the Creative Commons Attribution 4.0 License.
the Creative Commons Attribution 4.0 License.
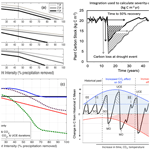
Exploring the impacts of unprecedented climate extremes on forest ecosystems: hypotheses to guide modeling and experimental studies
David M. Medvigy
Benjamin Smith
Jeffrey S. Dukes
Claus Beier
Mikhail Mishurov
Xiangtao Xu
Jeremy W. Lichstein
Craig D. Allen
Klaus S. Larsen
Cari Ficken
William T. Pockman
William R. L. Anderegg
Anja Rammig
Climatic extreme events are expected to occur more frequently in the future, increasing the likelihood of unprecedented climate extremes (UCEs) or record-breaking events. UCEs, such as extreme heatwaves and droughts, substantially affect ecosystem stability and carbon cycling by increasing plant mortality and delaying ecosystem recovery. Quantitative knowledge of such effects is limited due to the paucity of experiments focusing on extreme climatic events beyond the range of historical experience. Here, we present a road map of how dynamic vegetation demographic models (VDMs) can be used to investigate hypotheses surrounding ecosystem responses to one type of UCE: unprecedented droughts. As a result of nonlinear ecosystem responses to UCEs that are qualitatively different from responses to milder extremes, we consider both biomass loss and recovery rates over time by reporting a time-integrated carbon loss as a result of UCE, relative to the absence of drought. Additionally, we explore how unprecedented droughts in combination with increasing atmospheric CO2 and/or temperature may affect ecosystem stability and carbon cycling. We explored these questions using simulations of pre-drought and post-drought conditions at well-studied forest sites using well-tested models (ED2 and LPJ-GUESS). The severity and patterns of biomass losses differed substantially between models. For example, biomass loss could be sensitive to either drought duration or drought intensity depending on the model approach. This is due to the models having different, but also plausible, representations of processes and interactions, highlighting the complicated variability of UCE impacts that still need to be narrowed down in models. Elevated atmospheric CO2 concentrations (eCO2) alone did not completely buffer the ecosystems from carbon losses during UCEs in the majority of our simulations. Our findings highlight the consequences of differences in process formulations and uncertainties in models, most notably related to availability in plant carbohydrate storage and the diversity of plant hydraulic schemes, in projecting potential ecosystem responses to UCEs. We provide a summary of the current state and role of many model processes that give way to different underlying hypotheses of plant responses to UCEs, reflecting knowledge gaps which in future studies could be tested with targeted field experiments and an iterative modeling–experimental conceptual framework.
- Article
(2788 KB) - Full-text XML
-
Supplement
(864 KB) - BibTeX
- EndNote
Extreme climate and weather events, such as prolonged heatwaves and droughts as seen over the last 3 decades, are expected to continue to increase in frequency and magnitude, leading to progressively longer and warmer droughts on land (IPCC, 2012, 2021). Droughts are affecting all areas of the globe, more than any other natural disturbance, and recent droughts have broken long-standing records (Ciais et al., 2005; Phillips et al., 2009; Williams et al., 2012; Matusick et al., 2013; Griffin and Anchukaitis, 2014; Asner et al., 2016; Feldpausch et al., 2016; Seneviratne et al., 2021). Such “unprecedented climate extremes” (UCEs; “record-breaking events”, IPCC, 2012) that are larger in extent and longer-lasting than historical norms can have dramatic consequences for terrestrial ecosystem processes, including carbon uptake and storage and other ecosystem services (Reichstein et al., 2013; Allen et al., 2015; Brando et al., 2019; Kannenberg et al., 2020). Thus, to better anticipate the implications of climatic changes for the terrestrial carbon sink and other ecosystem services, we need to better understand how ecosystems respond to extreme droughts and other UCEs.
To learn how ecosystems respond to rarely experienced or unprecedented conditions, ecologists can experimentally manipulate environmental conditions (Rustad, 2008; Beier et al., 2012; Meir et al., 2015; Aguirre et al., 2021). However, the majority of such experiments apply moderate treatments based on a historical sense, which are mostly weaker in intensity and/or shorter in duration than potential future UCEs (Beier et al., 2012; Kayler et al., 2015; but see Luo et al., 2017), and single experiments have low power to detect effects of stressors on ecosystem responses (Yang et al., 2022). Additionally, most experiments examine low-stature ecosystems, such as grassland, shrubland, or tundra, due to lower requirements for infrastructure and financial investment compared to mature forests. However, forests may respond qualitatively differently to UCEs than other ecosystems, in part due to mortality of large trees and strong nonlinear ecosystem responses, with long-lasting consequences for ecosystem–climate feedbacks (Williams et al., 2014; Meir et al., 2015). Ecosystem responses to naturally occurring extreme droughts and heatwaves have been documented (Ciais et al., 2005; Breshears et al., 2009; Feldpausch et al., 2016; Matusick et al., 2016; Ruthrof et al., 2018; Powers et al., 2020); however, these rapidly mobilized post hoc studies are often unable to measure all critical variables and may lack consistently collected data for comparison with pre-drought conditions, thus limiting their inferential power and ability to improve quantitative models. The difficulties of performing controlled real-world experiments of UCEs at broad spatial and temporal scales make process-based modeling a valuable tool for studying potential ecosystem responses to extreme events.
Process-based models can be used to explore potential ecosystem impacts using projected climate change over broad spatial and temporal scales (Gerten et al., 2008; Luo et al., 2008; Zscheischler et al., 2014; Sippel et al., 2016), as seen in a few modeling studies that have synthesized and improved our process-level understanding of UCE effects (McDowell et al., 2013; Dietze and Matthes, 2014). However, due to the overly simplified representation of ecological processes in most land surface models (LSMs) – the terrestrial components of Earth system models (ESMs) used for climate projections – it is doubtful whether most of these models adequately capture ecosystem feedbacks and other responses to UCEs (Fisher and Koven, 2020). For example, only a few ESMs in recent coupled model intercomparison projects (CMIP6) (Arora et al., 2020; IPCC, 2021) include vegetation demographics (Döscher et al., 2022), and most rely on prescribed, static maps of plant functional types (PFTs) (Ahlström et al., 2012). Other LSMs simulate PFT shifts (i.e., dynamic global vegetation models, DGVMs; Sitch et al., 2008) based on bioclimatic limits, instead of emerging from the physiology- and competition-based demographic rates that determine resource competition and plant distributions in real ecosystems (Fisher et al., 2018). While a new generation of LSMs with more explicit ecological dynamics and structured demography is emerging (Holm et al., 2020; Koven et al., 2020; Döscher et al., 2022), most current ESMs are limited in ecological detail and realism (e.g., ecosystem structure, demography, and disturbances). Failing to mechanistically represent mortality, recruitment, and disturbance – each of which influences biomass turnover and carbon (C) allocation (Friend et al., 2014) – limits the ability of these models to realistically forecast ecosystem responses to anomalous environmental conditions like UCEs (Fisher et al., 2018).
Evaluating and improving the representation of physiological and ecological processes in ecosystem models are critical for reducing model uncertainties when projecting the effects of UCEs on long-term ecosystem dynamics and functioning. Vegetation demography, plant hydraulics, enhanced representations of plant trait variation, explicit treatments of resource competition (e.g., height-structured competition for light), and representing major disturbances (e.g., extreme drought) have all been identified as critical areas for advancing current models (Scheiter et al., 2013; Fisher et al., 2015; Weng et al., 2015; Choat et al., 2018; Fisher et al., 2018; Blyth et al., 2021) and are necessary advances for realistically representing the ecosystem impacts of UCEs. In this perspectives-focused paper we look at the differences in these processes and how they contribute to uncertainty across multiple temporal phases surrounding an extreme event: predicting an ecosystem's pre-disturbance resistance, which influences the degree of impact and recovery from UCEs. Table 1 describes a summary of model mechanisms that affect pre-drought resistance and post-drought recovery and that we suggest are critical areas for further research (cf. Frank et al., 2015).
Table 1Hypothesized plant processes and ecosystem state variables affecting pre-drought resistance and post-drought recovery in the context of unprecedented climate extremes (UCEs). The “Included in model?” column indicates which processes or state variables are represented in each of the two models studied in this paper. The mechanisms listed in the two right columns refer to real-world ecosystems and are not necessarily represented in the ED2 and LPJ-GUESS models. The contents of the table are based on a non-exhaustive literature review, expert knowledge, and modeling results presented here.
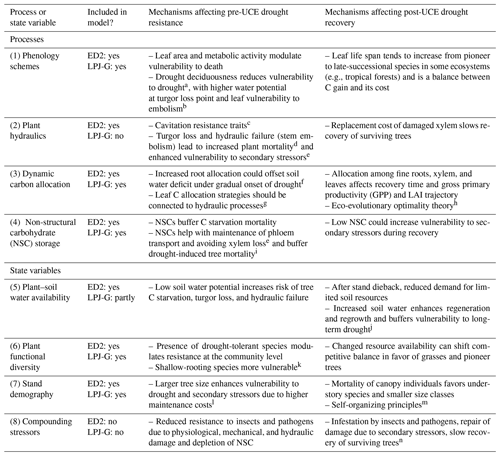
Letters refer to the following literature sources. a Borchert et al. (2002), Williams et al. (2008). b Zhu et al. (2018), Vargas et al. (2021). c Rowland et al. (2015), McDowell et al. (2013), Anderegg et al. (2015). d Adams et al. (2017b). e Dietze and Matthes (2014). f Joslin et al. (2000), Markewitz et al. (2010). g Trugman et al. (2019). h Franklin et al. (2012). i O'Brien et al. (2014), Signori-Müller et al. (2021). j McDowell et al. (2006), D'Amato et al. (2013). k Enquist and Enquist (2011), Greenwood et al. (2017), Powell et al. (2018). l Bennett et al. (2015), Rowland et al. (2015). m Franklin et al. (2020). n Hubbard et al. (2013).
1.1 Objectives
In order to inform our discussion, we explore the potential responses of forest ecosystems to UCEs using two state-of-the-art process-based demographic models (vegetation demographic models, VDMs; Fisher et al., 2018), a unique model exploration–discussion approach to help highlight new paths forward for model advancement. We first present conceptual frameworks and hypotheses on potential ecosystem responses to UCEs based on current knowledge. We then present VDM simulations for a range of hypothetical UCE scenarios to illustrate current state-of-the-art model representations of eco-physiological mechanisms expected to drive responses to UCEs, using droughts as an example. While a variety of UCE-linked biophysical tree disturbance processes (e.g., fire, wind, insect outbreaks) can drive nonlinear ecosystem responses, we focus specifically on extreme droughts, which have important impacts on many ecosystems around the world (e.g., Frank et al., 2015; IPCC, 2021). By studying modeled responses to UCEs, we explore the limits to our current understanding of ecosystem responses to extreme droughts and their corresponding thresholds and tipping points. As anthropogenic forcing has increased the frequency, duration, and intensity of droughts throughout the world (Chiang et al., 2021), we explore how eCO2 and rising temperatures may affect drought-induced C loss and recovery trajectories. This study can help guide how the scientific community can iteratively address these questions through future experiments and modeling studies. We believe the combination of using cutting-edge VDMs alongside an inspection of current gaps in knowledge will help guide modeling and experimental advances in order to address novel forest responses to climate extremes.
1.2 Conceptual and modeling framework for hypothesis testing
We combine conceptual frameworks (Fig. 1) and ecosystem modeling to test two
hypotheses on potential responses of plant carbon stocks to UCEs. The first
hypothesis is as follows.
Hypothesis (H1). Terrestrial ecosystem responses to UCEs will differ qualitatively from ecosystem responses to milder extremes because responses are nonlinear and highly variable. Nonlinearities can arise from multiple mechanisms – including shifts in plant hydraulics, C allocation, phenology, and stand demography – and can vary depending on the pre-drought state of the ecosystem.
We present three conceptual relationships that describe terrestrial
ecosystem responses to varying degrees of extreme events (Fig. 1). We
hypothesize that change in vegetation C stock is related to drought
intensity and/or drought duration, such that biomass loss increases
nonlinearly with increased drought intensity (i.e., reduction in
precipitation) represented by a threshold-based relationship (Fig. 1a, H1a),
increased drought duration (i.e., prolonged drought with the same intensity)
by shifting responses typically seen in milder extremes downwards via
increasing slopes (Fig. 1a, H1b), or the combination of both intensity and
duration (Fig. 1a, H1c). These hypotheses are supported by observations from
the Amazon basin and Borneo (Phillips et al., 2010), where tree mortality
rates increased nonlinearly with drought intensity. Similarly, plant
hydraulic theories predict nonlinear damage to the plant-water transport
systems, and thus mortality risk, as a function of drought stress (Sperry
and Love, 2015). In particular, longer droughts are more likely to lead to
lower soil water potentials, leading to a nonlinear xylem damage function
even if stomata effectively limit water loss (Sperry et al., 2016).
Hypothesis (H2). The effects of increasing atmospheric CO2 concentration (eCO2) will alleviate impacts of extreme drought stress through an increase in vegetation productivity and water-use efficiency, but only up to a threshold of drought severity, while increased temperature (and related water stress) will exacerbate tree mortality.
This second hypothesis is based on growing evidence that the effects of
eCO2 and climate warming may interact with the effects of drought intensity
on ecosystems. The CO2 fertilization effect enhances vegetation
productivity (e.g., net primary production, NPP) (Ainsworth and Long, 2005;
Norby et al., 2005; Wang et al., 2012), but this fertilization effect is
generally reduced by drought (Hovenden et al., 2014; Reich et al., 2014;
Gray et al., 2016). Drought events often coincide with increased
temperature, which intensifies the impact of drought on ecosystems (Allen et
al., 2015; Liu et al., 2017), resulting in nonlinear responses in mortality
rates (Adams et al., 2009, 2017a). The evaluation of C cycling
in VDMs with doubling of CO2 (only “beta effect”) showed a large
carbon sink in a tropical forest (Holm et al., 2020), but the inclusion of
climate interactions in VDMs needs to be further explored.
Here, we relate ecosystem responses to UCEs by calculating a “severity-drought index” (Fig. 1b and see Sect. 2.3), which integrates C loss from the beginning of the drought until the time when C stocks have recovered to 50 % of the pre-drought level. In response to drought, warming, and eCO2, divergent potential C responses (gains and losses; Fig. 1c) can be expected (Keenan et al., 2013; Zhu et al., 2016; Adams et al., 2017a). For example, a grassland macrocosm experiment found that eCO2 completely compensated for the negative impact of extreme drought on net carbon uptake due to increased root growth and plant nitrogen uptake and led to enhanced post-drought recovery (Roy et al., 2016). However, a 16-year grassland Free-Air Carbon dioxide Enrichment (FACE) and the Soybean Free Air Concentration Enrichment (SoyFACE) experiment showed that CO2 fertilization effects were reduced or eliminated under hotter and/or drier conditions (Gray et al., 2016; Obermeier et al., 2016). Reich et al. (2014) also found that CO2 fertilization effects were reduced in a perennial grassland by water and nitrogen limitation.
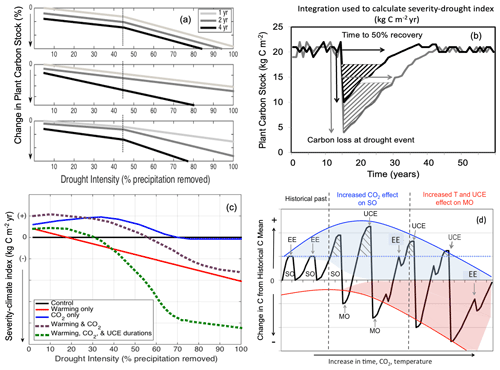
Figure 1Conceptual diagrams showing impacts of extreme droughts (unprecedented climate extremes, UCEs; i.e., record-breaking droughts) on plant C stocks. (a) Conceptual diagram of UCE C loss: potential loss in C stock as a function of increasing drought intensity (0 %–100 % precipitation removal) and drought duration (1, 2, or 4 years of drought). In this example, an arbitrary threshold of 45 % precipitation reduction and 4-year drought duration is assumed to correspond to a UCE. Hypotheses include nonlinear and threshold responses to drought intensity (H1a), drought duration via different slope responses (H1b), and combined effects of both drought intensity and durations (H1c). (b) Conceptualized diagram of integrated C change: responses of forest C stocks to a large (grey) and small (black) UCE. “Severity-drought index” (kg C m−2 yr) denotes the integral of the C loss over time and is calculated from the two arrows: the total loss in C (kg C m−2) due to drought and the time (year) to recover 50 % of the pre-drought C stock. (c) Conceptualized UCE-climate C change diagram: hypothetical response in terrestrial “severity-climate index” (kg C m−2 yr) due to eCO2 (blue line), rising temperature (red line), interaction between eCO2 and temperature (dashed purple), and combined interactions among eCO2, temperature, and UCEs of prolonged durations (green line), all relative to a reference drought of normal duration with no warming (black line). Severity-climate index denotes the difference in severity-drought index (see b) between a scenario of changing climatic drivers and the reference drought with no climate change (control). (d) Conceptual UCE amplification diagram: hypothetical amplified change in forest C stocks to eCO2 and temperature relative to the pre-warming historical past (based on Jump et al., 2017). Change in C stock greater than zero indicates a “structural overshoot” (SO) due to favorable environmental conditions and/or recovery from an extreme drought–heat event (EE). Hashed black areas indicate a structural overshoot due to eCO2, which occurs over the historical CO2 levels (dashed blue line). Initially, an eCO2 effect leads to a larger increase in structural overshoot (due to CO2 fertilization), driving more extreme vegetation mortality (“mortality overshoot” – MO) relative to historical dieback events and thus a greater decrease in C stock. Increased warming through time increasingly counteracts any CO2 fertilization effect. While the amplitude of post-UCE C stock recoveries remains large, net C stock values eventually decline (downward curvature and widening of the red shaded area) due to more pronounced loss in C stocks (and greater ecosystem state change) from hotter UCEs and longer recovery periods. We conceptualize how oscillations between SOs and MOs could be amplified, and the widening of the shaded areas represents increased variability in how unprecedented eCO2 levels and temperatures will affect ecosystems in the future compared to historically. SO refers to structural overshoot, MO refers to mortality overshoot, EE refers to historically extreme drought–heat event, and UCE refers to unprecedented climate extreme.
A corollary to our H2 is that conditions that favor productivity (e.g., longer growing seasons and/or CO2 fertilization) will enhance vegetation growth, leading to structural overshoot (SO; Fig. 1d; adapted from and supported by Jump et al., 2017), and can amplify the effects of UCEs. Enhanced vegetation growth coupled with environmental variability can lead to exceptionally high plant-water demand during extreme drought and water stress, resulting in a mortality overshoot (MO; Fig. 1d). We conceptualize how oscillations between SO and associated MO could be amplified by increasing climatic variability and UCEs (Fig. 1d). Additionally, more climatic variability from unprecedented eCO2 levels and warming will contribute to unknowns in how ecosystems are affected in the future (i.e., the widening and downward shape of the shaded areas compared to historically, Fig. 1d). We expect, however, that a rapidly changing climate, combined with effects of UCEs as a result of more frequent extreme drought/heat events and drought stress, can exacerbate and amplify SOs and MOs (Jump et al., 2017), leading to increasing C loss, even though various buffering mechanisms exist (cf. Lloret et al., 2012; Allen et al., 2015). Relative to our conceptual diagrams (Fig. 1d), we note that most experimental, observational, and modeling studies (Ciais et al., 2005; da Costa et al., 2010; Phillips et al., 2010; Meir et al., 2015) take into account only low to moderate drought intensities (such as 50 % rain excluded) or single events, or they combine drought with the moderate effects of temperature change. Where there has been 100 % rain exclusion, it was on very small plots of 1.5 m2 (Meir et al., 2015). As represented by the increasing amplitude of oscillations in Fig. 1d, the interactions between increased temperatures, UCE events, and vegetation feedbacks make ecosystem states become inherently unpredictable, particularly over longer timescales.
We argue that VDMs are well suited to address climate change impacts due to the inclusion of detailed process representation of dynamic plant growth, recruitment, and mortality, resulting in changes in abundance of different PFTs, as well as vertically stratified tree size and age class structured ecosystem demography. Community dynamics and age/size structure are emergent properties from competition for light, space, water, and nutrients, which dynamically and explicitly scale up from the tree to the stand to the ecosystem level. Within this characterization, VDMs also differ between each other and are set up in different configurations, allowing for various testing capabilities. For the full names of each model listed below and references, see Table S1 in the Supplement. For example, VDMs can aggregate and track the community-level disturbance into either patch-tiling sampling (e.g., ED2, FATES, LM3-PPA, ORCHIDEE, JSBACH4.0) or statistical approximations (e.g., LPJ-GUESS, SEIB-DGVM, CABLE-POP). VDMs could also vary in representing light competition within either multiple canopy layers (e.g., ED2, FATES, LM3-PPA, LPJ-GUESS, SEIB-DGVM) or a single canopy (e.g., JSBACH4.0, ORCHIDEE, CABLE-POP).
Powell et al. (2013) compared multiple VDMs and LSMs to interpret ecosystem responses to long-term droughts in the Amazon and are informative when conducting model–data comparisons, but studies of the cascade of ecosystem responses and mortality to UCEs are lacking. In a cutting-edge area of development, new mechanistic implementation of plant competition for water and plant hydraulics in VDMs (i.e., hydrodynamics) is improving our understanding of plant-water relations and stresses within plants, such as with TFSv.1-Hydro (Christoffersen et al., 2016), ED2-hydro (Xu et al., 2016) and FATES-HYDRO (Ma et al., 2021; Fang et al., 2022), compared to a more simplistic representation of a plant acquiring soil moisture not connected to plant physiology (e.g., LPJ-GUESS, LM3-PPA, CABLE-POP, SEIB-DGVM). For hydrodynamic representations in “big-leaf” LSMs such as CLM5, JULES, and Noah-MP-PHS see Kennedy et al. (2019), Eller et al. (2020), and Li et al. (2021) respectively.
The Discussion section provides a deeper investigation of model response to UCEs related to droughts. An exhaustive review of all VDMs and all plant processes is too large to be done here. Existing review papers of different VDM developments, processes, and uncertainties can be found in Fisher et al. (2018), Bonan (2019), Trugman et al. (2019), Hanbury-Brown et al. (2022), and Bugmann and Seidl (2022), as well as, specifically related to plant hydraulics, Mencuccini et al. (2019) and Anderegg and Venturas (2020). We use LPJ-GUESS and ED2 as example VDMs in an initial guide framework to explore hypotheses around vegetation mortality and severity index from UCEs and climate change impacts and highlight limiting model processes. Since field data needed to evaluate UCE responses are, by definition, unavailable, we do not perform model–data comparisons. Rather, we use the model results and conceptual framework as a road map to explore our hypotheses and illustrate their implications for ecosystem responses under UCEs, not historical drought events.
2.1 LPJ-GUESS and ED2 model descriptions
We explored our hypotheses at forested ecosystems in Australia and Central America using two VDMs: the Lund–Potsdam–Jena General Ecosystem Simulator (LPJ-GUESS) (Smith et al., 2001) version 3.0 (Smith et al., 2014) and the Ecosystem Demography model 2 (ED2) (Medvigy et al., 2009; Medvigy and Moorcroft, 2012). Both LPJ-GUESS and ED2 resolve vegetation into tree cohorts characterized by their PFT, in addition to age class in LPJ-GUESS and size and stem number density in ED2. Both models are driven by external environmental drivers (e.g., temperature, precipitation, solar radiation, atmospheric CO2 concentration, nitrogen deposition) and soil properties (soil texture, depth, etc.) and also depend on dynamic ecosystem state, which includes light attenuation, soil moisture, and soil nutrient availability. Establishment and growth of PFTs, and their carbon, nitrogen, and water cycles, are simulated across multiple patches per grid cell to account for landscape heterogeneity. Both models characterize PFTs by physiological and bioclimatic parameters, which vary between the models (Smith et al., 2001, 2014; Medvigy et al., 2009; Medvigy and Moorcroft, 2012).
The LPJ-GUESS includes three woody PFTs: evergreen, intermediate evergreen, and deciduous PFTs. Mortality in LPJ-GUESS is governed by a “growth-efficiency”-based function (kg C m−2 leaf yr−1), which captures the effects of water deficit, shading, heat stress, and tree size on plant productivity relative to its resource-uptake capacity (leaf area), with a threshold below which stress-related mortality risk increases markedly, in addition to background senescence and exogenous disturbances. Stress mortality can be reduced by plants using labile carbon storage, modeled implicitly using a “C debt” approach, which buffers low productivity, enhancing resilience to milder extremes (more details are given in Sect. 4.1.4). Total mortality can thus be impacted by variation in environmental conditions such as water limitation, low light conditions, and nutrient constraints, as well as current stand structure (Smith et al., 2001; Hickler et al., 2004).
The ED2 version used here (Xu et al., 2016) includes four woody PFTs: evergreen, intermediate evergreen, deciduous, brevi-deciduous, and deciduous stem succulent. This ED2 version includes coupled photosynthesis, plant hydraulics, and soil hydraulic modules (Xu et al., 2016), which together determine plant-water stress. The plant hydraulics module tracks water flow along a soil–plant–atmosphere continuum, connecting leaf water potential, stem sap flow, and transpiration, thus influencing controls on photosynthetic capacity, stomatal closure, phenology, and mortality. Leaf water potential depends on time-varying environmental conditions as well as time-invariant PFT traits. Leaf shedding is triggered when leaf water potential falls below the turgor loss point (a PFT trait) for a sufficient amount of time. Leaf flushing occurs when stem water potential remains high (above half of the turgor loss point) for a sufficient time (see Xu et al., 2016, for details). PFTs differ in their hydraulic traits, wood density, specific leaf area, allometries, rooting depth, and other traits. Stress-based mortality in the ED2 version used here includes two main physiological pathways in our current understanding of drought mortality (McDowell et al., 2013): C starvation and hydraulic failure. Mortality due to C starvation in ED2 results from a reduction of C storage, a proxy for non-structural carbohydrate (NSC) storage, which integrates the balance of photosynthetic gain and maintenance cost under different levels of light and moisture availability. Mortality due to hydraulic failure in ED2 is based on the percentage loss of stem conductivity. ED2 also includes a density-independent senescence mortality rate based on wood density.
2.2 Modeling guide
To exemplify how VDMs can be tools to explore new hypotheses related to UCEs, we applied the models at two field sites that were chosen due to being extensively studied, and the models used here have already been run at these sites and previously benchmarked against field data (see Xu et al., 2016; Medlyn et al., 2016; Medvigy et al., 2019, for model–data validation). The purpose of this paper was not to do a large multi-site comparison but rather to just select a few for hypothesis testing. In addition, the two sites span a range of vegetation types and are in warm, seasonally dry climates that are more likely to experience droughts in the future (Allen et al., 2017). The first is a mature eucalyptus (E. tereticornis) warm-temperate–subtropical transitional forest that is the site of the Eucalyptus Free Air CO2 enrichment (EucFACE) experiment in western Sydney, Australia (Medlyn et al., 2016; Ellsworth et al., 2017; Jiang et al., 2020). The second site is a seasonally dry tropical forest in the Parque Nacional Palo Verde in Costa Rica (Powers et al., 2009). Site description details can be found in the Supplement, in Sect. S1.
We performed a 100-year “baseline” simulation for each model at each site driven by constant near-ambient atmospheric CO2 (400 ppm) and recycled historical site-specific climate data (1992–2011 for EucFACE and 1970–2012 for Palo Verde; Sheffield et al., 2006) in the absence of drought treatments. A detailed description of the meteorological data and initial conditions used to drive the models is in Sect. S1. The two models were previously tuned for each site (Xu et al., 2016; Medlyn et al., 2016), and no additional site-level parameter tuning was conducted here due to evaluating responses from hypothetical UCEs. To describe the ecosystem impact of UCEs, we simulated 10 years of pre-drought conditions (continuing from the baseline simulation), followed by drought treatments that differed in intensity and duration, and followed by a 100-year post-drought recovery period. To explore the effects of drought intensity, we conducted 20 different artificial drought intensity simulations, in which precipitation during the whole year is reduced by 5 % to 100 % of its original amount in increments of 5 %. To explore the effects of drought duration, the 20 different drought intensities are maintained over 1, 2, and 4 years (Table S2). We examined model responses of aboveground biomass, leaf area index (LAI), stem density (number ha−1), plant-available soil water (mm), plant C storage (kg C m−2), change in stem mortality rate (yr−1), and PFT composition.
To explore how temperature, eCO2 concentration, and UCE droughts influence forest C dynamics individually and in combination, we implemented the following five experimental scenarios, some realistic and others hypothetical, for each model (Table S2): increased temperature only (+2 K over ambient), two experiments with eCO2 only (600 and 800 ppm), and two experiments with both increased temperature and eCO2 (+2 K 600 ppm; +2 K 800 ppm). Temperature and eCO2 manipulations were applied as step increases over the baseline conditions and are artificial scenarios as opposed to model-generated climate projections.
2.3 Linking concepts, hypotheses, and model outcomes
To relate our simulation results to Fig. 1a, we compared the total biomass loss as a result of each drought treatment by calculating the percentage of biomass reduction at the end of the drought period relative to the baseline (no drought) simulation. To explicitly consider biomass recovery rates over time, we calculated the severity-drought index (Eqs. 1–3), as a result of drought under the current climate, which is determined based on the concepts in Fig. 1b. We defined severity-drought index as the time-integrated carbon in biomass that is lost due to drought relative to what the vegetation would have stored in the absence of drought. That is, it is the difference between biomass in the presence of drought (Bd) at time (t) and biomass in the baseline simulation (no drought; Bbase), integrated over a defined recovery time period (in kg C m−2 yr):
To define the bounds of integration, in Eq. (1), t1 is defined as the time when the maximum amount of plant C is lost as a result of the drought:
Then, t2 is defined implicitly as the time when 50 % of the lost biomass has been recovered compared to the baseline:
since all severity-drought index results are taken as the difference from a non-drought baseline biomass (Bbase) and all droughts will result in a loss of C.
We also use the severity-drought index as a starting point to examine the role of drought, temperature, and eCO2 change for moderating or exacerbating the impacts of drought on forest C stocks, i.e., to evaluate the hypotheses illustrated in Fig. 1c. To assess these impacts of changing climates, we calculate a severity-climate index (Eq. 4), defined as the difference between the severity-drought index due to drought alone (Eqs. 1–3) under the present climate and the severity index due to the combined effects of drought and climate change (i.e., five scenarios of temperature increase and eCO2), still integrated over time to account for recovery:
Because we expect drought to reduce vegetation C stocks, and thus the severity-climate index to be negative, positive values of the severity-climate index indicate that changes in climatic drivers ameliorate the C losses from drought (i.e., buffering effects). Negative values of the severity-climate index indicate that the climate change scenario leads to either greater C losses or losses that persist for longer amounts of time (i.e., magnitude and/or duration) compared to a simulation with no climate change (i.e., “control” run).
As a basis for the treatment results presented here, we compared the baseline simulations (prior to drought or climate change treatments) of the two VDMs against observations and found strong model validation at both sites (Table S3, Fig. S1, Sect. S1 in the Supplement). These models are well-documented and investigated VDMs, with many studies that have looked into parameter uncertainty (see Sect. S1 for select references that explore model/parameter sensitivity).
The models displayed varied nonlinear responses to drought, differing substantially in their behavior and between sites. In general, ED2 shows sensitivity to drought duration (Hypothesis H1b), while LPJ-GUESS shows a stronger sensitivity to drought intensity (Hypothesis H1a). ED2's sensitivity to the duration of drought was mild at Palo Verde (Fig. 2a) and stronger at EucFACE, particularly during the 4-year drought, with a strong non-monotonic pattern (see explanation below) (Fig. 2b). When reporting only the percentage of biomass loss, ED2 predicts close to no UCE response at Palo Verde, with a maximum biomass reduction of only 40 % during 95 % precipitation removal and a 4-year drought event (i.e., UCE). LPJ-GUESS shows threshold-tipping patterns highly sensitive to drought intensity. The C loss predicted by LPJ-GUESS at Palo Verde reached a threshold at ∼ 65 % drought intensity, after which forests exhibit strong biomass losses up to 100 % (Fig. 2a). At the EucFACE site, both models predict a critical threshold of biomass loss at 35 %–45 % drought intensity, with LPJ-GUESS predicting total biomass loss (up to 100 %) after this drought intensity threshold (Fig. 2b). The EucFACE drought threshold is lower than that of the seasonally dry mixed tropical forest in Palo Verde.
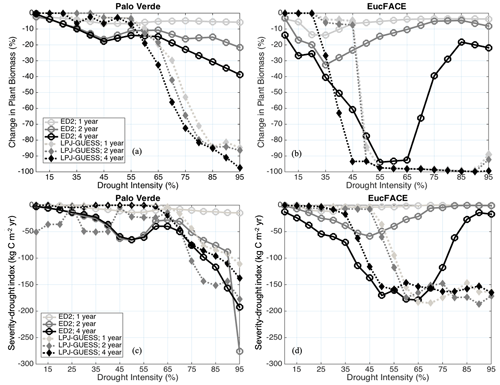
Figure 2Modeled change in biomass (%) at the end of drought periods of different lengths (1-, 2-, and 4-year droughts) and intensities (up to 95 % precipitation removed) at (a) Palo Verde and (b) EucFACE for the ED2 and LPJ-GUESS models. Modeled severity-drought index (C reduction due to extreme drought integrated over time until biomass recovers to 50 % of the non-drought baseline biomass) at (c) Palo Verde and (d) EucFACE.
With respect to C loss over a recovering time period (severity-drought index), the two models predict similar drought responses at Palo Verde (Fig. 2c) but not at EucFACE (Fig. 2d). At Palo Verde, the similarity between models in severity-drought index reflected longer biomass recovery time but less biomass loss in the short term in ED2 relative to LPJ-GUESS, which predicted greater biomass loss immediately after a drought but shorter recovery time. With the exception of the 1-year drought in ED2, both models predict a similar severity-drought index across a range of UCEs at Palo Verde via different pathways. The severity-drought index revealed an exacerbated response to drought duration in ED2, with drought durations greater than 1 year (Fig. 2c), compared to when only examining loss in biomass at the time of the event (Fig. 2a). The “V”-shaped patterns observed particularly in Fig. 2b arise from interactions between whole-leaf phenology and stomatal responses to drought in ED2. For drought intensities lower than 40 %, stomatal conductance is reduced but leaves are not fully shed. Leaf respiration continues, gradually depleting non-structural C pools, followed by a loss of biomass. However, for higher drought intensities, leaf water potentials quickly become systematically lower than leaf turgor loss points, and tree cohorts shed all their leaves. This strategy represents an immediate loss of C via leaf shedding but spares the cohort from slow, respiration-driven depletion of C stocks.
Predicted model responses to UCE droughts combined with increased temperature and/or eCO2
Relating to our second hypothesis of the additional effects of warming and eCO2, we tested 15 treatments in total, repeating the five climate change scenarios for each of the three drought durations. With the addition of climate change impacts, ED2 remained sensitive to the duration of drought, with warming negatively impacting severity-climate index most consistently during the 2- and 4-year drought durations. ED2 predicts that during the 2- and 4-year droughts at EucFACE losses are exacerbated when accompanied by warming, even with eCO2, with 600 ppm having a more detrimental impact than the more elevated 800 ppm (Fig. 3b–c). The average severity-climate index was −111.0 kg C m−2 yr across all 15 treatments (Table 2). Only during the 1-year drought duration did drought plus warming and eCO2 have a buffering effect on C stocks, seen in four out of our five scenarios but only during relatively modest drought intensities (Fig. 3a; i.e., positive severity-climate index, see also Table 2).
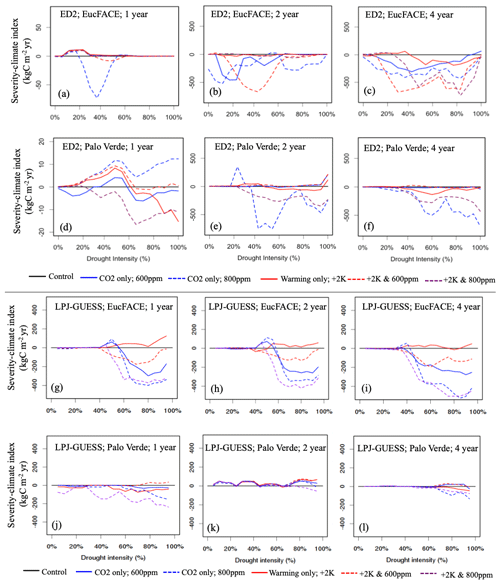
Figure 3Vegetation C response to interactions between drought intensity (0 % to 100 % precipitation reduction), drought durations (1, 2, 4-year droughts), and idealized scenarios of warming and eCO2 compared to the control simulation, simulated by two VDMs: ED2 (a–f) and LPJ-GUESS (g–l) at two sites (EucFACE and Palo Verde). The scenarios include a control (current temperature; 400 ppm atmospheric CO2), two eCO2 scenarios (600 or 800 ppm), elevated temperature (2 K above current), and a combination of eCO2 (600 or 800 ppm) and higher temperature. Vegetation response is quantified as severity-climate index (in kg C m−2 yr; Eq. 4), which is defined as the difference between severity-drought index (i.e., carbon loss due to only drought) and a given scenario of drought plus change in climatic drivers, relative to the control (i.e., no climate change). Negative values for severity-climate index indicate that warming and/or eCO2 leads to stronger C losses and/or longer recovery, while positive values for severity-climate index indicate a buffering effect.
Table 2Impact of eCO2 and/or temperature on the severity-climate index (kg C m−2 yr) relative to drought treatments with no additional warming or eCO2 for both models and both sites seen in Fig. 3. Quantified as the average and minimum severity-climate index across all 20 drought intensities for step-change scenarios of warming and eCO2. The percentage of each scenario that was negative in the severity-climate index (i.e., decreases in C loss). Bold values represent positive severity-climate index.
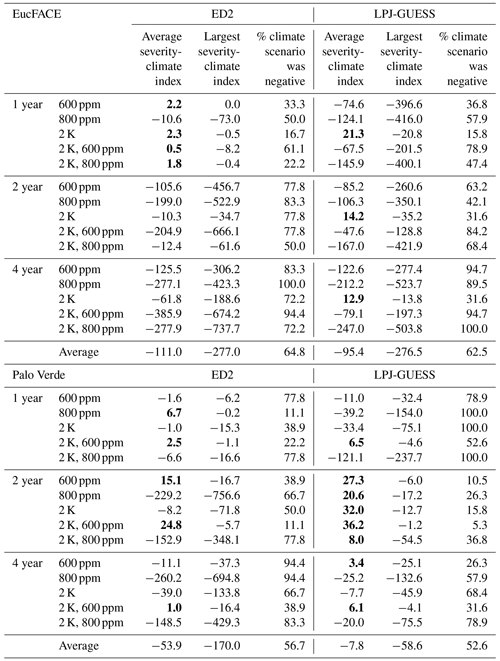
The ED2 simulations of the seasonally dry Palo Verde site (Fig. 3d–f) produced less-frequent negative impacts on drought and climate-change-driven C losses compared to EucFACE, with an average severity-climate index of −53.9 kg C m−2 yr across all 15 treatments (Table 2). During the 2-year drought, applying +2 K with eCO2 to 600 ppm showed a slight buffering effect to droughts and the most consistent positive severity-climate index (Fig. 3e; Table 2). Interestingly, an increase in only eCO2 to 800 ppm (no warming) when applied with the 2- and 4-year droughts resulted in the largest loss in carbon (Fig. 3e–f), larger than the expected “most severe” scenario, +2 K and 800 ppm.
Similar to ED2, the LPJ-GUESS model showed a nearly complete negative response in severity-climate index as a result of UCE drought and scenarios of warming and eCO2 at the EucFACE site (Fig. 3g–i) but showed mixed and more muted results at Palo Verde (Fig. 3j–l, Table 2). The average severity-climate index relative to the no climate change control case was −95.4 at EucFACE and −7.8 kg C m−2 yr at Palo Verde, both less negative compared to ED2. One notable pattern was that up until a drought intensity threshold of ∼ 40 %, the climate scenarios had no effect or response in severity-climate index at EucFACE and a muted response from warming and eCO2 at Palo Verde, compared to ED2. Surprisingly, the +2 K scenario switched the severity-climate index to positive, compared to the control case (Fig. 3g–i; red lines), which is potentially a physiological process in the model to increased temperatures only that signals an anomalous resiliency response. Similar to the results with no climate change, LPJ-GUESS remained sensitive to the intensity of drought, with ∼ 40 % precipitation reduction being a threshold.
When comparing the VDM responses to increasing drought severity and its interactions with warming and eCO2 (related to conceptual Fig. 1d), ED2 showed a more consistent MO response during UCEs with additional warming and eCO2 (Fig. 3; negative severity-climate index), especially at EucFACE, suggesting these ecosystems will remain in a depressed carbon condition driving vegetation mortality and/or longer recoveries. LPJ-GUESS produced more opportunities for SO with climate change. For example, at EucFACE CO2 fertilization created small SO periods that then led to MO with increasing drought severities, and at Palo Verde all +2 K and 600 ppm simulations led to an SO (Fig. 3j–l; Table 2).
Both models predicted that C losses due to drought interactions with increased temperature and eCO2 were less severe at the seasonally dry Palo Verde site compared to the somewhat less seasonal, more humid EucFACE site (Table 2), which could be attributed to higher diversity in PFT physiology at Palo Verde. Palo Verde's community composition that emerged following drought included either three (LPJ-GUESS) or four (ED2) PFTs, while only a single PFT existed at EucFACE. With rising temperatures under climate change, UCEs will be hotter and drier. A total of 9 out of the 12 simulations with both +2 K and 600 ppm CO2, and all but one +2 K and 800 ppm CO2, produced a negative severity-climate index, implying stronger C losses and/or longer recovery times when droughts are exacerbated by increasing temperatures (Table 2).
Vegetation demographic models (VDMs) allowed us to uniquely explore two hypotheses regarding a range of modeled responses of terrestrial ecosystems to unprecedented climate extremes (UCEs) and set the stage for the following perspectives to help guide future research. Key model results indicate strong differences in nonlinearities in C response to extreme drought intensities in LPJ-GUESS and, alternatively, drought durations in ED2 (at one of two sites), with differences in thresholds between the two models and ecosystems and only the ED2 model representing impacts from combined intensity and drought (hypothesis H1c). These nonlinearities may arise from multiple mechanisms that we begin to investigate here, including shifts in plant hydraulics or other functional traits, C allocation, phenology, stand size structure and/or age demography, and compositional changes, all of which vary among ecosystem types. A critical look at driving model mechanisms, which emerged from the hypothetical drought simulations used here, is summarized in Table 3. The models also show exacerbated biomass loss and recovery times in the majority of our scenarios of warming and eCO2, supporting hypothesis H2. Below, we discuss the underlying mechanisms that drive simulated ecosystem response to UCEs using the models and sites as conceptual “experimental tools” and observational evidence from the literature. We focus on two temporal stages of the UCE: the pre-drought ecosystem stage characterized as the quasi-stable state of the ecosystem prior to a UCE, which can mediate ecosystem resistance and disturbance impact, and the post-drought recovery stage (Table 1).
Table 3Summary of the suggested critical look at driving mechanisms (e.g., ecosystem or plant processes and state variables) which emerged from the hypothetical drought simulations used here to explore for future research in manipulation experiments, data collection, and model development and testing, as related to furthering our understanding of UCE resistance and recovery.
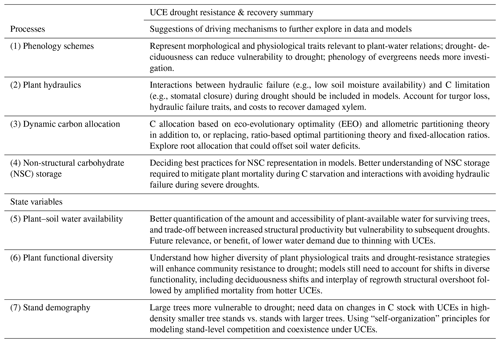
4.1 The role of ecosystem processes and states prior to UCEs
4.1.1 The role of phenology and phenological strategies prior to UCEs
Observations show that diversity of deciduousness contributes to successful alternative strategies for tropical forest response to water stress (Williams et al., 2008). For example, during the severe 1997 El Niño drought, brevi-deciduous trees and deciduous stem succulents within a tropical dry site in Guanacaste, Costa Rica, retained leaves during the extreme wet-season drought, behaving differently than during normal dry seasons (Borchert et al., 2002). Both models here predict that neither seasonal deciduousness nor drought-deciduous phenology at the seasonally dry tropical forest, Palo Verde (which consists of trees with different leaf phenological strategies), acts to buffer the forest from a large drop in LAI during UCEs (Fig. S1a–b). Even with this large decrease in LAI, ED2 predicted a very weak biomass loss at the time of UCEs (Fig. 2a), suggesting large-scale leaf loss is not a direct mechanism of plant mortality in ED2. Leaf loss is one component of total carbon turnover flux equations in terrestrial models, in addition to woody loss, fine roots, and reproductive tissues. Having a better understanding of when extreme levels of phenological turnover contribute to stand-level mortality could be improved. Among other turnover hypotheses explored, Pugh et al. (2020) found that phenological turnover fluxes where just as important as mortality fluxes in driving forest turnover time in the VDMs: LPJ-GUESS, CABLE-POP, and ORCHIDEE but not the LSM JULES. At the EucFACE site prior to the simulated extreme drought, LPJ-GUESS displayed strong inter-annual variability in LAI (Fig. S1a–b). This capability of large swings in LAI (5.8 to 0.8) by LPJ-GUESS could contribute to model uncertainty and the considerable mortality response at EucFACE. Modeled LAI was the largest source of variability in another ecosystem model, CABLE, when evaluating the simulated response to CO2 fertilization (Li et al., 2018). VDMs could be improved by better capturing different plant phenological responses to UCEs by better representing a range of leaf-level morphological and physiological characteristics relevant to plant-water relations such as leaf age, retention of young leaves even during extreme droughts (Borchert et al., 2002), and variation in hydraulic traits as a function of leaf habit (Vargas et al., 2021) (Table 3). Two such examples are seen in the FATES model, where the possibility for “trimming” the lowest leaf layer can occur when leaves are in negative carbon balance due to light limitation, thus optimizing maintenance costs and carbon gain, as well as leaf age classifications, providing variations in leaf productivity and turnover.
4.1.2 The role of plant hydraulics prior to UCEs
Susceptibility of plants to hydraulic stress is one of the strongest determinants of vulnerability to drought, with loss of hydraulic conductivity being a major predictor of drought mortality in temperate (McDowell et al., 2013; Anderegg et al., 2015; Sperry and Love, 2015; Venturas et al., 2021) and tropical forests (Rowland et al., 2015; Adams et al., 2017b), as well as a tractable mortality mechanism to represent in process-based models (Choat et al., 2018; Kennedy et al., 2019). Both LPJ-GUESS and ED2 exhibited a wide range in amount and pattern of plant-available water prior to drought (Fig. S1c–d), contributing to large differences in UCE response. LPJ-GUESS, which does not simulate hydrodynamics, predicted lower total plant-available water at both sites compared to ED2 and subsequently simulated greater mortality and a greater increase in plant-available water right after the UCEs as a result of less water demand. Due to ED2 using a static mortality threshold from conductivity loss (88 %), it likely does not accurately reproduce the wide range of observations of drought-induced mortality. In ED2, large trees with longer distances to transport water were at higher risk and suffered higher mortality (Fig. 4), demonstrating how stand demography, size structure, and tapering of xylem conduits can play an important role in ecosystem models (Petit et al., 2008; Fisher et al., 2018). Of the VDMs that are beginning to incorporate a continuum of hydrodynamics, e.g., ED2 (described in Methods, Sect. 2.1) and FATES-HYDRO (Fang et al., 2022, based on Christoffersen et al., 2016), they are able to solve for transient water from soils to roots, through the plant, and connect with transpiration demands. Therefore, instead of the plant-water stress function being based on soil water potentials, it is replaced with more realistic connections to leaf water potentials. Mortality is then caused by hydraulic failure via embolism controlled by the critical water potential (P50) that leads to 50 % loss of hydraulic conductivity. For advancements in tree-level hydrodynamic modeling see the FETCH3 model (Silva et al., 2022); for justification for plant hydrodynamics in conjunction with multi-layer vertical canopy profiles see Bonan et al. (2021). There are strong interdependencies and related mechanisms connecting both hydraulic failure (e.g., low soil moisture availability) and C limitation (e.g., stomatal closure) during drought (McDowell et al., 2008; Adams et al., 2017b), and these interactions should be incorporated into ecosystem modeling and further explored (Table 3).
4.1.3 The role of carbon allocation prior to UCEs
Plants have a variety of strategies to buffer vulnerability to water and nutrient stress caused by extreme droughts, such as allocating more C to deep roots (Joslin et al., 2000; Schenk and Jackson, 2005), investing in mycorrhizal fungi (Rapparini and Peñuelas, 2014), or reducing leaf area without shifting leaf nutrient content (Pilon et al., 1996). Alternatively, the presence of deep roots does not necessarily lead to deep soil moisture utilization, as seen in a 6-year Amazonian throughfall exclusion experiment where deep root water uptake was still limited, even with high volumetric water content (Markewitz et al., 2010). Elevated CO2 alone will enhance growth and water-use efficiency (Keenan et al., 2013), reducing susceptibility to drought. However, such increased productivity within a forest stand, and associated structural overshoot during favorable climate windows, can also be reversed by increased competition for light, nutrients, and water during unfavorable UCEs – potentially leading to mortality overshoot (Fig. 1d) and higher C loss. Mortality overshoot, as a result of structural overshoot, could be an explanation for the negative severity-climate index (i.e., C loss) in the majority of eCO2-only simulations (18 out of 24 scenarios; Table 2).
Effects of CO2 fertilization on plant C allocation strategies are uncertain. As a result, ecosystem models differ in their assumptions on controls of C allocation in response to eCO2, leading to divergent plant C use efficiencies (Fleischer et al., 2019). Global-scale terrestrial models are beginning to include optimal dynamic C allocation schemes, over fixed ratios, that account for concurrent environmental constraints on plants, such as water, and adjust allocation based on resource availability such as in LM3-PPA (Weng et al., 2015), but the representation of C allocation is still debated and progressing (De Kauwe et al., 2014; Montané et al., 2017; Reyes et al., 2017). Options for carbon allocation strategies can be based on the allometric partitioning theory (i.e., allocation follows a power allometry function between plant size and organs, which is insensitive to environmental conditions; Niklas, 1993) as an alternative to ratio-based optimal partitioning theory (i.e., allocation to plant organs based on the most limiting resources) (McCarthy and Enquist, 2007) or fixed ratios (Table 3), and the strategies should be further investigated, particularly due to VDMs' substantial use of allometric relationships. A meta-analysis of 164 studies found that allometric partitioning theory outperformed optimal partitioning theory in explaining drought-induced changes in C allocation (Eziz et al., 2017). Further eco-evolutionarily based approaches such as optimal response or game-theoretic optimization, as well as entropy-based approaches, are useful when wanting to simulate higher levels of complexity (reviewed in Franklin et al., 2012). With more frequent UCEs and the need for plants to reduce water consumption, a shift in the optimal strategy of allocation between leaves and fine roots should change. The goal functions (e.g., fitness proxy) used in optimal response modeling can account for these shifts in costs and benefits of allocation between all organs (Franklin et al., 2009, 2012).
4.1.4 The role of plant carbon storage prior to UCEs
Studies of neotropical and temperate seedlings show that pre-drought storage of non-structural carbohydrates (NSCs) provides the resources needed for growth, respiration osmoregulation, and phloem transport when stomata close during subsequent periods of water stress (Myers and Kitajima, 2007; Dietze and Matthes, 2014; O'Brien et al., 2014). Furthermore, direct correlations have been shown between NSC depletion and embolism accumulation and between the degree of pre-stress reserves and the utilization of soluble sugars (Tomasella et al., 2020). The amount of NSC storage required to mitigate plant mortality during C starvation and interactions with hydraulic failure from severe drought is difficult to quantify due to the many roles of NSCs in plant function and metabolism (Dietze and Matthes, 2014). For example, NSCs were not depleted after 13 years of experimental drought in the Brazilian Amazon (Rowland et al., 2015). As atmospheric CO2 increases with climate change, NSC concentrations may increase, as seen in manipulation experiments (Coley et al., 2002), but interactions with heat, water stress, enhanced leaf shedding, and nutrient limitation complicate this relationship and need to be further explored. Despite the recognition of the critical role that plant hydraulic functioning and NSCs play in tree resilience to extremes, knowledge gaps and uncertainties preclude fully incorporating these processes into ecosystem models.
Compared to ED2, LPJ-GUESS predicted low plant carbon storage (a model proxy for NSCs) prior to and during drought and at times became negative, thereby creating C costs (Fig. S2a–b), leading to C starvation and potentially explaining the larger biomass loss in LPJ-GUESS at both sites. Alternatively, ED2 maintained higher levels of NSCs, providing a buffer to stress and mitigating the negative effects of drought. Maintenance of NSCs in ED2 even during prolonged drought (at EucFACE) is due to (1) trees resorbing a fraction of leaf C during leaf shedding, (2) no maintenance costs for NSC storage in the current version, and (3) no allocation of NSCs to structural growth until NSC storage surpasses a threshold (the amount of C needed to build a full canopy of leaves and associated fine roots), allowing a buffer to accumulate. In LPJ-GUESS, accumulation and depletion of NSC are recorded as a C debt being paid back in later years. The contrasting responses of the two models to drought, and the likely role of NSCs in explaining differences in model behavior, highlight the need to better understand NSC dynamics and to accurately represent the relevant processes in models (Richardson et al., 2013; Dietze and Matthes, 2014). More observations of C accumulation patterns and how/where NSCs drive growth, respiration, transport, and cellular water relations would enable a more realistic implementation of NSC dynamics in models (Table 3).
4.1.5 Role of functional trait diversity prior to UCEs
Currently, LPJ-GUESS simulates the Palo Verde community using three PFTs, while ED2 uses four PFTs that differ in photosynthetic and hydraulic traits. The community composition simulated by ED2 is shown to be more resistant to UCEs compared to LPJ-GUESS (Fig. 5), perhaps due to relatively higher functional diversity (via more PFTs with additional phenological and hydraulic diversity). This additional diversity helps to buffer ecosystem response to drought by allowing more tolerant PFTs to benefit from reductions in less tolerant PFTs, thus buffering reductions in ecosystem function (Anderegg et al., 2018). Higher-diversity ecosystems were found to protect individual species from the negative effects of drought (Aguirre et al., 2021) and enhance productivity resilience following wildfire (Spasojevic et al., 2016); thus, functionally diverse communities may be key to enhancing tolerance to rising environmental stress.
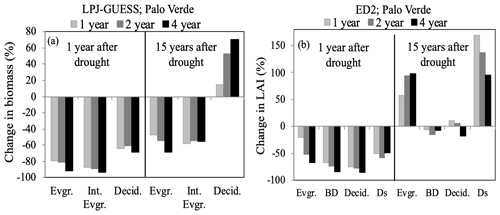
Figure 5Percent change in community composition, represented by plant functional type (PFT), the year following three drought durations of UCEs (1-, 2-, and 4-year droughts and 90 % precipitation removed) as well as 15 years after droughts for the tropical Palo Verde site by (a) LPJ-GUESS reported in biomass change and (b) ED2 reported in LAI change. Even though Ds had the strongest recovery, it should be noted it was the least abundant PFT at this site. Evgr. refers to evergreen, Int. Evgr. refers to intermediate evergreen, Decid. refers to deciduous, BD refers to brevi-deciduous, and Ds refers to deciduous stem succulent. The EucFACE data are not shown because only one PFT was present (evergreen tree).
Recent efforts to consolidate information on plant traits (Reich et al., 2007; Kattge et al., 2011) have contributed to identifying relationships that can impact community-level drought responses (Skelton et al., 2015; Anderegg et al., 2016a; Uriarte et al., 2016; Greenwood et al., 2017), such as life-history characteristics, and strategies of resource acquisition and conservation as predictors of ecosystem resistance (MacGillivray and Grime, 1995; Ruppert et al., 2015). While adding plant trait complexity in ESMs may be required to accurately simulate key vegetation dynamics, it necessitates more detailed parameterizations of processes that are not explicitly resolved (Luo et al., 2012). Further investigation of how VDMs represent interactions leading to functional diversity shifts is crucial to this issue. Enquist and Enquist (2011), as an example, show that long-term patterns of drought (20 years) have led to increases in drought-tolerant dry forest species, which could modulate resistance to future droughts. Higher diversity of plant physiological traits and drought-resistance strategies is expected to enhance community resistance to drought, and models should account for shifts in diverse functionality (Table 3).
4.2 The role of ecosystem processes and states in post-UCE recovery
4.2.1 The role of soil water resources post-UCEs
Our simulation results generally demonstrated a fast recovery of plant-available water and LAI at both sites (Fig. S1). Annual plant-available water substantially increased right after a drought by an average of 163 mm at Palo Verde and 213 mm at EucFACE in the LPJ-GUESS simulations compared to much lower increases in ED2 (50 and 12 mm at Palo Verde and EucFACE). This increase in available water post-drought can be attributed to reduced stand density and water competition (Fig. S2c–d; diamonds vs. circles), alleviating the demand for soil resources (water) and subsequent stress, which has also been shown in observations (McDowell et al., 2006; D'Amato et al., 2013). After large canopy tree mortality events, there can be relatively rapid recovery of forest biogeochemical and hydrological fluxes (Biederman et al., 2015; Anderegg et al., 2016b; Biederman et al., 2016). These crucial fluxes strongly influence plant regeneration and regrowth, which can buffer ecosystem vulnerability to future extreme droughts. However, this enhanced productivity has a limit. In a scenario where UCEs continue to intensify, causing greater reductions in soil water and reduced ecosystem recovery potential, the SO growth that typically occurs after UCEs may be dampened (Fig. 1d). In water-limited locations, similar to the dry forest sites used here, initial forest recovery from droughts was faster due to thinning-induced competitive release of the surviving trees and shallow roots not having to compete with neighboring trees for water, allowing for more effective water usage (Tague and Moritz, 2019), stressing the importance of root competition and distribution in models (Goulden and Bales, 2019). Tague and Moritz (2019) also reported that this increased water-use efficiency and SO ultimately led to water stress and related declines in productivity, similar to the MO concept (Jump et al., 2017; McDowell et al., 2006). Since a core strength of VDMs is predicting stand demography during recovery, improved quantification of density-dependent competition following stand dieback would be beneficial for model benchmarking (Table 3).
4.2.2 The role of lagged turnover and secondary stressors post-UCEs
Time lags in forest compositional response to and survival of drought could indicate community resistance or shifts to more competitive species and competitive exclusion. During a 15-year recovery period from extreme drought at Palo Verde, LPJ-GUESS predicted an increase in stem density (stems m2 yr−1) (Fig. S2c) compared to ED2, which predicted almost no impact in stem recovery. The mortality “spike” in ED2 due to drought was muted and slightly delayed, contributing to ED2's lower biomass loss and more stable behavior of plant processes over time at Palo Verde. At EucFACE, both models exhibited a pronounced lag effect in stem turnover response, i.e., ∼ 8–12 years after drought (Fig. S2d). After about a decade, strong recoveries and increased stem density occurred, which in ED2 was followed by delayed mortality/thinning of stems. Delayed tree mortality after droughts is common due to optimizing carbon allocation and growth (Trugman et al., 2018) but typically only up to several years post-drought, not a decade or more as seen in the model.
The versions of the VDMs used here do not directly consider post-drought secondary stressors such as infestation by insects or pathogens and the subsequent repair costs due to stress damage, which could substantially slow the recovery of surviving trees. Forest ecologists have long recognized the susceptibility of trees under stress, particularly drought, to insect attacks and pathogens (Anderegg et al., 2015). Tight connections between drought conditions and increased mountain pine beetle activity have been observed (Chapman et al., 2012; Creeden et al., 2014) and can ultimately lead to increased tree mortality (Hubbard et al., 2013). Leaf defoliation is a major concern from insect outbreaks following droughts and can have large impacts on C cycling, plant productivity, and C sequestration (Amiro et al., 2010; Clark et al., 2010; Medvigy et al., 2012). Implementing these secondary stressors in models could slow the rate of post-UCE recovery and lead to increased post-UCEs tree mortality. Additional background on secondary disturbances, lag effects, and repeated extremes can be found in Sect. S2 in the Supplement.
4.2.3 The role of stand demography post-UCEs
Change in stand structure is an important model process to capture because large trees have important effects on C storage, community resource competition, and hydrology (Wullschleger et al., 2001) (Table 3), and maintaining a positive carbohydrate balance is beneficial to sustaining (or repairing) hydraulic viability (McDowell et al., 2011). There is increasing evidence, both theoretical (McDowell and Allen, 2015) and empirical (Bennett et al., 2015; Rowland et al., 2015; Stovall et al., 2019), that large trees (particularly tall trees with high leaf area) contribute to the dominant fraction of dead biomass after drought events. Under rising temperatures (and decreasing precipitation), vapor pressure deficit (VPD) will increase, leading to a higher likelihood of large-tree death (Eamus et al., 2013; Stovall et al., 2019), driving MO events as hypothesized in Fig. 1d. Consistent with this expectation, ED2 predicted that the largest trees (>100 cm) experienced the largest decreases in basal area compared to all other size classes (Fig. 4). This drought-induced partial dieback and mortality of large dominant trees have substantial impacts on community-level C dynamics, as long-term sequestered C is liberated during the decay of new deadwood (Palace et al., 2008; Potter et al., 2011). In ED2, the intermediate size class (60–80 cm) increased in basal area following large-tree death, taking advantage of the newly open canopy space. However, small size classes do not necessarily benefit from canopy dieback. For example, in a dry tropical forest, prolonged drought led to a decrease in understory species and small-sized stems (Enquist and Enquist, 2011).
Due to VDMs being able to exhibit dynamic biogeography, they are more useful at predicting shifts in community composition beyond LSMs capabilities. Further areas of advancement (described in Franklin et al., 2020) include models of natural selection, self-organization, and entropy maximization, which can substantially improve community dynamic responses in varying environments such as UCEs. Eco-evolutionary optimality (EEO) theory can also help improve functional trait representation in global process-based models (reviewed in Harrison et al., 2021) through hypotheses in plant trait trade-offs and mechanistic links between processes such as resource demand, acquisition, and a plant's competitiveness and survival, traits associated with high degrees of sensitivity in models. The power of prognostic VDMs to predict shifts in demography and community migration with climate change is large but is rarely being constrained with plant-level EEO theory and thus will likely need to use stand-level competition and coexistence principles of how plants self-organize (Franklin et al., 2020).
4.2.4 The role of functional trait diversity and plant hydraulics post-UCEs
In field experiments, higher disturbance rates have shifted the recovery trajectory and competition of the plant community towards one that is composed of opportunistic fast-growing pioneer tree species, grasses (Shiels et al., 2010; Carreño-Rocabado et al., 2012), and/or deciduous species, as also seen in the model results (Hickler et al., 2004). In the treatments presented here, deciduous PFTs were also the strongest to recover after 15 years in both models, surpassing pre-drought values (Fig. 5). It should be noted that ED2 exhibited a strong recovery in the evergreen PFT as well, inconsistent with the above literature (Fig. 5b). PFTs in ED2 respond to drought conditions via stomatal closure and leaf shedding, buffering stem water potentials from falling below a set mortality threshold (i.e., 88 % of loss in conductivity). This conductivity threshold may need to be reconsidered if further examination reveals an unrealistic advantage under drought conditions for evergreen trees, which exhibited a lower impact from droughts (compared to deciduous and brevi-deciduous PFTs) in ED2. Nitrogen cycling feedbacks were not investigated here but could also be an explanation for a strong evergreen PFT recovery.
Recovery of surviving trees could be hindered by the high cost of replacing damaged xylem associated with cavitation (McDowell et al., 2008; Brodribb et al., 2010). Many studies have identified “drought legacy” effects of delayed growth or gross primary productivity following a drought (Anderegg et al., 2015; Schwalm et al., 2017), and the magnitude of these legacies across species correlates with the hydraulic risks taken during a drought itself (Anderegg et al., 2015). The conditions under which xylem can be refilled remain controversial, but it seems likely that many species, particularly gymnosperms, may need to entirely replace damaged xylem (Sperry et al., 2002), and trees worldwide operate within narrow hydraulic safety margins, suggesting that trees in all biomes are vulnerable to drought (Choat et al., 2012). The amount of damaged xylem from a given drought event and recovery rates also vary across trees of different sizes (Anderegg et al., 2018).
Plasticity in nutrient acquisition traits, intraspecific variation in plant hydraulic traits (Anderegg et al., 2015), and changes in allometry (e.g., Huber values) can have large effects on acclimation to extreme droughts. This suggests some capacity for physiological adaptation to extreme drought, as seen by short-term negative effects from drought and heat extremes being compensated for in the longer term (Dreesen et al., 2014). Still, given the shift towards more extreme droughts with climate change, vegetation mortality thresholds are likely to be exceeded, as reported in Amazonian long-term plots where mortality of wet-affiliated genera has increased, while simultaneously new recruits of dry-affiliated genera are also increasing (Esquivel-Muelbert et al., 2019). Increasing occurrences of heat events, water stress, and high VPD will lead to extended closure of stomata to avoid cavitation, progressively reducing CO2 enrichment benefits (Allen et al., 2015). Where CO2 fertilization has been seen to partially offset the risk of increasing temperatures, the risk response was mediated by plant hydraulic traits (Liu et al., 2017) using a soil–plant–atmosphere continuum (SPAC) model, yet interactions with novel extreme droughts were not considered. The VDM simulations suggest that the combination of elevated warming and potential structural overshoot from eCO2 (or inaccurate representation in NSC allocation/usage priority) will exacerbate consequences of UCEs by reductions in both C stocks and post-drought biomass recovery speeds (Fig. 3). Therefore, future UCE recovery may not be easily predicted from observations of historical post-disturbance recovery. An associated area for further investigation is to better understand the hypothesized interplay between amplified mortality from hotter UCEs followed by structural overshoot regrowth during wetter periods (Fig. 1d), which could potentially lead to continual large swings in MO and SO and vulnerable net ecosystem C fluxes through time (Table 3).
Model limitations and unknowns exposed by our simulations and literature review highlight current challenges in our ability to understand and forecast UCE effects on ecosystems. These limitations reflect a general lack of empirical experiments focused on UCEs. Insufficient data means that relevant processes may currently be poorly represented in models, and models may then misrepresent C losses during UCEs. The two VDMs used here had different sensitivities to drought duration or intensity, and CO2 and warming interactions, indicating the wide variety of unknowns and plausible options when trying to represent future UCEs that still need to be narrowed down (Fig. 1d). These model uncertainties could potentially be addressed by improved datasets on thresholds of conductivity loss at high drought intensities, the role of trait diversity (e.g., different strategies of drought deciduousness and EEO theory) in buffering ecosystem drought responses, and a better grasp of allocation to plant C storage stocks before, during, and after multi-year droughts. Our study takes some initial steps to identify and assess model gaps in terms of mechanisms and magnitudes of responses to UCEs, which can then be used to inform and develop field experiments targeting key knowledge gaps as well as to prioritize ongoing model development (Table 3). Our intention was not to create an exhaustive list of UCE simulation experiments, and additional modeling perturbations and experiments would be useful outcomes of future studies. For example, we began to investigate the duration of droughts, but we did not consider the frequency of back-to-back UCEs. Using VDMs as hypothesis testing tools offers strong potential to drive progress in improving our understanding of terrestrial ecosystem responses to UCEs and climate feedbacks, while informing the development of the next generation of models.
The source code for the ED2 model can be downloaded and is available publicly at https://github.com/EDmodel/ED2 (last access: 27 May 2023; https://doi.org/10.5281/zenodo.3978588, Xu et al., 2020). The source code for the LPJ-GUESS model version 3.0 can be downloaded and is available publicly at http://web.nateko.lu.se/lpj-guess/download.html (Smith and Mishurov, 2023).
The authors received the required permissions to use the site-level meteorological data from the EucFACE site used in this study. Data came from https://doi.org/10.1111/gcb.13268 (Medlyn et al., 2016). Meteorological data for the Palo Verde site came from re-analysis data from the Princeton Global Forcing dataset (Sheffield et al., 2006). Otherwise, no ecological or biological data were used in this study.
The supplement related to this article is available online at: https://doi.org/10.5194/bg-20-2117-2023-supplement.
JAH wrote the article with significant contributions from AR, BS, JSD, and DMM and with input and contributions from all authors. XX and MM were the primary leads running the model simulations, with model assistance and strong feedback from DMM and BS. All authors made contributions to this article and agreed to the submission.
The contact author has declared that none of the authors has any competing interests.
Publisher’s note: Copernicus Publications remains neutral with regard to jurisdictional claims in published maps and institutional affiliations.
This article is part of the special issue “Ecosystem experiments as a window to future carbon, water, and nutrient cycling in terrestrial ecosystems”. It is not associated with a conference.
We thank Belinda Medlyn and David Ellsworth of the Hawkesbury Institute for the Environment, Western Sydney University, for providing the meteorological forcing data series for the EucFACE site, a facility supported by the Australian Government through the Education Investment Fund and the Department of Industry and Science, in partnership with Western Sydney University.
Funding for the meetings that facilitated this work was provided by NSF-DEB-0955771: An Integrated Network for Terrestrial Ecosystem Research on Feedbacks to the Atmosphere; ClimatE (INTERFACE): Linking experimentalists, ecosystem modelers, and Earth System modelers, hosted by Purdue University; and Climate Change Manipulation Experiments in Terrestrial Ecosystems: Networking and Outreach (COST action ClimMani – ES1308), led by the University of Copenhagen. Jennifer A. Holm's time was supported as part of the Next-Generation Ecosystem Experiments–Tropics, funded by the U.S. Department of Energy, Office of Science, Office of Biological and Environmental Research under contract DE-AC02-05CH11231. Anja Rammig received funding from the CLIMAX Project funded by Belmont Forum and the German Federal Ministry of Education and Research (BMBF). Benjamin Smith and Mikhail Mishurov received support from the Strategic Research Area MERGE. William R. L. Anderegg received funding from the University of Utah Global Change and Sustainability Center, NSF grant 1714972, and the USDA National Institute of Food and Agriculture, Agricultural and Food Research Initiative Competitive Programme, Ecosystem Services and Agro-ecosystem Management, grant no. 2018-67019-27850. Jeremy W. Lichstein received support from the Northern Research Station of the USDA Forest Service (agreement 16-JV-11242306-050) and a sabbatical fellowship from sDiv, the Synthesis Centre of iDiv (DFG FZT 118, 202548816). Craig D. Allen received support from the USGS Land Change Science R&D Program.
This paper was edited by Sönke Zaehle and reviewed by Hisashi Sato and three anonymous referees.
Adams, H. D., Guardiola-Claramonte, M., Barron-Gafford, G. A., Villegas, J. C., Breshears, D. D., Zou, C. B., Troch, P. A., and Huxman, T. E.: Temperature sensitivity of drought-induced tree mortality portends increased regional die-off under global-change-type drought, P. Natl. Acad. Sci. USA, 106, 7063–7066, 2009.
Adams, H. D., Barron-Gafford, G. A., Minor, R. L., Gardea, A. A., Bentley, L. P., Law, D. J., Breshears, D. D., McDowell, N. G., and Huxman, T. E.: Temperature response surfaces for mortality risk of tree species with future drought, Environ. Res. Lett., 12, 115014, https://doi.org/10.1088/1748-9326/aa93be, 2017a.
Adams, H. D., Zeppel, M. J. B., Anderegg, W. R. L., et al.: A multi-species synthesis of physiological mechanisms in drought-induced tree mortality, Nature Ecol. & Evol., 1, 1285–1291, 2017b.
Aguirre, B. A., Hsieh, B., Watson, S. J., and Wright, A. J.: The experimental manipulation of atmospheric drought: Teasing out the role of microclimate in biodiversity experiments, J. Ecol., 109, 1986–1999, https://doi.org/10.1111/1365-2745.13595, 2021.
Ahlström, A., Schurgers, G., Arneth, A., and Smith, B.: Robustness and uncertainty in terrestrial ecosystem carbon response to CMIP5 climate change projections, Environ. Res. Lett., 7, 044008, https://doi.org/10.1088/1748-9326/7/4/044008, 2012.
Ainsworth, E. A. and Long, S. P.: What have we learned from 15 years of free-air CO2 enrichment (FACE)? A meta-analytic review of the responses of photosynthesis, canopy properties and plant production to rising CO2, New Phytol., 165, 351–372, 2005.
Allen, C. D., Breshears, D. D., and McDowell, N. G.: On underestimation of global vulnerability to tree mortality and forest die-off from hotter drought in the Anthropocene, Ecosphere, 6, 129, https://doi.org/10.1890/ES15-00203.1, 2015.
Allen, K., Dupuy, J. M., Gei, M. G., Hulshof, C. M., Medvigy, D., Pizano, C., Salgado-Negret, B., Smith, C. M., Trierweiler, A., Van Bloem, S. J., Waring, B. G., Xu, X., and Powers J. S.: Will seasonally dry tropical forests be sensitive or resistant to future changes in rainfall regimes?, Environ. Res. Lett., 12, 023001, https://doi.org/10.1088/1748-9326/aa5968, 2017.
Amiro, B. D., Barr, A. G., Barr, J. G., Black, T. A., Bracho, R., Brown, M., Chen, J., Clark, K. L., Davis, K. J., Desai, A. R., Dore, S., Engel, V., Fuentes, J. D., Goldstein A. H., Goulden M. L., Kolb, T. E., Lavigne, M. B., Law, B. E., Margolis, H. A., Martin, T., McCaughey, J. H., Misson, L., Montes-Helu, M., Noormets, A., Randerson, J. T., Starr, G., and Xiao, J.: Ecosystem carbon dioxide fluxes after disturbance in forests of North America, J. Geophys. Res.-Biogeo., 115, G00K02, https://doi.org/10.1029/2010JG001390, 2010.
Anderegg, W. R. L. and Venturas, M. D.: Plant hydraulics play a critical role in Earth system fluxes, New Phytol., 226, 1535–1538, https://doi.org/10.1111/nph.16548, 2020.
Anderegg, W. R. L., Hicke, J. A., Fisher, R. A., Allen, C. D., Aukema, J., Bentz, B., Hood, S., Lichstein, J. W., Macalady, A. K., McDowell, N., Pan, Y., Raffa, K., Sala, A., Shaw, J. D., Stephenson, N. L., Tague, C., and Zeppel, M.: Tree mortality from drought, insects, and their interactions in a changing climate, New Phytol., 208, 674–683, 2015.
Anderegg, W. R. L., Klein, T., Bartlett, M., Sack, L., Pellegrini, A. F. A., Choat, B., and Jansen, S.: Meta-analysis reveals that hydraulic traits explain cross-species patterns of drought-induced tree mortality across the globe, P. Natl. Acad. Sci. USA, 113, 5024–5029, 2016a.
Anderegg, W. R. L., Martinez-Vilalta, J., Cailleret, M., Camarero, J. J., Ewers, B. E., Galbraith, D., Gessler, A., Grote, R., Huang, C.-Y., Levick, S. R., Powell, T. L., Rowland, L., Sanchez-Salguero, R., and Trotsiuk, V.: When a Tree Dies in the Forest: Scaling Climate-Driven Tree Mortality to Ecosystem Water and Carbon Fluxes, Ecosystems, 19, 1133–1147, 2016b.
Anderegg, W. R. L., Konings, A. G., Trugman, A. T., Yu, K., Bowling, D. R., Gabbitas, R., Karp, D. S., Pacala, S., Sperry, J. S., Sulman, B. N., and Zenes, N.: Hydraulic diversity of forests regulates ecosystem resilience during drought, Nature, 561, 538–541, 2018.
Arora, V. K., Katavouta, A., Williams, R. G., Jones, C. D., Brovkin, V., Friedlingstein, P., Schwinger, J., Bopp, L., Boucher, O., Cadule, P., Chamberlain, M. A., Christian, J. R., Delire, C., Fisher, R. A., Hajima, T., Ilyina, T., Joetzjer, E., Kawamiya, M., Koven, C. D., Krasting, J. P., Law, R. M., Lawrence, D. M., Lenton, A., Lindsay, K., Pongratz, J., Raddatz, T., Séférian, R., Tachiiri, K., Tjiputra, J. F., Wiltshire, A., Wu, T., and Ziehn, T.: Carbon–concentration and carbon–climate feedbacks in CMIP6 models and their comparison to CMIP5 models, Biogeosciences, 17, 4173–4222, https://doi.org/10.5194/bg-17-4173-2020, 2020.
Asner, G. P., Brodrick, P. G., Anderson, C. B., Vaughn, N., Knapp, D. E., and Martin, R. E.: Progressive forest canopy water loss during the 2012–2015 California drought, P. Natl. Acad. Sci. USA, 113, E249–E255, 2016.
Beier, C., Beierkuhnlein, C., Wohlgemuth, T., Penuelas, J., Emmett, B., Körner, C., de Boeck, H., Christensen, J. H., Leuzinger, S., Janssens, I. A., and Hansen, K.: Precipitation manipulation experiments – challenges and recommendations for the future, Ecol. Lett., 15, 899–911, 2012.
Bennett, A. C., McDowell, N. G., Allen, C. D., and Anderson-Teixeira, K. J.: Larger trees suffer most during drought in forests worldwide, Nat. Plants, 1, 15139, https://doi.org/10.1038/nplants.2015.139, 2015.
Biederman, J. A., Somor, A. J., Harpold, A. A., Gutmann, E. D., Breshears, D. D., Troch, P. A., Gochis, D. J., Scott, R. L., Meddens, A. J. H., and Brooks, P. D.: Recent tree die-off has little effect on streamflow in contrast to expected increases from historical studies, Water Resour. Res., 51, 9775–9789, 2015.
Biederman, J. A., Meixner, T., Harpold, A. A., Reed, D. E., Gutmann, E. D., Gaun, J. A., and Brooks, P. D.: Riparian zones attenuate nitrogen loss following bark beetle-induced lodgepole pine mortality, J. Geophys. Res.-Biogeo., 121, 933–948, 2016.
Blyth, E. M., Arora, V. K., Clark, D. B., Dadson, S. J., De Kauwe, M. G., Lawrence, D. M., Melton, J. R., Pongratz, J., Turton, R. H., Yoshimura, K., and Yuan, H.: Advances in Land Surface Modelling, Curr. Clim. Change Rep., 7, 45–71, https://doi.org/10.1007/s40641-021-00171-5, 2021.
Bonan, G.: Vegetation Demography, in: Climate Change and Terrestrial Ecosystem Modeling, 1st Edn., Cambridge, Cambridge University Press, 344–364, https://doi.org/10.1017/9781107339217.020, 2019.
Bonan, G. B., Patton, E. G., Finnigan, J. J., Baldocchi, D. D., and Harman, I. N.: Moving beyond the incorrect but useful paradigm: reevaluating big-leaf and multilayer plant canopies to model biosphere-atmosphere fluxes – a review, Agr. Forest Meteorol., 306, 108435, https://doi.org/10.1016/j.agrformet.2021.108435, 2021.
Borchert, R., Rivera, G., and Hagnauer, W.: Modification of Vegetative Phenology in a Tropical Semi-deciduous Forest by Abnormal Drought and Rain, Biotropica, 34, 27–39, 2002.
Brando, P. M., Paolucci, L., Ummenhofer, C. C., Ordway, E. M., Hartmann, H., Cattau, M. E., Rattis, L., Medjibe, V., Coe, M. T., and Balch, J.: Droughts, Wildfires, and Forest Carbon Cycling: A Pantropical Synthesis, Annu. Rev. Earth Pl. Sc., 47, 555—581, 2019.
Breshears, D. D., Myers, O. B., Meyer, C. W., Barnes, F. J., Zou, C. B., Allen, C. D., McDowell, N. G., and Pockman, W. T.: Tree die-off in response to global change-type drought: mortality insights from a decade of plant water potential measurements, Front. Ecol. Environ., 7, 185–189, 2009.
Brodribb, T. J., Bowman, D. J. M. S., Nichols, S., Delzon, S., and Burlett, R.: Xylem function and growth rate interact to determine recovery rates after exposure to extreme water deficit, New Phytol., 188, 533–542, 2010.
Bugmann, H. and Seidl, R.: The evolution, complexity and diversity of models of long-term forest dynamics, J. Ecol., 110, 2288–2307, https://doi.org/10.1111/1365-2745.13989, 2022.
Carreño-Rocabado, G., Peña-Claros, M., Bongers, F., Alarcón, A., Licona, J.-C., and Poorter, L.: Effects of disturbance intensity on species and functional diversity in a tropical forest, J. Ecol., 100, 1453–1463, 2012.
Chapman, T. B., Veblen, T. T., and Schoennagel, T.: Spatiotemporal patterns of mountain pine beetle activity in the southern Rocky Mountains, Ecology, 93, 2175–2185, 2012.
Chiang, F., Mazdiyasni, O., and AghaKouchak, A.: Evidence of anthropogenic impacts on global drought frequency, duration, and intensity, Nat. Commun., 12, 2754, https://doi.org/10.1038/s41467-021-22314-w, 2021.
Choat, B., Jansen, S., Brodribb, T. J., Cochard, H., Delzon, S., Bhaskar, R., Bucci, S. J., Feild, T. S., Gleason, S. M., Hacke, U. G., Jacobsen, A. L., Lens, F., Maherali, H., Martínez-Vilalta, J., Mayr, S., Mencuccini, M., Mitchell, P. J., Nardini, A., Pittermann, J., Pratt, R. B., Sperry, J. S., Westoby, M., Wright, I. J., and Zanne, A. E.: Global convergence in the vulnerability of forests to drought, Nature, 491, 752–755, 2012.
Choat, B., Brodribb, T. J., Brodersen, C. R., Duursma, R. A., López, R., and Medlyn, B. E.: Triggers of tree mortality under drought, Nature, 558, 531–539, 2018.
Christoffersen, B. O., Gloor, M., Fauset, S., Fyllas, N. M., Galbraith, D. R., Baker, T. R., Kruijt, B., Rowland, L., Fisher, R. A., Binks, O. J., Sevanto, S., Xu, C., Jansen, S., Choat, B., Mencuccini, M., McDowell, N. G., and Meir, P.: Linking hydraulic traits to tropical forest function in a size-structured and trait-driven model (TFS v.1-Hydro), Geosci. Model Dev., 9, 4227–4255, https://doi.org/10.5194/gmd-9-4227-2016, 2016.
Ciais, P., Reichstein, M., Viovy, N., Granier, A., Ogée, J., Allard, V., Aubinet, M., Buchmann, N., Bernhofer, Chr., Carrara, A., Chevallier, F., De Noblet, N., Friend, A. D., Friedlingstein, P., Grünwald, T., Heinesch, B., Keronen, P., Knohl, A., Krinner, G., Loustau, D., Manca, G., Matteucci, G., Miglietta, F., Ourcival, J. M., Papale, D., Pilegaard, K., Rambal, S., Seufert, G., Soussana, J. F., Sanz, M. J., Schulze, E. D., Vesala, T., and Valentini, R.: Europe-wide reduction in primary productivity caused by the heat and drought in 2003, Nature, 437, 529, https://doi.org/10.1038/nature03972, 2005.
Clark, K. L., Skowronski, N., and Hom, J.: Invasive insects impact forest carbon dynamics, Glob. Change Biol., 16, 88–101, 2010.
Coley, P., Massa, M., Lovelock, C., and Winter, K.: Effects of elevated CO2 on foliar chemistry of saplings of nine species of tropical tree, Oecologia, 133, 62–69, https://doi.org/10.1007/s00442-002-1005-6, 2002.
Creeden, E. P., Hicke, J. A., and Buotte, P. C.: Climate, weather, and recent mountain pine beetle outbreaks in the western United States, Forest Ecol. Manag., 312, 239–251, 2014.
da Costa, A. C. L., Galbraith, D., Almeida, S., Portela, B. T. T., da Costa, M., de Athaydes Silva Junior, J., Braga, A. P., de Gonçalves, P. H. L., de Oliveira, A. A., Fisher, R., Phillips, O. L., Metcalfe, D. B., Levy, P., and Meir, P.: Effect of 7 yr of experimental drought on vegetation dynamics and biomass storage of an eastern Amazonian rainforest, New Phytol., 187, 579–591, https://doi.org/10.1111/j.1469-8137.2010.03309.x, 2010.
D'Amato, A. W., Bradford, J. B., Fraver, S., and Palik, B. J.: Effects of thinning on drought vulnerability and climate response in north temperate forest ecosystems, Eco. Appl., 23, 1735–1742, 2013.
De Kauwe, M. G., Medlyn, B. E., Zaehle, S., Walker, A. P., Dietze, M. C., Wang, Y., Luo, Y., Jain, A. K., El-Masri, B., Hickler, T., Wårlind, D., Weng, E., Parton, W. J., Thornton, P. E., Wang, S., Prentice, I. C., Asao, S., Smith, B., McCarthy, H. R., Iversen, C. M., Hanson, P. J., Warren, J. M., Oren, R., and Norby, R. J.: Where does the carbon go? A model-data intercomparison of vegetation carbon allocation and turnover processes at two temperate forest free-air CO2 enrichment sites, New Phytol., 203, 883–899, https://doi.org/10.1111/nph.12847, 2014.
Dietze, M. C. and Matthes, J. H.: A general ecophysiological framework for modelling the impact of pests and pathogens on forest ecosystems, Ecol. Lett., 17, 1418–1426, 2014.
Döscher, R., Acosta, M., Alessandri, A., Anthoni, P., Arsouze, T., Bergman, T., Bernardello, R., Boussetta, S., Caron, L.-P., Carver, G., Castrillo, M., Catalano, F., Cvijanovic, I., Davini, P., Dekker, E., Doblas-Reyes, F. J., Docquier, D., Echevarria, P., Fladrich, U., Fuentes-Franco, R., Gröger, M., v. Hardenberg, J., Hieronymus, J., Karami, M. P., Keskinen, J.-P., Koenigk, T., Makkonen, R., Massonnet, F., Ménégoz, M., Miller, P. A., Moreno-Chamarro, E., Nieradzik, L., van Noije, T., Nolan, P., O'Donnell, D., Ollinaho, P., van den Oord, G., Ortega, P., Prims, O. T., Ramos, A., Reerink, T., Rousset, C., Ruprich-Robert, Y., Le Sager, P., Schmith, T., Schrödner, R., Serva, F., Sicardi, V., Sloth Madsen, M., Smith, B., Tian, T., Tourigny, E., Uotila, P., Vancoppenolle, M., Wang, S., Wårlind, D., Willén, U., Wyser, K., Yang, S., Yepes-Arbós, X., and Zhang, Q.: The EC-Earth3 Earth system model for the Coupled Model Intercomparison Project 6, Geosci. Model Dev., 15, 2973–3020, https://doi.org/10.5194/gmd-15-2973-2022, 2022.
Dreesen, F. E., De Boeck, H. J., Janssens, I. A., and Nijs, I.: Do successive climate extremes weaken the resistance of plant communities? An experimental study using plant assemblages, Biogeosciences, 11, 109–121, https://doi.org/10.5194/bg-11-109-2014, 2014.
Eamus, D., Boulain, N., Cleverly, J., and Breshears, D. D.: Global change-type drought-induced tree mortality: vapor pressure deficit is more important than temperature per se in causing decline in tree health, Ecol. Evol., 3, 2711–2729, 2013.
Eller, C. B., Rowland, L., Mencuccini, M., Rosas, T., Williams, K., Harper, A., Medlyn, B. E., Wagner, Y., Klein, T., Teodoro, G. S., Oliveira, R. S., Matos, I. S., Rosado, B. H. P., Fuchs, K., Wohlfahrt, G., Montagnani, L., Meir, P., Sitch, S., and Cox, P. M.: Stomatal optimization based on xylem hydraulics (SOX) improves land surface model simulation of vegetation responses to climate, New Phytol., 226, 1622–1637, https://doi.org/10.1111/nph.16419, 2020.
Ellsworth, D. S., Anderson, I. C., Crous, K. Y., Cooke, J., Drake, J. E., Gherlenda, A. N., Gimeno, T. E., Macdonald, C. A., Medlyn, B. E., Powell, J. R., Tjoelker, M. G., and Reich, P. B.: Elevated CO2 does not increase eucalypt forest productivity on a low-phosphorus soil, Nat. Clim. Change, 7, 279–282, https://doi.org/10.1038/nclimate3235, 2017.
Enquist, B. J. and Enquist, C. A. F.: Long-term change within a Neotropical forest: assessing differential functional and floristic responses to disturbance and drought, Glob. Change Biol., 17, 1408–1424, 2011.
Esquivel-Muelbert, A., Baker, T. R., Dexter, K. G., et al.: Compositional response of Amazon forests to climate change, Glob. Change Biol., 25, 39–56, https://doi.org/10.1111/gcb.14413, 2019.
Eziz, A., Yan, Z., Tian, D., Han, W., Tang, Z., and Fang, J.: Drought effect on plant biomass allocation: A meta-analysis, Ecol. Evol, 7, 11002–11010, 2017.
Fang, Y., Leung, L. R., Knox, R., Koven, C., and Bond-Lamberty, B.: Impact of the numerical solution approach of a plant hydrodynamic model (v0.1) on vegetation dynamics, Geosci. Model Dev., 15, 6385–6398, https://doi.org/10.5194/gmd-15-6385-2022, 2022.
Feldpausch, T. R., Phillips, O. L., Brienen, R. J. W., Gloor, E., Lloyd, J., Lopez-Gonzalez, G., Monteagudo-Mendoza, A., Malhi, Y., Alarcón, A., Álvarez Dávila, E., Alvarez-Loayza, P., Andrade, A., Aragao, L. E. O. C., Arroyo, L., Aymard C., G. A., Baker, T. R., Baraloto, C., Barroso, J., Bonal, D., Castro, W., Chama, V., Chave, J., Domingues, T. F., Fauset, S., Groot, N., Honorio Coronado, E., Laurance, S., Laurance, W. F., Lewis, S. L., Licona, J. C., Marimon, B. S., Marimon-Junior, B. H., Mendoza Bautista, C., Neill, D. A., Oliveira, E. A., Oliveira dos Santos, C., Pallqui Camacho, N. C., Pardo-Molina, G., Prieto, A., Quesada, C. A., Ramírez, F., Ramírez-Angulo, H., Réjou-Méchain, M., Rudas, A., Saiz, G., Salomão, R. P., Silva-Espejo, J. E., Silveira, M., ter Steege, H., Stropp, J., Terborgh, J., Thomas-Caesar, R., van der Heijden, G. M. F., Vásquez Martinez, R., Vilanova, E., and Vos, V. A.: Amazon forest response to repeated droughts, Global Biogeochem. Cy., 30, 964–982, https://doi.org/10.1002/2015GB005133, 2016.
Fisher, R. A. and Koven, C. D.: Perspectives on the future of land surface models and the challenges of representing complex terrestrial systems, JAMES, 12, e2018MS001453, https://doi.org/10.1029/2018MS001453, 2020.
Fisher, R. A., Muszala, S., Verteinstein, M., Lawrence, P., Xu, C., McDowell, N. G., Knox, R. G., Koven, C., Holm, J., Rogers, B. M., Spessa, A., Lawrence, D., and Bonan, G.: Taking off the training wheels: the properties of a dynamic vegetation model without climate envelopes, CLM4.5(ED), Geosci. Model Dev., 8, 3593–3619, https://doi.org/10.5194/gmd-8-3593-2015, 2015.
Fisher, R. A., Koven, C. D., Anderegg, W. R. L., Christoffersen, B. O., Dietze, M. C., Farrior, C. E., Holm, J. A., Hurtt, G. C., Knox, R. G., Lawrence, P. J., Lichstein, J. W., Longo, M., Matheny, A. M., Medvigy, D., Muller-Landau, H. C., Powell, T. L., Serbin, S. P., Sato, H., Shuman, J. K., Smith, B., Trugman, A. T., Viskari, T., Verbeeck, H., Weng, E., Xu, C., Xu, X., Zhang, T., and Moorcroft, P. R.: Vegetation demographics in Earth System Models: A review of progress and priorities, Glob. Change Biol., 24, 35–54, https://doi.org/10.1111/gcb.13910, 2018.
Fleischer, K., Rammig, A., De Kauwe, M. G., Walker, A. P., Domingues, T. F., Fuchslueger, L., Garcia, S., Goll, D. S., Grandis, A., Jiang, M., Haverd, V., Hofhansl, F., Holm, J. A., Kruijt, B., Leung, F., Medlyn, B. E., Mercado, L. M., Norby, R. J., Pak, B., von Randow, C., Quesada, C. A., Schaap, K. J., Valverde-Barrantes, O. J., Wang, Y.-P., Yang, X., Zaehle, S., Zhu, Q., and Lapola, D. M.: Amazon forest response to CO2 fertilization dependent on plant phosphorus acquisition, Nat. Geosci., 12, 736–741, 2019.
Frank, D., Reichstein, M., Bahn, M., Thonicke, K., Frank, D., Mahecha, M. D., Smith, P., Velde, M., Vicca, S., Babst, F., Beer, C., Buchmann, N., Canadell, J. G., Ciais, P., Cramer, W., Ibrom, A., Miglietta, F., Poulter, B., Rammig, A., Seneviratne, S. I., Walz, A., Wattenbach, M., Zavala, M. A., and Zscheischler, J.: Effects of climate extremes on the terrestrial carbon cycle: concepts, processes and potential future impacts, Glob. Change Biol., 21, 2861–2880, 2015.
Franklin, O., McMurtrie, R. E., Iversen, C. M., Crous, K. Y., Finzi, A. C., Tissue, D. T., Ellsworth, D. S., Oren, R., and Norby, R. J.: Forest fine-root production and nitrogen use under elevated CO2: contrasting responses in evergreen and deciduous trees explained by a common principle, Glob. Change Biol., 15, 132–144, 2009.
Franklin, O., Johansson, J., Dewar, R. C., Dieckmann, U., McMurtrie, R. E., Brännström, Å., and Dybzinski, R.: Modeling carbon allocation in trees: a search for principles, Tree Physiol., 32, 648–666, https://doi.org/10.1093/treephys/tpr138, 2012.
Franklin, O., Harrison, S. P., Dewar, R., Farrior, C. E., Brännström, Å., Dieckmann, U., Pietsch, S., Falster, D., Cramer, W., Loreau, M., Wang, H., Mäkelä, A., Rebel, K. T., Meron, E., Schymanski, S. J., Rovenskaya, E., Stocker, B. D., Zaehle, S., Manzoni, S., van Oijen, M., Wright, I. J., Ciais, P., van Bodegom, P. M., Peñuelas, J., Hofhansl, F., Terrer, C., Soudzilovskaia, N. A., Midgley, G., and Prentice, I. C.: Organizing principles for vegetation dynamics, Nat. Plants, 6, 444–453, https://doi.org/10.1038/s41477-020-0655-x, 2020.
Friend, A. D., Lucht, W., Rademacher, T. T., Keribin, R., Betts, R., Cadule, P., Ciais, P., Clark, D. B., Dankers, R., Falloon, P. D., Ito, A., Kahana, R., Kleidon, A., Lomas, M. R., Nishina, K., Ostberg, S., Pavlick, R., Peylin, P., Schaphoff, S., Vuichard, N., Warszawski, L., Wiltshire, A., and Woodward, F. I.: Carbon residence time dominates uncertainty in terrestrial vegetation responses to future climate and atmospheric CO2, P. Natl. Acad. Sci. USA, 111, 3280–3285, 2014.
Gerten, D., Luo, Y., Le Maire, G., Parton, W. J., Keough, C., Weng, E., Beier, C., Ciais, P., Cramer, W., Dukes, J. S., Hanson, P. J., Knapp, A. A. K., Linder, S., Nepstad, D., Rustad, L., and Sowerby, A.: Modelled effects of precipitation on ecosystem carbon and water dynamics in different climatic zones, Glob. Change Biol., 14, 2365–2379, 2008.
Goulden, M. L. and Bales, R. C.: California forest die-off linked to multi-year deep soil drying in 2012–2015 drought, Nat. Geosci., 12, 632–637, 2019.
Gray, S. B., Dermody, O., Klein, S. P., Locke, A. M., McGrath, J. M., Paul, R. E., Rosenthal, D. M., Ruiz-Vera, U. M., Siebers, M. H., Strellner, R., Ainsworth, E. A., Bernacchi, C. J., Long, S. P., Ort, D. R., and Leakey, A. D. B.: Intensifying drought eliminates the expected benefits of elevated carbon dioxide for soybean, Nat. Plants, 2, 16132, https://doi.org/10.1038/nplants.2016.132, 2016.
Greenwood, S., Ruiz-Benito, P., Martínez-Vilalta, J., Lloret, F., Kitzberger, T., Allen, C. D., Fensham, R., Laughlin, D. C., Kattge, J., Bönisch, G., Kraft, N. J. B., and Jump, A. S.: Tree mortality across biomes is promoted by drought intensity, lower wood density and higher specific leaf area, Ecol. Lett., 20, 539–553, https://doi.org/10.1111/ele.12748, 2017.
Griffin, D. and Anchukaitis, K. J.: How unusual is the 2012–2014 California drought?, Geophys. Res. Lett., 41, 9017–9023, 2014.
Hanbury-Brown, A. R., Powell, T. L., Muller-Landau, H. C., Wright, S. J., and Kueppers, L. M.: Simulating environmentally-sensitive tree recruitment in vegetation demographic models, New Phytol., 235, 78–93, https://doi.org/10.1111/nph.18059, 2022.
Harrison, S. P., Cramer, W., Franklin, O., Prentice, I. C., Wang, H., Brännström, Å., Boer, H., Dieckmann, U., Joshi, J., Keenan, T. F., Lavergne, A., Manzoni, S., Mengoli, G., Morfopoulos, C., Peñuelas, J., Pietsch, S., Rebel, K. T., Ryu, Y., Smith, N. G., Stocker, B. D., and Wright, I. J.: Eco-evolutionary optimality as a means to improve vegetation and land-surface models, New Phytol., 231, 2125–2141, https://doi.org/10.1111/nph.17558, 2021.
Hickler, T., Smith, B., Sykes, M. T., Davis, M. B., Sugita, S., and Walker, K.: USING A GENERALIZED VEGETATION MODEL TO SIMULATE VEGETATION DYNAMICS IN NORTHEASTERN USA, Ecology, 85, 519–530, 2004.
Holm, J. A., Knox, R. G., Zhu, Q., Fisher, R. A., Koven, C. D., Nogueira Lima, A. J., Riley, W. J., Longo, M., Negrón-Juárez, R. I., Araujo, A. C., Kueppers, L. M., Moorcroft, P. R., Higuchi, N., and Chambers, J. Q.: The central Amazon biomass sink under current and future atmospheric CO2: Predictions from big-leaf and demographic vegetation models, J. Geophys. Res.-Biogeo., 125, e2019JG005500. https://doi.org/10.1029/2019JG005500, 2020.
Hovenden, M. J., Newton, P. C. D., and Wills, K. E.: Seasonal not annual rainfall determines grassland biomass response to carbon dioxide, Nature, 511, 583–586, https://doi.org/10.1038/nature13281, 2014.
Hubbard, R. M., Rhoades, C. C., Elder, K., and Negron, J.: Changes in transpiration and foliage growth in lodgepole pine trees following mountain pine beetle attack and mechanical girdling, Forest Ecol. Manag., 289, 312–317, 2013.
IPCC: Managing the Risks of Extreme Events and Disasters to Advance Climate Change Adaptation. A Special Report of Working Groups I and II of the Intergovernmental Panel on Climate Change, edited by: Field, C. B., Barros, V., Stocker, T. F., Qin, D., Dokken, D. J., Ebi, K. L., Mastrandrea, M. D., Mach, K. J., Plattner, G.-K., Allen, S. K., Tignor, M., and Midgley, P. M., Cambridge University Press, Cambridge, UK, and New York, NY, USA, 582 pp., 2012.
IPCC: Climate Change 2021: The Physical Science Basis. Contribution of Working Group I to the Sixth Assessment Report of the Intergovernmental Panel on Climate Change, edited by: Masson-Delmotte, V., Zhai, P., Pirani, A., Connors, S. L., Péan, C., Berger, S., Caud, N., Chen, Y., Goldfarb, L., Gomis, M. I., Huang, M., Leitzell, K., Lonnoy, E., Matthews, J. B. R., Maycock, T. K., Waterfield, T., Yelekçi, O., Yu, R., and Zhou, B., Cambridge University Press, Cambridge, United Kingdom and New York, NY, USA, 2391 pp., https://doi.org/10.1017/9781009157896, 2021.
Jiang, M., Medlyn, B. E., Drake, J. E., Duursma, R. A., Anderson, I. C., Barton, C. V. M., Boer, M. M., Carrillo, Y., Castañeda-Gómez, L., Collins, L., Crous, K. Y., De Kauwe, M. G., dos Santos, B. M., Emmerson, K. M., Facey, S. L., Gherlenda, A. N., Gimeno, T. E., Hasegawa, S., Johnson, S. N., Kännaste, A., Macdonald, C. A., Mahmud, K., Moore, B. D., Nazaries, L., Neilson, E. H. J., Nielsen, U. N., Niinemets, Ü., Noh, N. J., Ochoa-Hueso, R., Pathare, V. S., Pendall, E., Pihlblad, J., Piñeiro, J., Powell, J. R., Power, S. A., Reich, P. B., Renchon, A. A., Riegler, M., Rinnan, R., Rymer, P. D., Salomón, R. L., Singh, B. K., Smith, B., Tjoelker, M. G., Walker, J. K. M., Wujeska-Klause, A., Yang, J., Zaehle, S., and Ellsworth, D. S.: The fate of carbon in a mature forest under carbon dioxide enrichment, Nature, 580, 227–231, https://doi.org/10.1038/s41586-020-2128-9, 2020.
Joslin, J. D., Wolfe, M.H., and Hanson, P. J.: Effects of altered water regimes on forest root systems, New Phytol., 147, 117–129, 2000.
Jump, A. S., Ruiz‐Benito, P., Greenwood, S., Allen, C. D., Kitzberger, T., Fensham, R., Martínez‐Vilalta, J., and Lloret, F.: Structural overshoot of tree growth with climate variability and the global spectrum of drought-induced forest dieback, Glob. Change Biol., 23, 3742–3757, 2017.
Kannenberg, S. A., Schwalm, C. R., and Anderegg, W. R. L.: Ghosts of the past: how drought legacy effects shape forest functioning and carbon cycling, Ecol. Lett., 23, 891–901, https://doi.org/10.1111/ele.13485, 2020.
Kattge, J., Díaz, S., Lavorel, S., et al.: TRY – a global database of plant traits, Glob. Change Biol., 17, 2905–2935, 2011.
Kayler, Z. E., De Boeck, H. J., Fatichi, S., Grünzweig, J. M., Merbold, L., Beier, C., McDowell, N., and Dukes, J. S.: Experiments to confront the environmental extremes of climate change, Front. Ecol. Environ., 13, 219–225, 2015.
Keenan, T. F., Hollinger, D. Y., Bohrer, G., Dragoni, D., Munger, J. W., Schmid, H. P., and Richardson, A. D.: Increase in forest water-use efficiency as atmospheric carbon dioxide concentrations rise, Nature, 499, 324–327, 2013.
Kennedy, D., Swenson, S., Oleson, K. W., Lawrence, D. M., Fisher, R., Lola da Costa, A. C., and Gentine, P.: Implementing plant hydraulics in the Community Land Model, version 5, JAMES, 11, 485–513, https://doi.org/10.1029/2018MS001500, 2019.
Koven, C. D., Knox, R. G., Fisher, R. A., Chambers, J. Q., Christoffersen, B. O., Davies, S. J., Detto, M., Dietze, M. C., Faybishenko, B., Holm, J., Huang, M., Kovenock, M., Kueppers, L. M., Lemieux, G., Massoud, E., McDowell, N. G., Muller-Landau, H. C., Needham, J. F., Norby, R. J., Powell, T., Rogers, A., Serbin, S. P., Shuman, J. K., Swann, A. L. S., Varadharajan, C., Walker, A. P., Wright, S. J., and Xu, C.: Benchmarking and parameter sensitivity of physiological and vegetation dynamics using the Functionally Assembled Terrestrial Ecosystem Simulator (FATES) at Barro Colorado Island, Panama, Biogeosciences, 17, 3017–3044, https://doi.org/10.5194/bg-17-3017-2020, 2020.
Li, L., Yang, Z., Matheny, A. M., Zheng, H., Swenson, S. C., Lawrence, D. M., Barlage, M., Yan, B., McDowell, N. G., and Leung, L. R.: Representation of plant hydraulics in the Noah-MP land surface model: Model development and multiscale evaluation, JAMES, 13, e2020MS002214, https://doi.org/10.1029/2020MS002214, 2021.
Li, Q., Lu, X., Wang, Y., Huang, X., Cox, P. M., and Luo, Y.: Leaf area index identified as a major source of variability in modeled CO2 fertilization, Biogeosciences, 15, 6909–6925, https://doi.org/10.5194/bg-15-6909-2018, 2018.
Liu, Y., Parolari, A. J., Kumar, M., Huang, C.-W., Katul, G. G., and Porporato, A.: Increasing atmospheric humidity and CO2 concentration alleviate forest mortality risk, P. Natl. Acad. Sci. USA, 114, 9918–9923, 2017.
Lloret, F., Escudero, A., Iriondo, J. M., Martínez-Vilalta, J., and Valladares, F.: Extreme climatic events and vegetation: the role of stabilizing processes, Glob. Change Biol., 18, 797–805, 2012.
Luo, Y., Gerten, D., Le Maire, G., Parton, W. J., Weng, E., Zhou, X., Keough, C., Beier, C., Ciais, P., Cramer, W., Dukes, J. S., Emmett, B., Hanson, P. J., Knapp, A., Linder, S., Nepstad, D., and Rustad, L.: Modeled interactive effects of precipitation, temperature, and [CO2] on ecosystem carbon and water dynamics in different climatic zones, Glob. Change Biol., 14, 1986–1999, 2008.
Luo, Y., Jiang, L., Niu, S., and Zhou, X.: Nonlinear responses of land ecosystems to variation in precipitation, New Phytol., 214, 5–7, 2017.
Luo, Y. Q., Randerson, J. T., Abramowitz, G., Bacour, C., Blyth, E., Carvalhais, N., Ciais, P., Dalmonech, D., Fisher, J. B., Fisher, R., Friedlingstein, P., Hibbard, K., Hoffman, F., Huntzinger, D., Jones, C. D., Koven, C., Lawrence, D., Li, D. J., Mahecha, M., Niu, S. L., Norby, R., Piao, S. L., Qi, X., Peylin, P., Prentice, I. C., Riley, W., Reichstein, M., Schwalm, C., Wang, Y. P., Xia, J. Y., Zaehle, S., and Zhou, X. H.: A framework for benchmarking land models, Biogeosciences, 9, 3857–3874, https://doi.org/10.5194/bg-9-3857-2012, 2012.
Ma, W., Zhai, L., Pivovaroff, A., Shuman, J., Buotte, P., Ding, J., Christoffersen, B., Knox, R., Moritz, M., Fisher, R. A., Koven, C. D., Kueppers, L., and Xu, C.: Assessing climate change impacts on live fuel moisture and wildfire risk using a hydrodynamic vegetation model, Biogeosciences, 18, 4005–4020, https://doi.org/10.5194/bg-18-4005-2021, 2021.
MacGillivray, C. W. and Grime, J. P.: Testing Predictions of the Resistance and Resilience of Vegetation Subjected to Extreme Events, Funct. Ecol., 9, 640–649, 1995.
Markewitz, D., Devine, S., Davidson, E. A., Brando, P., and Nepstad, D. C.: Soil moisture depletion under simulated drought in the Amazon: impacts on deep root uptake, New Phytol., 187, 592–607, 2010.
Matusick, G., Ruthrof, K. X., Brouwers, N. C., Dell, B., and Hardy, G. S. J.: Sudden forest canopy collapse corresponding with extreme drought and heat in a mediterranean-type eucalypt forest in southwestern Australia, Eur. J. For. Res., 132, 497–510, 2013.
Matusick, G., Ruthrof, K. X., Fontaine, J. B., and Hardy, G. E. S. J.: Eucalyptus forest shows low structural resistance and resilience to climate change-type drought, J. Veg. Sci., 27, 493–503, 2016.
McCarthy, M. C. and Enquist, B. J.: Consistency between an allometric approach and optimal partitioning theory in global patterns of plant biomass allocation, Funct. Ecol., 21, 713–720, 2007.
McDowell, N., Pockman, W. T., Allen, C. D., Breshears, D. D., Cobb, N., Kolb, T., Plaut, J., Sperry, J., West, A., Williams, D. G., and Yepez, E. A.: Mechanisms of plant survival and mortality during drought: why do some plants survive while others succumb to drought?, New Phytol., 178, 719–739, 2008.
McDowell, N. G., Adams, H. D., Bailey, J. D., Hess, M., and Kolb, T. E.: Homeostatic Maintenance Of Ponderosa Pine Gas Exchange In Response To Stand Density Changes, Ecol. Appl., 16, 1164–1182, 2006.
McDowell, N. G. and Allen, C. D.: Darcy's law predicts widespread forest mortality under climate warming, Nat. Clim. Change, 5, 669–672, 2015.
McDowell, N. G., Beerling, D. J., Breshears, D. D., Fisher, R. A., Raffa, K. F., and Stitt, M.: The interdependence of mechanisms underlying climate-driven vegetation mortality, Trends Ecol. Evol., 26, 523–532, 2011.
McDowell, N. G., Fisher, R. A., Xu, C., Domec, J. C., Hölttä, T., Mackay, D. S., Sperry, J. S., Boutz, A., Dickman, L., Gehres, N., Limousin, J. M., Macalady, A., Martínez‐Vilalta, J., Mencuccini, M., Plaut, J. A., Ogée, J., Pangle, R. E., Rasse, D. P., Ryan, M. G., Sevanto, S., Waring, R. H., Williams, A. P., Yepez, E. A., and Pockman, W. T.: Evaluating theories of drought-induced vegetation mortality using a multimodel–experiment framework, New Phytol., 200, 304–321, 2013.
Medlyn, B. E., De Kauwe, M. G., Zaehle, S., Walker, A. P., Duursma, R. A., Luus, K., Mishurov, M., Pak, B., Smith, B., Wang, Y.-P., Yang, X., Crous, K. Y., Drake, J. E., Gimeno, T. E., Macdonald, C. A., Norby, R. J., Power, S. A., Tjoelker, M. G., and Ellsworth, D. S.: Using models to guide field experiments: a priori predictions for the CO2 response of a nutrient- and water-limited native Eucalypt woodland, Glob. Change Biol., 22, 2834–2851, https://doi.org/10.1111/gcb.13268, 2016.
Medvigy, D. and Moorcroft, P. R.: Predicting ecosystem dynamics at regional scales: an evaluation of a terrestrial biosphere model for the forests of northeastern North America, Philos. T. Roy. Soc. B, 367, 222–235, 2012.
Medvigy, D., Wofsy, S., Munger, J., Hollinger, D., and Moorcroft, P.: Mechanistic scaling of ecosystem function and dynamics in space and time: Ecosystem Demography model version 2, J. Geophys. Res.-Biogeo., 114, 2008JG000812, https://doi.org/10.1029/2008JG000812, 2009.
Medvigy, D., Clark, K. L., Skowronski, N. S., and Schäfer, K. V. R.: Simulated impacts of insect defoliation on forest carbon dynamics, Environ. Res. Lett., 7, 045703, https://doi.org/10.1088/1748-9326/7/4/045703, 2012.
Medvigy, D., Wang, G., Zhu, Q., Riley, W. J., Trierweiler, A. M., Waring, B., Xu, X., and Powers, J. S.: Observed variation in soil properties can drive large variation in modelled forest functioning and composition during tropical forest secondary succession, New Phytol., 223, 1820–1833, https://doi.org/10.1111/nph.15848, 2019.
Mencuccini, M., Manzoni, S., and Christoffersen, B.: Modelling water fluxes in plants: from tissues to biosphere, New Phytol., 222, 1207–1222, https://doi.org/10.1111/nph.15681, 2019.
Meir, P., Wood, T. E., Galbraith, D. R., Brando, P. M., Da Costa, A. C. L., Rowland, L., and Ferreira, L. V.: Threshold Responses to Soil Moisture Deficit by Trees and Soil in Tropical Rain Forests: Insights from Field Experiments, BioScience, 65, 882–892, 2015.
Montané, F., Fox, A. M., Arellano, A. F., MacBean, N., Alexander, M. R., Dye, A., Bishop, D. A., Trouet, V., Babst, F., Hessl, A. E., Pederson, N., Blanken, P. D., Bohrer, G., Gough, C. M., Litvak, M. E., Novick, K. A., Phillips, R. P., Wood, J. D., and Moore, D. J. P.: Evaluating the effect of alternative carbon allocation schemes in a land surface model (CLM4.5) on carbon fluxes, pools, and turnover in temperate forests, Geosci. Model Dev., 10, 3499–3517, https://doi.org/10.5194/gmd-10-3499-2017, 2017.
Myers, J. A. and Kitajima, K.: Carbohydrate storage enhances seedling shade and stress tolerance in a neotropical forest, J. Ecol., 95, 383–395, 2007.
Niklas, K. J.: The scaling of plant height: A comparison among major plant clades and anatomical grades, Ann. Bot., 72, 165–172, https://doi.org/10.1006/anbo.1993.1095, 1993.
Norby, R. J., DeLucia, E. H., Gielen, B., Calfapietra, C., Giardina, C. P., King, J. S., Ledford, J., McCarthy, H. R., Moore, D. J. P., Ceulemans, R., De Angelis, P., Finzi, A. C., Karnosky, D. F., Kubiske, M. E., Lukac, M., Pregitzer, K. S., Scarascia-Mugnozza, G. E., Schlesinger, W. H., and Oren, R.: Forest response to elevated CO2 is conserved across a broad range of productivity, P. Natl. Acad. Sci. USA, 102, 18052–18056, 2005.
Obermeier, W. A., Lehnert, L. W., Kammann, C. I., Müller, C., Grünhage, L., Luterbacher, J., Erbs, M., Moser, G., Seibert, R., Yuan, N., and Bendix, J.: Reduced CO2 fertilization effect in temperate C3 grasslands under more extreme weather conditions, Nat. Clim. Change, 7, 137–141, https://doi.org/10.1038/nclimate3191, 2016.
O'Brien, M. J., Leuzinger, S., Philipson, C. D., Tay, J., and Hector, A.: Drought survival of tropical tree seedlings enhanced by non-structural carbohydrate levels, Nat. Clim. Change, 4, 710–714, https://doi.org/10.1038/nclimate2281, 2014.
Palace, M., Keller, M., and Silva, H.: NECROMASS PRODUCTION: STUDIES IN UNDISTURBED AND LOGGED AMAZON FORESTS, Ecol. Appl., 18, 873–884, 2008.
Petit, G., Anfodillo, T., and Mencuccini, M.: Tapering of xylem conduits and hydraulic limitations in sycamore (Acer pseudoplatanus) trees, New Phytol., 177, 653–664, https://doi.org/10.1111/j.1469-8137.2007.02291.x, 2008.
Phillips, O. L., Aragão, L. E. O. C., Lewis, S. L., Fisher, J. B., Lloyd, J., López-González, G., Malhi, Y., Monteagudo, A., Peacock, J., Quesada, C. A., van der Heijden, G., Almeida, S., Amaral, I., Arroyo, L., Aymard, G., Baker, T. R., Bánki, O., Blanc, L., Bonal, D., Brando, P., Chave, J., de Oliveira, Á. C. A., Cardozo, N. D., Czimczik, C. I., Feldpausch, T. R., Freitas, M. A., Gloor, E., Higuchi, N., Jiménez, E., Lloyd, G., Meir, P., Mendoza, C., Morel, A., Neill, D. A., Nepstad, D., Patiño, S., Peñuela, M. C., Prieto, A., Ramírez, F., Schwarz, M., Silva, J., Silveira, M., Thomas, A. S., Steege, H. ter, Stropp, J., Vásquez, R., Zelazowski, P., Dávila, E. A., Andelman, S., Andrade, A., Chao, K.-J., Erwin, T., Di Fiore, A., C., E. H., Keeling, H., Killeen, T. J., Laurance, W. F., Cruz, A. P., Pitman, N. C. A., Vargas, P. N., Ramírez-Angulo, H., Rudas, A., Salamão, R., Silva, N., Terborgh, J., and Torres-Lezama, A.: Drought Sensitivity of the Amazon Rainforest, Science, 323, 1344–1347, 2009.
Phillips, O. L., van der Heijden, G., Lewis, S. L., López-González, G., Aragão, L. E. O. C., Lloyd, J., Malhi, Y., Monteagudo, A., Almeida, S., Dávila, E. A., Amaral, I., Andelman, S., Andrade, A., Arroyo, L., Aymard, G., Baker, T. R., Blanc, L., Bonal, D., de Oliveira, Á. C. A., Chao, K.-J., Cardozo, N. D., da Costa, L., Feldpausch, T. R., Fisher, J. B., Fyllas, N. M., Freitas, M. A., Galbraith, D., Gloor, E., Higuchi, N., Honorio, E., Jiménez, E., Keeling, H., Killeen, T. J., Lovett, J. C., Meir, P., Mendoza, C., Morel, A., Vargas, P. N., Patiño, S., Peh, K. S.-H., Cruz, A. P., Prieto, A., Quesada, C. A., Ramírez, F., Ramírez, H., Rudas, A., Salamão, R., Schwarz, M., Silva, J., Silveira, M., Ferry Slik, J. W., Sonké, B., Thomas, A. S., Stropp, J., Taplin, J. R. D., Vásquez, R., and Vilanova, E.: Drought–mortality relationships for tropical forests, New Phytol., 187, 631–646, 2010.
Pilon, C. E., Côté, B., and Fyles, J. W.: Effect of an artificially induced drought on leaf peroxidase activity, mineral nutrition and growth of sugar maple, Plant Soil, 179, 151–158, 1996.
Potter, C., Klooster, S., Hiatt, C., Genovese, V., and Castilla-Rubio, J. C.: Changes in the carbon cycle of Amazon ecosystems during the 2010 drought, Environ. Res. Lett., 6, 034024, https://doi.org/10.1088/1748-9326/6/3/034024, 2011.
Powell, T. L., Galbraith, D. R., Christoffersen, B. O., Harper, A., Imbuzeiro, H. M. A., Rowland, L., Almeida, S., Brando, P. M., Costa, A. C. L., Costa, M. H., Levine, N. M., Malhi, Y., Saleska, S. R., Sotta, E., Williams, M., Meir, P., and Moorcroft, P. R.: Confronting model predictions of carbon fluxes with measurements of Amazon forests subjected to experimental drought, New Phytol., 200, 350–365, 2013.
Powell, T. L., Koven, C. D., Johnson, D. J., Faybishenko, B., Fisher, R. A., Knox, R. G., McDowell, N. G., Condit, R., Hubbell, S. P., Wright, S. J., Chambers, J. Q., and Kueppers, L. M.: Variation in hydroclimate sustains tropical forest biomass and promotes functional diversity, New Phytol., 219, 932–946, 2018.
Powers, J. S., Becknell, J. M., Irving, J., and Pèrez-Aviles, D.: Diversity and structure of regenerating tropical dry forests in Costa Rica: Geographic patterns and environmental drivers, Forest Ecol. Manag., 258, 959–970, 2009.
Powers, J. S., Vargas G., G., Brodribb, T. J., Schwartz, N. B., Pérez‐Aviles, D., Smith‐Martin, C. M., Becknell, J. M., Aureli, F., Blanco, R., Calderón‐Morales, E., Calvo‐Alvarado, J. C., Calvo‐Obando, A. J., Chavarría, M. M., Carvajal‐Vanegas, D., Jiménez‐Rodríguez, C. D., Murillo Chacon, E., Schaffner, C. M., Werden, L. K., Xu, X., and Medvigy, D.: A catastrophic tropical drought kills hydraulically vulnerable tree species, Glob. Change Biol., 26, 3122–3133, https://doi.org/10.1111/gcb.15037, 2020.
Pugh, T. A. M., Rademacher, T., Shafer, S. L., Steinkamp, J., Barichivich, J., Beckage, B., Haverd, V., Harper, A., Heinke, J., Nishina, K., Rammig, A., Sato, H., Arneth, A., Hantson, S., Hickler, T., Kautz, M., Quesada, B., Smith, B., and Thonicke, K.: Understanding the uncertainty in global forest carbon turnover, Biogeosciences, 17, 3961–3989, https://doi.org/10.5194/bg-17-3961-2020, 2020.
Rapparini, F. and Peñuelas, J.: Mycorrhizal Fungi to Alleviate Drought Stress on Plant Growth, in: Use of Microbes for the Alleviation of Soil Stresses, Vol. 1, edited by: Miransari, M., Springer New York, NY, 21–42, https://doi.org/10.1007/978-1-4614-9466-9_2, 2014.
Reich, P. B., Wright, I. J., and Lusk, C. H.: PREDICTING LEAF PHYSIOLOGY FROM SIMPLE PLANT AND CLIMATE ATTRIBUTES: A GLOBAL GLOPNET ANALYSIS, Ecol. Appl., 17, 1982–1988, 2007.
Reich, P. B., Hobbie, S. E., and Lee, T. D.: Plant growth enhancement by elevated CO2 eliminated by joint water and nitrogen limitation, Nat. Geosci., 7, 920–924, https://doi.org/10.1038/ngeo2284, 2014.
Reichstein, M., Bahn, M., Ciais, P., Frank, D., Mahecha, M. D., Seneviratne, S. I., Zscheischler, J., Beer, C., Buchmann, N., Frank, D. C., Papale, D., Rammig, A., Smith, P., Thonicke, K., Van Der Velde, M., Vicca, S., Walz, A., and Wattenbach, M.: Climate extremes and the carbon cycle, Nature, 500, 287–295, 2013.
Reyes, J. J., Tague, C. L., Evans, R. D., and Adam, J. C.: Assessing the Impact of Parameter Uncertainty on Modeling Grass Biomass Using a Hybrid Carbon Allocation Strategy, J. Adv. Model. Earth Sy., 9, 2968–2992, 2017.
Richardson, A. D., Carbone, M. S., Keenan, T. F., Czimczik, C. I., Hollinger, D. Y., Murakami, P., Schaberg, P. G., and Xu, X.: Seasonal dynamics and age of stemwood nonstructural carbohydrates in temperate forest trees, New Phytol., 197, 850–861, 2013.
Rowland, L., Da Costa, A. C. L., Galbraith, D. R., Oliveira, R. S., Binks, O. J., Oliveira, A. A. R., Pullen, A. M., Doughty, C. E., Metcalfe, D. B., Vasconcelos, S. S., Ferreira, L. V., Malhi, Y., Grace, J., Mencuccini, M., and Meir, P.: Death from drought in tropical forests is triggered by hydraulics not carbon starvation, Nature, 528, 119–122, https://doi.org/10.1038/nature15539, 2015.
Roy, J., Picon-Cochard, C., Augusti, A., Benot, M.-L., Thiery, L., Darsonville, O., Landais, D., Piel, C., Defossez, M., Devidal, S., Escape, C., Ravel, O., Fromin, N., Volaire, F., Milcu, A., Bahn, M., and Soussana, J.-F.: Elevated CO2 maintains grassland net carbon uptake under a future heat and drought extreme, P. Natl. Acad. Sci. USA, 113, 6224–6229, 2016.
Ruppert, J. C., Harmoney, K., Henkin, Z., Snyman, H. A., Sternberg, M., Willms, W., and Linstädter, A.: Quantifying drylands' drought resistance and recovery: the importance of drought intensity, dominant life history and grazing regime, Glob. Change Biol., 21, 1258–1270, 2015.
Rustad, L. E.: The response of terrestrial ecosystems to global climate change: Towards an integrated approach, Sci. Total Environ., 404, 222–235, 2008.
Ruthrof, K. X., Breshears, D. D., Fontaine, J. B., Froend, R. H., Matusick, G., Kala, J., Miller, B. P., Mitchell, P. J., Wilson, S. K., Van Keulen, M., Enright, N. J., Law, D. J., Wernberg, T., and Hardy, G. E. St. J.: Subcontinental heat wave triggers terrestrial and marine, multi-taxa responses, Sci. Rep., 8, 13094, https://doi.org/10.1038/s41598-018-31236-5, 2018.
Scheiter, S., Langan, L., and Higgins, S. I.: Next-generation dynamic global vegetation models: learning from community ecology, New Phytol., 198, 957–969, 2013.
Schenk, H. J. and Jackson, R. B.: Mapping the global distribution of deep roots in relation to climate and soil characteristics, Geoderma, 126, 129–140, 2005.
Schwalm, C. R., Anderegg, W. R. L., Michalak, A. M., Fisher, J. B., Biondi, F., Koch, G., Litvak, M., Ogle, K., Shaw, J. D., Wolf, A., Huntzinger, D. N., Schaefer, K., Cook, R., Wei, Y., Fang, Y., Hayes, D., Huang, M., Jain, A., and Tian, H.: Global patterns of drought recovery, Nature, 548, 202–205, https://doi.org/10.1038/nature23021, 2017.
Seneviratne, S. I., Zhang, X., Adnan, M., Badi, W., Dereczynski, C., Di Luca, A., Ghosh, S., Iskandar, I., Kossin, J., Lewis, S., Otto, F., Pinto, I., Satoh, M., Vicente-Serrano, S. M., Wehner, M., and Zhou, B.: Weather and Climate Extreme Events in a Changing Climate, in Climate Change 2021: The Physical Science Basis. Contribution of Working Group I to the Sixth Assessment Report of the Intergovernmental Panel on Climate Change, edited by: Masson-Delmotte, V., Zhai, P., Pirani, A., Connors, S. L., Péan, C., Berger, S., Caud, N., Chen, Y., Goldfarb, L., Gomis, M. I., Huang, M., Leitzell, K., Lonnoy, E., Matthews, J. B. R., Maycock, T. K., Waterfield, T., Yelekçi, O., Yu, R., and Zhou, B., Cambridge University Press, Cambridge, United Kingdom and New York, NY, USA, 1513–1766, https://doi.org/10.1017/9781009157896.013, 2021.
Sheffield, J., Goteti, G., and Wood, E. F.: Development of a 50-Year High-Resolution Global Dataset of Meteorological Forcings for Land Surface Modeling, J. Climate, 19, 3088–3111, 2006.
Shiels, A. B., Zimmerman, J. K., García-Montiel, D. C., Jonckheere, I., Holm, J., Horton, D., and Brokaw, N.: Plant responses to simulated hurricane impacts in a subtropical wet forest, Puerto Rico, J. Ecol., 98, 659–673, 2010.
Signori-Müller, C., Oliveira, R. S., Barros, F. D. V., Tavares, J. V., Gilpin, M., Diniz, F. C., Zevallos, M. J. M., Yupayccana, C. A. S., Acosta, M., Bacca, J., Chino, R. S. C., Cuellar, G. M. A., Cumapa, E. R. M., Martinez, F., Mullisaca, F. M. P., Nina, A., Sanchez, J. M. B., Da Silva, L. F., Tello, L., Tintaya, J. S., Ugarteche, M. T. M., Baker, T. R., Bittencourt, P. R. L., Borma, L. S., Brum, M., Castro, W., Coronado, E. N. H., Cosio, E. G., Feldpausch, T. R., Fonseca, L. d'Agosto M., Gloor, E., Llampazo, G. F., Malhi, Y., Mendoza, A. M., Moscoso, V. C., Araujo-Murakami, A., Phillips, O. L., Salinas, N., Silveira, M., Talbot, J., Vasquez, R., Mencuccini, M., and Galbraith, D.: Non-structural carbohydrates mediate seasonal water stress across Amazon forests, Nat. Commun., 12, 2310, https://doi.org/10.1038/s41467-021-22378-8, 2021.
Silva, M., Matheny, A. M., Pauwels, V. R. N., Triadis, D., Missik, J. E., Bohrer, G., and Daly, E.: Tree hydrodynamic modelling of the soil–plant–atmosphere continuum using FETCH3, Geosci. Model Dev., 15, 2619–2634, https://doi.org/10.5194/gmd-15-2619-2022, 2022.
Sippel, S., Zscheischler, J., and Reichstein, M.: Ecosystem impacts of climate extremes crucially depend on the timing, P. Natl. Acad. Sci. USA, 113, 5768–5770, 2016.
Sitch, S., Huntingford, C., Gedney, N., Levy, P. E., Lomas, M., Piao, S. L., Betts, R., Ciais, P., Cox, P., Friedlingstein, P., Jones, C. D., Prentice, I. C., and Woodward, F. I.: Evaluation of the terrestrial carbon cycle, future plant geography and climate-carbon cycle feedbacks using five Dynamic Global Vegetation Models (DGVMs), Glob. Change Biol., 14, 2015–2039, 2008.
Skelton, R. P., West, A. G., and Dawson, T. E.: Predicting plant vulnerability to drought in biodiverse regions using functional traits, P. Natl. Acad. Sci. USA, 112, 5744–5749, 2015.
Smith, B. and Mishurov, M.: LPJ-GUESS Ecosystem Model [code], https://web.nateko.lu.se/lpj-guess/download.html, last access: 7 May 2023.
Smith, B., Prentice, I. C., and Sykes, M. T.: Representation of vegetation dynamics in the modelling of terrestrial ecosystems: comparing two contrasting approaches within European climate space, Global Ecol. Biogeogr., 10, 621–637, 2001.
Smith, B., Wårlind, D., Arneth, A., Hickler, T., Leadley, P., Siltberg, J., and Zaehle, S.: Implications of incorporating N cycling and N limitations on primary production in an individual-based dynamic vegetation model, Biogeosciences, 11, 2027–2054, https://doi.org/10.5194/bg-11-2027-2014, 2014.
Spasojevic, M. J., Bahlai, C. A., Bradley, B. A., Butterfield, B. J., Tuanmu, M.-N., Sistla, S., Wiederholt, R., and Suding, K. N.: Scaling up the diversity–resilience relationship with trait databases and remote sensing data: the recovery of productivity after wildfire, Glob. Change Biol., 22, 1421–1432, 2016.
Sperry, J. S. and Love, D. M.: What plant hydraulics can tell us about responses to climate-change droughts, New Phytol., 207, 14–27, 2015.
Sperry, J. S., Hacke, U. G., Oren, R., and Comstock, J. P.: Water deficits and hydraulic limits to leaf water supply, Plant Cell Environ., 25, 251–263, 2002.
Sperry, J. S., Wang, Y., Wolfe, B. T., Mackay, D. S., Anderegg, W. R. L., McDowell, N. G., and Pockman, W. T.: Pragmatic hydraulic theory predicts stomatal responses to climatic water deficits, New Phytol., 212, 577–589, 2016.
Stovall, A. E. L., Shugart, H., and Yang, X.: Tree height explains mortality risk during an intense drought, Nat. Commun., 10, 4385, https://doi.org/10.1038/s41467-019-12380-6, 2019.
Tague, C. L, and Moritz, M. A.: Plant Accessible Water Storage Capacity and Tree-Scale Root Interactions Determine How Forest Density Reductions Alter Forest Water Use and Productivity, Front. Forests and Global Change, 2, 36, https://doi.org/10.3389/ffgc.2019.00036, 2019.
Tomasella, M., Petrussa, E., Petruzzellis, F., Nardini, A., and Casolo, V.: The Possible Role of Non-Structural Carbohydrates in the Regulation of Tree Hydraulics, Int. J. Mol. Sci., 21, 144, https://doi.org/10.3390/ijms21010144, 2020.
Trugman, A. T., Detto, M., Bartlett, M. K., Medvigy, D., Anderegg, W. R. L., Schwalm, C., Schaffer, B., and Pacala, S. W.: Tree carbon allocation explains forest drought-kill and recovery patterns, Ecol. Lett., 21, 1552–1560, 2018.
Trugman, A. T., Anderegg, L. D. L., Sperry, J. S., Wang, Y., Venturas, M., and Anderegg, W. R. L.: Leveraging plant hydraulics to yield predictive and dynamic plant leaf allocation in vegetation models with climate change, Glob. Change Biol., 25, 4008–4021, https://doi.org/10.1111/gcb.14814, 2019.
Uriarte, M., Lasky, J. R., Boukili, V. K., and Chazdon, R. L.: A trait-mediated, neighbourhood approach to quantify climate impacts on successional dynamics of tropical rainforests, Funct. Ecol., 30, 157–167, 2016.
Vargas G., G., Brodribb, T. J., Dupuy, J. M., González‐M., R., Hulshof, C. M., Medvigy, D., Allerton, T. A. P., Pizano, C., Salgado‐Negret, B., Schwartz, N. B., Van Bloem, S. J., Waring, B. G., and Powers, J. S.: Beyond leaf habit: generalities in plant function across 97 tropical dry forest tree species, New Phytol., 232, 148–161, https://doi.org/10.1111/nph.17584, 2021.
Venturas, M. D., Todd, H. N., Trugman, A. T., and Anderegg, W. R.: Understanding and predicting forest mortality in the western United States using long-term forest inventory data and modeled hydraulic damage, New Phytol., 230, 1896–1910, 2021.
Wang, D., Heckathorn, S. A., Wang, X., and Philpott, S. M.: A meta-analysis of plant physiological and growth responses to temperature and elevated CO2, Oecologia, 169, 1–13, 2012.
Weng, E. S., Malyshev, S., Lichstein, J. W., Farrior, C. E., Dybzinski, R., Zhang, T., Shevliakova, E., and Pacala, S. W.: Scaling from individual trees to forests in an Earth system modeling framework using a mathematically tractable model of height-structured competition, Biogeosciences, 12, 2655–2694, https://doi.org/10.5194/bg-12-2655-2015, 2015.
Williams, A. P., Allen, C. D., Macalady, A. K., Griffin, D., Woodhouse, C. A., Meko, D. M., Swetnam, T. W., Rauscher, S. A., Seager, R., Grissino-Mayer, H. D., Dean, J. S., Cook, E. R., Gangodagamage, C., Cai, M., and McDowell, N. G.: Temperature as a potent driver of regional forest drought stress and tree mortality, Nat. Clim. Change, 3, 292–297, https://doi.org/10.1038/nclimate1693, 2012.
Williams, A. P., Seager, R., Berkelhammer, M., Macalady, A. K., Crimmins, M. A., Swetnam, T. W., Trugman, A. T., Buenning, N., Hryniw, N., McDowell, N. G., Noone, D., Mora, C. I., and Rahn, T.: Causes and Implications of Extreme Atmospheric Moisture Demand during the Record-Breaking 2011 Wildfire Season in the Southwestern United States, J. Appl. Meteorol. Clim., 53, 2671–2684, 2014.
Williams, L. J., Bunyavejchewin, S., and Baker, P. J.: Deciduousness in a seasonal tropical forest in western Thailand: interannual and intraspecific variation in timing, duration and environmental cues, Oecologia, 155, 571–582, 2008.
Wullschleger, S. D., Hanson, P. J., and Todd, D. E.: Transpiration from a multi-species deciduous forest as estimated by xylem sap flow techniques, Forest Ecol. Manag., 143, 205–213, 2001.
Xu, X., Medvigy, D., Powers, J. S., Becknell, J. M., and Guan, K.: Diversity in plant hydraulic traits explains seasonal and inter-annual variations of vegetation dynamics in seasonally dry tropical forests, New Phytol., 212, 80–95, 2016.
Xu, X., Longo, M., and Moorcroft, P.: ED-2.2-hydro (v1.0), Zenodo [code], https://doi.org/10.5281/zenodo.3978588, 2020.
Yang, Y., Hillebrand, H., Lagisz, M., Cleasby, I., and Nakagawa, S.: Low statistical power and overestimated anthropogenic impacts, exacerbated by publication bias, dominate field studies in global change biology, Glob. Change Biol., 28, 969–989, https://doi.org/10.1111/gcb.15972, 2022.
Zhu, K., Chiariello, N. R., Tobeck, T., Fukami, T., and Field, C. B.: Nonlinear, interacting responses to climate limit grassland production under global change, P. Natl. Acad. Sci. USA, 113, 10589–10594, 2016.
Zhu, S.-D., Chen, Y.-J., Ye, Q., He, P.-C., Liu, H., Li, R.-H., Fu, P.-L., Jiang, G.-F., and Cao, K.-F.: Leaf turgor loss point is correlated with drought tolerance and leaf carbon economics traits, Tree Physiol., 38, 658–663, https://doi.org/10.1093/treephys/tpy013, 2018.
Zscheischler, J., Mahecha, M. D., Von Buttlar, J., Harmeling, S., Jung, M., Rammig, A., Randerson, J. T., Schölkopf, B., Seneviratne, S. I., Tomelleri, E., Zaehle, S., and Reichstein, M.: A few extreme events dominate global interannual variability in gross primary production, Environ. Res. Lett., 9, 035001, https://doi.org/10.1088/1748-9326/9/3/035001, 2014.
- Abstract
- Introduction
- Vegetation demographic model (VDM) approaches
- Results
- Discussion
- Summary of perspectives for model advancement
- Code availability
- Data availability
- Author contributions
- Competing interests
- Disclaimer
- Special issue statement
- Acknowledgements
- Financial support
- Review statement
- References
- Supplement
- Abstract
- Introduction
- Vegetation demographic model (VDM) approaches
- Results
- Discussion
- Summary of perspectives for model advancement
- Code availability
- Data availability
- Author contributions
- Competing interests
- Disclaimer
- Special issue statement
- Acknowledgements
- Financial support
- Review statement
- References
- Supplement