the Creative Commons Attribution 4.0 License.
the Creative Commons Attribution 4.0 License.
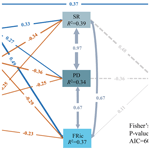
Contemporary biodiversity pattern is affected by climate change at multiple temporal scales in steppes on the Mongolian Plateau
Zijing Li
Zhiyong Li
Xuze Tong
Lei Dong
Ying Zheng
Jinghui Zhang
Bailing Miao
Lixin Wang
Liqing Zhao
Lu Wen
Guodong Han
Frank Yonghong Li
Cunzhu Liang
Present and historical climate conditions jointly determine contemporary biodiversity patterns and ecosystem functions. However, it remains unclear how contemporary climate and paleoclimate changes together affect the three dimensions of biodiversity (i.e., taxonomic diversity, functional diversity and phylogenetic diversity) and their relationship with ecosystem functions. Here, we assess the impact of current climate, paleoclimate and its anomalies on contemporary biodiversity and ecosystem functions. We estimated the taxonomic, functional and phylogenetic diversity of grassland on the Mongolian Plateau using vegetation survey data and trait information. We then used random forest and structural equation models to assess the relative importance of the present, the Mid-Holocene and the Last Glacial Maximum climate as well as paleoclimate changes as determinants of diversity and aboveground biomass. Our results showed that paleoclimate changes and modern climate jointly determined contemporary biodiversity patterns, while community biomass was mainly affected by modern climate, namely the aridity index. Modern aridity and temperature were two major influences on all three dimensions of biodiversity. Mid-Holocene climate anomalies had a strong effect on species richness and phylogenetic diversity, while functional diversity had mainly been affected by temperature anomalies since the Last Glacial Maximum. These findings suggest that contemporary biodiversity patterns may be affected by processes at divergent temporal scales. Our results show that simultaneously exploring the response of the three dimensions of biodiversity in different periods of climate change and the theoretical framework for its impact on community biomass is helpful to provide a more comprehensive understanding of patterns of biodiversity and its relationship with ecosystem functions.
- Article
(1997 KB) - Full-text XML
-
Supplement
(913 KB) - BibTeX
- EndNote
Global climate changes, such as increasing temperature and changing precipitation, are altering biodiversity patterns at an unprecedented rate and have serious consequences for ecosystem functions related to biodiversity changes (Mottl et al., 2021; Nolan et al., 2018; Ye et al., 2019). Ecosystem functioning associated with biodiversity as well as contemporary diversity patterns and their relationships may be regulated by modern climate, past climate and climate change (Fordham et al., 2020; Eiserhardt et al., 2015; Svenning et al., 2015).
Ecosystem functions are strongly influenced by biodiversity (Isbell et al., 2011; Hooper et al., 2005), but understanding the effects of different dimensions of biodiversity (i.e., taxonomic diversity, functional diversity and phylogenetic diversity) on ecosystem functions is still a challenge in ecology (Van Der Plas, 2019; Cadotte et al., 2008; Swenson, 2011). Traditionally, most studies of biodiversity and ecosystem functions have predominantly focused on the relationship between taxonomic diversity (i.e., species richness) and biomass or productivity. However, only considering taxonomic diversity has the limitation that it may ignore important ecological processes such as the formation of function traits or the evolutionary history of species (Flynn et al., 2011; Swenson, 2011). The importance of taking into account other aspects of biodiversity, including functional diversity and phylogenetic diversity, has increasingly been recognized. The effects of plant functional diversity on ecosystem functioning are largely driven by variations in plant functional traits, such as adult plant height and leaf size (Diaz et al., 2016). Phylogenetic diversity (PD) is a key driver of community assembly and ecosystem functions (Srivastava et al., 2012; Cavender-Bares et al., 2009). Previous research has shown that PD may be a better metric than species richness or functional diversity for predicting plant biomass (Cadotte et al., 2008). Communities with higher PD have stronger anti-interference ability because of their evolutionary potential to adapt to changing environmental conditions. However, the role of multi-dimensional biodiversity in driving ecosystem functions in response to climate change still needs further research. Elucidating the impacts of multi-dimensional biodiversity on biomass in a changing world can deepen our understanding of the direct and indirect effects of changes in climate and biodiversity on terrestrial ecosystems and improve predictions of the ecological consequences of global climate changes.
Changes in community biodiversity and composition caused by climate change have been explored in various ecosystems (Avolio et al., 2021), including tropical forests in the Amazon (Esquivel-Muelbert et al., 2019), alpine meadows on the Qinghai–Tibet Plateau (H. Liu et al., 2018) and a heterogeneous California grassland (Harrison et al., 2015). Dispersal limitation and environmental filtering (i.e., climatic conditions and paleoclimate change) play an important role in sorting species from the global species pool and in shaping large-scale diversity patterns (Kubota et al., 2018; Liu et al., 2021). Many studies have found that climate changes in the Mid-Holocene and the Quaternary interglacial period are the main driving factors of current species distribution, functional traits and community phylogeny (Svenning and Skov, 2007b). The current patterns of species richness and distribution of European flora are jointly affected by contemporary climatic conditions and climate change during the late Quaternary glacial–interglacial period (Svenning and Skov, 2007a, b). Contemporary plant functional traits and composition are highly sensitive to climate and are influenced by climate from tens of thousands of years ago, with important consequences for ecosystem functions (Blonder et al., 2018; Butler et al., 2017). For example, in Europe patterns of plant functional diversity exhibited prominent glacial–interglacial climate change imprints (Ordonez and Svenning, 2015; Ordonez and Svenning, 2017). This is partly due to the non-random removal of functional combinations that may have occurred during the Last Glacial Maximum (LGM; ∼ 21 000 years ago), increasing functional differences between native and migratory species, leading to smaller and dispersed functional spaces (Ordonez and Svenning, 2017). Evidence derived from the fossil pollen sequence and ecological data sets has shown that the acceleration of biodiversity changes began millennia ago, whereas vegetation changes during the Late Pleistocene to Early Holocene were driven primarily by changing climate (Mottl et al., 2021). In the South American tropics, the species composition and structure of Last Glacial Maximum forests were quite different from those of today, and biomass was also lower than that of contemporary forests (Mayle et al., 2009).
Furthermore, the effects of climate change on biodiversity should depend partly on climate displacement rate (climate change velocity) (Sandel et al., 2011). For example, phylogenetic clustering increased with increasing intensity of Quaternary glacial–interglacial climatic oscillations in South America and Africa (Kissling et al., 2012). There is evidence that vegetation changes in northern Europe were non-linear and varied greatly among regions in the Early Holocene–Mid-Holocene (Seddon et al., 2014). Paleoclimate simulations since 21 ka suggest that there are great similarities between past and future temperature changes in Eurasian temperate grasslands (Fordham et al., 2020). During the last glacial–interglacial transition period, global warming and related climate change led to changes in ecosystems to a degree comparable to the predicted warming under future high-emission scenarios (Nolan et al., 2018). Therefore, studying the impact of past climate and its effects on contemporary biodiversity patterns will help us understand the potential effects of future climate changes. However, most studies exploring the impact of present climate and paleoclimate drivers on ecosystem functions have focused on forest ecosystems, while grassland ecosystems remain understudied. In addition, it is not yet clear whether taxonomy, function and phylogenetic diversity will vary with climate gradients and climate changes, and the intensity of any such changes remains unclear. Therefore, there is an urgent need to consider the impact of current climate, paleoclimate and its anomalies on contemporary biodiversity and ecosystem functions.
The Mongolian Plateau is located in the arid and semi-arid area of eastern Eurasia. Our previous research has shown that climate is an important direct factor mediating the relationship between species richness and aboveground biomass in the Mongolian Plateau steppe (Li et al., 2021, 2020). However, in the late Quaternary, the Mongolian Plateau experienced severe alternating dry and wet climatic fluctuations (Maestre et al., 2021; Tian et al., 2017), which may have a significant impact on current plant diversity and ecosystem function. However, the impacts of climate in different periods and climate legacy effects on aboveground biomass of steppes in the Mongolian Plateau, as well as whether these effects are biodiversity dependent, remain to be evaluated. To address these knowledge gaps, we coupled data from a field survey of 152 sites in the Mongolian Plateau (Fig. S1 in the Supplement) to existing databases on present and past climates to explore present climate and the legacy effects of climate during the Last Glacial Maximum and the Mid-Holocene on current biodiversity and biomass.
Here, we aim to evaluate the relative importance of taxonomic, functional and phylogenetic diversity to community biomass of steppes in the Mongolian Plateau and to assess whether and how climate in different periods and its changes affect the three dimensions of diversity and their relationships with community biomass. We specifically ask the following questions. (1) Do the three dimensions of diversity affect community biomass, and if so, to what extent? (2) Do climate at divergent temporal scales and paleoclimatic changes affect different dimensions of biodiversity, and if so, how? (3) If there are effects, how do they affect the biodiversity–biomass relationship?
2.1 Study area
We conducted field surveys in the grasslands of the Mongolian Plateau, including the Inner Mongolia Autonomous Region in China and Mongolia, from June to August of 2014–2018 (Fig. S1). The Mongolian Plateau is located in the eastern part of Eurasia, with a geographic range of 37.61 to 53.35∘ N, 87.83 to 125.95∘ E, and an elevation range from 85 to 4203 m a.s.l. (above sea level). The mean annual temperature (MAT) of the study region ranges from −12 to 10 ∘C, and mean annual precipitation (MAP) ranges from 26 to 641 mm.
2.2 Data collection
2.2.1 Sites sampled and vegetation investigation
We investigated 152 grassland sites set in flat areas with little human interference and a relatively homogeneous environment. “Little human interference” mainly refers to the selection of areas with fewer human activities such as grazing for investigation. Specific indicators include the following aspects: (1) avoid choosing areas with manure of cattle and sheep; (2) dominant species such as Leymus chinensis and Stipa spp. are eaten less by livestock; (3) there were fewer degradation indicators. It mainly refers to the decrease in the number of established species or dominant species in the grassland, while the degeneration indicator plants such as Convolvulus ammannii, Potentilla acaulis, Artemisia frigida, Stellera chamaejasme and Vincetoxicum mongolicum appeared in large numbers. These sites were separated by about 50 km. According to the methods and protocols for plant community inventories proposed by Fang et al. (2009), most of the vegetation surveys in this study were conducted by setting one 10 m × 10 m quadrat at the respective site. However, in a few areas with sparse vegetation and large heterogeneity, we set three 10 m × 10 m quadrats to ensure the accuracy of the survey data. Within each quadrat, five 1 m × 1 m plots were placed at each corner and at the center of the quadrat to investigate vegetation. A total of 296 vascular plant species were recorded.
We measured height, density, coverage and standing biomass of each species in each plot. Three 1 m × 1 m plots along the diagonal line of the 10 m × 10 m quadrat were selected from the five plots to measure the standing biomass of each species. Plant species were cut at ground level and separately bagged, then oven-dried at 65 ∘C to constant weight, and finally weighed for dry matter.
2.2.2 Climate data
Mean annual temperature (MAT; ∘C) and mean annual precipitation (MAP; mm) were obtained from the CHELSA (Climatologies at High Resolution for the Earth's Land Surface Areas; http://chelsa-climate.org/, last access: 12 June 2022) database (Karger et al., 2017), a data set consisting of a monthly temperature and precipitation climatology from 1979 to 2013. Potential evapotranspiration (PET) was obtained from the CGIAR-CSI (Consortium of International Agricultural Research Centers Consortium for Spatial Information; https://cgiarcsi.community/, last access: 10 June 2022) based on latitude and longitude data for each site (Trabucco and Zomer, 2019). The resolution of both is 30 arcsec. Aridity was measured using the aridity index (AI; AI = MAPPET). Higher AI values indicate lower aridity and higher humidity. Paleoclimate data were downloaded from WorldClim (http://worldclim.com/paleo-climate1, last access: 6 June 2022) with a resolution of 2.5 min. The database was made available by CMIP5 (Coupled Model Intercomparison Project Phase 5) and was calibrated based on the current climate using WorldClim 1.4. The MAT and MAP of the Mid-Holocene (MID; about 6000 years ago) and the Last Glacial Maximum (LGM; about 22 000 years ago) were simulated based on CCMS4 (Community Climate System Model version 4). Climate anomaly (i.e., present-day values minus paleoclimate values) was used to indicate the degree of climate change since the MID and the LGM (Sandel et al., 2011). Temperature-change velocity from the LGM to the present (velocity; m yr−1) was obtained from DRYAD (https://datadryad.org, last access: 10 June 2022) (Sandel et al., 2011). The climate variables used in this study are shown in Table 1.
2.2.3 Plant functional traits
The functional traits of each plant species include growth form, life form, phyllotaxy, single or compound leaf, petiole, inflorescence, fruit type, flowering period, fruit ripening period, length of the flowering period, water ecotypes, leaf length, leaf width, and plant height (Table S1 in the Supplement). These traits were looked up in the Flora of Inner Mongolia (third edition) or Flora of China (online edition) (http://www.iplant.cn/frps, last access: 15 May 2022). The trait data for leaf length and leaf width were the mean values provided in Flora of China (Fig. S2). For example, if the leaf length of Leymus chinensis was described as 7–18 cm in the Flora of China, then leaf length was defined as 12.5 cm.
2.3 Aboveground biomass
The aboveground biomass of the community was calculated based on the dry-matter accumulation value (g m−2) of all plant samples in each plot, and the average aboveground biomass of each plot in each quadrat was calculated to represent the aboveground biomass of the plant community (g m−2).
2.4 Diversity calculations
2.4.1 Taxonomic diversity
We used species richness (SR), the Shannon–Wiener index and the Pielou index as measures of community taxonomic diversity. The species richness (number of species per square meter) at each site was calculated as the average number of species per plot. The Shannon–Wiener index and Pielou index were calculated using the “diversity” function in the vegan R package (Oksanen et al., 2022).
2.4.2 Functional diversity
The functional richness (FRic), functional evenness (FEve), functional divergence (FDiv), functional dispersion (FDis) and Rao index (RaoQ) were calculated based on the Gower distance of functional traits using the “dbFD” function in the FD R package (Laliberté and Legendre, 2010).
2.4.3 Phylogenetic diversity
All plant species' names recorded in surveys were checked in the World Flora Online (WFO) Plant List (https://wfo-about.rbge.info/plant-list/, last access: 20 May 2022) to obtain acceptable species names. Based on the APG III system (The Angiosperm Phylogeny Group, 2009), we entered the taxonomic information (family, genus, species) of all species into the Phylomatic online plant database (Phylomatic Version 3, http://phylodiversity.net/phylomatic/, last access: 25 May 2022), selected storedtree = “zanne2014” (Zanne et al., 2014, plants), and output a phylogenetic tree with branch length and differentiation time (Fig. S2). Faith's phylogenetic diversity (PD, the sum of the phylogenetic branch lengths), the mean pairwise distance (MPD) and the mean nearest taxon distance (MNTD) between species in a community were calculated using the “mpd” function in the picante R package (Kembel et al., 2010).
2.5 Data analysis
First, we conducted a random forest model to estimate the relative importance of the three dimensions of biodiversity to community biomass. The random forest model can alleviate multicollinearity and complex interactions between independent variables. We built 5000 regression trees in the random forest, and each tree was fitted with of the data. The other of the data were used to estimate the importance of each predictor variable, which was represented by the increase in mean square error (MSE). In the random forest model, the variables that ranked first in terms of taxonomic, functional and phylogenetic diversity were selected as proxies for the three dimensions of biodiversity.
To explore the influence of climate in different periods and climate anomalies on biodiversity, we also used the random forest model to analyze the relative importance of the present climate, the Mid-Holocene climate, the Last Glacial Maximum climate and paleoclimate change on the three dimensions of biodiversity. To evaluate the relative importance of climate drivers of biodiversity, we calculated the ratio between the increase in MSE of the predictor and the sum of all the increases in MSE, which is expressed as a percentage.
To study the effects of climate in different periods and climate anomalies on biodiversity and aboveground biomass, we performed piecewise structural equation models (pSEMs) to test the direct and indirect causal relationships between the climate in different periods and paleoclimate change, species diversity, functional diversity, phylogenetic diversity and biomass. Model construction procedures involved the following three stages. First, based on the results of the random forest model, we identified the climate variables that significantly affected biodiversity and divided them into composite variables. The model contained two composite variables that potentially represent collections of variables in terms of the present climate and paleoclimate change. We calculated Pearson correlations between the climate factors (Fig. S3) and deleted the predictors with high correlation coefficients (>0.85, P<0.05) to avoid multicollinearity. Before pSEM analysis, all selected predictors were standardized. Second, principal component analyses (PCAs) were used for the composite variables with multiple predictors. For each composite variable, the first principal component (PC1) explained 64 %–71 % of the total variance and was used in the subsequent pSEM analysis (Table S2). Third, pSEM was developed from the full conceptual model (Fig. S4). We fitted the component models of the pSEM as linear models and reported the standardized coefficient for each path from each component model. We used Shipley's d-separation test and Fisher's C statistic to evaluate the overall fit of the pSEM and Akaike information criteria (AIC) to select the best model. We used standardized path coefficients to measure the direct, indirect and total size of the effects of the present climate and paleoclimate changes on aboveground biomass (AGB).
We conducted all analyses in R x64 4.0.5. The random forest model was run using the “randomForest” function in the randomForest package (Cutler et al., 2007; Liaw and Winner, 2002), and the significance of the variables was tested using the “rp.importance” function in the rfPermute package (Archer, 2022). PCA was performed using the “princomp” function in the psych package in R (Revelle, 2021). Piecewise structural equation modeling was conducted and tested using the piecewiseSEM package (Lefcheck, 2016).
3.1 Effects of biodiversity on aboveground biomass
Among the 11 biodiversity variables, SR, PD and Pielou index identified by the random forest model were the three most significant predictors influencing aboveground biomass (Fig. 1a). Taken together, taxonomic diversity represented by SR and the Pielou and Shannon–Wiener indexes collectively contributed 43.78 % of the random forest model for aboveground biomass, while phylogenetic diversity represented by PD (including MNTD and MPD) and functional diversity represented by FRic (including FDiv, FEve, FDis and RaoQ) contributed 30.02 % and 26.2 %, respectively (Fig. 1b).
Based on the ranking results from the random forest model, SR, PD and FRic were selected as agents of taxonomic, phylogenetic and functional diversity, respectively, and used for subsequent analysis.
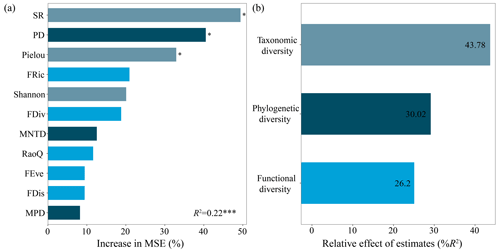
Figure 1The importance of each biodiversity indicator to aboveground biomass (a) and relative contributions of the three dimensions of biodiversity to aboveground biomass (b). An increase in mean square error (MSE) denotes an increase in the percentage of mean square error. Significance levels are as follows: * P<0.05 and P<0.001.
3.2 Paleoclimate and present climate predictors of contemporary diversity
Random forest models explained 35 % (cross-validation R2=0.35, P<0.001), 31 % (cross-validation R2=0.31, P<0.001) and 31 % (cross-validation R2=0.31, P<0.001) of the variance in SR, PD and FRic, respectively (Fig. 2). Temperatures in different periods, including MATmid, MAT and MATlgm, were the main factors driving SR and PD (P<0.05; Fig. 2a and b), followed by climate anomalies in various periods. However, the current climate (i.e., AI, MAT and MAP) had a stronger impact on FRic (P<0.05; Fig. 2c).
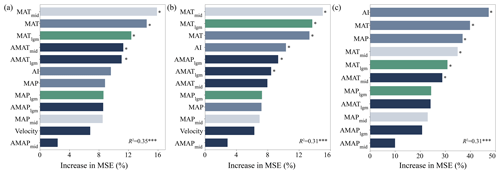
Figure 2The importance of climate variables in different periods and paleoclimate change to species richness (a), phylogenetic diversity (b) and functional richness (c). An increase in mean square error (MSE) denotes an increase in the percentage of mean square error. Significance levels are as follows: * P<0.05 and P<0.001.
In terms of relative contributions, the current climate and the paleoclimate changes had greater impacts on the three dimensions of biodiversity. Paleoclimate change was the best predictor of SR (33.90 %) and PD (32.10 %). Strikingly, the present climate (38.68 %) showed a larger contribution to the prediction of FRic than paleoclimate change (26.06 %; Fig. 3).
3.3 Influence of climate factors on biodiversity and aboveground biomass
The present climate, MAT of the Mid-Holocene and the Last Glacial Maximum, and paleoclimate change explained 38 % (R2=0.38), 33 % (R2=0.33), 36 % (R2=0.36) and 29 % (R2=0.29) of the variances in SR, PD, FRic and AGB, respectively (Fig. 4). The present climate had direct and indirect significant associations with AGB via all biodiversity variables (Fig. 4). The present climate was significantly and positively associated with SR (, P<0.05), PD (, P<0.05) and FRic (, P<0.05; Fig. 4). However, the paleoclimate change had direct significant negative effects on SR (, P<0.05), PD (, P<0.05) and FRic (, P<0.05; Fig. 4). MATmid and MATlgm had no significant effects on SR, PD, FRic and AGB (Fig. 4).
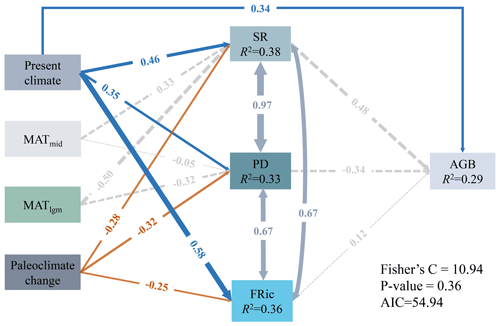
Figure 4pSEMs fitted to the effects of climate in different periods and paleoclimate change on diversity and AGB. The present climate is PCA component 1 of the AI and MAT. The paleoclimate change represents a composite variable including MAT anomaly from the Mid-Holocene to the present, MAT and MAP anomaly from the Last Glacial Maximum to the present. Numbers adjacent to arrows represent the standardized path coefficients (r∂). R2 indicates the proportion of variance explained. Solid blue (positive paths) and orange (negative paths) arrows indicate significant paths (P<0.05), and dashed gray arrows indicate non-significant paths (P>0.05).
For the present climate, the direct effects of AI on SR (, P<0.05), PD (, P<0.05), FRic (, P<0.05) and AGB (, P<0.05) were all significantly positive, while the direct effects of MAT on the three dimensions of biodiversity (SR: ; PD: ; FRic: ; P<0.05 in all cases) were all significantly negative (Fig. 5). In addition, SR (, P<0.05) and PD (, P<0.05) were negatively affected by the MAT anomaly from the Mid-Holocene to the present (AMATmid), but FRic (, P<0.05) was mainly driven by the MAT anomaly from the Last Glacial Maximum to the present (AMATlgm; Fig. 5). AI accounted for the largest proportion of variation in AGB (80.06 %), followed by MAT at 10.81 % (Table 2). The direct effect () of AI on AGB was greater than the indirect effect (; Table 2).
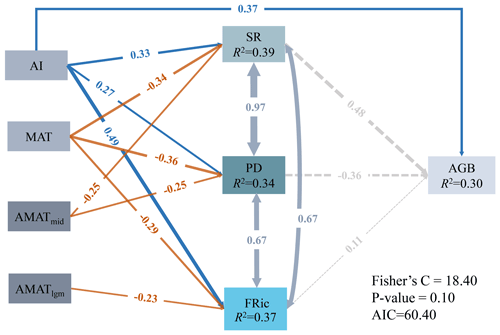
Figure 5The effects of present climate (AI and MAT) and paleoclimate change (AMATmid and AMATlgm) on diversity and AGB. Numbers adjacent to arrows represent the standardized path coefficients. R2 indicates the proportion of variance explained. Solid blue (positive paths) and orange (negative paths) arrows indicate significant paths (P<0.05), and dashed gray arrows indicate non-significant paths (P>0.05).
4.1 The effects of different dimensions of biodiversity on aboveground biomass
Our research showed that, without considering the influence of climatic conditions, taxonomic diversity was the most important factor influencing the aboveground biomass of Mongolian Plateau grasslands, followed by phylogenetic diversity and functional diversity. However, a large number of studies have emphasized the importance of phylogenetic diversity and functional diversity for ecosystem functions (Srivastava et al., 2012; Cadotte et al., 2008; Swenson et al., 2012). For example, research on subtropical forests in northern China showed that functional diversity is more important than taxonomic diversity in controlling aboveground biomass, which is related to the acquisitive resource use strategy of functional traits (Hanif et al., 2019). However, in Mongolian Plateau grasslands taxonomic diversity may be more critical. Selection effects and compensatory effects are the main mechanisms through which biodiversity impacts biomass. The grassland community structure of the Mongolian Plateau is relatively simple, and biomass mainly depends on the number or dominance of dominant species. However, functional traits considered in this study, such as leaf size and plant height, do not have a strong impact on biomass. The Mongolian Plateau is located in an arid and semi-arid area, and drought-tolerant species invest a lot in seed resources, leaf structure and root biomass (Wolf et al., 2021). Studies have shown that these traits are negatively correlated with aboveground biomass, while functional traits consistent with growth strategies, such as leaf nitrogen concentration and photosynthetic utilization efficiency, can promote aboveground biomass (Wolf et al., 2021).
Secondly, phylogenetic diversity also had a significant impact on aboveground biomass. Phylogenetic diversity contains the evolutionary information of species ecology. It is influenced by the average degree of correlation between species and the number of existing species and can reflect the evolutionary sequence of characteristics related to habitat and resource utilization (Srivastava et al., 2012). If species make better use of all available resources, productivity may increase. Therefore, our research suggests that protecting taxonomic diversity and conserving evolutionarily different species are equally important in effectively maintaining high-yielding communities.
4.2 Relationship between paleoclimate anomalies and current biodiversity patterns
Although a rich body of research has explored the factors affecting biodiversity patterns and the relationship between biodiversity and biomass (Van Der Plas, 2019; Tilman et al., 2012), it is not clear how current climate and paleoclimate together affect the relationship, especially in grassland communities. Here, our results showed that paleoclimate changes and present climate factors were critical variables influencing contemporary biodiversity patterns. This illustrates the importance of historical factors, such as evolution, as well as contemporary factors, such as climate, in determining the geographic ranges of species and the accumulation of species in the region (Fine, 2015). Previous studies aiming to explain patterns of biodiversity have focused on the current climate (Harrison et al., 2015), energy (Kreft and Jetz, 2007), soil attributions (Ulrich et al., 2014), human activities (Hautier et al., 2015; Newbold et al., 2015) or water availability (Jiao et al., 2021; Liu et al., 2021). Modern climate research on biodiversity and ecosystem functions has been widely confirmed (Walther et al., 2002; Liu et al., 2021; Yang et al., 2011), but our research shows that the pattern of biodiversity is also affected by past climates, and especially climate anomalies. Based on climate-related traits, climate anomalies filtered the regional species pool, which in turn affected contemporary biodiversity patterns.
Species richness is affected by the significant positive effects of AI and the significant negative effects of MAT and AMATmid. Jansson (2003) showed that the total number of vascular plant species had decreased with increasing temperature change since the LGM in mainland areas (Jansson, 2003), which is inconsistent with our results. We found that the species richness of Mongolian Plateau grassland had been more affected by temperature changes since the Mid-Holocene. In Europe, the richness of widespread species was largely controlled by the modern climate, while the LGM climate and climatic heterogeneity were codominant with modern climate as controls of richness for restricted and intermediate species, which appear to still be associated with their glacial refugia (Svenning and Skov, 2007b).
Phylogenetic diversity (PD) is usually used to quantify the uniqueness of a region's evolution. On longer timescales, the effects of climate on speciation and extinction may cumulatively affect current PD patterns (Svenning et al., 2015). Our research showed that PD was negatively correlated with MAT and AMATmid but not significantly related to temperature anomalies during the Last Glacial Maximum. However, the PD of global forest ecosystems is positively associated with temperature and precipitation and is negatively associated with Quaternary precipitation change (Kubota et al., 2018). This indicates the importance of paleoprecipitation variation in shaping the pattern of contemporary phylogenetic diversity in forest ecosystems, possibly by survival and recolonization from refugia along hydrological gradients (Blonder et al., 2018). Additionally, Quaternary glacial–interglacial climate oscillations led to the extinction of trees in many parts of the globe, for example in Australia (Macphail et al., 1995) and Europe (Svenning, 2003), affecting the PD of global forest ecosystems. It was shown that herbaceous plants had mainly been affected by climate change since the Mid-Holocene, while woody plants had mainly been affected by climate change since the LGM, which may be due to the differences in the morphology of herbaceous and woody plants (Y. Liu et al., 2018; Wang et al., 2021). Woody plants with large conduits are vulnerable to drought or freezing and cause clogging of conduits (Zanne et al., 2014) and thus were more susceptible to cold Quaternary climatic conditions. In contrast, herbs can mitigate the effects of extreme weather by producing underground buds and stems or by wilting of aboveground parts (Qian et al., 2017).
Our results suggested that functional richness was negatively related to the Last Glacial Maximum climate anomaly. This may be due to the intolerance of plant traits to past climatic conditions (Bhagwat and Willis, 2008) and the postglacial migration lag (Svenning et al., 2008). In the late Quaternary, the Mongolian Plateau experienced severe alternating dry and wet climate fluctuations (Tian et al., 2017) and likely experienced a non-random removal of functional combinations and change in functional diversity (Ordonez and Svenning, 2017). Previous research has indicated that the magnitude of past glacial-to-interglacial warming was sufficient at most locations across the globe (including the Mongolian Plateau) to drive moderate to large changes in vegetation composition and structure (Nolan et al., 2018). When climate changes, inappropriate species may be quickly ruled out, and appropriate species may migrate more slowly, resulting in a negative correlation between the paleoclimate anomaly and contemporary FD in the Mongolian Plateau. This is also limited by the spreading ability or migration potential of plants after the glaciers retreated, when species with inappropriate characteristics disappeared from the community and were not replaced by other species (Blonder et al., 2018). Studies in Europe indicate that the lagging effect of glacial–interglacial climate instability on functional diversity is > 10 000 years (Ordonez and Svenning, 2015).
4.3 Effects of climate on the relationship between biodiversity and biomass
The influence of climate on the relationship between contemporary diversity and biomass may be caused by a combination of climate change and human activities in the Mongolian Plateau grasslands. In the eastern part of the Mongolian Plateau, the interval of 35 230 to 25 150 years BP was a forest–steppe landscape under humid conditions, represented by Pinaceae, Compositae and Chenopodiaceae (Tian et al., 2017). Subsequently, the forest patches gradually shrank, the vegetation transitioned to the steppe landscape, and drought began to intensify. During the Last Glacial Maximum period, the vegetation types on the Mongolian Plateau were mainly polar, alpine and temperate deserts (Ray and Adams, 2001), and the main plants were Compositae and Chenopodiaceae. By the Holocene, xerophytes had declined sharply, and plants of humid climates had increased. The humidity increased during the Early Holocene, and generally humid conditions lasted from 10 400 until 7000 years BP; the region experienced the Holocene climatic optimum (Lavliakan humid phase; Lioubimtseva, 2004; Yin et al., 2011), with more hygrophilous species, such as Cyperaceae (Tian et al., 2017). This climate condition was also conducive to the development and spread of Compositae and Gramineae families in the steppes of Central Asia (Lioubimtseva, 2004). From approximately 5200 years BP to present, the climate has become more arid, with corresponding vegetation change and strong eolian activity (Yin et al., 2011).
As far as aboveground biomass is concerned, after taking into account climatic factors, it is mainly directly affected by the aridity index, while the three dimensions of biodiversity have no significant impact on it, which is consistent with our previous research (Li et al., 2020). A meta-analysis of 40 global grassland plant communities showed that the response of plant diversity to climate warming was an important factor in determining grassland aboveground biomass (Shao et al., 2021). Similarly, paleoclimate changes also have no direct effects on aboveground biomass, which indirectly affects aboveground biomass through regulation of biodiversity. This is mainly because paleoclimatic filtering can alter the historical legacies of species and functional diversity (removal or accumulation over time of species and functional states) through evolution, extinction or recolonization (Svenning et al., 2015). Legacies in the species and functional-trait pool further affect ecosystem function, leading to changes in biomass (Svenning et al., 2015).
In addition, the relationship between biodiversity and biomass is also affected by human activities (Buisson et al., 2022). Human history has become profoundly intertwined with grassland communities, from the evolution of the Homo in savannas 2 million years ago to the emergence of species domestication in agricultural societies 10 000 years ago (Strömberg and Staver, 2022). Paleoecological and archeological evidence shows that people have shaped most of terrestrial nature for at least 12 000 years through activities like burning, hunting, cultivation and domestication (Ellis et al., 2021). In the Mongolian Plateau, especially in the Inner Mongolia region of northern China, grazing appeared at ∼ 5.7–5.5 ka and further intensified again after ∼ 4.2–4.0 ka (Huang et al., 2021). A large number of studies have shown that the intensification of grazing activities also causes changes in biodiversity and biomass (Liang et al., 2021; Herrero-Jáuregui and Oesterheld, 2018; Díaz et al., 2007). Therefore, there is an urgent need for future research to develop systematic analytical frameworks to assess the importance of paleoclimate and climate change in shaping contemporary biodiversity and ecosystems, particularly under the influence of human activities.
We conclude that unique evolutionary and ecological histories played key roles in explaining biodiversity patterns in the Mongolian Plateau. When climatic conditions are not considered, taxonomic diversity, phylogenetic diversity and functional diversity all have effects on community biomass, and taxonomic diversity has a more obvious impact on biomass. However, the climate after the Last Glacial Maximum has left a strong legacy affecting contemporary biodiversity patterns. These findings extend our understanding of the spatial and temporal scale of climate effects on biodiversity and aboveground biomass, providing additional confidence that the paleoclimate had a key role in shaping contemporary biodiversity patterns.
The data that support the findings of this study are available on request from the corresponding author.
The supplement related to this article is available online at: https://doi.org/10.5194/bg-20-2869-2023-supplement.
ZiL analyzed the data and wrote the first draft of the manuscript. ZhL, JZ, GH, FYL and CL contributed to later versions of the manuscript, and all authors provided constructive comments to improve the manuscript. FYL and CL designed and led the field survey. ZiL, ZhL, XT, JZ, LD, YZ, BM, WM, LZ, LW, LW, CL and FYL conducted the experiment and collected the data.
The contact author has declared that none of the authors has any competing interests.
Publisher's note: Copernicus Publications remains neutral with regard to jurisdictional claims in published maps and institutional affiliations.
This study was supported by the National Natural Science Foundation of China (grant no. 31960261); the Basic Scientific Research Foundation special project of the Institute of Water Resources and Hydropower Research (grant no. MK2023J01); the Science and Technology program of the Inner Mongolia Autonomous Region of China (grant no. 2020GG0126); the Natural Science Foundation of the Inner Mongolia Autonomous Region of China (grant no. 2020MS03026); the Major Science and Technology project of the Inner Mongolia Autonomous Region of China (grant no. zdzx2018020); the Inner Mongolia Autonomous Region Science and Technology Achievement Transformation project (grant no. 2019CG069); the innovative team of the Ministry of Education of China (grant no. IRT_17R59); and Yinshanbeilu Grassland Eco-hydrology National Observation and Research Station, China Institute of Water Resources and Hydropower Research (grant no. YSS202108).
This paper was edited by Nicolas Brüggemann and reviewed by two anonymous referees.
Archer, E.: rfPermute: Estimate Permutation p-Values for Random Forest Importance Metrics, R package version 2.5.1, https://CRAN.R-project.org/package=rfPermute (last access: 13 July 2023), 2022.
Avolio, M. L., Komatsu, K. J., Collins, S. L., Grman, E., Koerner, S. E., Tredennick, A. T., Wilcox, K. R., Baer, S., Boughton, E. H., Britton, A. J., Foster, B., Gough, L., Hovenden, M., Isbell, F., Jentsch, A., Johnson, D. S., Knapp, A. K., Kreyling, J., Langley, J. A., Lortie, C., McCulley, R. L., McLaren, J. R., Reich, P. B., Seabloom, E. W., Smith, M. D., Suding, K. N., Suttle, K. B., and Tognetti, P. M.: Determinants of community compositional change are equally affected by global change, Ecol. Lett., 24, 1892–1904, https://doi.org/10.1111/ele.13824, 2021.
Bhagwat, S. A. and Willis, K. J.: Species persistence in northerly glacial refugia of Europe: a matter of chance or biogeographical traits?, Biogeography, 35, 464–482, https://doi.org/10.1111/j.1365-2699.2007.01861.x, 2008.
Blonder, B., Enquist, B. J., Graae, B. J., Kattge, J., Maitner, B. S., Morueta-Holme, N., Ordonez, A., Simova, I., Singarayer, J., Svenning, J. C., Valdes, P. J., and Violle, C.: Late Quaternary climate legacies in contemporary plant functional composition, Glob. Change Biol., 24, 4827–4840, https://doi.org/10.1111/gcb.14375, 2018.
Buisson, E., Archibald, S., Fidelis, A., and Suding, K. N.: Ancient grasslands guide ambitious goals in grassland restoration, Science, 377, 594–598, https://doi.org/10.1126/science.abo4605, 2022.
Butler, E. E., Datta, A., Flores-Moreno, H., Chen, M., Wythers, K. R., Fazayeli, F., Banerjee, A., Atkin, O. K., Kattge, J., Amiaud, B., Blonder, B., Boenisch, G., Bond-Lamberty, B., Brown, K. A., Byun, C., Campetella, G., Cerabolini, B. E. L., Cornelissen, J. H. C., Craine, J. M., Craven, D., de Vries, F. T., Díaz, S., Domingues, T. F., Forey, E., González-Melo, A., Gross, N., Han, W., Hattingh, W. N., Hickler, T., Jansen, S., Kramer, K., Kraft, N. J. B., Kurokawa, H., Laughlin, D. C., Meir, P., Minden, V., Niinemets, Ü., Onoda, Y., Peñuelas, J., Read, Q., Sack, L., Schamp, B., Soudzilovskaia, N. A., Spasojevic, M. J., Sosinski, E., Thornton, P. E., Valladares, F., van Bodegom, P. M., Williams, M., Wirth, C., and Reich, P. B.: Mapping local and global variability in plant trait distributions, P. Natl. Acad. Sci. USA, 114, E10937–E10946, https://doi.org/10.1073/pnas.1708984114, 2017.
Cadotte, M. W., Cardinale, B. J., and Oakley, T. H.: Evolutionary history and the effect of biodiversity on plant productivity, P. Natl. Acad. Sci. USA, 105, 17012–17017, https://doi.org/10.1073/pnas.0805962105, 2008.
Cavender-Bares, J., Kozak, K. H., Fine, P. V., and Kembel, S. W.: The merging of community ecology and phylogenetic biology, Ecol. Lett., 12, 693–715, https://doi.org/10.1111/j.1461-0248.2009.01314.x, 2009.
Cutler, D. R., Edwards, J. T. C., Beard, K. H., Cutler, A., Hess, K. T., Gibson, J., and Lawler, J. J.: Random forest for classification ecology, Ecology, 88, 2783–2792, https://doi.org/10.1890/07-0539.1 2007.
Diaz, S., Kattge, J., Cornelissen, J. H., Wright, I. J., Lavorel, S., Dray, S., Reu, B., Kleyer, M., Wirth, C., Prentice, I. C., Garnier, E., Bonisch, G., Westoby, M., Poorter, H., Reich, P. B., Moles, A. T., Dickie, J., Gillison, A. N., Zanne, A. E., Chave, J., Wright, S. J., Sheremet'ev, S. N., Jactel, H., Baraloto, C., Cerabolini, B., Pierce, S., Shipley, B., Kirkup, D., Casanoves, F., Joswig, J. S., Gunther, A., Falczuk, V., Ruger, N., Mahecha, M. D., and Gorne, L. D.: The global spectrum of plant form and function, Nature, 529, 167–171, https://doi.org/10.1038/nature16489, 2016.
Díaz, S., Lavorel, S., McIntyre, S. U. E., Falczuk, V., Casanoves, F., Milchunas, D. G., Skarpe, C., Rusch, G., Sternberg, M., Noy-Meir, I., Landsberg, J., Zhang, W. E. I., Clark, H., and Campbell, B. D.: Plant trait responses to grazing – a global synthesis, Glob. Change Biol., 13, 313–341, https://doi.org/10.1111/j.1365-2486.2006.01288.x, 2007.
Eiserhardt, W. L., Borchsenius, F., Plum, C. M., Ordonez, A., and Svenning, J. C.: Climate-driven extinctions shape the phylogenetic structure of temperate tree floras, Ecol. Lett., 18, 263–272, https://doi.org/10.1111/ele.12409, 2015.
Ellis, E. C., Gauthier, N., Klein Goldewijk, K., Bliege Bird, R., Boivin, N., Diaz, S., Fuller, D. Q., Gill, J. L., Kaplan, J. O., Kingston, N., Locke, H., McMichael, C. N. H., Ranco, D., Rick, T. C., Shaw, M. R., Stephens, L., Svenning, J. C., and Watson, J. E. M.: People have shaped most of terrestrial nature for at least 12,000 years, P. Natl. Acad. Sci. USA, 118, e2023483118, https://doi.org/10.1073/pnas.2023483118, 2021.
Esquivel-Muelbert, A., Baker, T. R., Dexter, K. G., Lewis, S. L., Brienen, R. J. W., Feldpausch, T. R., Lloyd, J., Monteagudo-Mendoza, A., Arroyo, L., Alvarez-Davila, E., Higuchi, N., Marimon, B. S., Marimon-Junior, B. H., Silveira, M., Vilanova, E., Gloor, E., Malhi, Y., Chave, J., Barlow, J., Bonal, D., Davila Cardozo, N., Erwin, T., Fauset, S., Herault, B., Laurance, S., Poorter, L., Qie, L., Stahl, C., Sullivan, M. J. P., Ter Steege, H., Vos, V. A., Zuidema, P. A., Almeida, E., Almeida de Oliveira, E., Andrade, A., Vieira, S. A., Aragao, L., Araujo-Murakami, A., Arets, E., Aymard, C. G., Baraloto, C., Camargo, P. B., Barroso, J. G., Bongers, F., Boot, R., Camargo, J. L., Castro, W., Chama Moscoso, V., Comiskey, J., Cornejo Valverde, F., Lola da Costa, A. C., Del Aguila Pasquel, J., Di Fiore, A., Fernanda Duque, L., Elias, F., Engel, J., Flores Llampazo, G., Galbraith, D., Herrera Fernandez, R., Honorio Coronado, E., Hubau, W., Jimenez-Rojas, E., Lima, A. J. N., Umetsu, R. K., Laurance, W., Lopez-Gonzalez, G., Lovejoy, T., Aurelio Melo Cruz, O., Morandi, P. S., Neill, D., Nunez Vargas, P., Pallqui Camacho, N. C., Parada Gutierrez, A., Pardo, G., Peacock, J., Pena-Claros, M., Penuela-Mora, M. C., Petronelli, P., Pickavance, G. C., Pitman, N., Prieto, A., Quesada, C., Ramirez-Angulo, H., Rejou-Mechain, M., Restrepo Correa, Z., Roopsind, A., Rudas, A., Salomao, R., Silva, N., Silva Espejo, J., Singh, J., Stropp, J., Terborgh, J., Thomas, R., Toledo, M., Torres-Lezama, A., Valenzuela Gamarra, L., van de Meer, P. J., van der Heijden, G., van der Hout, P., Vasquez Martinez, R., Vela, C., Vieira, I. C. G., and Phillips, O. L.: Compositional response of Amazon forests to climate change, Glob. Change Biol., 25, 39–56, https://doi.org/10.1111/gcb.14413, 2019.
Fang, J.-Y., Wang, X.-P., Shen, Z.-H., Tang, Z.-Y., He, J.-S., Yu, D., Jiang, Y., Wang, Z.-H., Zheng, C.-Y., Zhu, J.-L., and Guo, Z.-D.: Methods and protocols for plant community inventory, Biodiversity Science, 17, 533–548, https://www.biodiversity-science.net/EN/10.3724/SP.J.1003.2009.09253 (last access: 13 July 2023), 2009.
Fine, P. V. A.: Ecological and Evolutionary Drivers of Geographic Variation in Species Diversity, Annu. Rev. Ecol. Evol. S., 46, 369–392, https://doi.org/10.1146/annurev-ecolsys-112414-054102, 2015.
Flynn, D. F. B., Mirotchnick, N., Jain, M., Palmer, M. I., and Naeem, S.: Functional and phylogenetic diversity as predictors of biodiversity-ecosystem-function relationships, Ecology, 92, 1573–1581, https://doi.org/10.1890/10-1245.1, 2011.
Fordham, D. A., Jackson, S. T., Brown, S. C., Huntley, B., Brook, B. W., Dahl-Jensen, D., Gilbert, M. T. P., Otto-Bliesner, B. L., Svensson, A., Theodoridis, S., Wilmshurst, J. M., Buettel, J. C., Canteri, E., McDowell, M., Orlando, L., Pilowsky, J., Rahbek, C., and Nogues-Bravo, D.: Using paleo-archives to safeguard biodiversity under climate change, Science, 369, eabc5654, https://doi.org/10.1126/science.abc5654, 2020.
Hanif, M. A., Yu, Q., Rao, X., and Shen, W.: Disentangling the Contributions of Plant Taxonomic and Functional Diversities in Shaping Aboveground Biomass of a Restored Forest Landscape in Southern China, Plants, 8, 612, https://doi.org/10.3390/plants8120612, 2019.
Harrison, S. P., Gornish, E. S., and Copeland, S.: Climate-driven diversity loss in a grassland community, P. Natl. Acad. Sci. USA, 112, 8672–8677, https://doi.org/10.1073/pnas.1502074112, 2015.
Hautier, Y., Tilman, D., Isbell, F., Seabloom, E. W., Borer, E. T., and Reich, P. B.: Anthropogenic environmental changes affect ecosystem stability via biodiversity, Science, 348, 336–340, https://doi.org/10.1126/science.aaa1788, 2015.
Herrero-Jáuregui, C. and Oesterheld, M.: Effects of grazing intensity on plant richness and diversity: a meta-analysis, Oikos, 127, 757–766, https://doi.org/10.1111/oik.04893, 2018.
Hooper, D. U., Chapin, F. S., Ewel, J. J., Hector, A., Inchausti, P., Lavorel, S., Lawton, J. H., Lodge, D. M., Loreau, M., Naeem, S., Schmid, B., Setälä, H., Symstad, A. J., Vandermeer, J., and Wardle, D. A.: Effects of biodiversity on ecosystem functioning: a consensus of current knowledge, Ecol. Monogr., 75, 3–35, https://doi.org/10.1890/04-0922, 2005.
Huang, X., Zhang, J., Ren, L., Zhang, S., and Chen, F.: Intensification and Driving Forces of Pastoralism in Northern China 5.7 ka Ago, Geophys. Res. Lett., 48, e2020GL092288, https://doi.org/10.1029/2020GL092288, 2021.
Isbell, F., Calcagno, V., Hector, A., Connolly, J., Harpole, W. S., Reich, P. B., Scherer-Lorenzen, M., Schmid, B., Tilman, D., van Ruijven, J., Weigelt, A., Wilsey, B. J., Zavaleta, E. S., and Loreau, M.: High plant diversity is needed to maintain ecosystem services, Nature, 477, 199–202, https://doi.org/10.1038/nature10282, 2011.
Jansson, R.: Global patterns in endemism explained by past climatic change, P. Roy. Soc. B-Biol. Sci., 270, 583–590, https://doi.org/10.1098/rspb.2002.2283, 2003.
Jiao, W., Wang, L., Smith, W. K., Chang, Q., Wang, H., and D'Odorico, P.: Observed increasing water constraint on vegetation growth over the last three decades, Nat. Commun., 12, 3777, https://doi.org/10.1038/s41467-021-24016-9, 2021.
Karger, D. N., Conrad, O., Bohner, J., Kawohl, T., Kreft, H., Soria-Auza, R. W., Zimmermann, N. E., Linder, H. P., and Kessler, M.: Climatologies at high resolution for the earth's land surface areas, Sci. Data, 4, 170122, https://doi.org/10.1038/sdata.2017.122, 2017.
Kembel, S. W., Cowan, P. D., Helmus, M. R., Cornwell, W. K., Morlon, H., Ackerly, D. D., Blomberg, S. P., and Webb, C. O.: Picante: R tools for integrating phylogenies and ecology, Bioinformatics, 26, 1463–1464, https://doi.org/10.1093/bioinformatics/btq166, 2010.
Kissling, W. D., Eiserhardt, W. L., Baker, W. J., Borchsenius, F., Couvreur, T. L., Balslev, H., and Svenning, J. C.: Cenozoic imprints on the phylogenetic structure of palm species assemblages worldwide, P. Natl. Acad. Sci. USA, 109, 7379–7384, https://doi.org/10.1073/pnas.1120467109, 2012.
Kreft, H. and Jetz, W.: Global patterns and determinants of vascular plant diversity, P. Natl. Acad. Sci. USA, 104, 5925–5930, https://doi.org/10.1073/pnas.0608361104, 2007.
Kubota, Y., Kusumoto, B., Shiono, T., Ulrich, W., and Duarte, L.: Environmental filters shaping angiosperm tree assembly along climatic and geographic gradients, J. Veg. Sci., 29, 607–618, https://doi.org/10.1111/jvs.12648, 2018.
Laliberté, E. and Legendre, P.: A distance-based framework for measuring functional diversity from multiple traits, Ecology, 91, 299–305, https://doi.org/10.1890/08-2244.1, 2010.
Lefcheck, J. S.: piecewiseSEM: Piecewise structural equation modelling in r for ecology, evolution, and systematics, Meth. Ecol. Evol., 7, 573–579, https://doi.org/10.1111/2041-210x.12512, 2016.
Li, Z., Li, Z., Tong, X., Zhang, J., Dong, L., Zheng, Y., Ma, W., Zhao, L., Wang, L., Wen, L., Dang, Z., Tuvshintogtokh, I., Liang, C., and Li, F. Y.: Climatic humidity mediates the strength of the species richness–biomass relationship on the Mongolian Plateau steppe, Sci. Total Environ., 718, 137252, https://doi.org/10.1016/j.scitotenv.2020.137252, 2020.
Li, Z., Liang, M., Li, Z., Mariotte, P., Tong, X., Zhang, J., Dong, L., Zheng, Y., Ma, W., Zhao, L., Wang, L., Wen, L., Tuvshintogtokh, I., Gornish, E. S., Dang, Z., Liang, C., Li, F. Y., and Schöb, C.: Plant functional groups mediate effects of climate and soil factors on species richness and community biomass in grasslands of Mongolian Plateau, J. Plant Ecol., 14, 679–691, https://doi.org/10.1093/jpe/rtab021, 2021.
Liang, M., Liang, C., Hautier, Y., Wilcox, K. R., and Wang, S.: Grazing-induced biodiversity loss impairs grassland ecosystem stability at multiple scales, Ecol. Lett., 24, 2054–2064, https://doi.org/10.1111/ele.13826, 2021.
Liaw, A. and Wiener, M.: Classification and Regression by randomForest, R News, 2, 18–22, https://CRAN.R-project.org/doc/Rnews/ (last access: 14 July 2023), 2002.
Lioubimtseva, E.: Climate change in arid environments: revisiting the past to understand the future, Prog. Phys. Geogr., 28, 502–530, https://doi.org/10.1191/0309133304pp422oa, 2004.
Liu, D., Zhang, C., Ogaya, R., Fernández-Martínez, M., Pugh, T. A. M., and Peñuelas, J.: Increasing climatic sensitivity of global grassland vegetation biomass and species diversity correlates with water availability, New Phytol., 230, 1761–1771, https://doi.org/10.1111/nph.17269, 2021.
Liu, H., Mi, Z., Lin, L., Wang, Y., Zhang, Z., Zhang, F., Wang, H., Liu, L., Zhu, B., Cao, G., Zhao, X., Sanders, N. J., Classen, A. T., Reich, P. B., and He, J. S.: Shifting plant species composition in response to climate change stabilizes grassland primary production, P. Natl. Acad. Sci. USA, 115, 4051–4056, https://doi.org/10.1073/pnas.1700299114, 2018.
Liu, Y., Su, X., Shrestha, N., Xu, X., Wang, S., Li, Y., Wang, Q., Sandanov, D., and Wang, Z.: Effects of contemporary environment and Quaternary climate change on drylands plant diversity differ between growth forms, Ecography, 42, 334–345, https://doi.org/10.1111/ecog.03698, 2018.
MacPhail, M. K., Colhoun, E. A., and Fitzsimons, S. J.: Key Periods in the Evolution of the Cenozoic Vegetation and Flora in Western Tasmania: the Late Pliocene, Aust. J. Bot., 43, 505–526, https://doi.org/10.1071/bt9950505, 1995.
Maestre, F. T., Benito, B. M., Berdugo, M., Concostrina-Zubiri, L., Delgado-Baquerizo, M., Eldridge, D. J., Guirado, E., Gross, N., Kefi, S., Le Bagousse-Pinguet, Y., Ochoa-Hueso, R., and Soliveres, S.: Biogeography of global drylands, New Phytol., 231, 540–558, https://doi.org/10.1111/nph.17395, 2021.
Mayle, F. E., Burn, M. J., Power, M., and Urrego, D. H.: Vegetation and Fire at the Last Glacial Maximum in Tropical South America, in: Past Climate Variability in South America and Surrounding Regions, edited by: Vimeux, F., Sylvestre, F., and Khodri, M., Developments in Paleoenvironmental Research, Vol. 14, Springer, Dordrecht, https://doi.org/10.1007/978-90-481-2672-9_4, 2009.
Mottl, O., Flantua, S. G. A., Bhatta, K. P., Felde, V. A., Giesecke, T., Goring, S., Grimm6, E. C., Haberle, S., Hooghiemstra, H., Ivory, S., Kuneš, P., Wolters, S., Seddon, A. W. R., and Williams, J. W.: Global acceleration in rates of vegetation change over the past 18,000 years, Science, 372, 860–864, https://doi.org/10.1126/science.abg1685, 2021.
Newbold, T., Hudson, L. N., Hill, S. L., Contu, S., Lysenko, I., Senior, R. A., Borger, L., Bennett, D. J., Choimes, A., Collen, B., Day, J., De Palma, A., Diaz, S., Echeverria-Londono, S., Edgar, M. J., Feldman, A., Garon, M., Harrison, M. L., Alhusseini, T., Ingram, D. J., Itescu, Y., Kattge, J., Kemp, V., Kirkpatrick, L., Kleyer, M., Correia, D. L., Martin, C. D., Meiri, S., Novosolov, M., Pan, Y., Phillips, H. R., Purves, D. W., Robinson, A., Simpson, J., Tuck, S. L., Weiher, E., White, H. J., Ewers, R. M., Mace, G. M., Scharlemann, J. P., and Purvis, A.: Global effects of land use on local terrestrial biodiversity, Nature, 520, 45–50, https://doi.org/10.1038/nature14324, 2015.
Nolan, C., Overpeck, J. T., Allen, J. R. M., Anderson, P. M., Betancourt, J. L., Binney, H. A., Brewer, S., Bush, M. B., Chase, B. M., Cheddadi, R., Djamali, M., Dodson, J., Edwards, M. E., Gosling, W. D., Haberle, S., Hotchkiss, S. C., Huntley, B., Ivory, S. J., Kershaw, A. P., Kim, S.-H., Latorre, C., Leydet, M., Lézine, A.-M., Liu, K.-B., Liu, Y., Lozhkin, A. V., McGlone, M. S., Marchant, R. A., Momohara, A., Moreno, P. I., Müller, S., Otto-Bliesner, B. L., Shen, C., Stevenson, J., Takahara, H., Tarasov, P. E., Tipton, J., Vincens, A., Weng, C., Xu, Q., Zheng, Z., and Jackson, S. T.: Past and future global transformation of terrestrial ecosystems under climate change, Science, 361, 920–923, https://doi.org/10.1126/science.aan5360, 2018.
Oksanen, J., Simpson, G., Blanchet, F., Kindt, R., Legendre, P., Minchin, P., O'Hara, R., Solymos, P., Stevens, M., Szoecs, E., Wagner, H., Barbour, M., Bedward, M., Bolker, B., Borcard, D., Carvalho, G., Chirico, M., De Caceres, M., Durand, S., Evangelista, H., FitzJohn, R., Friendly, M., Furneaux, B., Hannigan, G., Hill, M., Lahti, L., McGlinn, D., Ouellette, M., Ribeiro Cunha, E., Smith, T., Stier, A., Ter Braak, C., and Weedon, J.: vegan: Community Ecology Package_, R package version 2.6-4, https://CRAN.R-project.org/package=vegan (last access: 13 July 2023), 2022.
Ordonez, A. and Svenning, J.-C.: Geographic patterns in functional diversity deficits are linked to glacial-interglacial climate stability and accessibility, Global Ecol. Biogeogr., 24, 826–837, https://doi.org/10.1111/geb.12324, 2015.
Ordonez, A. and Svenning, J. C.: Consistent role of Quaternary climate change in shaping current plant functional diversity patterns across European plant orders, Sci. Rep.-UK, 7, 42988, https://doi.org/10.1038/srep42988, 2017.
Qian, H., Jin, Y., and Ricklefs, R. E.: Phylogenetic diversity anomaly in angiosperms between eastern Asia and eastern North America, P. Natl. Acad. Sci. USA, 114, 11452–11457, https://doi.org/10.1073/pnas.1703985114, 2017.
Ray, N. and Adams, J. M.: A GIS-based Vegetation Map of the World at the Last Glacial Maximum (25,000–15,000 BP), Internet Archaeology, 11, 1–44, https://doi.org/10.11141/ia.11.2, 2001.
Revelle, W.: Package “psych”: Procedures for Psychological, Psychometric, and Personality Research, https://CRAN.R-project.org/package=psych (last access: 13 July 2023), 2021.
Sandel, B., Arge, L., Dalsgaard, B., Davies, R. G., Gaston, K. J., Sutherland, W. J., and Svenning, J. C.: The influence of Late Quaternary climate-change velocity on species endemism, Science, 334, 660–664, https://doi.org/10.1126/science.1210173, 2011.
Seddon, A. W. R., Macias-Fauria, M., and Willis, K. J.: Climate and abrupt vegetation change in Northern Europe since the last deglaciation, Holocene, 25, 25–36, https://doi.org/10.1177/0959683614556383, 2014.
Shao, J., Zhou, X., Groenigen, K. J., Zhou, G., Zhou, H., Zhou, L., Lu, M., Xia, J., Jiang, L., Hungate, B. A., Luo, Y., He, F., Thakur, M. P., and Mayfield, M.: Warming effects on grassland productivity depend on plant diversity, Global Ecol. Biogeogr., 31, 588–598, https://doi.org/10.1111/geb.13441, 2021.
Srivastava, D. S., Cadotte, M. W., MacDonald, A. A., Marushia, R. G., and Mirotchnick, N.: Phylogenetic diversity and the functioning of ecosystems, Ecol. Lett., 15, 637–648, https://doi.org/10.1111/j.1461-0248.2012.01795.x, 2012.
Strömberg, C. A. E. and Staver, A. C.: The history and challenge of grassy biomes-Grassy biomes are > 20 million years old but are undervalued and under threat today, Science, 377, 592–593, https://doi.org/10.1126/science.add1347, 2022.
Svenning, J.-C.: Deterministic Plio-Pleistocene extinctions in the European cool-temperate tree flora, Ecol. Lett., 6, 646–653, https://doi.org/10.1046/j.1461-0248.2003.00477.x, 2003.
Svenning, J.-C. and Skov, F.: Could the tree diversity pattern in Europe be generated by postglacial dispersal limitation?, Ecol. Lett., 10, 453–460, https://doi.org/10.1111/j.1461-0248.2007.01038.x, 2007a.
Svenning, J.-C. and Skov, F.: Ice age legacies in the geographical distribution of tree species richness in Europe, Global Ecol. Biogeogr., 16, 234–245, https://doi.org/10.1111/j.1466-8238.2006.00280.x, 2007b.
Svenning, J.-C., Normand, S., and Skov, F.: Postglacial dispersal limitation of widespread forest plant species innemoral Europe, Ecography, 31, 316–326, https://onlinelibrary.wiley.com/doi/epdf/10.1111/j.0906-7590.2008.05206.x (last access: 13 July 2023), 2008.
Svenning, J.-C., Eiserhardt, W. L., Normand, S., Ordonez, A., and Sandel, B.: The Influence of Paleoclimate on Present-Day Patterns in Biodiversity and Ecosystems, Annu. Rev. Ecol. Evol. S., 46, 551–572, https://doi.org/10.1146/annurev-ecolsys-112414-054314, 2015.
Swenson, N. G.: The role of evolutionary processes in producing biodiversity patterns, and the interrelationships between taxonomic, functional and phylogenetic biodiversity, Am. J. Bot., 98, 472–480, https://doi.org/10.3732/ajb.1000289, 2011.
Swenson, N. G., Erickson, D. L., Mi, X., Bourg, N. A., Forero-Montaña, J., Ge, X., Howe, R., Lake, J. K., Liu, X., Ma, K., Pei, N., Thompson, J., Uriarte, M., Wolf, A., Wright, S. J., Ye, W., Zhang, J., Zimmerman, J. K., and Kress, W. J.: Phylogenetic and functional alpha and beta diversity in temperate and tropical tree communities, Ecology, 93, S112–S125, 2012.
The Angiosperm Phylogeny Group: An update of the Angiosperm Phylogeny Group classification for the orders and families of flowering plants APG III, Bot. J. Linn. Soc., 161, 105–121, https://doi.org/10.1111/boj.12385, 2009.
Tian, F., Wang, Y., Chi, Z., Liu, J., Yang, H., Jiang, N., and Tang, W.: Late Quaternary vegetation and climate reconstruction based on pollen data from southeastern Inner Mongolia, China, Rev. Palaeobot. Palyno., 242, 33–42, https://doi.org/10.1016/j.revpalbo.2017.03.003, 2017.
Tilman, D., Reich, P. B., and Isbell, F.: Biodiversity impacts ecosystem productivity as much as resources, disturbance, or herbivory, P. Natl. Acad. Sci. USA, 109, 10394–10397, https://doi.org/10.1073/pnas.1208240109 2012.
Trabucco, A. and Zomer, R. J.: Global Aridity Index and Potential Evapo-Transpiration (ET0) Climate Database v2, https://doi.org/10.6084/m9.figshare.7504448.v3, 2019.
Ulrich, W., Soliveres, S., Maestre, F. T., Gotelli, N. J., Quero, J. L., Delgado-Baquerizo, M., Bowker, M. A., Eldridge, D. J., Ochoa, V., Gozalo, B., Valencia, E., Berdugo, M., Escolar, C., Garcia-Gomez, M., Escudero, A., Prina, A., Alfonso, G., Arredondo, T., Bran, D., Cabrera, O., Cea, A., Chaieb, M., Contreras, J., Derak, M., Espinosa, C. I., Florentino, A., Gaitan, J., Muro, V. G., Ghiloufi, W., Gomez-Gonzalez, S., Gutierrez, J. R., Hernandez, R. M., Huber-Sannwald, E., Jankju, M., Mau, R. L., Hughes, F. M., Miriti, M., Monerris, J., Muchane, M., Naseri, K., Pucheta, E., Ramirez-Collantes, D. A., Raveh, E., Romao, R. L., Torres-Diaz, C., Val, J., Veiga, J. P., Wang, D., Yuan, X., and Zaady, E.: Climate and soil attributes determine plant species turnover in global drylands, J. Biogeogr., 41, 2307–2319, https://doi.org/10.1111/jbi.12377, 2014.
Van der Plas, F.: Biodiversity and ecosystem functioning in naturally assembled communities, Biol. Rev., 94, 1220–1245, https://doi.org/10.1111/brv.12499, 2019.
Walther, G.-R., Post, E., Convey, P., Menzel, A., Parmesan, C., Beebee, T. J. C., Fromentin, J.-M., Hoegh-Guldberg, O., and Bairlein, F.: Ecological responses to recent climate change, Nature, 416, 389–395, https://doi.org/10.1038/416389a, 2002.
Wang, Q., Li, Y., Zou, D., Su, X., Cai, H., Luo, A., Jiang, K., Zhang, X., Xu, X., Shrestha, N., and Wang, Z.: Phylogenetic niche conservatism and variations in species diversity–climate relationships, Ecography, 44, 1856–1868, https://doi.org/10.1111/ecog.05759, 2021.
Wolf, A. A., Funk, J. L., Selmants, P. C., Morozumi, C. N., Hernandez, D. L., Pasari, J. R., and Zavaleta, E. S.: Trait-based filtering mediates the effects of realistic biodiversity losses on ecosystem functioning, P. Natl. Acad. Sci. USA, 118, e2022757118, https://doi.org/10.1073/pnas.2022757118, 2021.
Yang, H., Wu, M., Liu, W., Zhang, Z. H. E., Zhang, N., and Wan, S.: Community structure and composition in response to climate change in a temperate steppe, Glob. Change Biol., 17, 452–465, https://doi.org/10.1111/j.1365-2486.2010.02253.x, 2011.
Ye, J. S., Delgado-Baquerizo, M., Soliveres, S., and Maestre, F. T.: Multifunctionality debt in global drylands linked to past biome and climate, Glob. Change Biol., 25, 2152–2161, https://doi.org/10.1111/gcb.14631, 2019.
Yin, Y., Liu, H., He, S., Zhao, F., Zhu, J., Wang, H., Liu, G., and Wu, X.: Patterns of local and regional grain size distribution and their application to Holocene climate reconstruction in semi-arid Inner Mongolia, China, Palaeogeogr. Palaeocl., 307, 168–176, https://doi.org/10.1016/j.palaeo.2011.05.011, 2011.
Zanne, A. E., Tank, D. C., Cornwell, W. K., Eastman, J. M., Smith, S. A., FitzJohn, R. G., McGlinn, D. J., O'Meara, B. C., Moles, A. T., Reich, P. B., Royer, D. L., Soltis, D. E., Stevens, P. F., Westoby, M., Wright, I. J., Aarssen, L., Bertin, R. I., Calaminus, A., Govaerts, R., Hemmings, F., Leishman, M. R., Oleksyn, J., Soltis, P. S., Swenson, N. G., Warman, L., and Beaulieu, J. M.: Three keys to the radiation of angiosperms into freezing environments, Nature, 506, 89–92, https://doi.org/10.1038/nature12872, 2014.