Ocean pt. 8: Rotaliidae, Amphisteginidae, Calcarinidae, Cymbaloporettidae, Globorotaliidae, Anomalinidae, Planorbulinidae, Rupertiidae, and Homotremidae, Bulletin of the United States National Museum, 1–179, https://doi.org/10.5479/si.03629236.104.7, 1931.
De Goeyse, S., Webb, A. E., Reichart, G. J., and De Nooijer, L. J.: Carbonic anhydrase is involved in calcification by the benthic foraminifer Amphistegina lessonii, Biogeosciences, 18, 393–401, https://doi.org/10.5194/bg-18-393-2021, 2021.
De Nooijer, L. J., Langer, G., Nehrke, G., and Bijma, J.: Physiological controls on seawater uptake and calcification in the benthic foraminifer Ammonia tepida, Biogeosciences, 6, 2669–2675, https://doi.org/10.5194/bg-6-2669-2009, 2009.
Di Bella, L., Conte, A. M., Conti, A., Esposito, V., Gaglioti, M., Ingrassia, M., De Vittor, C., and Bigi, S.: Potential resilience to ocean acidification of benthic foraminifers living in Posidonia oceanica Meadows: The case of the shallow venting site of Panarea, Geosciences, 12, 184, https://doi.org/10.3390/geosciences12050184, 2022.
Dias, B. B., Hart, M. B., Smart, C. W., and Hall-Spencer, J. M.: Modern seawater acidification: the response of foraminifera to high-CO2 conditions in the Mediterranean Sea, J. Geol. Soc. Lond., 167, 843–846, https://doi.org/10.1144/0016-76492010-050, 2010.
Dickson, A. G., Sabine, C. L., and Christian, J. R.: Guide to best practices for ocean CO2 measurements, North Pacific Marine Science Organization, Sidney, BC, Canada, ISBN: 1-897176-07-4, 2007.
Doney, S. C., Busch, D. S., Cooley, S. R., and Kroeker, K. J.: The impacts of ocean acidification on marine ecosystems and reliant human communities, Annu. Rev. Environ. Resour., 45, 83–112, https://doi.org/10.1146/annurev-environ-012320-083019, 2020.
Dong, S., Lei, Y., Li, T., and Jian, Z.: Changing structure of benthic foraminiferal communities due to declining pH: Results from laboratory culture experiments, Sci. China Earth Sci., 62, 1151–1166, https://doi.org/10.1007/s11430-018-9321-6, 2019.
Dong, S., Lei, Y., Li, T., and Jian, Z.: Response of benthic foraminifera to pH changes: Community structure and morphological transformation studies from a microcosm experiment, Mar. Micropaleontol., 156, 101819, https://doi.org/10.1016/j.marmicro.2019.101819, 2020.
Doo, S. S., Hamylton, S., Finfer, J., and Byrne, M.: Spatial and temporal variation in reef-scale carbonate storage of large benthic foraminifera: a case study on One Tree Reef, Coral Reefs, 36, 293–303, https://doi.org/10.1007/s00338-016-1506-0, 2016.
Doo, S. S., Leplastrier, A., Graba-Landry, A., Harianto, J., Coleman, R. A., and Byrne, M.: Amelioration of ocean acidification and warming effects through physiological buffering of a macroalgae, Ecol. Evol., 10, 8465–8475, https://doi.org/10.1002/ece3.6552, 2020.
Engel, B. E., Hallock, P., Price, R. E., and Pichler, T.: Shell dissolution in larger benthic foraminifers exposed to pH and temperature extremes: Results from an in-situ experiment, J. Foramin. Res., 45, 190–203, https://doi.org/10.2113/gsjfr.45.2.190, 2015.
Escudero, M., Mendonza, E., and Silva, R.: Micro sand engine beach stabilization strategy at Puerto Morelos, Mexico, J. Mar. Sci, 8, 247, https://doi.org/10.3390/jmse8040247, 2020.
Eyre, B. D., Cyronak, T., Drupp, P., De Carlos, E. H., Sach, J. P., and Andersson, A. J.: Coral reefs will transition to net dissolving before end of century, Science, 359, 908–911, https://doi.org/10.1126/science.aao1118, 2018.
Fabricius, K. E., Langdon, C., Uthicke, S., Humphrey, C., Noonan, S., De`ath, G., Okazaki, R., Muehllehner, N., Glas, M. S., and Lough, J. M.: Losers and winners in coral reefs acclimatized to elevated carbon dioxide concentrations, Nat. Clim. Change, 1, 165–169, https://doi.org/10.1038/NCLIMATE1122, 2011.
Fujita, K., Hikami, M., Suzuki, A., Kuroyanagi, A., Sakai, K., Kawahata, H., and Nojiri, Y.: Effects of ocean acidification on calcification of symbiont-bearing reef foraminifers, Biogeosciences, 8, 2089–2098, https://doi.org/10.5194/bg-8-2089-2011, 2011.
Geerken, E., De Nooijer, L. J., Toyofuku, T., Roepert, A., Middelburg, J. J., Kienhuis, M. V. M., Nagai, Y., Polerecky, L., and Reichart, G. J.: High precipitation rates characterize biomineralization in the benthic foraminifer Ammonia beccarii, Geochim. Cosmochim. Ac., 318, 70–82, https://doi.org/10.1016/j.gca.2021.11.026, 2022.
Girard, E., B., Estradivari, Ferse, S., Ambo-Rappe, R., Jompa, J., and Renema, W.: Dynamics of large benthic foraminiferal assemblages: A tool to foreshadow reef degradation?, Environ. Pollut., 811, 151396, https://doi.org/10.1016/j.scitotenv.2021.151396, 2022.
Gischler, E. and Möder, A.: Modern benthic foraminifera on Banco Chinchorro, Quintana Roo, Mexico, Facies, 55, 27–35, https://doi.org/10.1007/s10347-008-0162-4, 2009.
Glas, M. S., Fabricius, K. E., De Beer, D., and Uthicke, S.: The O2, pH and Ca2+ Microenvironment of Benthic Foraminifera in a High CO2 World, PLOS ONE, 7, e50010, https://doi.org/10.1371/journal.pone.0050010, 2012.
Hallock, P.: Larger foraminifera: A tool for paleoenvironmental Analysis of Cenozoic Carbonate Depositional Facies, Palaios, 1, 55–64, 1986.
Hallock, P., Lidz, B. H., Burkhard-Cockey, E. M., and Donnelly, K. B.: Foraminifera as bioindicators in coral reef assessment and monitoring: The FORAM Index, Environ. Monit. Assess., 81, 221–238, https://doi.org/10.1023/A:1021337310386, 2003.
Haynert, K., Schönfeld, J., Polovodova-Asteman, I., and Thomsen, J.: The benthic foraminiferal community in a naturally CO2-rich coastal habitat of the southwestern Baltic Sea, Biogeosciences, 9, 4421–4440, https://doi.org/10.5194/bg-9-4421-2012, 2012.
Hernandez-Terrones, L. M., Street, J., Null, K., and Paytan, A.: Groundwater chemistry and Sr isotope ratios shed light on connectivity and water-rock interactions in the coastal aquifer of the Caribbean coast, Mexico, Cont. Shelf Res., 212, 104293, https://doi.org/10.1016/j.csr.2020.104293, 2021.
Hughes, T. P., Barnes, M. L., Bellwood, D. R., Cinner, J. E., Cumming, G. S., Jackson, J. B.C., Kleypas, J., Van De Leemput, I. A., Lough, J. M., Morrison, T. H., Palumbi, S. R., Van Nes, E. H., and Scheffer, M.: Coral reefs in the Anthropocene, Nature, 546, 82–90, https://doi.org/10.1038/nature22901, 2017.
IPCC: Climate Change 2021: The Physical Science Basis, contribution of working group I to the sixth assessment report of the intergovernmental panel on climate change, edited by: Masson-Delmotte, V., Zhai, P., Pirani, A., Connors, S. L., Péan, C., Berger, S., Caud, N., Chen, Y., Goldfarb, L., Gomis, M. I., Huang, M., Leitzell, K., Lonnoy, E., Matthews, J. B. R., Maycock, T. K., Waterfield, T., Yelekçi, O., Yu, R., and Zhou, B., Cambridge University Press, https://doi.org/10.1017/9781009157896, 2021.
Iwasaki, S., Kimoto, K., Okazaki, Y., and Ikehara, M.: X-ray micro-CT scanning of tests of three planktic foraminiferal species to clarify dissolution process and progress, Geochem. Geophy. Geosy., 20, 6051–6065, https://doi.org/10.1029/2019GC008456, 2019.
Jones, R. W.: The challenger foraminifera – The Natural History Museum. Oxford University Press, London, ISBN: 0198540965, 1994.
Kawahata, H., Fujita, K., Iguchi, A., Inoue, M., Iwasaki, S., Kuroyanagi, A., Maeda, A., Manaka, T., Moriya, K., Takagi, H., Toyofuku, T., Yoshimura, T., and Suzuki, A.: Perspective on the response of marine calcifiers to global warming and ocean acidification — Behavior of corals and foraminifera in a high CO2 world “hot house”, Prog. Earth Planet. Sci., 6, 1–37, https://doi.org/10.1186/s40645-018-0239-9, 2019.
Knorr, P. O., Robbins, L. L., Harries, P. J., Hallock, P., and Wynn, J.: Response of the miliolid Archaias angulatus to simulated ocean acidification, J. Foramin. Res., 45, 109–127, https://doi.org/10.2113/gsjfr.45.2.109, 2015.
Koehler-Rink, S. and Kuehl, M.: Microsensor studies of photosynthesis and respiration in larger symbiotic foraminifera. I The physico-chemical microenvironment of Marginopora vertebralis, Amphistegina lobifera and Amphisorus hemprichii, Mar. Biol., 137, 473–486, https://doi.org/10.1007/s002270000335, 2000.
Kroeker, K. J., Kordas, R. L., Crim, R., Hendriks, I. E., Ramajo, L., Singh, G. S., Duarte, C. M., and Gattuso, J. P.: Impacts of ocean acidification on marine organisms: quantifying sensitivities and interaction with warming, Glob. Change Biol., 19, 1884–1896, https://doi.org/10.1111/gcb.12179, 2013.
Kuroyanagi, A., Iriem T., Kinoshita, S., Kawahata, H., Suzuki, A., Nishi, H., Sasaki, O., Takashima, R., and Fujita, K.: Decrease in volume and density of foraminiferal shells with progressing ocean acidification, Sci. Rep., 11, 19988, https://doi.org/10.1038/s41598-021-99427-1, 2021.
Kwiatkowski, L., Torres, O., Bopp, L., Aumont, O., Chamberlain, M., Christian, J. R., Dunne, J. P., Gehlen, M., Ilyina, T., John, J. G., Lenton, A., Li, H., Lovenduski, N. S., Orr, J. C., Palmieri, J., Santana-Falcón, Y., Schwinger, J., Séférian, R., Stock, C. A., Tagliabue, A., Takano, Y., Tjiputra, J., Toyama, K., Tsujino, H., Watanabe, M., Yamamoto, A., Yool, A., and Ziehn, T.: Twenty-first century ocean warming, acidification, deoxygenation, and upper-ocean nutrient and primary production decline from CMIP6 model projections, Biogeosciences, 17, 3439–3470, https://doi.org/10.5194/bg-17-3439-2020, 2020.
Langer, M. R.: Assessing the contribution of foraminiferan protists to global ocean carbonate production, J. Eukaryotic Microbiol., 55, 163–169, https://doi.org/10.1111/j.1550-7408.2008.00321.x, 2008.
Langer, M. R., Lipps, J. H., Silk, M. T., and Lipps, J. H. Global ocean carbonate and carbon dioxide production: the role of reef foraminifera, J. Foramin. Res., 27, 271–277, https://doi.org/10.2113/gsjfr.27.4.271, 1997.
Lida, Y., Takatani, Y., Kojima, A., and Ishii, M.: Global trends of ocean CO2 sink and ocean acidification: an observationbased reconstruction of surface ocean inorganic carbon variables, J. Oceanogr., 77, 323–358, https://doi.org/10.1007/s10872-020-00571-5, 2021.
Lueker, T. J., Dickson, A. G., and Keeling, C. D.: Ocean pCO2 calculated from dissolved inorganic carbon, alkalinity, and equations for K1 and K2: validation based on laboratory measurements of CO2 in gas and seawater at equilibrium, Mar. Chem., 70, 105–119, https://doi.org/10.1016/S0304-4203(00)00022-0, 2000.
Lüthi, D., Le Floch, M., Bereiter, B., Blunier, T., Barnola, J. M., Siegenthaler, U., Raynaud, D., Jouzel, J., Fischer, H., Kawamura, K., and Stocker, T. F.: High-resolution carbon dioxide concentration record 650,000-800,000 years before present, Nature, 453, 379–382, https://doi.org/10.1038/nature06949, 2008.
Maiklem, W. R.: Black and brown speckled foraminiferal sand from the southern part of the Great Barrier Reef, J. Sediment. Res., 34, 1023–1030, https://doi.org/10.1306/74D71820-2B21-11D7-8648000102C1865D, 1967.
Martin, R. E.: Habitat and distribution of the foraminifer Archaias angulatus (Fichtel and Moll) (Miliolina, Soritidae), northern Florida Keys, J. Foramin. Res., 16, 3, 201–206, https://doi.org/10.2113/gsjfr.16.3.201, 1986.
Martinez, A., Hernández-Terrones, L., Rebolledo-Vieyra, M., and Paytan, A.: Impact of carbonate saturation on large Caribbean benthic foraminifera assemblages, Biogeosciences, 15, 6819–6832, https://doi.org/10.5194/bg-15-6819-2018, 2018.
Martinez, A., Crook, E. D., Barshis, D. J., Potts, D. C., Rebolledo-Vieyra, M., Hernandez, L., and Paytan, A.: Species-specific calcification response of Caribbean corals after 2-year transplantation to a low aragonite saturation submarine spring, Proc. R. Soc. B, 286, 20190572, https://doi.org/10.1098/rspb.2019.0572, 2019.
McIntyre-Wressnig, A., Bernhard, J., M., McCorkle, D., C., and Hallock, P.: Non-lethal effects of ocean acidification on the symbiont-bearing benthic foraminifer Amphistegina gibbosa, Mar. Ecol.-Prog. Ser., 472, 45–60, https://doi.org/10.3354/meps09918, 2013.
Milker, Y. and Schmiedl, G.: A taxonomic guide to modern benthic shelf foraminifera of the western Mediterranean Sea, Palaeontol. Electronica, 15, 1–134, https://doi.org/10.26879/271, 2012.
Moodley, L., Boschker, H. T. S., Middelburg, J. J., Pel, R., Herman, P. M. J., De Deckere, E., and Heip, C. H. R.: Ecological significance of benthic foraminifera: 13C Labelling experiments, Mar. Ecol. Prog. Ser., 202, 289–295, https://doi.org/10.3354/meps202289, 2000.
Murray, J. W.: Ecology and applications of benthic foraminifera. Cambridge University Press, Cambridge, New York, ISBN: 9780511535529, 2006.
Narayan, G. R., Reymond, C. E., Stuhr, M., Doo, S., Schmidt, C., Mann, T., and Westphal, H.: Response of large benthic foraminifera to climate and local changes: Implications for future carbonate production, Sedimentology, 69, 121–161, https://doi.org/10.1111/sed.12858, 2021.
Nehrke, G., Keul, N., Langer, G., De Nooijer, L. J., Bijma, J., and Meibom, A.: A new model for biomineralization and trace-element signatures of Foraminifera tests, Biogeosciences, 10, 6759–6767, https://doi.org/10.5194/bg-10-6759-2013, 2013.
Null, K. A., Knee, K. L., Crook, E. D., Sieyes, N. R., Rebolledo-Vieyra, M., Hernández-Terrones, L., and Paytan, A.: Composition and fluxes of submarine groundwater along the Caribbean coast of the Yucatan Peninsula, Cont. Shelf Res., 77, 38–50, https://doi.org/10.1016/j.csr.2014.01.011, 2014.
Penã, V., Harvey, B. P., Agostini, S., Porzio, L., Milazzo, M., Horta, P., Gall, L. L., and Hall-Spencer, J. M.: Major loss of coralline algal diversity in response to ocean acidification, Glob. Change Biol., 27, 4785–4798, https://doi.org/10.1111/gcb.15757, 2021.
Perry, E., Velazquez-Oliman, G., and Marin, L.: The hydrogeochemistry of the karst aquifer system of the northern Yucatan peninsula, Mexico, Int. Geol. Rev., 44, 191–221, https://doi.org/10.2747/0020-6814.44.3.191, 2002.
Peters, G. P., Andrew, R. M., Canadell, J. G., Friedlingstein, P., Jackson, R. B., Korsbakken, J. I., Le Quéré, C., and Peregon, A.: Carbon dioxide emissions continue to grow amidst slowly emerging climate policies, Nat. Clim. Change, 10, 3–6, https://doi.org/10.1038/s41558-019-0659-6, 2020.
Petit, J. R., J. Jouzel, Raynaud, D., Barnola, J. M., Basile, I., Bender, M., Chappellaz, J., Davis, M., Delaygue, G., Delmotte, M., Kotlyakov, V. M., Legrand, M., Lipenkov, V. Y., Lorius, C., Pépin, L., Ritz, C., Saltzman, E., and Stievenard, M: Climate and atmospheric history of the past 420,000 years from the Vostok ice core, Antarctica, Nature, 399, 429–436, https://doi.org/10.1038/20859, 1999.
Pettit, L. R., Hart, M. B., Medina-Sánchez, A. N., Smart, C. W., Rodolfo-Metalpa, R., Hall-Spencer, J. M., and Prol-Ledesma, R. M.: Benthic foraminifera show some resilience to ocean acidification in the northern Gulf of California, Mexico, Mar. Pollut. Bull., 73, 452–462, https://doi.org/10.1016/j.marpolbul.2013.02.011, 2013.
Pettit, L. R., Smart, C. W., Hart, M. B., Milazzo, M., and Hall-Spencer, J. M.: Seaweed fails to prevent ocean acidification impact on foraminifera along a shallow-water CO2 gradient, Ecol. Evol., 5, 1–10, https://doi.org/10.1002/ece3.1475, 2015.
Pierrot, D. E., Levis, E., and Wallace, D. W. R.: MS Excel Program Developed for CO2 System Calculations, Oak Ridge, TN: U.S. Department of Energy: Carbon Dioxide Information Analysis Center, Oak Ridge National Laboratory, https://doi.org/10.3334/CDIAC/otg.CO2SYS_XLS_CDIAC105a, 2006.
Prazeres, M., Uthicke, S., and Pandolfi, J. M.: Ocean acidification induces biochemical and morphological changes in the calcification process of large benthic foraminifera, Proc. R. Soc. B, 282, 20142782, https://doi.org/10.1098/rspb.2014.2782, 2015.
Prazeres, M., Martínez-Colón, M., and Hallock, P.: Foraminifera as bioindicators of water quality: The FoRAM indez revisited, Environ. Pollut., 257, 113612, https://doi.org/10.1016/j.envpol.2019.113612, 2020.
Price, N. N., Martz, T. R., Brainard, R. E., and Smith, J. E.: Diel variability in seawater pH relates to calcification and benthic community structure on coral reefs, PLoS ONE, 7, e4384, https://doi.org/10.1371/journal.pone.0043843, 2012.
R Core Team: R: A language and environment for statistical computing. R Foundation for Statistical Computing, Vienna, Austria, https://www.R-project.org/ (last access: 31 March 2022), 2020.
Sariaslan, N. and Langer, M. R.: Atypical, high-diversity assemblages of foraminifera in a mangrove estuary in northern Brazil, Biogeosciences, 18, 4073–4090, https://doi.org/10.5194/bg-18-4073-2021, 2021.
Schiebel, R.: Planktic foraminiferal sedimentation and the marine calcite budget, Global Biogeochem. Cy., 16, 3-1–3-21, https://doi.org/10.1029/2001GB001459, 2002.
Schmidt, C., Kucera, M., and Uthicke, S.: Combined effects of warming and ocean acidification on coral reef Foraminifera Marginopora vertebralis and Heterostegina depressa, Coral Reefs, 33, 805–818, https://doi.org/10.1007/s00338-014-1151-4, 2014.
Schneider, C. A., Rasband, W. S., and Eliceiri, K. W.: Nih Image to ImageJ: 25 years of image analysis, Nat. Methods, 9, 671–675, https://doi.org/10.1038/nmeth.2089, 2012.
Scott, D. B. and Medioli, F. S.: Living vc. Total foraminiferal populations: Their relative usefulness in paleoecology, J. Foraminiferal Res., 54, 814–831, http://www.jstor.org/stable/1304312 (last access: 17 June 2022), 1980.
Stephenson, C. M., Hallock, P., and Kelmo, F.: Foraminiferal assemblage indices: A comparison of sediment and reef rubble samples from Conch Reef, Florida, USA, Ecol. Indic., 48, 1–7, https://doi.org/10.1016/j.ecolind.2014.07.004, 2015.
Stuhr, M., Cameron, L. P., Blank-Landeshammer, B., Reymond, C. E., Doo, S. S., Westphal, H., Sickmann, A., and Ries, J. B.: Divergent proteomic responses offer insights into resistant physiological responses of a reef-foraminifera to climate change scenarios, Oceans, 2, 281–314, https://doi.org/10.3390/oceans2020017, 2021.
Toyofuku, T., Matsuo, M. Y., De Nooijer, L. J., Nagai, Y., Kawada, S., Fujita, K., Reichart, G. J., Nomaki, H., Tsuchiya, M., Sakaguchi, H., and Kitazato, H.: Proton pumping accompanies calcification in foraminifera, Nat. Commun., 8, 14145, https://doi.org/10.1038/ncomms14145, 2017.
Uppström, L. R.: The boron/chlorinity ratio of deep-sea water from the Pacific Ocean, Deep-Sea Res. Oceanogr. Abstr., 21, 161–162, 1974.
Uthicke, S. and Fabricius, K. E.: Productivity gains do not compensate for reduced calcification under near-future ocean acidification in the photosynthetic benthic foraminifer species Marginopora vertebralis, Glob. Change Biol., 18, 2781–2791, https://doi.org/10.1111/j.1365-2486.2012.02715.x, 2012.
Uthicke, S., Momigliano, P., and Fabricius, K. E.: High risk of extinction of benthic foraminifera in this century due to ocean acidification, Sci. Rep., 3, 1–5, https://doi.org/10.1038/srep01769, 2013.
Vogel, N. and Uthicke, S.: Calcification and photobiology in symbiont-bearing benthic foraminifera and responses to a high CO2 environment, J. Exp. Mar. Biol. Ecol, 424/425, 15–24, https://doi.org/10.1016/j.jembe.2012.05.008, 2012.
Wilson, B. and Wilson, J. I.: Shoreline foraminiferal thanatacoenoses around five eastern Caribbean islands and their environmental and biogeographic implications, Cont. Shelf Res., 31, 857–866, https://doi.org/10.1016/j.csr.2011.02.010, 2011.
WoRMS Editorial Board: World Register of Marine Species, VLIZ, https://doi.org/10.14284/170, 2022.
Yamamoto, S., Kayanne, H., Terai, M., Watanabe, A., Kato, K., Negishi, A., and Nozaki, K.: Threshold of carbonate saturation state determined by CO2 control experiment, Biogeosciences, 9, 1441–1450, https://doi.org/10.5194/bg-9-1441-2012, 2012.
Yamano, H., Miyajima, T., and Koike, I.: Importance of foraminifera for the formation and maintenance of a coral sand cay: Green Island, Australia, Coral Reefs, 19, 51–58, https://doi.org/10.1007/s003380050226, 2000.
Yordanova, E. K. and Hohenegger, W.: Taphonomy of Larger Foraminifera: Relationships between Living Individuals and Empty Tests on Flat Reef Slopes (Sesoko Island, Japan), FACIES, 46, 169–204, https://doi.org/10.1007/BF02668080, 2002.