the Creative Commons Attribution 4.0 License.
the Creative Commons Attribution 4.0 License.
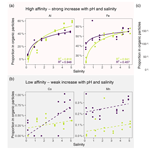
Estuarine flocculation dynamics of organic carbon and metals from boreal acid sulfate soils
Joonas J. Virtasalo
Peter Österholm
Eero Asmala
Flocculation of riverine dissolved organic matter to the particulate form in estuaries is an important mechanism for capturing dissolved metals to newly formed organic particles, regulating the metal transport from land to sea. The process is particularly relevant for rivers draining boreal acid sulfate soils of western Finland, which are known to deliver large amounts of trace metals with detrimental environmental consequences for the recipient estuaries in the eastern Gulf of Bothnia in the northern Baltic Sea. This is the first study to investigate dissolved metal (Al, Fe, Mn, Co, and Cu) association with flocculating organic particles in the laboratory, by mixing of acidic metal-rich water from acid-sulfate-soil-impacted rivers and particle-free artificial seawater. Water samples were collected in April 2021 from the Laihianjoki and Sulvanjoki rivers in western Finland. Experiments with an in situ laser-diffraction-based particle size distribution sensor and a multiparameter water quality sonde were run to continuously monitor the development of a suspended particle pool over the salinity gradient from 0 to 6, corresponding to the salinity range observed in these estuarine systems. Flocculator experiments with discrete salinity treatments were carried out to investigate metal behaviour with the collection of flocculated material on glass fibre filters. Filtrate was analysed for coloured dissolved-organic-matter absorbance and fluorescence for the characterization of potential changes in the organic matter pool during the flocculation process. Retentate on the filter was subjected to persulfate digestion of organic particles and metal oxyhydroxides (pH < 2.3), and the digestion supernatants were analysed for metal concentrations. The laboratory experiments showed strong transfer of Al and Fe already at a salinity of 0–2 to newly formed organically dominated flocs that were generally larger than 80 µm. Very strong coupling between the decrease in humic fluorescence and the increase in organically bound Al demonstrated that Al transfer to the flocs was stronger than that of Fe. The flocs in the suspended particle pool were complemented by a smaller population of Al- and Fe-oxyhydroxide-dominated flocculi (median size of 11 µm) after pH exceeded ca. 5.5. Cobalt and Mn transfer to the particle pool was weak, although some transfer to Mn oxyhydroxides as well as Co association with the flocs took place. Up to 50 % of Cu was found to be bound to humic substances in the flocs in the river waters, and this proportion did not significantly change during mixing with seawater. The findings of this study demonstrate that salinity and pH are important independent but connected controls of the flocculation behaviour of dissolved metals from boreal acid sulfate soils and the seaward transport and environmental consequences of the metals in the marine environment.
Among the most important processes affecting the global biogeochemical cycles of carbon, nutrients, and trace metals is the physicochemical transformation of terrestrial dissolved and colloidal material into suspended particles at the freshwater and seawater mixing zone in estuaries (Boyle et al., 1974; Bianchi, 2007; Canuel and Hardison, 2016). The influence of seawater cations induces the flocculation of riverine dissolved organic matter (DOM) to the particulate form, which provides a mechanism for the capture of dissolved metals that have a tendency to be adsorbed to particle surfaces (Sholkovitz, 1976, 1978; Asmala et al., 2022). In addition to salinity, pH also typically increases towards seawater and provides another key variable that controls metal sorption to particle surfaces (Mosley and Liss, 2020). The role of pH is particularly relevant in the context of acid sulfate (AS) soils that can generate extremely low-pH conditions and high trace metal concentrations in surface waters (Dent and Pons, 1995). Flocculation of dissolved riverine material into sinking particles is a key component of the coastal filter, which effectively removes terrestrial material from the freshwater inputs in the coastal salinity gradient (Asmala et al., 2017). The differing physical chemistries of trace metals lead to a variety of geochemical behaviours in the estuarine flocculation, with consequences for their seaward transport (Shiller and Boyle, 1991; Beck et al., 2012; Fu et al., 2013).
AS soils occupy ca. 50 million ha−1 globally, including areas in Australia, South and Southeast Asia, Africa, Central and South America, and northern and western Europe (Andriesse and van Mensvoort, 2006). The largest occurrences of AS soils in Europe are found in Finland, where they occupy an area on the order of 1 million ha−1 (Jaakko Auri, personal communication, 2022). The Finnish AS soils are predominantly sulfide-bearing organically rich muds, which were originally deposited in the Baltic Sea during its brackish-water phase, which began ca. 7000 years ago in the Gulf of Bothnia (Virtasalo et al., 2007; Häusler et al., 2017), but these coastal areas have since emerged above sea level due to rapid glacio-isostatic land uplift (Kakkuri, 2012). Artificial drainage and reclamation of these lands for farming purposes, particularly in the 1960s and 1970s, have caused a significant lowering of the groundwater level, which has enabled rapid oxidation of the sulfide minerals, producing H2SO4 and resulting in AS soils with a pH < 4 (Yli-Halla et al., 1999; Sohlenius and Öborn, 2004; Boman et al., 2010; Saarinen et al., 2010; Yu et al., 2015; Nyman et al., 2023). Under these extremely acidic conditions, large quantities of metals are released to the porewater due to the oxidative dissolution of metal sulfides and the weathering of silicate minerals. Particularly during high-water-flow conditions in spring and autumn, acidic porewater rich in metals (typically Al, Cd, Co, Cu, Mn, Ni, and Zn) is flushed to recipient streams (Österholm and Åström, 2008), with detrimental ecological consequences for aquatic life (Hudd and Kjellman, 2002; Fältmarsch et al., 2008; Wallin et al., 2015; Sutela and Vehanen, 2017).
Previous field studies and geochemical modelling results on the west coast of Finland have concluded that when acidic metal-rich river waters from the boreal AS soil landscape are discharged to estuaries with higher pH and salinity, the metals are complexed with organic matter or co-precipitated with Al, Fe, and Mn oxyhydroxides and consequently deposited in sediments (Nordmyr et al., 2008a, b; Nystrand et al., 2016). Specifically, it was concluded that Al, Fe, and Cu are deposited with organic matter close to the Vöyrinjoki river mouth, whereas Cd, Co, Ni, and Zn are preferentially transported farther out at sea, where they co-precipitate and are deposited with Mn oxyhydroxides (Nordmyr et al., 2008a, b; Nystrand et al., 2016). However, a recent study of seafloor sediments shows that Cu, Cd, Co, Ni, and Zn are all enriched at least 26 km away from the mouths of the nearby Laihianjoki and Sulvanjoki rivers, whereas the Mn enrichment extends less than 14 km from the river mouths (Virtasalo et al., 2020). This discrepancy between the observed seafloor metal distribution and the previously concluded seaward transport behaviour of the metals calls for further investigation of the flocculation process in boreal humus-rich rivers that are strongly impacted by acidity and metal release from AS soils.
This study investigated organic matter flocculation and aggregation and trace metal association with the newly formed organic particles. To address this phenomenon, we carried out laboratory experiments, mixing natural acidic river water with particle-free artificial seawater. River water was collected from the Laihianjoki and Sulvanjoki rivers, which are among the most AS-soil-impacted rivers in Finland and Europe (Roos and Åström, 2005). Large-volume bucket experiments with high-frequency in situ instruments were continuously run to study the development of suspended particle size distribution and organic matter characteristics and to identify possible threshold salinities for further experimentation. Small-volume flocculator experiments with discrete salinity treatments were carried out to investigate metal behaviour in the flocculation with subsequent laboratory analyses of the flocculated material. The focus was on Al, Fe, and Cu, which, according to previous studies in the area, to a large extent form complexes with flocculating organic matter and precipitate as Al and Fe oxyhydroxide minerals in the early phases of freshwater–seawater mixing, as well as on Co and Mn, which are relatively persistent in solution (Nordmyr et al., 2008a, b; Nystrand et al., 2016). The studied elements Al, Mn, Co, and Cu are known to be extensively leached from AS soils and much less so from the local industry and other human activities in the area (e.g. Åström and Björklund, 1997; Åström and Corin, 2000; Österholm and Åström, 2002; Sundström et al., 2002). Similarly, background concentrations of Fe are typically very high in coastal areas of Finland (e.g. Lahermo et al., 1996).
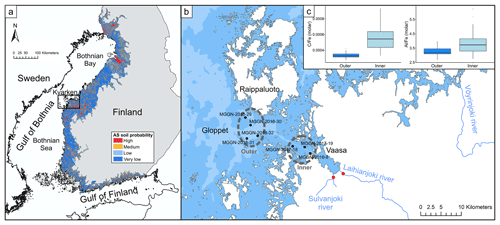
Figure 1Maps of the Baltic Sea and the study area, with a boxplot of molar Al, Fe, and C ratios in the inner and outer archipelago. (a) Map of the Baltic Sea and the probability of acid sulfate soil occurrence in Finland. The black square indicates the location of the study area on the west coast of Finland. (b) Nautical chart of the study area in the Kvarken Archipelago. Red dots indicate the water sampling locations of this study. Black dots indicate the coring sites of sediment samples from Virtasalo et al. (2020) that were used for the molar element ratio calculations in the inner and outer archipelago. (c) Molar and ratios in the inner and outer archipelago. Sources: acid sulfate soil probability map – Geological Survey of Finland, 2018; nautical chart – Finnish Transport Agency, 2017.
The objectives of this investigation were to (1) assess the quantity of dissolved metals removed from acidic and metal-rich river water by association with flocculating organic particles and (2) quantify changes in the size distribution of suspended particles due to increasing salinity and pH. We hypothesize that (1) with increasing salinity and pH, the proportion of dissolved metals that are associated with organic particles increases, (2) the size of suspended particles increases throughout the experimental salinity gradient as dissolved substances and smaller particles aggregate into larger particles, and (3) differences in metal concentrations and water quality between the studied rivers affect the flocculation behaviour of DOM and metals.
The Laihianjoki and Sulvanjoki rivers are located in central–western Finland, where they drain into the shallow Sundominlahti Bay near the town of Vaasa in the Kvarken Archipelago of the Gulf of Bothnia (Fig. 1). Laihianjoki is 60 km long and has a catchment area of 506 km2 with an overall flat topography that is dominated by forests and peatlands (61 %) and agricultural land (27 %; Table 1; Korhonen and Haavalammi, 2012). Sulvanjoki is only 33 km long and drains a catchment area of 144 km2, with a clearly larger share (36 %) occupied by agricultural land. In both catchments, most of the agricultural land consist of well-drained, fine-grained AS soils that leach large quantities of acidity and metals. Due to the significantly higher proportion of AS soils in the Sulvanjoki catchment area, its acidity and metal concentrations are expected to be higher than in Laihianjoki.
Table 1Properties of river catchments and mean annual values of discharge (Q), total Al concentration, total Fe concentration, and molar Al:Fe ratio (Korhonen and Haavalammi, 2012). “Agriculture” includes pasture and cropland; “open” consists of areas with no vegetation; and “water” includes lakes, streams, etc. Details about sampling location and water chemistry during sampling are also given.
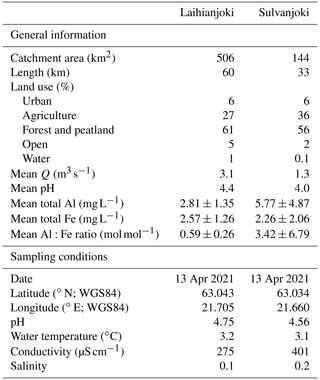
Crystalline bedrock in the study area is dominated by Paleoproterozoic granitoids and gneisses that are overlain by an on average 5 m thick till layer and occasional till ridges (De Geer moraines, drumlins) and glaciofluvial landforms (Breilin et al., 2005). Following the north-westward retreat of the Fennoscandian continental ice sheet ca. 10 400 years ago, the study area was submerged by a ca. 250 m deep lake (Sauramo, 1929; Saarnisto and Saarinen, 2001; Stroeven et al., 2016). A glacial varved to weakly layered mud blanket was deposited on the till and glaciofluvial deposits during the successive glaciolacustrine and post-glacial lake phases. The lake sediments are sharply overlain by an organically rich mud layer that was deposited after the onset of the modern brackish-water sea phase ca. 7000 years ago (Virtasalo et al., 2007; Häusler et al., 2017). The area has since emerged above sea level as a result of the glacio-isostatic rebound, which continues today at a notable rate of 8 mm yr−1 (Kakkuri, 2012). During the emergence, mud was removed by coastal erosion from the local topographic highs and redeposited in local depressions. Today, agricultural fields in the area are largely developed in the lowland areas with brackish-water, sulfide-bearing, organically rich muds that are active or potential AS soils (Yli-Halla et al., 1999; Boman et al., 2010).
The study area belongs to the continental subarctic climate zone with severe dry winters and warm summers. The mean annual air temperature is 4.2 ∘C, with a mean minimum temperature of 2.1 ∘C and a mean maximum temperature of 6.6 ∘C during the period 1981–2010 (Pirinen et al., 2012). The mean annual precipitation is 497 mm. The mean annual sea surface salinity in the Kvarken Archipelago ranges between 3.5 and 4, and the mean annual sea surface temperature is between 3.5 and 7 ∘C. The low salinity results from the high riverine runoff from the large catchment area of the Gulf of Bothnia and from the long distance to the narrow connection to the North Sea through the Danish straits (Kuosa et al., 2017). The sea is essentially non-tidal, but irregular water level fluctuations of as much as ± 1.5 m take place due to variations in wind and atmospheric pressure (Wolski et al., 2014). Stratification of the shallow archipelago waters is governed by a thermocline that develops each summer. Seasonal ice cover in the inland and coastal waters is typical during winter months.
3.1 Field sampling
Water samples were collected from the Laihianjoki and Sulvanjoki rivers in April 2021 using a bucket and poured into 30 L containers (Table 1). Springtime, several weeks after the flow peak induced by snowmelt, was chosen for the sampling because then the variation in water quality in AS-soil-impacted rivers is the smallest, and the most representative samples can be obtained (Österholm and Åström, 2008). The river waters were immediately measured for pH, temperature, electrical conductivity, and salinity by a WTW MultiLine P4 meter (WTW GmbH, Weilheim, Germany). The water samples were transported under cool conditions to the Tvärminne field station of the University of Helsinki, where the laboratory experiments commenced within 24 h.
3.2 Experimental set-ups
A large-volume “bucket” experiment was carried out to determine the dynamics of suspended particle size distribution and associated nutrients during the gradual transition from fresh to brackish water conditions following the experimental protocol described by Asmala et al. (2022). In short, a salt solution was prepared by diluting artificial sea salt (Aquarium Systems Instant Ocean) to ultrapure water and filtered through a pre-combusted glass fibre filter (nominal pore size of 0.7 µm) to remove any possible remaining particles. The final salinity of the solution was 66.3 g kg−1. The experiment was conducted on Laihianjoki and Sulvanjoki river waters, screened with a 200 µm nylon mesh for removing debris. Initially, 20 L of river water was poured into an acid-washed polypropylene basin (bucket) with a mounted spinning device rotating at a fixed speed of ca. 100 rpm to ensure proper mixing throughout the experiment. Artificial seawater was added to the bucket at the rate of 6.7 mL min−1 for the 4 h duration of the experiment. Changes in water chemistry and suspended particle size distribution were recorded continuously with an EXO2 multiparameter water quality sonde (YSI, Yellow Springs, OH, USA) and a LISST-100X type B laser-diffraction-based particle size distribution analyser (Sequoia Scientific, Bellevue, WA, USA). The EXO2 multiparameter sonde was equipped with six electronic and optical sensors, measuring conductivity (mS cm−1), temperature (∘C), dissolved oxygen (mg L−1), pH, fluorescent dissolved organic matter (FDOM; in quinine sulfate units, QSU) and turbidity (formazin nephelometric units, FNU). The LISST particle size sensor measures suspended particle (aggregate) size distribution and concentration in situ over 32 size classes (bins) in the range of 1.25 to 250 µm (Agrawal and Pottsmith, 2000). Both instruments were calibrated using manufacturers' guidelines, and the measuring interval for both instruments was 1 Hz. The artificial seawater was gradually added using a peristaltic pump and acid-washed tubing to the experimental basin containing the river water in order to simulate an increasing estuarine salinity gradient from 0.0 to 6.0 g kg−1. All measured concentration values were corrected for the dilution by the particle-free artificial seawater in subsequent analyses.
After the bucket experiment, LISST data were processed using the random particle shape model in the instrument data processing software. Weighted mean particle size was calculated using the median size of each LISST size class. The values for salinity, temperature, pH, FDOM, and turbidity were determined from the factory-calibrated sensor readings by the integral algorithms of the EXO2 multiparameter sonde software.
Table 2Metal concentrations in the Laihianjoki and Sulvanjoki river water samples and artificial seawater.

A set of small-volume jar experiments were carried out to collect particle samples from standardized and replicable conditions using a VELP Scientifica FC6S six-unit flocculator apparatus (VELP Scientifica, Usmate, Italy). The same artificial seawater was used as in the bucket experiment with in situ instruments. Sample water from study rivers was mixed with ultrapure water and artificial seawater to create a salinity gradient from 0 to 6 g kg−1 to a final volume of 1000 mL. The flocculator test duration was 1 h at a fixed paddle speed of 60 rpm. At the end of the test cycle, suspended material was collected on triplicate pre-combusted glass fibre filters with a nominal pore size of 0.7 µm. Filtrate was collected for the analysis of coloured dissolved organic matter (CDOM).
3.3 Laboratory analyses
Triplicate samples of the Laihianjoki and Sulvanjoki river waters and the artificial seawater were acidified by HNO3 and analysed for Al and Fe concentrations by a Thermo Scientific iCAP 7600 Duo inductively coupled plasma optical emission spectrometer (ICP-OES) and for Co, Cu, and Mn concentrations by a Thermo Scientific iCAP Q inductively coupled plasma mass spectrometer (ICP-MS; Thermo Fisher Scientific Inc., Waltham, MA, USA). The ICP-OES and ICP-MS were used for the selected metals for improved analytical precision. The detection limits for Al, Co, Cu, Fe, and Mn were 200, 0.05, 0.1, 30, and 0.02 µg L−1, respectively.
Filtrate samples from the flocculator experiment were measured for CDOM absorption using a Shimadzu 2401PC spectrophotometer (Shimadzu, Kyoto, Japan) with a 5 cm quartz cuvette over the spectral range of 200 to 800 nm with 1 nm resolution. Ultrapure water was used as the blank for all samples. Excitation–emission matrices (EEMs) of FDOM were measured with a Varian Cary Eclipse fluorometer (Agilent Technologies, Santa Clara, CA, USA). Processing of the EEMs was done using the eemR package for R software (Massicotte, 2016). A blank sample of ultrapure water was subtracted from the EEMs, and the Rayleigh and Raman scattering bands were removed from the spectra after calibration. EEMs were calibrated by normalizing to the area under the Raman water scatter peak (excitation wavelength of 350 nm) of an ultrapure water sample run in the same session as the samples and were corrected for inner filter effects with absorbance spectra (Murphy et al., 2010). For assessing the characteristics and the quality of the DOM pool, fluorescence peaks (Coble, 1996) were calculated from the EEMs (peak C: humic-substance-like; peak T: protein-like).
Filter retentate samples, collected in triplicate in the flocculator experiments, were analysed for particulate organic carbon (POC) and particulate organically bound metals. POC analysis was performed using a Europa Scientific ANCA-MS 20-20 15N/13C mass spectrometer (Sercon, Crewe, UK) from filter samples. Metal concentrations were determined after total digestion of organic particles on filter samples by persulfate oxidation according to Pujo-Pay and Raimbault (1994). Persulfate digestion results in a pH < 2.3, which is thought to effectively dissolve metal oxyhydroxides (Schwertmann, 1991). Al, Co, Cu, Fe, and Mn concentrations in the digestion supernatants were analysed by ICP-MS, controlled with blank reagent samples. Only ICP-MS was used because of the persulfate matrix. The detection limits for Al, Co, Cu, Fe, and Mn were 20, 0.2, 10, 100, and 1 µg L−1, respectively. Values below detection were rounded to half the detection limits. The final element concentrations were then calculated by subtracting the concentrations detected on the equipment blank samples.
TSM (total suspended matter) was determined in triplicate by filtering a known volume of sample water through a pre-weighed GF/F filter and subsequently drying the filters overnight at 60 ∘C and then weighing again. The apparent particle density (Thomas et al., 2017) was calculated by dividing TSM (mass concentration; mg L−1) by the particle total volume concentrations measured by LISST (volumetric concentration; µL L−1) from matching salinities in bucket and flocculator experiments. It should be noted, however, that the apparent particle density represents only particles measurable by the LISST instrument, i.e. particles within a hydrodynamic radius between 1 and 250 µm. As the proportion of particles in the sample that are larger than our upper detection limit is non-trivial, it can be expected that our density results are biased as the largest particles are not included in the analysis.
4.1 River water initial composition
Compared to Laihianjoki (Table 2), samples from Sulvanjoki had higher total concentrations of Al, Co, and Mn; similar concentrations of Cu; and lower concentrations of Fe.
4.2 Large-volume bucket experiment
4.2.1 Transformation of dissolved substances into particulate form
Initial characteristics of the particulate and dissolved-organic-matter pools were different between the study rivers (Fig. 2). In Laihianjoki, the mean particle size was 84 µm, and the total volume concentration of 1–250 µm particles was 201 µL L−1, whereas in Sulvanjoki the values were higher, 130 µm and 266 µL L−1, respectively. On the other hand, initial turbidity was higher in Laihianjoki (25 FNU) than in Sulvanjoki (10 FNU). The initial DOM concentration, as indicated by humic fluorescence, was 90 QSU in Laihianjoki and 126 QSU in Sulvanjoki.
Turbidity and total particle volume concentration increased throughout the experimental salinity gradient in both rivers (Fig. 2b and d). In Laihianjoki, turbidity increased to 37 FNU and particle volume concentration to 880 µL L−1 at a salinity of 5.0, and in Sulvanjoki turbidity increased to 34 FNU and particle volume concentration to 1300 µL L−1, respectively. It should be noted that the rate of increase was considerably lower above a salinity ∼ 2 in Laihianjoki. Conversely, humic fluorescence decreased in both rivers, to 55 QSU in Laihianjoki and to 63 QSU in Sulvanjoki (Fig. 2a). The mean particle size in Laihianjoki showed a dynamic response to seawater addition, displaying a peak value of 115 µm at a salinity of 1 followed by a steady decline to a value of 100 µm at a salinity of 5 (Fig. 2e). In contrast, the mean particle size was sustained at a high level in Sulvanjoki across the experimental salinity gradient, showing only a small decrease from 130 to 120 µm in the salinity range of 3–5. During the bucket experiment, the seawater addition caused an increase in pH, from the initial 5.2 to 6.9 in Laihianjoki and from 5.2 to 6.3 in Sulvanjoki (Fig. 2c). In Laihianjoki, pH 5.5 was reached at a salinity of 0.5, while it was reached later in Sulvanjoki, at a salinity of 1.4.
4.2.2 Changes in suspended particle size distribution
Development of particle volume concentrations in the 32 size classes during the bucket experiment was measured by the LISST particle size distribution analyser (Fig. A1 in the Appendix). Two main types of behaviour were identified by visual inspection among the size classes: the finer classes with median particle sizes of 9.35 and 11.0 µm (bins 14 and 15) showed low initial values until the concentrations started to increase strongly at a salinity of 1 (pH 5.75) in Laihianjoki and at a salinity of 2 (pH 5.59) in Sulvanjoki, whereas in the coarsest size classes with median particle sizes of 156.0 and 184.1 µm (bins 31 and 32), the concentrations began to increase strongly from the start of the experiment at a salinity of 0 (pH 5.30 in Laihianjoki and 5.33 in Sulvanjoki). The strong initial increase in the coarsest particle concentrations levelled off and began to decrease at a salinity of 2 (pH 6.16) in the Laihianjoki river. The finest size classes (1.0–8.6 µm, bins 1–13) each displayed very low volume concentrations of less than 1 % of the coarsest class; these finest classes were considered insignificant for the studied flocculation process and were not considered further.
The size classes 11.0 and 184.1 µm (bins 15 and 32 with ranges of 10.2–12.0 and 169–200 µm, respectively) were selected for further investigation of the development of suspended particle size distribution during the bucket experiment (Fig. 3). The size class concentration ratio of µm shows a dominant initial increase in the coarsest particles in the flocculation in the salinity range of 0 to 1. Above a salinity of 1, however, the proportion of the coarsest particles decreases, and the proportion of the finer particles increases continuously until the end of the experiment. The observed changes in the proportions are 3 orders of magnitude larger in Sulvanjoki, where the proportion of the coarsest particles decreases strongly between salinities of 1 and 3, but the change slows down to a similar rate to that in Laihianjoki above a salinity of 3 (on the logarithmic scale).
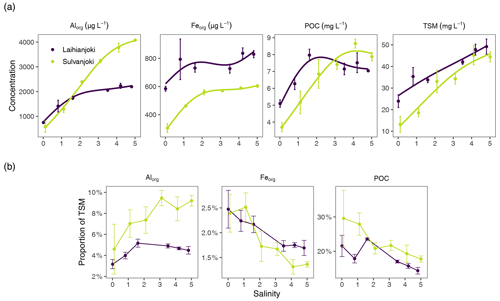
Figure 4(a) Concentration of Alorg and Feorg (µg L−1) as well as POC (mg L−1) in the organic particle pool and TSM in the flocculator experiments. (b) Proportions of Alorg, Feorg, and POC of TSM in the flocculator experiments. Points denote mean values of triplicate filter retentate samples, and error bars denote 1 standard deviation.
4.3 Small-volume flocculator experiments
4.3.1 Dynamics of major flocculating elements
In Laihianjoki, flocculator experiments, with discrete salinity treatments, showed that salinity increase induces particle formation at an almost constant rate throughout the experimental salinity range, as indicated by TSM concentration (Fig. 4a). Concentration of organically bound particulate aluminium (Alorg) in the organic particulate pool, as determined by the persulfate digestion of triplicate filter retentate samples, more than doubled from the initial 750 to 1740 µg L−1 between salinities of 0 and 1.6, above which only a moderate increase was observed. Organically bound particulate iron (Feorg) concentration increased from 585 to 793 µg L−1 until a salinity of 0.8, above which there was no significant increase. POC concentration increased strongly, from 5.1 mg L−1 at a salinity of 0 to the maximum of 8.0 mg L−1 at a salinity of 1.6 and decreased slowly afterwards.
In Sulvanjoki, TSM concentration increased at an almost linear rate throughout the salinity range (Fig. 4a). Alorg increased at a similar linear rate, from 578 µg L−1 at a salinity of 0 to 4080 µg L−1 at a salinity of 5. Feorg increased from 305 to 560 µg L−1 between salinities of 0 and 2.1, above which only a moderate increase was observed. POC increased strongly, from 3.7 mg L−1 at a salinity of 0 to the maximum of 8.7 mg L−1 at a salinity of 4.1, above which a small decrease took place.
The inspection of the proportions of Alorg, Feorg, and POC of TSM provided further insight into the composition of the suspended particle pool. In Laihianjoki, the proportion of Alorg increased from 3.2 % to 5.2 % of TSM between salinities of 0 and 1.6, above which the Alorg proportion slowly decreased (Fig. 4b). In Sulvanjoki, the Alorg proportion showed an even stronger increase, doubling from 4.6 % to 9.2 % of TSM over the experimental salinity range. In contrast, the Feorg proportion of TSM decreased in both rivers from the initial 2.5 % to 1.7 % and 1.3 % at high salinities in Laihianjoki and Sulvanjoki, respectively. Similarly, the POC proportion of TSM in Laihianjoki decreased from 22 % at a salinity of 0 to 14 % at a salinity of 4.8. In Sulvanjoki, the POC proportion decreased from 30 % at a salinity of 0 to 18 % at a salinity of 5. Clearly, the flocculation rate of Al was higher than that of Fe and organic C and resulted in an increasing proportion of Alorg in the particle pool throughout the experiment.
Mean apparent particle density at a salinity of 0 was 0.12 mg L−1 in Laihianjoki and 0.05 mg L−1 in Sulvanjoki (Fig. 5). At a salinity of 1, Laihianjoki showed a decrease in particle density, but still higher values compared to Sulvanjoki (0.09 and 0.04 mg L−1, respectively). At salinities above 2, particle density remained relatively stable in both rivers, ranging between 0.03 and 0.06 mg L−1.
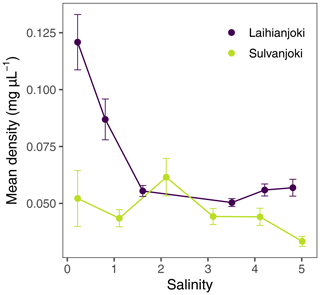
Figure 5Mean apparent density of suspended particles in the flocculator experiments along the artificial salinity gradient. Points denote mean values of triplicate filter retentate samples, and error bars denote 1 standard deviation.
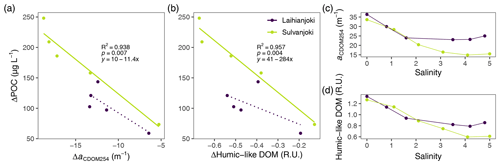
Figure 6Relationship between changes in POC concentration and (a) CDOM absorption coefficient at 254 nm (aCDOM254) and (b) humic fluorescence (peak C; ex350/em420–480 nm; Coble, 1996) in the flocculator experiments. A significant (p < 0.05) linear relationship is indicated with a solid line, and a non-significant linear relationship is indicated with a dotted line. Changes in (c) aCDOM254 and (d) humic fluorescence along the salinity gradient of the flocculator experiments.
4.3.2 Changes in DOM optical properties
UV absorbance (a(CDOM254)) and humic DOM fluorescence (peak C) decreased strongly in response to increasing salinity in both rivers (Fig. 6a and b). These decreases in aromatic, humus-like DOM compounds were linked with increases in POC, indicating transformation of DOM into particulate form (Figs. 6c, d, A2, and A3).
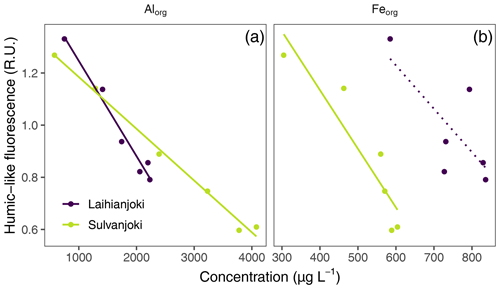
Figure 7Relationship between the concentrations of Alorg and Feorg (mg L−1) and humic fluorescence (peak C; ex350/em420–480 nm; Coble, 1996) in the flocculator experiments. A significant (p < 0.05) linear relationship is indicated with a solid line, and a non-significant linear relationship is indicated with a dotted line.
Humic DOM fluorescence also showed a significant inverse relationship with Alorg and Feorg concentrations in the suspended particle pool (Fig. 7). In the experiment units without seawater addition, the humic fluorescence was the highest and Alorg and Feorg concentrations the lowest. With increasing salinity, humic DOM fluorescence decreased, and organically bound particulate metal concentrations increased.
4.3.3 Dynamics of trace metals
Some of the flocculator experiment treatments showed large variability in trace metal concentrations, as determined based on the triplicate filter retentate samples (Fig. 8). Therefore, the focus here is on the overall trends and on the metal concentrations in the lowest- and in the highest-salinity treatments.
Concentrations of organically bound particulate cobalt (Coorg) and manganese (Mnorg) increased in response to salinity increase in both Laihianjoki and Sulvanjoki (Fig. 8a and c). Concentrations of Coorg increased from 0.013 µg L−1 at a salinity of 0 to 0.025 µg L−1 at a salinity of 4.8 in Laihianjoki and from 0.00 µg L−1 at a salinity of 0 to 0.023 µg L−1 at a salinity of 5 in Sulvanjoki. For Mnorg, there was only a minor concentration increase from 0.56 to 0.64 µg L−1 in Laihianjoki, but, in Sulvanjoki the concentration doubled from 0.19 to 0.37 µg L−1.
In contrast, the response of Cuorg concentration to the salinity increase was ambiguous (Fig. 8b). The concentration of Cuorg showed a modest increase from 1.66 µg L−1 at a salinity of 0 to 2.20 µg L−1 at a salinity of 4.8 in Laihianjoki, while it decreased slightly from 2.88 µg L−1 at a salinity of 0 to 2.45 µg L−1 at a salinity of 5 in Sulvanjoki.
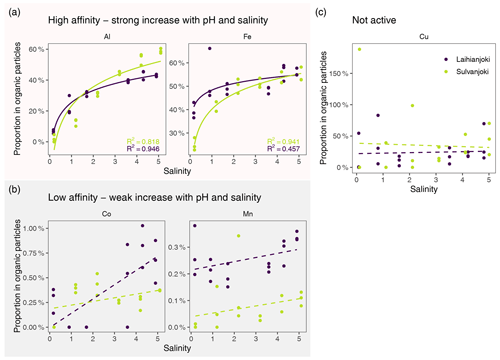
Figure 9Flocculation affinity of Al, Fe, Cu, Co, and Mn, as indicated by change in the proportion of particulate fraction of total concentration along the experimental seawater gradient. Solid lines indicate significant (log-)linear trends (p < 0.05), and dotted lines indicate non-significant (log-)linear trends. Points denote mean values of triplicate filter retentate samples, and error bars denote 1 standard deviation.
4.3.4 Flocculation affinity of metals
To estimate the susceptibility of metals to flocculation, the proportion of each metal in the organic particulate fraction was determined compared to its total concentration in the river water (Fig. 9). Using this approach, an increasing proportion value indicated an increasing proportion of the dissolved fraction transferring to the organic particle pool. The studied metals could be divided into three groups in terms of how they responded to the increasing salinity and pH: (1) Al and Fe, with increasing transfer to the organic particle pool, i.e. high affinity to flocculation; (2) Cu, with high initial affinity to organic particles but no significant response to increasing salinity and pH; and (3) Co and Mn, with low affinity to organic particles throughout the salinity and pH gradient.
The flocculation affinity was strongest for Al, whose proportion in the organic particle pool increased from the initial 0 %–5 % to 40 %–60 % at a salinity of 5 (Fig. 9a). Iron was to a significant degree (25 %–40 %) associated with organic particles already at the start of the experiment, and its particulate proportion increased to 55 % at a salinity of 5. Both Co and Mn showed low flocculation affinity, as their particulate proportion increased from 0 %–0.26 % to 0.27 %–0.7 % and from 0 %–0.27 % to 0.10 %–0.34 %, respectively, throughout the salinity and pH gradient and in addition showed high variability among triplicate filter retentate samples (Fig. 9b). Copper had a relatively stable particulate proportion of 10 %–50 %, although the variability among retentate samples was high (Fig. 9c).
This study investigated the flocculation of organic matter and metals from dissolved to particulate form in response to mixing particle-free artificial seawater with water from Laihianjoki and Sulvanjoki rivers, which are strongly impacted by acidity and metal release from AS soils. A large-volume bucket experiment with continuously measuring, high-frequency in situ instruments was run to monitor the development of particle size distribution and organic matter characteristics in the suspended particle pool and to identify representative salinities for further experimentation. Small-volume flocculator experiments with discrete salinity treatments in a flocculator apparatus were used to investigate metal behaviour in flocculation.
At the time of water sample collection, metal concentrations were extremely high, typical for streams with a high proportion of AS soils, and the metals were mainly in a dissolved form due to the very low pH (Tables 1 and 2). Compared to Laihianjoki, Sulvanjoki was more acidic and had higher concentrations of Al, Co, and Mn; similar concentrations of Cu; and lower concentrations of Fe. On the other hand, higher initial turbidity and lower humic fluorescence (FDOM) in the bucket experiment indicated higher lithic particle concentration for Laihianjoki (Fig. 2a and b). The differences in metal concentrations and water quality between the rivers were explained by the stronger impact of AS soils on Sulvanjoki due to more intense agriculture in its catchment and to the longer flow distance of Laihianjoki (Table 1).
5.1 Suspended particle size populations
Development of the suspended particle size distribution in response to seawater addition during the bucket experiments was characterized by two populations with distinct types of behaviour: the finer particles, represented by the size class of 11.0 µm median diameter, and the coarsest measured size class of 184.1 µm median diameter (Fig. 3). These size classes agree with previous laser-diffraction-based field studies in the Baltic Sea and elsewhere, which distinguish between particles smaller than 20 µm that are termed flocculi and larger particles, known as flocs (Mikkelsen et al., 2006; Lee et al., 2012; Asmala et al., 2022). Indeed, Asmala et al. (2022) recently identified similar particle populations of 8 and 184 µm median diameter in the seawater mixing experiments of the Karjaanjoki river (not significantly affected by AS soils) on the Finnish south coast. The 184 µm size class is the coarsest measurable class by the LISST instrument used, which likely underestimates the floc size in suspension that has been observed to range from tens to thousands of micrometres in field studies (Mikkelsen et al., 2006). For consistency with the previous studies, the terms flocculi and flocs are adopted here to represent the fine and coarsest measured particle populations, respectively.
The bucket experiments showed strong increases in both the volume concentration and proportion of flocs in the suspended particle pool already at a salinity of 0–2 in both Laihianjoki and Sulvanjoki (Fig. 3). These increases were accompanied by strong increases in turbidity and strong decreases in humic fluorescence (FDOM) in both rivers (Fig. 2a and b), which strongly indicates intense transformation of DOM into particulate form (Carstea et al., 2020). Mean particle size in both rivers was well above 80 µm throughout the experiment (Fig. 2e), which shows that a large majority of the newly formed particles were in the floc size range. It is thus concluded that flocs were predominantly formed by the flocculation of DOM. The floc concentration continued to increase throughout the experimental salinity gradient in Sulvanjoki, whereas the increase was levelled off and began to decrease at a salinity of 2 in Laihianjoki (Fig. 3c). This difference was likely due to the higher concentrations of floc precursors in Sulvanjoki, as shown, for example, by the much higher initial FDOM (Fig. 2a).
In contrast to the flocs, the concentration of flocculi did not increase until salinity exceeded 1 in Laihianjoki and 2 in Sulvanjoki (Fig. 3b). Instead of a straightforward dependence on salinity, however, the formation of flocculi seems to also be controlled by pH, as it begun when pH exceeded ca. 5.5 in each river (Fig. 3a). Previous field studies of AS-soil-impacted rivers in Finland have shown that Al and Fe are mainly in the dissolved form in pH levels below ca. 5.5 but are efficiently precipitated as amorphous Al and Fe oxyhydroxides and complexed with organic particles at higher pH when mixing with seawater (Åström and Corin, 2000; Åström et al., 2012; Nystrand et al., 2012). Indeed, suspended ferrihydrite particles were documented from the mouth of Laihianjoki in a recent X-ray absorption spectroscopy study of Fe phases (Yu et al., 2015). The metal hydroxide composition is in line with previous studies, which conclude that flocculi are typically single mineral grains or small aggregates of finer mineral grains (Mikkelsen et al., 2006; Lee et al., 2012). It is thus concluded that flocculi to a significant degree are composed of amorphous Al and Fe oxyhydroxides that start to precipitate at pH above ca. 5.5.
The size population ratio of µm highlights the strong initial flocculation of dissolved organic carbon in the salinity range of 0 to 1 in Laihianjoki and particularly in Sulvanjoki (Fig. 3a). Above a salinity of 1, the proportion of organically dominated flocs decreased, and the proportion of oxyhydroxide-dominated flocculi increased continuously until the end of the experiment. Flocculi and small flocs were incorporated into larger flocs with the continuing aggregation (Gregory and O'Melia, 1989; Asmala et al., 2022); however, the increasing proportion of flocculi shows that their precipitation rate exceeded the rate at which they were being incorporated into the flocs (Fig. 3a). Indeed, the declining trends in the particle size in both rivers were partially caused by the systematic underestimation of the floc concentration as they aggregated to form particles larger than the LISST measurement range as well as by the continuous precipitation of flocculi.
5.2 Association of Al and Fe with POC
Of the studied elements, the concentrations of Al, Fe, and POC were highest in the organic particle pool and made up 3.2 %–9.2 %, 1.3 %–2.5 %, and 14 %–30 % of TSM, respectively, in the flocculator experiments (Fig. 4b). These elements are therefore considered to be the main flocculating elements.
The initial concentration of dissolved Al was particularly high in Sulvanjoki, 6.50 mg L−1 (Table 2), as was the transformation of Al into the particle form in the experiments. In Sulvanjoki, Al that was bound to organic particles (Alorg), as determined by the persulfate digestion, increased almost linearly by a factor of 7, from 578 µg L−1 at a salinity of 0 to 4080 µg L−1 at a salinity of 5 (Fig. 4a). In contrast, in Laihianjoki, dissolved-Al concentration was slightly lower, 3.94 mg L−1, and Alorg increased only by a factor of 2.3 (from 750 to 1740 µg L−1) throughout the experiment. The flocculation of Fe was also stronger in Sulvanjoki, where Feorg increased by a factor of 1.8 (from 305 to 560 µg L−1) until a salinity of 2.1, while Feorg increased only by a factor of 1.3 (from 585 to 793 µg L−1) until a salinity of 0.8 in Laihianjoki (Fig. 4a). At higher salinities, only modest increases in Feorg were measured for both rivers. The difference in the Feorg increase between the rivers was smaller compared to the difference in the Alorg increase between the rivers, which is likely due to the smaller difference in dissolved-Fe concentrations between the rivers (1.4 mg L−1 in Sulvanjoki, 1.0 mg L−1 in Laihianjoki; Table 2). Indeed, the flocculation rate of Al was much higher than that of Fe, as well as that of DOC, which resulted in an increasing proportion of Al of TSM throughout the experiment, whereas the proportions of Fe and POC decreased (Fig. 4b).
POC increased by a factor of 1.6 (from 5.1 to 8.0 mg L−1) until a salinity of 1.6 in Laihianjoki (Fig. 4a), resulting in a steep decrease in the mean apparent density of suspended particles from 1.2 mg µL−1 to 0.06 mg µL−1 in the low salinity range and in a relatively stable and low particle density at higher salinities (Fig. 5). The bucket experiment demonstrated that Laihianjoki had a higher initial proportion of small and dense lithic particles compared to Sulvanjoki, as was shown by the higher initial turbidity (Fig. 2b), lower initial humic FDOM concentration (Fig. 2a), and smaller initial mean particle size (Fig. 2e). It seems that the strong decrease in the mean apparent density of suspended particles in the Laihianjoki flocculator experiments resulted from the intense formation of low-density organic particles, which exceeded the effect of dense lithic particles on the mean apparent density. A comparable decrease in the mean particle density with increasing salinity was not observed in Sulvanjoki, where the mean apparent density of suspended particles remained roughly at 0.05 mg µL−1 despite a relatively stronger increase in POC by a factor of 2.3 (from 3.7 to 8.7 mg L−1) until a salinity of 4.1. The relative stability of the mean apparent particle density in Sulvanjoki was likely due to the low initial proportion of dense lithic particles.
Organic matter flocculation is known to be selective towards aromatic and humic fractions of the organic matter pool (Asmala et al., 2014), which was also apparent in the flocculator experiments. The observed strong decrease in humic fluorescence and UV absorbance at 254 nm (Fig. 6) showed how these fractions were transformed regarding their optical properties or removed through POC formation. Protein-like fluorescence and VIS absorbance at 440 nm showed weaker responses to increasing salinity (Figs. A2 and A3). Linkage between a decrease in CDOM and an increase in POC was more pronounced in Sulvanjoki, suggesting higher susceptibility of organic C from this system for particle formation. The observed very strong coupling between the decrease in humus-like organic matter and the increase in Alorg indicates co-precipitation of these dissolved constituents (Fig. 7a), instead of, for example, preferential organic matter adsorption to lithic particles already present. Coupling of organic matter and Feorg was weaker (Fig. 7b), which suggests that the complexation of Fe with organic matter is less important in overall floc formation. Together with the rapid increase in the floc and flocculi ratio (Fig. 3a) and the decrease in particle density (Fig. 5), it is evident that organically dominated flocs are the preferred pathway for Al especially and to some extent for Fe particle formation.
The higher affinity of Al than Fe to organic matter during flocculation was also reflected in the composition of the organic particle pool. The proportions of Al and Fe in the organic particle pool increased by 35 % and 15 %, respectively, in Laihianjoki and as much as 60 % and 30 %, respectively, in Sulvanjoki over the salinity range of 0 to 2 (Fig. 9a). Such a strong transfer to particulate form indicates efficient capture of dissolved Al and Fe in organically dominated flocs, whose formation is the principal flocculation process in the lowest salinity range (Sect. 5.1). The strong organic flocculation affinities of Al and Fe are in line with previous flocculation studies of boreal rivers that typically have high DOC and Fe concentrations (Pettersson et al., 1997; Yu et al., 2015; Jilbert et al., 2018; Khoo et al., 2022), as well as AS-soil-impacted rivers that also have high Al concentrations (Nordmyr et al., 2008a, b; Nystrand and Österholm, 2013; Nystrand et al., 2016).
In addition to the floc formation, Fe is to a lesser extent precipitated as oxyhydroxides, particularly ferrihydrite, during mixing with seawater of Finnish AS-soil-impacted rivers, while hydroxysulfate minerals are largely absent (Yu et al., 2015). Accordingly, the increasing concentrations of flocculi, beginning when pH exceeds ca. 5.5 at a salinity of 1 in Laihianjoki and 2 in Sulvanjoki, indicate that the precipitation of Al and Fe hydroxides, as well as the potential Al and Fe adsorption on the oxyhydroxide surfaces, also contributes to the particulate Al and Fe pool (Bigham and Nordstom, 2000; Åström and Corin, 2000). However, the low relative concentration of flocculi implies that Al and Fe hydroxides are minor components of the particulate Al and Fe pool.
5.3 Flocculation behaviour of trace metals Co, Mn, and Cu
The proportions of Co and Mn associated with organic particles were < 1 % and < 0.4 %, respectively, and increased only slightly with salinity and pH in both Laihianjoki and Sulvanjoki (Fig. 9b). These observations agree with previous geochemical modelling and field studies in Finnish AS-soil-impacted rivers, which have shown that Co and Mn are weakly associated with organic complexes and are relatively persistent in solution during mixing with seawater in estuaries (Åström and Corin, 2000; Nystrand and Österholm, 2013; Nystrand et al., 2016). The observations are further supported by previous laboratory studies that have shown Mn and Co binding to humic substances to be relatively minor (Sholkovitz, 1978; Hamilton-Taylor et al., 2002). However, the proportions of Mn and in particular Co in the particle pool did increase slightly towards the end of the experiments. Both Mn hydroxide precipitation and Co complexation with organic substances are comparably slow processes and require a pH > 6 (Pontér et al., 1992; Moffet and Ho, 1996; Turner and Mawji, 2005), which was reached only at the end of the experiments (Fig. 3a). It is, therefore, probable that a longer experiment time or a stronger increase in salinity would have resulted in more efficient transfer of Mn and Co to the particle pool. It is worth noting, however, that salinity in the recipient Gulf of Bothnia is lower than the maximum salinity of 6 of the experiment.
Previous studies in the nearby Vöyrinjoki estuary have concluded that Co co-precipitates with Mn oxyhydroxides (Nordmyr et al., 2008a, b; Nystrand et al., 2016), whereas studies elsewhere have concluded Co flocculation to be driven by binding to organic material and decoupled from Mn oxyhydroxide precipitation (Moffet and Ho, 1996). Indeed, the transfer of Co to the particle pool was roughly twice that of Mn in both studied rivers, which suggests that Co flocculation is at least partly decoupled from Mn oxyhydroxide precipitation.
The proportion of Cu in the organic particle pool varied between 10 % and 50 % during the experiment in both rivers (Fig. 9c), which is comparable to other AS-soil-impacted rivers in Finland, where up to 60 % of Cu is found in strong association with humic particles (Pettersson et al., 1997; Nystrand and Österholm, 2013; Nystrand et al., 2016). It is notable that no additional net transfer of Cu to the organic particle pool was observed as a response to the increasing salinity and pH in either river. Also previous laboratory experiments have shown that the binding of Cu to organic ligands, particularly humic substances, may be unaffected by increases in salinity in estuaries (Hamilton-Taylor et al., 2002). Interestingly, up to 56 % of Cu occurs complexed, possibly with organic matter in the Gulf of Bothnia (Bordin et al., 1988). It appears that a substantial proportion of Cu is bound to organic particles in the studied acidic and humus-rich rivers, and this proportion is not significantly changed during mixing with seawater.
5.4 Implications for seaward metal transport
Previous field and geochemical modelling studies on the west coast of Finland have concluded that when acidic metal-rich river waters from the boreal AS soil landscape are discharged to estuaries with higher pH and salinity, Al and Fe to a large extent form complexes with flocculating organic matter and precipitate as Al and Fe oxyhydroxide minerals that are deposited in sediments close to the river mouths (Nordmyr et al., 2008a, b; Nystrand et al., 2016). Flocculator experiments carried out here showed relatively stronger complexation with the organically dominated flocs for Al (Sect. 5.2), which may explain the higher relative Al contents in seafloor sediments in the inner archipelago compared to the outer archipelago (Fig. 1c). In particular, Al was shown to be enriched in the innermost sediment core MGGN-2016-8 above levels that are typically measured in the sea area in sediments deposited before the intensive artificial drainage of the AS soil landscape in the 1960s (Virtasalo et al., 2020), confirming the mechanism of early flocculation and deposition at a short distance from these rivers for Al.
Mn and Co were transferred to the particulate form only in small amounts in the experimental seawater treatments (Fig. 9b). Previous studies have shown that Mn hydroxide precipitation and Co complexation with organic substances are slow processes and take place at a comparably high pH, above 6 (Pontér et al., 1992; Moffet and Ho, 1996; Turner and Mawji, 2005), which suggests that the previously proposed mechanism of Mn precipitation and deposition as Mn oxyhydroxides farther out at sea may be the controlling mechanism of seafloor Mn distribution (Nordmyr et al., 2008a, b; Nystrand et al., 2016). Co transfer to particulate form was twice that of Mn, which highlights Co complexation with flocs in addition to Mn oxyhydroxides. This interpretation is supported by the seaward enrichment patterns of Co and Mn in the front of Laihianjoki and Sulvanjoki, where both Co and Mn are strongly enriched at up to 12 km distance, while a lower Co enrichment continues at a distance of at least 26 km (Virtasalo et al., 2020).
Up to 50 % of total river-borne Cu was bound to humic substances in flocs in Laihianjoki and Sulvanjoki (Fig. 9c), which is similar to other boreal AS-soil-impacted rivers draining to the Gulf of Bothnia (Pettersson et al., 1997; Nystrand and Österholm, 2013; Nystrand et al., 2016). This proportion was not significantly changed due to mixing with seawater in the flocculator experiment. Previous studies in the nearby Vöyrinjoki estuary have documented that Cu to a large extent is deposited in association with POC close to the river mouth (Nordmyr et al., 2008a, b; Nystrand et al., 2016). However, minor Cu enrichment in sediments at least 26 km seaward from the Laihianjoki and Sulvanjoki river mouths requires some Cu to be transported and deposited farther out at sea (Virtasalo et al., 2020). Previous studies have concluded Cu binding to organic ligands to be a significant mechanism for Cu distribution in the Gulf of Bothnia and elsewhere (e.g. van den Berg et al., 1987; Bordin et al., 1988; Muller and Batchelli, 2013).
A previous geochemical study of seafloor sediments in the front of Laihianjoki and Sulvanjoki found that AS-soil-derived metals such as Cu and Co are strongly positively correlated with organic matter, particularly in the 2–6 µm sediment grain size range (Virtasalo et al., 2020). Also Al showed a weak correlation with this grain size range. Organically dominated flocs that were formed during the bucket experiment here were generally larger than 80 µm, and the flocculi were predominantly composed of Al and Fe oxyhydroxides (Sect. 5.1). It seems that the floc size in suspension does not correspond to the size of metal-rich organic grains in the sediments, which is probably the consequence of the post-depositional break-up of flocs to their smaller constituent particles (Mikkelsen et al., 2006; Lee et al., 2012). The large and soft flocs are easily broken after deposition by organic matter degradation and sediment compaction with burial and by sediment mixing by benthic organisms (e.g. Rhoads and Boyer, 1982).
Climate change is predicted to result in increasing temperatures and evapotranspiration during summer in Finland (Olsson et al., 2015). This effect is likely to enhance the drying and oxidation of sulfides in AS soils (Österholm and Åström, 2008; Job et al., 2020) and increase the acidic runoff and metal loading of AS soils to the recipient water bodies (Saarinen et al., 2010; Nystrand et al., 2016). The flocculator experiments showed that Co, Cu, and Mn largely remain in dissolved form during estuarine mixing, which will favour the seaward transport of their riverine concentrations, which are expected to increase due to climate change. Aluminium was more strongly transferred to organically dominated flocs than Fe, and the flocs provide a mechanism for the transport of increasing riverine Al concentrations to the innermost archipelago. Iron is not enriched in the Gulf of Bothnia by loading from the boreal AS soils (Nordmyr at al., 2008a; Nystrand et al., 2016; Virtasalo et al., 2020), and this condition is unlikely to change due to climate change.
This study shows that mixing of acidic and dissolved-metal-rich waters from boreal AS-soil-impacted rivers with seawater induces strong flocculation of dissolved organic matter to the particulate form already at a salinity of 0–2. Al and Fe were strongly transferred to the newly formed organically dominated flocs, which were generally larger than 80 µm. Al transfer to the flocs was stronger than that of Fe, which is also reflected in the previously documented relatively higher Al contents in the seafloor sediments of the innermost parts of the recipient archipelago of the studied rivers. The flocs in the suspended particle pool were complemented by a smaller population of Al- and Fe-oxyhydroxide-dominated flocculi (median size of 11 µm) after pH exceeded ca. 5.5. Cobalt and Mn transfer to the particle pool was weak, although some transfer to Mn oxyhydroxides as well as Co association with flocs was inferred. Therefore, Co and Mn must be transported to the sea in predominantly dissolved form. Up to 50 % of Cu was found to be bound to humic substances in flocs in the AS-soil-impacted rivers, and this proportion did not significantly change during mixing with seawater.
This study highlights that, in addition to salinity, pH should be considered in estuarine flocculation and coastal filter studies, particularly when AS soils are present in the catchment area.
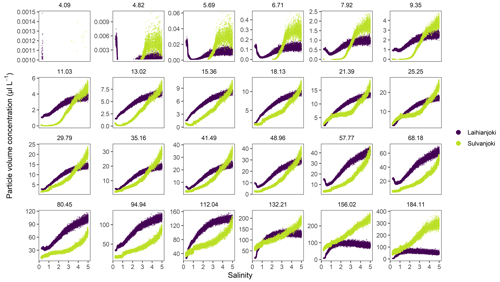
Figure A1Volume concentrations of LISST-100X particle size classes (bins) in the bucket experiment. The size classes are labelled by their median size (µm). The finest 8 (1.18–3.76 µm) of altogether 32 size classes are excluded because they displayed very low concentrations and were not considered further.
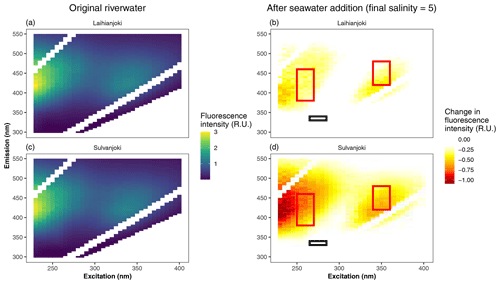
Figure A2Original CDOM fluorescence excitation–emission matrix (a, c) and changes in fluorescence intensity due to salt addition (b, d). A negative change in fluorescence intensity indicates removal of fluorophores, in this case substances with humus-like fluorescence. Humus-like fluorescence peaks (b, d) are marked with red rectangles, and protein-like peaks (T) are marked with a black rectangle. Peaks defined following Coble (1996). In essence, the largest decreases in CDOM fluorescence are observed in the humus-like substances, whereas removal of protein-like substances is negligible.
Suspension particle size, water quality, and geochemical data from the flocculation experiments will be available from PANGAEA (https://doi.pangaea.de/10.1594/PANGAEA, Asmala et al., 2023).
JJV conceptualized the study. EA planned and led the laboratory experiments. PÖ identified the sampling locations. JJV and EA conducted the formal analyses and wrote the original draft. PÖ contributed to the reviewing and editing of the manuscript.
The contact author has declared that none of the authors has any competing interests.
Publisher's note: Copernicus Publications remains neutral with regard to jurisdictional claims in published maps and institutional affiliations.
This study has utilized research infrastructure facilities provided by the Finnish Marine Research Infrastructure (FINMARI) network. We thank Matias Scheinin (Pro Litore) for providing the EXO2 multiparameter sonde used in the experiment. Help from the laboratory personnel at the Tvärminne station is gratefully acknowledged. Two anonymous reviewers are thanked for constructive comments that helped improve the manuscript.
This research has been supported by the Academy of Finland project FERMAID (grant no. 332249).
This paper was edited by Yuan Shen and reviewed by two anonymous referees.
Agrawal, Y. C. and Pottsmith, H. C.: Instruments for particle size and settling velocity observations in sediment transport, Mar. Geol., 168, 89–144, https://doi.org/10.1016/S0025-3227(00)00044-X, 2000.
Andriesse, W. and van Mensvoort, M. E. F.: Acid sulfate soils: distribution and extent, in: Encyclopedia of Soil Science, edited by: Lal, R., CRC Press, Boca Raton, USA, 14–19, https://doi.org/10.1081/E-ESS3-120006641, 2006.
Asmala, E., Bowers, D. G., Autio, R., Kaartokallio, H., and Thomas, D. N.: Qualitative changes of riverine dissolved organic matter at low salinities due to flocculation, J. Geophys. Res.-Biogeo., 119, 1919–1933, https://doi.org/10.1002/2014JG002722, 2014.
Asmala, E., Carstensen, J., Conley, D. J., Slomp, C. P., Stadmark, J., and Voss, M.: Efficiency of the coastal filter: nitrogen and phosphorus removal in the Baltic Sea, Limnol. Oceanogr., 62, S222–S238, https://doi.org/10.1002/lno.10644, 2017.
Asmala, E., Virtasalo, J. J., Scheinin, M., Newton, S., and Jilbert, T.: Role of particle dynamics in processing of terrestrial nitrogen and phosphorus in the estuarine mixing zone, Limnol. Oceanogr., 67, 1–12, https://doi.org/10.1002/lno.11961, 2022.
Asmala, E., Österholm, P., and Virtasalo, J. J.: Suspension particle size, water quality and geochemical data from Laihianjoki and Sulvanjoki flocculation experiments, PANGAEA [data set], https://doi.pangaea.de/10.1594/PANGAEA, in review, 2023.
Åström, M. and Björklund, A.: Geochemistry and acidity of sulphide-bearing postglacial sediments of western Finland, Environ. Geochem. Hlth., 19, 155–164, https://doi.org/10.1023/A:1018462824486, 1997.
Åström, M. and Corin, N.: Abundance, sources and speciation of trace elements in humus-rich streams affected by acid sulphate soils, Aquat. Geochem., 6, 367–383, https://doi.org/10.1023/A:1009658231768, 2000.
Åström, M. E., Österholm, P., Gustafsson, J. P., Nystrand, M., Peltola, P., Nordmyr, L., and Boman, A.: Attenuation of rare earth elements in a boreal estuary, Geochim. Cosmochim. Ac., 96, 105–119, https://doi.org/10.1016/j.gca.2012.08.004, 2012.
Beck, M., Dellwig, O., Fischer, S., Schnetger, B., and Brumsack, H.-J.: Trace metal geochemistry of organic carbon-rich watercourses draining the NW German coast, Estuar. Coast. Shelf S., 104–105, 66–79, https://doi.org/10.1016/j.ecss.2012.03.025, 2012.
Bianchi, T. S.: Biogeochemistry of Estuaries, Oxford University Press, New York, USA, 706 pp., ISBN 0-19-516082-7, 2007.
Bigham, J. M. and Nordstom, D. K.: Iron and aluminum hydroxysulfates from acid sulfate waters, Rev. Mineral. Geochem., 40, 351–403, https://doi.org/10.2138/rmg.2000.40.7, 2000.
Boman, A., Fröjdö, S., Backlund, K., and Åström, M. E.: Impact of isostatic land uplift and artificial drainage on oxidation of brackish-water sediments rich in metastable iron sulfide, Geochim. Cosmochim. Ac., 74, 1268–1281, https://doi.org/10.1016/j.gca.2009.11.026, 2010.
Bordin, G., Perttilä, M., and Scheinen, H.: Distribution of total and ASV-labile cadmium, lead, and copper in sea water in the northern parts of the Baltic Sea in 1985–1986, Mar. Pollut. Bull., 19, 325–327, https://doi.org/10.1016/0025-326X(88)90428-6, 1988.
Boyle, E., Collier, R., Dengler, A. T., Edmond, J. M., Ng, A. C., and Stallard, R. F.: On the chemical mass-balance in estuaries, Geochim. Cosmochim. Ac., 38, 1719–1728, https://doi.org/10.1016/0016-7037(74)90188-4, 1974.
Breilin, O., Kotilainen, A., Nenonen, K., and Räsänen, M.: The unique moraine morphology, stratotypes and ongoing geological processes at the Kvarken Archipelago on the land uplift area in the western coast of Finland, in: Quaternary Studies in the Northern and Arctic Regions of Finland: Proceedings of the Workshop Organized within the Finnish National Committee for Quaternary Research (INQUA), Kilpisjärvi Biological Station, Finland, 13–14 January 2005, edited by: Ojala, A. E. K., Geological Survey of Finland, Special Paper, 40, 97–111, 2005.
Canuel, E. A. and Hardison, A. K.: Sources, ages, and alteration of organic matter in estuaries, Annu. Rev. Mar. Sci., 8, 409–434, https://doi.org/10.1146/annurev-marine-122414-034058, 2016.
Carstea, E. M., Popa, C. L., Baker, A., and Bridgeman, J.: In situ fluorescence measurements of dissolved organic matter: a review, Sci. Total Environ., 699, 134361, https://doi.org/10.1016/j.scitotenv.2019.134361, 2020.
Coble, P.: Characterization of marine and terrestrial DOM in seawater using excitation–emission matrix spectroscopy, Mar. Chem., 51, 325–346, https://doi.org/10.1016/0304-4203(95)00062-3, 1996.
Dent, D. L. and Pons, L. J.: A world perspective on acid sulphate soils, Geoderma, 67, 263–276, https://doi.org/10.1016/0016-7061(95)00013-E, 1995.
Fältmarsch, R. M., Åström, M. E., and Vuori, K.-M.: Environmental risks of metals mobilised from acid sulphate soils in Finland: a literature review, Boreal Environ. Res., 13, 444–456, 2008.
Fu, J., Tang, X.-L., Zhang, J., and Balzer, W.: Estuarine modification of dissolved and particulate trace metals in major rivers of East-Hainan, China, Cont. Shelf Res., 57, 59–72, https://doi.org/10.1016/j.csr.2012.06.015, 2013.
Gregory, J. and O'Melia, C. R.: Fundamentals of flocculation, Crit. Rev. Env. Contr., 19, 185–230, https://doi.org/10.1080/10643388909388365, 1989.
Hamilton-Taylor, J., Postill, A. S., Tipping, E., and Harper, M. P.: Laboratory measurements and modeling of metal–humic interactions under estuarine conditions, Geochim. Cosmochim. Ac., 66, 403–415, https://doi.org/10.1016/S0016-7037(01)00777-3, 2002.
Häusler, K., Moros, M., Wacker, L., Hammerschmidt, L., Dellwig, O., Leipe, T., Kotilainen, A., and Arz, H. W.: Mid- to late Holocene environmental separation of the northern and central Baltic Sea basins in response to differential land uplift, Boreas, 46, 111–128, https://doi.org/10.1111/bor.12198, 2017.
Hudd, R. and Kjellman, J.: Bad matching between hatching and acidification: a pitfall for the burbot, Lota lota, off the river Kyrönjoki, Baltic Sea, Fish. Res., 55, 153–160, https://doi.org/10.1016/S0165-7836(01)00303-4, 2002.
Jilbert, T., Asmala, E., Schröder, C., Tiihonen, R., Myllykangas, J.-P., Virtasalo, J. J., Kotilainen, A., Peltola, P., Ekholm, P., and Hietanen, S.: Impacts of flocculation on the distribution and diagenesis of iron in boreal estuarine sediments, Biogeosciences, 15, 1243–1271, https://doi.org/10.5194/bg-15-1243-2018, 2018.
Job, T., Penny, D., and Morgan, B.: Geochemical signatures of acidic drainage recorded in estuarine sediments after an extreme drought, Sci. Total Environ., 749, 141435, https://doi.org/10.1016/j.scitotenv.2020.141435, 2020.
Kakkuri, J.: Fennoscandian land uplift: past, present and future, in: From the Earth's Core to Outer Space, edited by: Haapala, I., Lecture Notes in Earth System Sciences, Springer, Berlin, Heidelberg, 137, 127–136, https://doi.org/10.1007/978-3-642-25550-2_8, 2012.
Khoo, C. L. L., Sipler, R. E., Fudge, A. R., Foroutani, M. B., Boyd, S. G., and Ziegler, S. E.: Salt-induced flocculation of dissolved organic matter and iron is controlled by their concentration and ratio in boreal coastal systems, J. Geophys. Res.-Biogeo., 127, e2022JG006844, https://doi.org/10.1029/2022JG006844, 2022.
Korhonen, J. and Haavalammi, E.: Hydrologinen vuosikirja 2006–2010/Hydrological Yearbook 2006–2010, Suomen Ympäristö, Helsinki, 8/2012, 234 pp., http://hdl.handle.net/10138/38812 (last access: 26 May 2023), 2012.
Kuosa, H., Fleming-Lehtinen, V., Lehtinen, S., Lehtiniemi, M., Nygård, H., Raateoja, M., Raitaniemi, J., Tuimala, J., Uusitalo, L., and Suikkanen, S.: A retrospective view of the development of the Gulf of Bothnia ecosystem, J. Marine Syst., 167, 78–92, https://doi.org/10.1016/j.jmarsys.2016.11.020, 2017.
Lahermo, P., Väänänen, P., Tarvainen, T., and Salminen, R.: Geochemical Atlas of Finland, Part 3, Environmental geochemistry – stream waters and sediments, Geological Survey of Finland, Espoo, Finland, 149 pp., ISBN 9516906788, 1996.
Lee, B. J., Fettweis, M., Toorman, E., and Molz, F. J.: Multimodality of a particle size distribution of cohesive suspended particulate matters in a coastal zone, J. Geophys. Res., 117, C03014, https://doi.org/10.1029/2011JC007552, 2012.
Massicotte, P.: eemR: tools for pre-processing emission-excitation-matrix (EEM) fluorescence data, CRAN [code], https://CRAN.R-project.org/package=eemR (last access: 3 July 2023), 2016.
Mikkelsen, O. A., Hill, P. S., and Milligan, T. G.: Single-grain, microfloc and macrofloc volume variations observed with a LISST-100 and a digital floc camera, J. Sea Res., 55, 87–102, https://doi.org/10.1016/j.seares.2005.09.003, 2006.
Moffet, J. W. and Ho, J.: Oxidation of cobalt and manganese in seawater via a common microbially catalyzed pathway, Geochim. Cosmochim. Ac., 60, 3415–3424, https://doi.org/10.1016/0016-7037(96)00176-7, 1996.
Mosley, L. M. and Liss, P. S.: Particle aggregation, pH changes and metal behaviour during estuarine mixing: review and integration, Mar. Freshwater Res., 71, 300–310, https://doi.org/10.1071/MF19195, 2020.
Muller, F. L. L. and Batchelli, S.: Copper binding by terrestrial versus marine organic ligands in the coastal plume of River Thurso, North Scotland, Estuar. Coast. Shelf S., 133, 137e146, https://doi.org/10.1016/j.ecss.2013.08.024, 2013.
Murphy, K. R., Butler, K. D., Spencer, R. G. M., Stedmon, C. A., Boehme, J. R., and Aiken, G. R.: The measurement of dissolved organic matter fluorescence in aquatic environments: an interlaboratory comparison, Environ. Sci. Technol., 44, 9405–9412, https://doi.org/10.1021/es102362t, 2010.
Nordmyr, L., Åström, M., and Peltola, P.: Metal pollution of estuarine sediments caused by leaching of acid sulphate soils, Estuar. Coast. Shelf S., 76, 141–152, https://doi.org/10.1016/j.ecss.2007.07.002, 2008a.
Nordmyr, L., Österholm, P., and Åström, M.: Estuarine behaviour of metal loads leached from coastal lowland acid sulphate soils, Mar. Environ. Res., 66, 378–393, https://doi.org/10.1016/j.marenvres.2008.06.001, 2008b.
Nyman, A., Johnson, A., Yu, C., Dopson, M., and Åström, M.: Multi-element features of active acid sulfate soils across the Swedish coastal plains, Appl. Geochem., 152, 105653, https://doi.org/10.1016/j.apgeochem.2023.105653, 2023.
Nystrand, M. I. and Österholm, P.: Metal species in a boreal river system affected by acid sulfate soils, Appl. Geochem., 31, 133–141, https://doi.org/10.1016/j.apgeochem.2012.12.015, 2013.
Nystrand, M. I., Österholm, P., Nyberg, M. E., and Gustafsson, J. P.: Metal speciation in rivers affected by enhanced soil erosion and acidity, Appl. Geochem., 27, 906–916, https://doi.org/10.1016/j.apgeochem.2012.01.009, 2012.
Nystrand, M. I., Österholm, P., Yu, C., and Åström, M.: Distribution and speciation of metals, phosphorus, sulfate and organic material in brackish estuary water affected by acid sulfate soils, Appl. Geochem., 66, 264–274, https://doi.org/10.1016/j.apgeochem.2016.01.003, 2016.
Olsson, T., Jakkila, J., Veijalainen, N., Backman, L., Kaurola, J., and Vehviläinen, B.: Impacts of climate change on temperature, precipitation and hydrology in Finland – studies using bias corrected Regional Climate Model data, Hydrol. Earth Syst. Sci., 19, 3217–3238, https://doi.org/10.5194/hess-19-3217-2015, 2015.
Österholm, P. and Åström, M.: Spatial trends and losses of major and trace elements in agricultural acid sulphate soils distributed in the artificially drained Rintala area, W. Finland, Appl. Geochem., 17, 1209–1218, https://doi.org/10.1016/S0883-2927(01)00133-0, 2002.
Österholm, P. and Åström, M.: Meteorological impacts on the water quality in the Pajuluoma acid sulphate area, W. Finland, Appl. Geochem., 23, 1594–1606, https://doi.org/10.1016/j.apgeochem.2008.01.011, 2008.
Pettersson, C., Allard, B., and Borén, H.: River discharge of humic substances and humic-bound metals to the Gulf of Bothnia, Estuar. Coast. Shelf S., 44, 533–541, https://doi.org/10.1006/ecss.1996.0159, 1997.
Pirinen, P., Simola, H., Aalto, J., Kaukoranta, J.-P., Karlsson, P., and Ruuhela, R.: Climatological statistics of Finland 1981–2010, Finnish Meteorological Institute, Helsinki, Reports 2012:1, 83 pp., 2012.
Pontér, C., Ingri, J., and Boström, K.: Geochemistry of manganese in the Kalix River, northern Sweden, Geochim. Cosmochim. Ac., 56, 1485–1494, https://doi.org/10.1016/0016-7037(92)90218-8, 1992.
Pujo-Pay, M. and Raimbault, P.: Improvement of the wet-oxidation procedure for simultaneous determination of particulate organic nitrogen and phosphorus collected on filters, Mar. Ecol. Prog. Ser., 105, 203–207, https://doi.org/10.3354/meps105203, 1994.
Rhoads, D. C. and Boyer, L. F.: The effects of marine benthos on physical properties of sediments: a successional perspective, in: Animal-Sediment Relations, edited by: McCall, P. L. and Tevesz, M. J. S., Topics in Geobiology, Springer, Boston, MA, 100, 3–52, https://doi.org/10.1007/978-1-4757-1317-6_1, 1982.
Roos, M. and Åström, M.: Hydrochemistry of rivers in an acid sulphate soil hotspot area in western Finland, Agr. Food Sci., 14, 24–33, https://doi.org/10.2137/1459606054224075, 2005.
Saarinen, T., Vuori, K.-M., Alasaarela, E., and Kløve, B.: Long-term trends and variation of acidity, CODMn and colour in coastal rivers of Western Finland in relation to climate and hydrology, Sci. Total Environ., 408, 5019–5027, https://doi.org/10.1016/j.scitotenv.2010.07.009, 2010.
Saarnisto, M. and Saarinen, T.: Deglaciation chronology of the Scandinavian Ice Sheet from the Lake Onega Basin to the Salpausselkä end moraines, Global Planet. Change, 31, 387–405, https://doi.org/10.1016/S0921-8181(01)00131-X, 2001.
Sauramo, M.: The Quaternary geology of Finland, Bulletin de la Commission Géologique de Finlande, Helsinki, 86, 1–110, 1929.
Schwertmann, U.: Solubility and dissolution of iron oxides, Plant Soil, 130, 1–25, https://doi.org/10.1007/BF00011851, 1991.
Shiller, A. M. and Boyle, E. A.: Trace elements in the Mississippi River Delta outflow region: behavior at high discharge, Geochim. Cosmochim. Ac., 55, 3241–3251, https://doi.org/10.1016/0016-7037(91)90486-O, 1991.
Sholkovitz, E. R.: Flocculation of dissolved organic and inorganic matter during the mixing of river water and seawater, Geochim. Cosmochim. Ac., 40, 831–845, https://doi.org/10.1016/0016-7037(76)90035-1, 1976.
Sholkovitz, E. R.: The flocculation of dissolved Fe, Mn, Al, Cu, Ni, Co and Cd during estuarine mixing, Earth Planet. Sc. Lett., 41, 77–86, https://doi.org/10.1016/0012-821X(78)90043-2, 1978.
Sohlenius, G. and Öborn, I.: Geochemistry and partitioning of trace metals in acid sulphate soils in Sweden and Finland before and after sulphide oxidation, Geoderma, 122, 167–175, https://doi.org/10.1016/j.geoderma.2004.01.006, 2004.
Stroeven, A. P., Hättestrand, C., Kleman, J., Heyman, J., Fabel, D., Fredin, O., Goodfellow, B. W., Harbor, J. M., Jansen, J. D., Olsen, L., Caffee, M. W., Fink, D., Lundqvist, J., Rosqvist, G. C., Strömberg, B., and Jansson, K. N.: Deglaciation of Fennoscandia, Quaternary Sci. Rev., 147, 91–121, https://doi.org/10.1016/j.quascirev.2015.09.016, 2016.
Sundström, R., Åström, M., and Österholm, P.: Comparison of the metal content in acid sulfate soil runoff and industrial effluents in Finland, Environ. Sci. Technol., 36, 4269–4272, https://doi.org/10.1021/es020022g, 2002.
Sutela, T. and Vehanen, T.: The effects of acidity and aluminium leached from acid-sulphate soils on riverine fish assemblages, Boreal Environ. Res., 22, 385–391, 2017.
Thomas, L. P., Marino, B. M., Szupiany, R. N., and Gallo, M. N.: Characterisation of the suspended particulate matter in a stratified estuarine environment employing complementary techniques, Cont. Shelf Res., 148, 37–43, https://doi.org/10.1016/j.csr.2017.08.024, 2017.
Turner, A. and Mawji, E.: Hydrophobicity and reactivity of trace metals in the low-salinity zone of a turbid estuary, Limnol. Oceanogr., 50, 1011–1019, https://doi.org/10.4319/lo.2005.50.3.1011, 2005.
van den Berg, C. M. G., Merks, A. G. A., and Duursma, E. K.: Organic complexation and its control of the dissolved concentrations of copper and zinc in the Scheldt estuary, Estuar. Coast. Shelf S., 24, 785–797, https://doi.org/10.1016/0272-7714(87)90152-1, 1987.
Virtasalo, J. J., Kotilainen, A. T., Räsänen, M. E., and Ojala, A. E. K.: Late-glacial and post-glacial deposition in a large, low relief, epicontinental basin: the northern Baltic Sea, Sedimentology, 54, 1323–1344, https://doi.org/10.1111/j.1365-3091.2007.00883.x, 2007.
Virtasalo, J. J., Österholm, P., Kotilainen, A. T., and Åström, M. E.: Enrichment of trace metals from acid sulfate soils in sediments of the Kvarken Archipelago, eastern Gulf of Bothnia, Baltic Sea, Biogeosciences, 17, 6097–6113, https://doi.org/10.5194/bg-17-6097-2020, 2020.
Wallin, J., Karjalainen, A. K., Schultz, E., Järvistö, J., Leppänen, M., and Vuori, K.-M.: Weight-of-evidence approach in assessment of ecotoxicological risks of acid sulphate soils in the Baltic Sea river estuaries, Sci. Total Environ., 508, 452–461, https://doi.org/10.1016/j.scitotenv.2014.11.073, 2015.
Wolski, T., Wiśniewski, B., Giza, A., Kowalewska-Kalkowska, H., Boman, H., Grabbi-Kaiv, S., Hammarklint, T., Holfort, J., and Lydeikaite, Z.: Extreme sea levels at selected stations on the Baltic Sea coast, Oceanologia, 56, 259–290, https://doi.org/10.5697/oc.56-2.259, 2014.
Yli-Halla, M., Puustinen, M., and Koskiaho, J.: Area of cultivated acid sulfate soils in Finland, Soil Use Manage., 15, 62–67, https://doi.org/10.1111/j.1475-2743.1999.tb00065.x, 1999.
Yu, C., Virtasalo, J. J., Karlsson, T., Peltola, P., Österholm, P., Burton, E. D., Arppe, L., Hogmalm, J. K., Ojala, A. E. K., and Åström, M. E.: Iron behavior in a northern estuary: large pools of non-sulfidized Fe(II) associated with organic matter, Chem. Geol., 413, 73–85, https://doi.org/10.1016/j.chemgeo.2015.08.013, 2015.