the Creative Commons Attribution 4.0 License.
the Creative Commons Attribution 4.0 License.
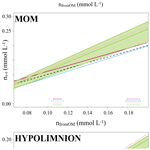
Mineralization of autochthonous particulate organic carbon is a fast channel of organic matter turnover in Germany's largest drinking water reservoir
Michael Seewald
Karsten Rinke
Kurt Friese
Robert van Geldern
Jakob Schmidmeier
Johannes A. C. Barth
Turnover of organic matter (OM) is an essential ecological function in inland water bodies and relevant for water quality. This is especially important for the potential of dissolved organic carbon (DOC) removal as well as for emissions of CO2. In this study, we investigated various phases of OM including DOC, autochthonous particulate organic carbon (auto-POC), allochthonous particulate organic carbon (allo-POC), and sedimentary matter (SED) in a temperate drinking water reservoir (Rappbode Reservoir, Germany) by means of dissolved inorganic carbon (DIC) concentrations and carbon stable isotope ratios. In order to best outline carbon turnover, we focused on the metalimnion and the hypolimnion of the reservoir, where respiration is expected to be dominant and hardly disturbed by atmospheric exchange or photosynthesis. DIC concentrations ranged between 0.30 and 0.53 mmol L−1, while δ13CDIC values ranged between −15.1 ‰ and −7.2 ‰ versus the VPDB (Vienna PeeDee Belemnite) standard. Values of δ13CDOC and δ13Cauto-POC ranged between −28.8 ‰ and −27.6 ‰ and between −35.2 ‰ and −26.8 ‰, respectively. Isotope compositions of sedimentary material and allochthonous POC were inferred from the literature and from measurements from previous studies with δ13C ‰ and δ13Callo-POC ranging from −31.8 ‰ to −28.6 ‰. Comparison of DIC concentration gains and stable isotope mass balances showed that auto-POC from primary producers was the main contributor to increases in the DIC pool. Calculated OM turnover rates (0.01 to 1.3 µmol L−1 d−1) were within the range for oligotrophic water bodies. Some higher values in the metalimnion are likely due to increased availability of settling auto-POC from the photic zone. Samples from a metalimnetic oxygen minimum (MOM) also showed dominance of respiration over photosynthesis. Our work shows that respiration in temperate lentic water bodies largely depends on auto-POC production as a major carbon source. Such dependencies can influence the vulnerabilities of these aqueous systems.
- Article
(5300 KB) -
Supplement
(577 KB) - BibTeX
- EndNote
Among the carbon phases in terrestrial surface waters, dissolved inorganic carbon (DIC) is usually most abundant, with concentrations that range from 0.1 mmol L−1 to more than 1.0 mmol L−1 (Cole and Praire, 2014). This wide range of concentrations also links to metabolic processes because DIC is at the same time a reagent for photosynthesis and a product of respiration. Respiration rates vary with temperature, organic matter load and nutrient availability, and phytoplankton assemblages (Gattuso et al., 2002; Hanson et al., 2003; Pace and Prairie, 2005; Wu and Chen, 2011; Mazuecos et al., 2015). A fundamental contribution to DIC budgets in water systems stems from atmospheric and soil CO2. For instance, atmospheric CO2 influences DIC concentrations through the entire water column of lentic bodies during the mixing period, while it mainly affects the epilimnion during stratification periods. Because of its high solubility in water, this gas plays a major role in the shallower parts of lentic aquatic ecosystems from which it either degasses (in the case of heterotrophy) or becomes dissolved from the atmosphere (autotrophy) into the surface layer (Wetzel, 1983; Cole et al., 2007; Tranvik et al., 2009; Raymond et al., 2013; Koschorreck et al., 2017; DelSontro et al., 2018). On the other hand, carbon contributions from organic matter (OM) to DIC usually become important at greater water depths. Such respiratory turnover is particularly important for the hypolimnion of eutrophic lakes and reservoirs, where a substantial increase in total inorganic carbon (ΣCO2) can often be observed (Wetzel, 1983). This feature is far less marked in oligotrophic lentic water bodies.
Other carbon phases in water are represented by OM. Organic carbon compounds result from decomposition processes of dead organic matter within (i.e., autochthonous) or outside (i.e., allochthonous) a water body (Kritzberg et al., 2004). Almost all autochthonous material in the pelagic zone of a water body consists of dead particulate organic carbon (auto-POC) and, to a smaller extent, dissolved organic carbon (DOC) by leaching (Wetzel, 1984). Only a small fraction of the auto-POC accounts for living biota (Kawasaki and Benner, 2006). Allochthonous OM is primarily of terrestrial plant origin and is transported to lentic systems by runoff as dissolved or particulate phase (allo-DOC and allo-POC). To a minor extent, allo-DOC and allo-POC phases can also derive from atmospheric inputs such as transport of dust by wind (Willey et al., 2000). Residual autochthonous and allochthonous carbon that reaches the bottom of a lentic water body can accumulate and generate sedimentary organic carbon (SED). This carbon burial in sediments is one of the main mechanisms of carbon sequestration within water bodies (Regnier et al., 2013).
DOC and POC in lentic waters are of primary importance in terms of water quality. DOC absorbs light and may inhibit photosynthesis (Karlsson et al., 2009). This affects dissolved oxygen (DO) and DIC dynamics (Blough, 2001; Schindler, 2004). In many cases, DOC can also cause browning of water that is associated with numerous economic disadvantages. In water used for drinking water supply, elevated DOC contents pose not only aesthetic concerns. For instance, DOC reactions with chlorine during water treatment disinfection can produce numerous harmful by-products (Karst et al., 2004; Bond et al., 2014; Fisher et al., 2017). Therefore, the evaluation of OM turnover, especially with respect to DOC, is of critical importance for water quality management of surface water used for drinking water production. On the other hand, the rate of POC turnover may also affect sedimentation rates and therefore control the amounts of carbon deposited in sediments (Azam et al., 1983; Keaveney et al., 2020). Such carbon accumulation may then lead to excessive losses of methane from lentic water bodies, which is relevant for greenhouse gas production (Bastviken et al., 2010; Huang et al., 2019; Jansen et al., 2022).
In addition, mineralization of OM into DIC has implications for greenhouse gas dynamics as it releases CO2 into the water, a process that also increases DIC (Sun et al., 2016). In lakes that may also be affected by inorganic carbon sequestration processes such as photosynthesis or calcite precipitation (Khan et al., 2022), increases in DIC concentrations may lead to CO2 degassing from the water column. From this perspective, lentic water bodies are not only reactors for carbon turnover but also sources of CO2 (Åberg et al., 2004; Cole et al, 2007). This highlights the need for aqueous studies with closer investigation of OM contributions to the DIC pool.
Carbon turnover in aqueous systems can be investigated by means of concentrations and carbon stable isotopes (δ13C) of DIC, DOC, and POC (MacKenzie et al., 2004; Schulte et al., 2011; van Geldern et al., 2015; Piatka et al., 2022). A common scheme is that respiration causes decreases in δ13CDIC that are associated with increases in DIC concentrations in water (Stiller and Nissenbaum, 1999; Gammons et al., 2014). Such δ13CDIC decreases relate to the fact that processed OM is usually enriched in 12C and therefore has more negative δ13C values when compared to its inorganic counterparts. During OM degradation, this more negative isotope signal becomes transferred to the DIC pool. Therefore, mass balances that combine carbon concentration data with isotopes may serve as useful source tracers in studies of aqueous carbon turnover (Barth et al., 2017).
In this study, we investigated the turnover of OM into DIC with data from the Rappbode Reservoir, which is Germany's largest drinking water reservoir. Our principal aim was to constrain preferential turnover of the various sources of OM. A further objective was to define turnover rates and to outline in which compartments of the reservoir they might be most pronounced. We also applied this approach to a metalimnetic oxygen minimum (MOM) in the reservoir. Here carbon turnover may be even more pronounced, as MOM is known for its strong depletion in dissolved oxygen (Shapiro, 1960; Nix, 1981; Kreling et al., 2017; Dordoni et al., 2022). The Rappbode Reservoir offered an ideal location for this study because in recent years its logistic settings helped to develop it into an increasingly acknowledged large-scale laboratory of ecological behavior for man-made lentic water bodies (Kong et al., 2019; Wentzky et al., 2019; Herzsprung et al., 2020; Mi et al., 2020).
The Rappbode Reservoir in the Harz Mountains has a surface area of 3.9 km2 and a maximum depth up to 90 m. Its maximum volume can reach up to 0.113 km3. The reservoir also produces hydropower (Rinke et al., 2013). It receives water from two streams, the Hassel and the Rappbode. Two pre-reservoirs with a total volume <0.002 km3 are located on the path of these two rivers and are described in detail by Friese et al. (2014). Another input to the Rappbode Reservoir is an artificial water transfer gallery from the Königshütte Reservoir. The mixing season at the Rappbode Reservoir normally occurs between the beginning of December and the middle of March. Complete stratification is visible from the end of May, and boundaries between the epilimnion, metalimnion, and hypolimnion usually persist until middle November (see Supplement S1, Fig. S1). During the stratification period, the metalimnion and the hypolimnion act as closed systems and evolve independently from other seasons. For example, dissolved oxygen (DO) can hardly be exchanged between the different parts of the reservoir, and its concentrations are higher in the epilimnion due to biota developments in spring and summer and related photosynthetic production of O2 (Wentzky et al., 2019). On the other hand, respiration dominates the metalimnion and hypolimnion of the reservoir to different degrees (Dordoni et al., 2022).
Sampling campaigns at the Rappbode Reservoir were carried out between March 2020 and December 2020 at a central location of the water body (51∘44′19′′ N, 10∘53′30′′ E, 420 m a.s.l.; Fig. 1). This monitoring station is located at a distance of 515 m from the main dam and is the best representative point of the reservoir for processes in the water column (Wentzky et al., 2019; Mi et al., 2020; Dordoni et al., 2022). Sampling campaigns took place at least every 2 weeks. For this work, we investigated depths at 13, 16, 22, 40, and 65 m below the water surface. Samples were collected with a LIMNOS Water Sampler™ (HYDRO-BIOS) in 1 L airtight amber–glass borosilicate bottles (DURAN™). They were prepared for laboratory analyses within 1 h after sampling.
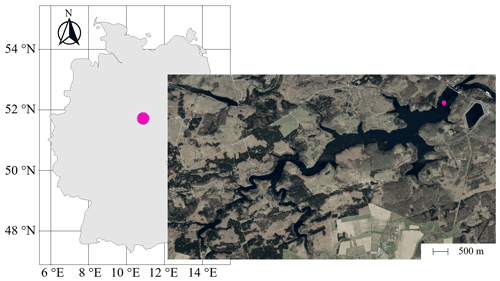
Figure 1Position of the Rappbode Reservoir in Germany with the sampling location marked by a pink point in the main reservoir (source: ESRI).
In the field, measurements of temperature and pH were performed on each depth-specific sample by a digital multi-parameter instrument (HQ40d; Hach Company, Loveland, CO, USA). Water samples for dissolved inorganic carbon (DIC) and dissolved organic carbon (DOC) were filtered through 0.45 µm pore-size syringe disk filters (Minisart High Flow PES, Sartorius AG, Germany) into 40 mL amber–glass vials that were closed airtight by butyl rubber septa. These vessels conform to US Environmental Protection Agency (EPA) standards and were pre-poisoned with 20 µL of a supersaturated mercuric chloride (HgCl2) solution to avoid secondary microbial activities after sampling. Duplicate samples were stored in the dark at 4 ∘C.
In the laboratory, water samples were analyzed for carbon stable isotopes of DIC (δ13CDIC) and DOC (δ13CDOC) by an Aurora 1030W TIC–TOC analyzer (OI Analytical, College Station, Texas) that was coupled in continuous flow mode to a Thermo Scientific Delta V plus isotope ratio mass spectrometer (IRMS). Concentrations were determined from the signal of the OI internal non-dispersive infrared sensor (NDIR) and a set of calibration standards. All stable isotope values in our work are reported as δ notations in per mill (‰) versus the standard reference for carbon isotopes (Vienna Pee Dee Belemnite – VPDB) according to
where R is the molar ratio of the heavy and light carbon isotopes. Standard deviations of both δ13CDIC and δ13CDOC measurements were better than ±0.3 ‰ (1σ).
Particulate organic carbon (auto-POC) from water samples was collected on pre-heated (400 ∘C) glass fiber filters (GFFs) with a pore size of 0.4 µm (Macherey Nagel GF-5). The GFFs were freeze-dried and subsequently pulverized using a ball mill (Retsch CryoMill). The resulting powder was fumigated by concentrated HCl in a desiccator for 24 h to eliminate possible carbonate particles on the filters. Afterwards, the sample was stored for 1 h at 50 ∘C to allow degassing of the remaining acid fumes. Ground and decalcified GFF sample material was then weighed into tin capsules for isotope analyses. Samples were analyzed for their δ13Cauto-POC signals using a Costech Elemental Analyzer (ECS 4010; Costech International, Pioltello, Italy; now NC Technologies, Bussero, Italy) in continuous flow mode coupled to a Thermo Scientific Delta V plus isotope ratio mass spectrometer (Thermo Fisher Scientific, Bremen, Germany). The standard deviation of these measurements was better than ± 0.3 ‰ (1σ).
The vertical division of the water column into the compartments (epilimnion, metalimnion, and hypolimnion) was arranged according to temperature data (Supplement S1, Fig. S1).
The mass balance for carbon turnover calculations works best in environments where respiration is the dominant process. This is because in the epilimnion the δ13CDIC signal is also influenced by photosynthesis and by degassing of CO2 to the atmosphere. Both processes shift the δ13CDIC values towards more positive values in patterns that are difficult to predict and depend on the type of algae community and magnitudes of CO2 degassing. These uncertainties render a closed mass balance approach difficult for the epilimnion (Barth et al., 1998; van Geldern et al., 2015). Therefore, we restricted mass balance calculations for OM turnover to the metalimnion and the hypolimnion. The isotope mass balance equation relies on the determination of molar contributions from OM to DIC pool as follows:
where nfromOM is the molar C contribution of OM to the DIC pool, nt is the molar concentration of the DIC at the time when the water column was homogenized by the lake turnover at the beginning of the year (time 0, i.e., 17 March 2020), δ13Ct is its corresponding carbon isotope composition at time 0, δ13Cs is the isotope composition of DIC of any sampling event later than time 0, and δ13COM is the isotope composition of the considered OM source material that may have been turned into DIC.
Equation (2) is a re-arrangement of the following mass balance:
Here δ13Cs refers to isotope compositions at any given sampling after time 0, the subscript “t” refers to time 0 concentration and isotope values, and the subscript “OM” refers to organic matter sources (auto-POC, DOC, SED, or allo-POC).
Further details regarding Eq. (2) are provided in the Supplement S1.
One requirement for using this method is the increase in DIC from time 0 to each subsequent sampling event.
The organic matter (OM) sources studied were DOC, auto-POC, SED, and particulate organic carbon of allochthonous origin (allo-POC). Their individual carbon isotope compositions (δ13COM) were the variable that was replaced for each sample calculation in Eq. (2). We used field data for all the variables of the equation, except for δ13CSED and δ13Callo-POC. For δ13CSED, we used data with an average of −31.1 ‰ (Liu et al., 2022). For δ13Callo-POC, we used a range of values between −31.8 ‰ and −28.6 ‰ that were retrieved from the literature (Tittel et al., 2019) and from 18 unpublished data points that were collected upstream from entering rivers (Supplement S2). These data were provided by the Helmholtz Zentrum für Umweltforschung in Magdeburg and collected in 2020. Note that we were not able to sample sedimentary and river input data at the same frequency as the water samples from the reservoir. However, because they are likely less variable we think that they are transferable to our study as averages.
We also tested the validity of Eq. (2) via an error propagation approach (Ku, 1966; Kretz, 1985):
where S is the total standard variation and s refers to the standard variation of each sampling date. More information about this method is available in the Supplement S1.
The evaluation of OM turnover into the DIC pool was limited to the metalimnion and the hypolimnion of the Rappbode Reservoir because only in these zones did we obtain a good correlation between DIC and its isotopes (Supplement S1, Fig. S2). For comparison to the isotope mass balance (Eq. 2) we also determined DIC gains with concentration differences from time 0 (ns−t, i.e., 17 March 2020). Here we aimed to find out which correlations would be closest to a 1:1 line in order to narrow down the most plausible source of OM turnover.
We also calculated OM to DIC turnover rates in the metalimnion and in the hypolimnion. For considerations of the metalimnion, we additionally separated the layer showing the minimal oxygen concentration (metalimnetic oxygen minimum, MOM). Our results are expressed in µmol L−1 d−1 for individual sampling dates with their time differences between time 0 (17 March 2020) up to a maximum of 259 d (i.e., 8 December 2020). In order to evaluate OM seasonal turnover rates, we subdivided our database following Wang et al. (2021). According to this scheme, spring ranged from 17 February to 11 June, summer from 12 June to 14 September, autumn from 15 September to 5 December, and winter from 6 December to 16 February.
3.1 Dissolved inorganic carbon concentrations
Figure 2 shows DIC concentration profiles of the studied time period divided into seasons. DIC concentrations generally increased from time 0 (17 March 2020) over the course of the study period. The spring profiles showed the smallest range of DIC concentrations (0.29 to 0.36 mmol L−1) and an overall homogeneity throughout the water column (Fig. 2a). This is in accordance with biogeochemical uniformity during and shortly after mixing periods of lentic water bodies (Wetzel, 1984). Also, all DIC contents at this starting period were lower when compared to subsequent samples. These low DIC values related to equilibration with the atmosphere that spread over the entire depth due to lake turnover. In addition, metabolic processes were still low in March because of lower temperatures. One exception was a decrease in DIC at 16 m depth on 28 April 2020. This may have been related to some residual photosynthetic DIC uptake or upwelling of DIC-depleted waters from greater depths.
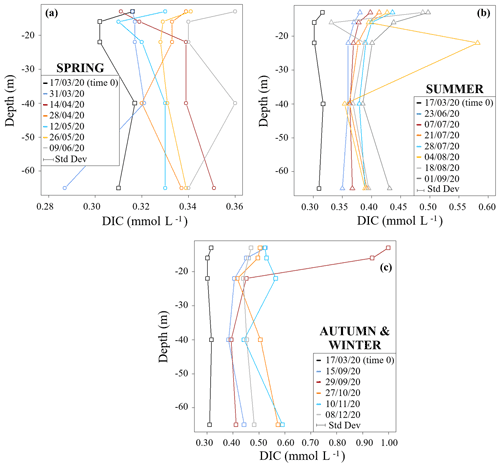
Figure 2DIC seasonal concentration profiles for (a) spring, (b) summer, and (c) autumn and winter. Standard deviations relative to each dataset (± 3 %) are reported in the plot below the legend.
Concentration differences between the different depth profiles were smallest in summer. However, they showed a pronounced increase in their DIC concentrations when compared to the springtime profiles. During this time period DIC concentrations ranged from 0.33 to 0.58 mmol L−1 (Fig. 2b). A sample from 22 m depth on 4 August 2020 had an exceptionally high DIC content. This may either be a wrong measurement or indicates a temporary zone of high respiration.
Samples from autumn and winter had the highest DIC concentrations with values between 0.38 and 0.59 mmol L−1 (Fig. 2c). In the sampling event of 29 September 2020, samples from 13 and 16 m depth showed the highest DIC concentrations with values of 1.00 and 0.94 mmol L−1, respectively (Fig. 2c). Again, this indicates a zone of high respiratory turnover.
Overall, continuous increases in DIC below the photic zone in the metalimnion and in the hypolimnion are most plausibly due to respiration and associated turnover of OM. These effects of respiration can best be shown in the hypolimnion, where the counteracting effects of photosynthesis and CO2 degassing (processes that both reduce DIC contents) are minimal.
DIC concentration increases near the bottom of the lake (65 m) may have been related to further contributions from processes involving organic turnover within the sediments (Wetzel, 1984). On the other hand, higher DIC concentrations found in the metalimnion are related to respiration processes that likely link to OM inputs from the overlying epilimnion.
Note that the MOM samples had the highest DIC concentrations, with values up to 1 mmol L−1. This matches the findings by Giling et al. (2017), who also found that the metalimnion may act as a metabolic hotspot in oligotrophic water bodies. Additionally, MOM samples also had the highest auto-POC and DOC concentrations (see Supplement S2). Such high OM contents commonly mark zones close to phytoplankton assemblages (Wetzel et al., 1972). They may indicate the collapse of an algae bloom, when subsequently settling detritus becomes directly subject to decomposition and accelerates respiratory turnover. This hypothesis is supported by our data that show predominant autochthonous POC consumption. Temperature-related viscosity changes that are typical of the metalimnion may also have enhanced the residence time of organic matter and therewith may have accelerated respiration. Overall, these findings suggest a link between the MOM and a productive biotic assemblage in vertical proximity above the MOM. This connection is also supported by data from the literature (Kreling et al., 2017; McDonald et al., 2022). Moreover, a strong respiratory turnover of OM was also highlighted independently by previous studies with dissolved oxygen isotopes on the Rappbode Reservoir (Dordoni et al., 2022).
3.2 Carbon stable isotopes of organic matter and its mineralization
3.2.1 Epilimnion
It was not possible to evaluate OM contributions to the DIC pool for the epilimnion. This is because no reasonable correlation between DIC concentrations and its isotopes was found in this compartment (Supplement S1, Fig. S2). This result was expected due to influences of CO2 degassing to the atmosphere and due to photosynthesis. Both of these processes generate more positive δ13CDIC with overlapping isotope effects that are difficult to account for (Ahad et al., 2008; Wachniew, 2006; Gammons et al., 2011). Therefore, OM turnover calculations could not be corrected for these counteracting effects. Nonetheless, it is likely that OM turnover rates in the epilimnion are at least as intense as in the metalimnion and in the hypolimnion because the photic zone directly offers fresh autochthonous POC material for turnover. In some studies respiration was shown to exceed photosynthesis in the epilimnion of oligotrophic water bodies (del Giorgio and Peters, 1993; del Giorgio et al., 1997; Duarte and Agustí, 1998),
3.2.2 Metalimnion, MOM, and hypolimnion
Variations of δ13CDOC during our observation period were small and ranged from −28.8 ‰ to −27.1 ‰ (Fig. 3). Instead, changes in δ13Cauto-POC followed the development of stratification. Here the mixing period was characterized by lower values (down to −35.2 ‰ in the upper part of the water column). On the other hand, we also found δ13Cauto-POC values that reached above −24.0 ‰ at around 16 m depth at the end of August 2020 (Fig. 3). This range of values likely indicates developments of different species of biota in the water column with different δ13Cauto-POC. In the MOM, a species that likely drives the carbon cycle is the cyanobacterium Planktothrix rubescens. This type of cyanobacterium finds an optimum at metalimnetic depths (Nürnberg et al., 2003; Gisriel et al., 2020). Its blooms in the metalimnion of the Rappbode Reservoir from late summer to autumn have been reported before (Wentzky et al., 2019).
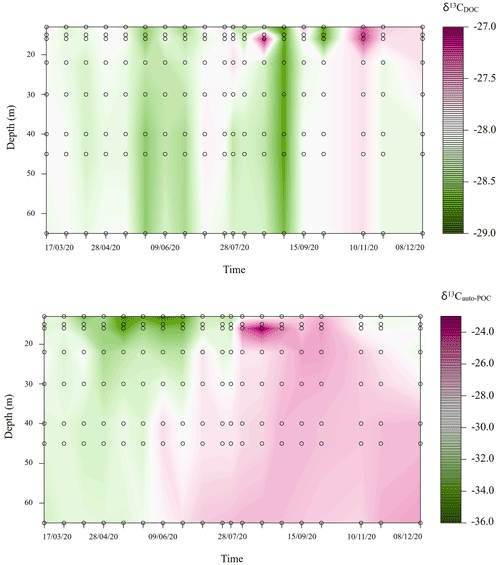
Figure 3Carbon stable isotope variations (‰) of dissolved organic carbon (DOC) and autochthonous particulate organic carbon (auto-POC) during the observation period in the metalimnion and hypolimnion of Rappbode Reservoir.
In order to evaluate OM contributions to the DIC pool, we determined DIC gains with concentration differences from time 0 (ns−t) and compared them to DIC concentration gains as calculated by the isotope mass balance (Eq. 2 and Fig. 4). With this analysis, results with input from auto-POC showed the best linear regression that was closest to a 1:1 line (Table 1). This was most obvious when only the hypolimnion was considered, with an angular coefficient of the regression line for auto-POC of 1.00. Note that the green zone in Fig. 4 marks the minimum and maximum δ13C values of the allo-POC according to data that come from Rappbode catchment. This shows that in the metalimnion and in the MOM a clear differentiation between allo- and auto-POC was not possible. Despite this fact, it is still likely that in the metalimnion auto-POC is also the main contributor to DIC increases. This is supported by the clear signal from the hypolimnion. Moreover, the metalimnion is the zone that receives most of the freshly produced OM that mostly consists of auto-POC. Except for samples at the bottom of the reservoir, it is also unlikely that sedimentary OM influenced DIC increases in the entire water column above. Note that calculation-related uncertainties of this type of OM input may be higher because we used average δ13C input values of SED and allo-POC from the literature to constrain their contribution to the DIC pool. This is because neither sedimentary material nor allo-POC was sampled in a fortnightly interval over the observation period.
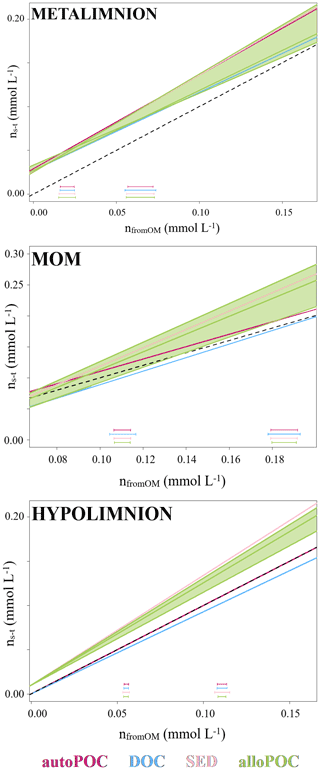
Figure 4Molar gain by concentration from time 0 (ns−t) and as calculated by mass balance with carbon stable isotopes (nfromOM) in the metalimnion, MOM, and hypolimnion of the Rappbode Reservoir. Datasets are reported in different colors according to the considered OM. Green fields represent the variation of allo-POC. Standard variations for each OM are reported at the bottom of the plot.
Table 1Equations of the regression lines for autochthonous particulate organic carbon (auto-POC), dissolved organic carbon (DOC), sedimentary material (SED), and allochthonous particulate organic carbon (allo-POC) in the metalimnion, MOM, and hypolimnion of the Rappbode Reservoir. The coefficient of determination (R2) is reported below each equation. All p values < 0.001.
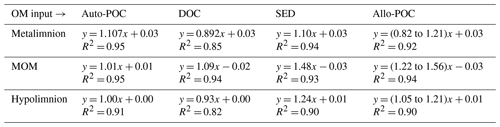
One reason why allochthonous-related OM does not seem to contribute significantly to the DIC pool in the reservoir might be that its mass is insufficient to exert enough impact on the entire carbon budget. This suggests either low inputs from the tributaries and the catchment or low turnover of this carbon phase within the reservoir. The latter option is more plausible as POC concentrations were often higher than expected by autochthonous POC concentrations and reached values of up to 1.05 mg L−1 (Supplement S2). In addition, the averages of δ13Callo-POC and δ13CSED are very close with values of −30.6 ‰ and −31.1 ‰. This isotope proximity of both OM pools is another indication that most of the allo-POC reaches the sedimentary pool with little or no further processing.
DOC may also have had secondary influences on DIC increases. This is obvious from the regression lines in Fig. 4. Although studies on rivers have proven that DOC can fuel metabolic processes in peat-dominated heterotrophic ecosystems (Thurman, 1985; Billett et al., 2010), the DOC pool in lentic oligotrophic water bodies consists primarily of carbon compounds that are older and more resistant to bacterial decomposition (Wetzel, 1984). As a result, bacteria preferentially consume autochthonous POC that consists mainly of fresh material (Cole et al., 1984; Barth et al., 2017). Note, however, that if part of the DOC pool found in the Rappbode Reservoir resulted from leaching of auto-POC, it should have the same isotope composition as the original POC material itself. If this part of the DOC pool becomes turned over into DIC by respiration, we would not be able to differentiate from a direct auto-POC input.
3.3 Turnover rates
DIC production rates as estimated from auto-POC turnover as the dominant OM input in the water column of the Rappbode Reservoir are reported in Fig. 5. They ranged from 0.1 to 1.3 µmol L−1 d−1 and were comparable to those found in other oligotrophic water bodies (Cole et al., 1984; Scavia and Laird, 1987; Carignan et al., 2000). Therefore, our results seem to successfully approximate the expected carbon processing of the aquatic environment and could be used as an alternative approach to quantify turnover rates of OM without the use of in situ incubation experiments.
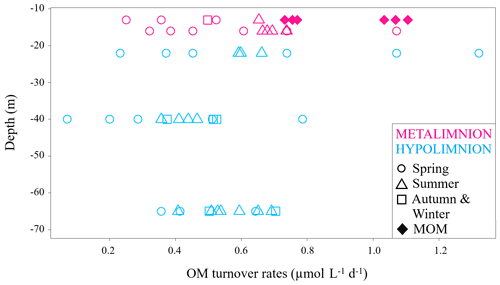
Figure 5DIC production rates at specific depths of the hypolimnion and the metalimnion during the study period, marked with different symbols according to their sampling season. MOM samples only belong to summertime and are displayed as diamond shapes.
Data from the metalimnion and the upper part of the hypolimnion showed higher turnover rates of up to 1.3 µmol L−1 d−1. The metalimnion showed fewer variations than the hypolimnion and ranged from 0.3 to 1.1 µmol L−1 d−1. Again, the highest DIC production rates were found within the MOM samples. This observation matches the fact that oxygen depletion is most intense in this layer (Dordoni et al., 2022). DIC production rates for the hypolimnion ranged from 0.1 to 1.3 µmol L−1 d−1, with the highest variance at a water depth of 22 m (0.2 to 1.3 µmol L−1 d−1). Generally higher rates of auto-POC turnover above 23 m depth may have been caused by higher availabilities of fresh auto-POC produced by photosynthesis within the photic zone. This also indicates that OM turnover in this part of the water column depends largely on freshly produced auto-POC material that sinks downward and decomposes more rapidly due to high oxygen availability (Pace and Prairie, 2005). This observation agrees with the appearance of epilimnetic diatom blooms in early spring and with phytoplankton blooms in the metalimnion of the Rappbode Reservoir from summer to early autumn (Wentzky et al., 2019).
Samples from 40 m depth had the lowest DIC productivity with values below 0.2 µmol L−1 d−1. An explanation for such low rates may be that OM has already been reduced in the water layers above this depth. Moreover, below the metalimnion the rate of respiratory carbon turnover depends primarily on the residence time of OM (Robarts, 1986). In support of this explanation, summer and autumn turnover rates of our study are higher than the spring ones, particularly at this depth (Fig. 5).
With one exception at 40 m depth, data from 65 m depth showed higher rates but covered a narrower range of variation (0.5 to 0.7 µmol L−1 d−1). Increased turnover rates for this depth may appear to be a contradiction. However, such values likely result from detritus decomposition with carbon contributions from the sediments.
Turnover rates from springtime had the highest spread of auto-POC turnover for each depth with only one exception at 13 m depth. At this depth, MOM waters exceeded the springtime range of variation. Samples from summer and autumn showed higher turnover rates than those of spring. Overall, the highest carbon turnover rates into DIC were found in springtime and during summer, when the MOM developed. The reason why spring turnover values are generally lower may relate to combined effects of temperature, residence time, and nature of decomposing detritus. During springtime, most of the organic matter derived from diatom blooms is sequestered within the epilimnion (Sommer et al., 1986). Therefore, the amount of detritus that can reach greater depths to become mineralized is lower. Additionally, heavy silica shells of diatoms decrease their residence time in the water column.
Unlike the rest of the metalimnion, MOM samples hardly seem to be influenced by photosynthesis and show a clear predominance of respiration. In this zone auto-POC was also determined to be the main contributor to the DIC pool with turnover rates that were highest for summertime and among the highest in the whole database (Fig. 5). Once again, this result suggests close relationships between metabolism of the autochthonous phytoplankton community and its decay with subsequent emergence of the MOM (Shapiro, 1960). This finding also supports interpretations by Kreling et al. (2017) and McDonald et al. (2022). These studies observed downward fluxes of POC that promote the development of MOM zones. Overall, our study agrees with preliminary studies on the pre-reservoir dams in the Rappbode system (Barth et al., 2017). This suggests that similar OM turnover principles apply despite volume differences between the main water body and its pre-reservoirs.
Comparisons between DIC concentration differences and stable carbon isotope mass balances with different OM inputs in an oligotrophic drinking water reservoir demonstrated dominant turnover of freshly produced auto-POC. On the other hand, DOC turnover seemed negligible, unless part of this pool was generated by leaching from the auto-POC pool. This sort of DOC would be isotopically identical to its precursor POC material. Nonetheless, if DOC in reservoirs is deemed a problem for water quality, it is unlikely to be solved by respiratory turnover into DIC.
Our results confirm that calculated rates of auto-POC turnover into DIC by respiration are typical of oligotrophic water bodies. This shows that assessments of such turnover with our isotope methods seem possible without the use of in situ incubation experiments. Therefore, our approach may provide a promising alternative to such complex experiments. However, such findings based on isotope mass balances should be tested in parallel with data from incubations to evaluate and narrow down uncertainties.
Our study reveals the environmental fragility of the Rappbode Reservoir. For instance, if large amounts of carbon were added to the system, only part of this load could be transferred into the DIC pool. Such a scenario could occur with excessive algae blooms under higher nutrient loads or increased mobilization of external OM under different land use and climate conditions with more heavy rain events. In such scenarios, the current in situ respiration would likely be unable to cope with excessive C loads. This in turn would lead to higher OM sedimentation rates and water browning. Likely, such modifications of metabolic balances would also compromise the water quality by overuse of dissolved oxygen without adequate replenishment. Overall, a comparison between DIC production rates with DO depletion as described by Dordoni et al. (2022) should be evaluated in order to prove the consistency of both isotope approaches. Such investigations should be the subject of future studies.
The above considerations are transferable to other temperate lentic systems where links between auto-POC turnover and associated DIC gains may operate under similar conditions but at different rates. Our results may help to improve water management strategies to help foster economic and environmental management of drinking water reservoirs. Nonetheless, this study only treated one single point with detailed depth profiles at high frequency. We therefore suggest that future studies should also investigate spatial and lateral heterogeneities.
Data are available in the Supplement S2.
The supplement related to this article is available online at: https://doi.org/10.5194/bg-19-5343-2022-supplement.
MD performed the formal analysis and was responsible for investigation, data curation, and visualization. MD and JACB cured the conceptualization, methodology, validation, resources, and writing of the original draft. MS, KR, and RvG oversaw the article review and editing. KR and JACB were responsible for project administration and funding acquisition. JACB provided constant supervision.
The contact author has declared that none of the authors has any competing interests.
Publisher’s note: Copernicus Publications remains neutral with regard to jurisdictional claims in published maps and institutional affiliations.
The authors would like to thank Karsten Rahn, Michael Herzog, Martin Wieprecht, Silke Meyer, Luisa Daxeder, and Lucas Heiß for assistance in the field during sampling, as well as Christian Hanke and Anja Schuster for their commitment to laboratory analyses. We are grateful to the “Talsperrenbetrieb Sachsen-Anhalt” (TSB) for their support and permissions.
This research has been supported by the Deutsche Forschungsgemeinschaft (grant nos. BA 2207/18-1 and RI 2040/4-1).
This paper was edited by Sebastian Naeher and reviewed by two anonymous referees.
Åberg, J., Bergström, A.-K., Algesten, G., Söderbackj, K., and Jansson, M.: A comparison of the carbon balances of a natural lake (L. Örträsket) and a hydroelectric reservoir (L. Skinnmuddselet) in northern Sweden, Water Res., 38, 531–538, https://doi.org/10.1016/j.watres.2003.10.035, 2004.
Ahad, J. M. E., Barth, J. A. C., Ganeshram, R. S., Spencer, R. G. M., and Uher, G.: Controls on carbon cycling in two contrasting temperate zone estuaries: The Tyne and Tweed, UK, Estuar. Coast. Shelf Sci., 78, 685–693, https://doi.org/10.1016/j.ecss.2008.02.006, 2008.
Azam, F., Fenchel, T., Field, J. G., Gray, J. S., Meyer-Reil, L. A., and Thingstad, F.: The Ecological Role of Water-Column Microbes in the Sea, Mar. Ecol. Prog. Ser., 10, 257–263, https://doi.org/10.3354/meps010257, 1983.
Barth, J. A. C., Mader, M., Nenning, F., van Geldern, R., and Friese, K.: Stable isotopes mass balances versus concentration differences of dissolved inorganic carbon – implications for tracing carbon turnover in reservoirs, Isot. Environ. Health Stud., 53, 413–426, https://doi.org/10.1080/10256016.2017.1282478, 2017.
Barth, J. A. C., Veizer, J., and Mayer, B.: Origin of particulate organic carbon in the upper St. Lawrence: Isotopic constraints, Earth Planet. Sc. Lett., 162, 111–121, https://doi.org/10.1016/S0012-821X(98)00160-5, 1998.
Bastviken, D., Santoro, A. L., Marotta, H., Queiroz Pinho, L., Fernandes Calheiros, D., Crill, P., and Enrich-Prast, A.: Methane Emissions from Pantanal, South America, during the Low Water Season: Toward More Comprehensive Sampling, Environ. Sci. Technol., 44, 5450–5455, https://doi.org/10.1021/es1005048, 2010.
Billett, M. F., Charman, D. J., Clark, J. M., Evans, C. D., Evans, M. G., Ostle, N. J., Worrall, F., Burden, A., Dinsmore, K. J., Jones, T., McNamara, N. P., Parry, L., Rowson, J. G., and Rose, R.: Carbon balance of UK peatlands: current state of knowledge and future research challenges, Clim. Res., 45, 13–29, https://doi.org/10.3354/cr00903, 2010.
Blough, N. V.: Photochemical Processes, in: Encyclopedia of Ocean Sciences, edited by: Thorpe, S. A. and Turekian, K. K., Academic Press, Photochemical Processes, 2162, https://doi.org/10.1006/rwos.2001.0072, 2001.
Bond, T., Huang, J., Graham, N. J. D., and Templeton, M. R.: Examining the interrelationship between DOC, bromide and chlorine dose on DBP formation in drinking water – a case study, Sci. Total Environ., 470/471, 469–479, https://doi.org/10.1016/j.scitotenv.2013.09.106, 2014.
Carignan, R., Planas, D., and Vis, C.: Planktonic production and respiration in oligotrophic Shield lakes, Limnol. Oceanogr., 45, 189–199, https://doi.org/10.4319/lo.2000.45.1.0189, 2000.
Cole, J. J. and Prairie, Y. T.: Dissolved CO2 in Freshwater Systems, in: Reference Module in Earth Systems and Environmental Sciences, 1–5, ISBN: 9780124095489, https://doi.org/10.1016/B978-0-12-409548-9.09399-4, 2014.
Cole, J. J., Likens, G. E., and Hobbie, J. E.: Decomposition of planktonic algae in an oligotrophic lake, Oikos, 42, 257–266, https://doi.org/10.2307/3544393, 1984.
Cole, J. J., Prairie, Y. T., Caraco, N. F., McDowell, W. H., Tranvik, L. J., Striegl, R. G., Duarte, C. M., Kortelainen, P., Downing, J. A., Middelburg, J. J., and Melack, J.: Plumbing the Global Carbon Cycle: Integrating Inland Waters into the Terrestrial Carbon Budget, Ecosystems, 10, 172–185, https://doi.org/10.1007/s10021-006-9013-8, 2007.
del Giorgio, P. A. and Peters, R. H.: The balance between phytoplankton production and plankton respiration in lakes, Can. J. Fish. Aquat. Sci., 50, 282–289, https://doi.org/10.1139/f93-032, 1993.
del Giorgio, P. A., Cole, J. J., and Cimbleris, A.; Respiration rates in bacteria exceed phytoplankton production in unproductive aquatic systems, Nature, 385, 148–150, https://doi.org/10.1038/385148a0, 1997.
DelSontro, T., Beaulieu, J. J., and Downing, J. A.: Greenhouse gas emissions from lakes and impoundments: Upscaling in the face of global change, Limnol. Oceanog. Lett., 3, 64–75, https://doi.org/10.1002/lol2.10073, 2018.
Dordoni, M., Seewald, M., Rinke, K., Schmidmeier, J., and M., Barth, J. A. C.: Novel evaluations of sources and sinks of dissolved oxygen via stable isotopes in lentic water bodies, Sci. Total Environ., 838, 156541, https://doi.org/10.1016/j.scitotenv.2022.156541, 2022.
Duarte, C. M. and Agustí, S.: The CO2 Balance of Unproductive Aquatic Ecosystems, Science, 281, 234–236, https://doi.org/10.1126/science.281.5374.234, 1998.
Emerson, S.: Chemically enhanced CO2 gas exchange in a euthrophic lake: A general model, Limnol. Oceanog., 20, 743–753, https://doi.org/10.4319/lo.1975.20.5.0743, 1975.
Fisher, I. H., Kastl, G., and Sathasivan, A.: New model of chlorine-wall reaction for simulating chlorine concentration in drinking water distribution systems, Water Res., 125, 427–437, https://doi.org/10.1016/j.watres.2017.08.066, 2017.
Friese, K., Schultze, M., Böhrer, B., Büttner, O., Herzsprung, P., Koschorreck, M., Kuehn, B., Rönicke, H., Tittel, J., Wendt-Potthoff, K., Wollschläger, U., Dietze, M., and Rinke, K.: Ecological response of two hydro-morphological similar pre-dams to contrasting land-use in the Rappbode reservoir system (Germany), Int. Rev. Ges. Hydrobiol. Hydrogr., 99, 335–349, https://doi.org/10.1002/iroh.201301672, 2014.
Gammons, C. H., Babcock, J. N., Parker, S. R., and Poulson, S. R.: Diel cycling and stable isotopes of dissolved oxygen, dissolved inorganic carbon, and nitrogenous species in a stream receiving treated municipal sewage, Chem. Geol., 283, 44–55, https://doi.org/10.1016/j.chemgeo.2010.07.006, 2011.
Gammons, C. H., Henne, W., Poulsom, S. R., Parker, S. R., Johnston, T. B., Dore, J. E., and Boyd, E. S.: Stable isotopes track biogeochemical processes under seasonal ice cover in a shallow, productive lake, Biogeochemistry, 120, 359–379, https://doi.org/10.1007/s10533-014-0005-z, 2014.
Gattuso, J.-P., Peduzzi, S., Pizay, M.-D., and Tonolla, M.: Changes in freshwater bacterial community composition during measurements of microbial and community respiration, J. Plank. Res., 24, 1197–1206, https://doi.org/10.1093/plankt/24.11.1197, 2002.
Giling, D. P., Staehr, P. A., Grossart, H. P., Andersen, M. R., Boehrer, B., Scot, C., Evrendilek, F., Gómez-Gener, L., Gisriel, C., Shen, G., Kurashow, V., Ho, M.-Y., Zhang, S., Williams, D., Golbeck, J. H., Fromme, P., and Bryant, D. A.: The structure of Photosystem I acclimated to far-red light illuminates an ecologically important acclimation process in photosynthesis, Sci. Adv., 6, 1–11, https://doi.org/10.1126/sciadv.aay6415, 2020.
Hanson, P. C., Bade, D. L., Carpenter, S. R., and Kratz, T. K.: Lake metabolism: Relationships with dissolved organic carbon and phosphorus, Limnol. Oceanogr., 48, 1112–1119, https://doi.org/10.4319/lo.2003.48.3.1112, 2003.
Herzsprung, P., Wentzky, V., Kamjunke, N., von Tumpling, W., and Wilske, C.: Improved understanding of dissolved organic matter processing in freshwater using complementary experimental and machine learning approaches, Environ. Sci. Technol., 54, 13556–13565, https://doi.org/10.1021/acs.est.0c02383, 2020.
Huang, C., Chen, Z., Gao, Y., Luo, Y., Huang, T., Zhu, A., Yang, H., and Yang, B.: Enhanced mineralization of sedimentary organic carbon induced by excess carbon from phytoplankton in a eutrophic plateau lake, J. Soils Sediments, 19, 2613–2623, https://doi.org/10.1007/s11368-019-02261-2, 2019.
Jansen, J., Woolway, R. I., Kraemer, B. M., Albergel, C., Bastviken, D., Weyhenmeyer, G. A., Marcé, R., Sharma, S., Sobek, S., Tranvik, L. J., Perroud, M., Golub, M., Moore, T. N., Vinnå, L. R., La Fuente, S., Grant, L., Pierson, D. C., Thiery, W., and Jennings, E.: Global increase in methane production under future warming of lake bottom waters, Glob. Change Biol., 28, 5427–5440, https://doi.org/10.1111/gcb.16298, 2022.
Karlsson, J., Bystrom, P., Ask, J., Ask, P., Persson, L., and Jansson, M.: Light limitation of nutrient-poor lake ecosystems, Nature, 460, 506–509, https://doi.org/10.1038/nature08179, 2009.
Karst, G., Sathasivan, A., Fisher, I. H., and van Leeuwen, J.: Modeling DOC Removal by Enhanced Coagulation, J. Am. WATER Work Assoc., 96, 79–89, https://doi.org/10.1002/j.1551-8833.2004.tb10557.x, 2004.
Kawasaki, N. and Benner, R.: Bacterial release of dissolved organic matter during cell growth and decline: molecular origin and composition, Limnol. Oceanogr., 51, 2170–2180, https://doi.org/10.4319/lo.2006.51.5.2170, 2006.
Keaveney, E. M., Radbourne, A. D., McGowan, S., Ryves, D. B., and Reimer, P. J.: Source and quantity of carbon influence its sequestration in Rostherne Mere (UK) sediment: a novel application of stepped combustion radiocarbon analysis, J. Paleolimnol., 64, 347–363, https://doi.org/10.1007/s10933-020-00141-1, 2020.
Khan, H., Marcé, R., Laas, A., and Obrador, B.: The relevance of pelagic calcification in the global carbon budget of lakes and reservoirs, Limnetica, 41, 17–25, https://doi.org/10.23818/limn.41.02, 2022.
Kong, X., Zhan, Q., Boehrer, B., and Rinke, K.: High frequency data provide new insights into evaluating and modeling nitrogen retention in reservoirs, Water Res., 166, 115017, https://doi.org/10.1016/j.watres.2019.115017, 2019.
Koschorreck, M., Hentschel, I., and Boehrer, B.: Oxygen Ebullition From Lakes, Geophys. Res. Lett., 44, 9372–9378, https://doi.org/10.1002/2017GL074591, 2017.
Kreling, J., Bravidor, J., Engelhardt, C., Hupfer, M., Koschorreck, M., and Lorke, A.: The importance of physical transport and oxygen consumption for the development of a metalimnetic oxygen minimum in a lake, Limnol. Oceanogr., 62, 348–363, https://doi.org/10.1002/lno.10430, 2017.
Kretz, R.: Calculation and illustration of uncertainty in geochemical analyses, J. Geol. Educ., 33, 40–44, https://doi.org/10.5408/0022-1368-33.1.40, 1985.
Kritzberg, E. S., Cole, J. J., Pace, M. L., Granéli, W., and Bade, D. L.: Autochthonous versus allochthonous carbon sources of bacteria: Results from whole-lake 13C addition experiments, Limnol. Oceanog., 49, 588–596, https://doi.org/10.4319/lo.2004.49.2.0588, 2004.
Ku, H. H.: Notes on the use of propagation of error formulas, J. Res. Nat. Bur. Stand. Sect. C., 70, 263–273, 1966.
Liu, X., Wendt-Potthof, K., Barth, J. A. C., and Friese, K.: Post-depositional alteration of stable isotope signals by preferential degradation of algae-derived organic matter in reservoir sediments, Biogeochemistry, 159, 315–336, https://doi.org/10.1007/s10533-022-00930-y, 2022.
MacKenzie, A. B., Cook, G. T., Barth, J., Gulliver, P., and McDonald, P.: 14C and δ13C characteristics of organic matter and carbonate in saltmarsh sediments from south west Scotland, J. Environ. Monit., 6, 441–447, https://doi.org/10.1039/B315766K, 2004.
Mazuecos, I. P., Arístegui, J., Vázquez-Domínguez, E., Ortega-Retuerta, E., Gasol, J. M., and Reche, I.: Temperature control of microbial respiration and growth efficiency in the mesopelagic zone of the South Atlantic and Indian Oceans, Deep-Sea Res. Pt. I, 95, 131–138, https://doi.org/10.1016/j.dsr.2014.10.014 2016.
McDonald, C. P., Saeed, M. N., Robertson, D. M., and Prellwitz, S.: Temperature explains the formation of a metalimnetic oxygen minimum in a deep mesotrophic lake, Inland Waters, 12, 1–10, https://doi.org/10.1080/20442041.2022.2029318, 2022.
Mi, C., Shatwell, T., Ma, J., Wentzky, V. C., Boehrer, B., Xu, Y., and Rinke, K.: The formation of a metalimnetic oxygen minimum exemplifies how ecosystem dynamics shape biogeochemical processes: A modelling study, Water Res., 175, 115701, https://doi.org/10.1016/j.watres.2020.115701, 2020.
Nix, J.: Contribution of hypolimnetic water on metalimnetic dissolved oxygen minima in a reservoir, Water Res., 17, 329–332, https://doi.org/10.1029/WR017i002p00329, 1981.
Nürnberg, G. K., LaZerte, B. D., and Olding, D. D.: An Artificially induced Planktothrix rubescens surface bloom in a small kettle lake in Southern Ontario compared to blooms world-wide, Lake Reservoir Manag., 19, 307–322, https://doi.org/10.1080/07438140309353941, 2003.
Pace, M. L. and Prairie, Y.: Respiration in lakes, in: Respiration in Aquatic Ecosystems, edited by: del Giorgio, P. and Williams, P., Oxford Academic, chap. 7, Respiration in lakes, 103–121, https://doi.org/10.1093/acprof:oso/9780198527084.003.0007, 2005.
Piatka, D. R., Frank, A. H., Köhler, I., Castiglione, K., van Geldern, R., and Barth, J. A. C.: Balance of carbon species combined with stable isotope ratios show critical switch towards bicarbonate uptake during cyanobacteria blooms, Sci. Total Environ., 807, https://doi.org/10.1016/j.scitotenv.2021.151067, 2022.
Raymond, P. A., Hartmann, J., Lauerwald, R., Sobek, S., McDonald, C., Hoover, M., Butman, D., Striegl, R., Mayorga, E., Humborg, C., Kortelainen, P., Dürr, H., Meybeck, M., Ciais, P., and Guth, P.: Global carbon dioxide emissions from inland waters, Nature, 503, 355–359, https://doi.org/10.1038/nature12760, 2013.
Regnier, P., Friedlingstein, P., Ciais, P., Mackenzie, F. T., Gruber, N., Janssens, I. A., Laruelle, G. G., Lauerwald, R., Luyssaert, S., Andersson, A. J., Arndt, S., Arnosti, C., Borges, A. V., Dale, A. W., Gallego-Sala, A., Goddéris, Y., Goossens, N., Hartmann, J., Heinze, C., Ilyina, T., Joos, F., LaRowe, D. E., Leifeld, J., Meysman, F. J. R., Munhoven, G., Raymond, P. A., Spahni, R., Suntharalingam, P., and Thullner, M.: Anthropogenic perturbation of the carbon fluxes from land to ocean, Nat. Geosci., 6, 597–607, https://doi.org/10.1038/NGEO1830, 2013.
Rinke, K., Kuehn, B., Bocaniov, S., Wendt-Potthoff, K., Büttner, O., Tittel, J., Schultze, M., Herzsprung, P., Rönicke, H., Rink, K., Rinke, K., Dietze, M., Matthes, M., Paul, L., and Friese, K.: Reservoirs as sentinels of catchments: the Rappbode Reservoir Observatory (Harz Mountains, Germany), Environ. Earth Sci., 69, 523–536, https://doi.org/10.1007/s12665-013-2464-2, 2013.
Robarts, R. D.: Decomposition is freshwater ecosystems, J. Limnol. Soc. Southern Afr., 12, 72–89, https://doi.org/10.1080/03779688.1986.9639399, 1986.
Scavia, D. and Laird, G. A.: Bacterioplankton in Lake Michigan: Dynamics, controls, and significance to carbon flux, Limnol. Oceanogr., 32, 1017–1033, https://doi.org/10.4319/lo.1987.32.5.1017, 1987.
Schindler, D. W. and Gunn, J. M.: Dissolved organic carbon as a controlling variable in lake trout and other Boreal Shield lakes. Boreal Shield watersheds: Lake trout ecosystems in a changing environment, edited by: Gunn, J. M., Steedman, R. J., and Ryder, R. A., Lewis Publishers, Boca Raton, Fla, 133–146, https://doi.org/10.1201/9780203495087.CH8, 2004.
Schulte, P., van Geldern, R., Freitag, H., Karim, A., Négrel, P., Petelet-Giraud, E., Probst, J.-L., Telmer, K., Veizer, J., and Barth, J. A. C.: Applications of stable water and carbon isotopes in watershed research: weathering, carbon cycling, and water balances, Earth Sci. Rev., 109, 20–31, https://doi.org/10.1016/j.earscirev.2011.07.003, 2011.
Shapiro, J.: The cause of a metalimnetic minimum of dissolved oxygen, Limnol. Oceanogr., 5, 216–227, 1960.
Sommer, U., Gliwicz, Z. M., Lampert, W. I., and Duncan, A.: The PEG-model of seasonal succession of planktonic events in fresh waters, Arch. Hydrobiol., 106, 433–471, 1986.
Stiller, M. and Nissenbaum, A.: A stable carbon isotope study of dissolved inorganic carbon in hardwater Lake Kinneret (Sea of Galilee), S. Afr. J. Sci., 95, 166–170, 1999.
Sun, L., Leybourne, M., Rissman, C., and Brikowski, T.: Geochemistry of a large impoundment – part II: Fe and Mn cycling and metal transport, Geochem. Explor. Environ. Anal., 16, 165–177, https://doi.org/10.1144/geochem2015-361, 2016.
Thurman, E. M.: Organic geochemistry of natural waters, Martinus Nijhoff/Dr. W. Junk Publishers, ISBN: 978-94-009-5095-5, 1985.
Tittel, J., Hüls, M., and Koschorreck, M.: Terrestrial Vegetation Drives Methane Production in the Sediments of two German Reservoirs, Sci. Rep., 9, 15944, https://doi.org/10.1038/s41598-019-52288-1, 2019.
Tranvik, L. J., Downing, J. A., Cotner, J. B., Loiselle, S. A., Striegl, R.G., Ballatore, T. J., Dillon, P., Finlay, K., Fortino, K., Knoll, L. B., Kortelainen, P. L., Kutser, T., Larsen, S., Laurion, I., Leech, D. M., McCallister, S. L., McKnight, D. M., Melack,, J. M., Overholt, E., Porter, J. A., Prairie, Y., Renwick, W. H., Roland, F., Sherman, B. S., Schindler, D. W., Sobek, S., Tremblay, A., Vanni, M. J., Verschoor, A. M., von Wachenfeldt, E., and Weyhenmeyer, G. A.: Lakes and reservoirs as regulators of carbon cycling and climate, Limnol. Oceanogr., 54, 2298–2314, https://doi.org/10.4319/lo.2009.54.6_part_2.2298, 2009.
van Geldern, R., Schulte, P., Mader, M., Baier, A., and Barth, J. A. C.: Spatial and temporal variations of pCO2, dissolved inorganic carbon, and stable isotopes along a temperate karstic watercourse, Hydrol. Process., 29, 3423–3440, https://doi.org/10.1002/hyp.10457, 2015.
Wachniew, P.: Isotopic composition of dissolved inorganic carbon in a large polluted river: the Vistula, Poland, Chem. Geol., 233, 293–308, https://doi.org/10.1016/j.chemgeo.2006.03.012, 2006.
Wang, J., Guan, Y., Wu, L., Guan, X., Cai, W., Huang, J., Dong, W., and Zhang, B.: Changing Lengths of the Four Seasons by Global Warming, Geophys. Res. Lett., 48, https://doi.org/10.1029/2020GL091753, 2021.
Wentzky, V., Frassl, M. A., Rinke, K., and Boehrer, B.: Metalimnetic oxygen minimum and the presence of Planktothrix rubescens in a low-nutrient drinking water reservoir, Water Res., 148, 208–218, https://doi.org/10.1016/j.watres.2018.10.047, 2019.
Wetzel, R. G.: Limnology, 2nd Edn., Saunders College Publishing, Philadelphia, ISBN: 0-03-057913-9, 1983.
Wetzel, R. G., Rich, P. H., Miller, M. C., and Allen, H. L.: Metabolism of dissolved and particulate detrital carbon in a temperate hard-water lake, Mem. Ist. Ita. Idrobiol., 29, 185–243, 1972.
Willey, J. D., Kieber, R. J., Eyman Jr., M. S., and Brooks Avery, G.: Rainwater dissolved organic carbon concentrations and global flux, Global Biogeochem. Cy., 14, 139–148, https://doi.org/10.1029/1999GB900036, 2000.
Wu, J. L. and Chen, M.: Effects of nitrogen and phosphorus on phytoplankton composition and biomass in 15 subtropical, urban shallow lakes in Wuhan, China, Limnologica, 41, 48–56, https://doi.org/10.1016/j.limno.2010.03.003, 2011.