the Creative Commons Attribution 4.0 License.
the Creative Commons Attribution 4.0 License.
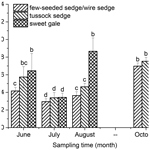
Plant mercury accumulation and litter input to a Northern Sedge-dominated Peatland
Ting Sun
Brian A. Branfireun
Plant foliage plays an essential role in accumulating mercury (Hg) from the atmosphere and transferring it to soils in terrestrial ecosystems, and many studies have focused on forested ecosystems. Hg input from plants to northern peatland peat soils has not been nearly as well studied and is likely equally important from a mass balance perspective. In this study, we investigated the accumulation of atmospheric Hg by the dominant plant species, few-seeded sedge (Carex oligosperma Michx.), wire sedge (Carex lasiocarpa Ehrh), tussock sedge (Carex stricta Lamb.), and sweet gale (Myrica gale L.), in a boreal sedge-dominated peatland. Foliar Hg concentrations decreased early in the growing season due to growth dilution, and after that they were subsequently positively correlated with leaf age (time). Hg concentrations were 1.4–1.7 times higher in sweet gale than in sedges. A leaching experiment showed that sweet gale leached less Hg but more bioaccessible dissolved organic matter (DOM) by mass than sedges. Leaching of Hg was positively related to the aromaticity of DOM in leachate, suggesting the importance of DOM with higher aromaticity in controlling Hg mobility. Annual inputs of Hg through senesced leaf material to peat soils were 9.88, 1.62, and 8.29 mg ha−1 yr−1 for sweet gale, tussock sedge, and few-seeded sedge and wire sedge, respectively. Future investigations into foliar Hg accumulation and input from other plant species to the sedge-dominated peatland are needed to estimate the annual Hg inputs precisely.
- Article
(3687 KB) -
Supplement
(437 KB) - BibTeX
- EndNote
Mercury (Hg), especially methylmercury (MeHg), is a global concern due to its potential toxicity and ubiquitous presence in the environment (Morel et al., 1998). Hg is emitted to the atmosphere from both natural (e.g., volcanoes, wildfires, geothermal activity) and anthropogenic sources (e.g., coal combustion, artisanal gold mining, incineration) (Schroeder and Munthe, 1998; Streets et al., 2011). Atmospheric Hg exists as gaseous elemental mercury (GEM, Hg(0)), reactive gaseous mercury (RGM, Hg(II)), and particulate-bound mercury (PBM, Hgp) with GEM as the dominant species (> 95 %) (Schroeder and Munthe, 1998). RGM and PBM have shorter atmospheric residence time ranging from hours to days, whereas GEM has a longer atmospheric residence time of several months to a year and thus is transported globally (Schroeder and Munthe, 1998). These atmospheric Hg species are eventually deposited into aquatic and terrestrial ecosystems via wet deposition (precipitation, such as rain, snow, and fog) and dry deposition (particle settling or direct partitioning to vegetation, water, and soil surface or direct absorption by vegetation foliage) (Lindberg et al., 2007). Hg dry deposition is a larger input than wet deposition to vegetated terrestrial landscapes, contributing 70 %–85 % of total Hg deposition (dry and wet deposition) in terrestrial ecosystems (Graydon et al., 2008; Risch et al., 2012, 2017; St Louis et al., 2001; Wang et al., 2016; Zhang et al., 2016), and more than 70 % of Hg dry deposition is by vegetation litterfall and incorporation into soil organic matter (SOM) (Obrist et al., 2017; Wang et al., 2016).
Vegetation is generally considered a sink for atmospheric Hg, with the majority of Hg in vegetation leaves accumulated from the atmosphere (Jiskra et al., 2018; Obrist et al., 2017). Plant leaves accumulate Hg from the atmosphere mainly through stomatal uptake (Lindberg et al., 1992). Stamenkovic and Gustin (2009) suggested that the non-stomatal pathway of Hg deposition to the leaf cuticle and subsequent retention and incorporation into leaf tissue also play an important role in accumulating atmospheric Hg. Plant roots are thought to generally act as a barrier to Hg transport from soils to shoots (Wang et al., 2015), and it has been shown that less than 10 % of Hg in roots is transported to the aboveground portion of plants (Ericksen et al., 2003; Mao et al., 2013). Some studies have found that a great proportion of foliar Hg in halophytes in salt marshes was translocated from the root (Canário et al., 2017; Cabrita et al., 2019; Weis and Weis, 2004). The plausible reason is that plants in the hydroponic growth system have fewer apoplastic barriers (i.e., Casparian bands and suberin lamellae) in root architecture than plants grown in contaminated soils (Redjala et al., 2011).
Forest ecosystems are important sinks of atmospheric Hg and have received widespread attention from researchers (Risch et al., 2012; St Louis et al., 2001; Wang et al., 2016; Zhang et al., 2009); however, studies about foliar Hg accumulation in other plant types in boreal peatland ecosystems are few (see Moore et al., 1995) despite their critical role in the carbon (Gorham, 1991) and Hg cycles (Grigal, 2003). Boreal peatlands are a type of wetland that stores large amounts of Hg (Grigal, 2003) and can be major MeHg sources to downstream ecosystems (Branfireun et al., 1996; Mitchell et al., 2008; St Louis et al., 1994), given their anaerobic conditions, non-limiting amounts of inorganic Hg, and often available but limited amounts of sulfate (Blodau et al., 2007; Schmalenberger et al., 2007) and bioaccessible carbon facilitating net MeHg production (Mitchell et al., 2008). Elucidation of foliar Hg input from the dominant plant types to boreal peatlands is important to further estimate the supply of bioavailable Hg(II) for net MeHg production.
Previous studies have found that the majority of Hg in plant leaves in wetlands was from the atmosphere (Brahmstedt et al., 2021; Enrico et al., 2016; Fay and Gustin, 2007) and nonvascular plants (e.g., fungi, lichens, and mosses) had higher foliar Hg concentrations than vascular plants (Moore et al., 1995; Pech et al., 2022). Although foliar Hg concentration is lower in vascular plants than in nonvascular plants, Hg mass input to peatlands may be substantial, given the greater litter input from vascular plants than from nonvascular plants (Frolking et al., 2001). With more bioaccessible litter than bryophytes (Hobbie, 1996; Lyons and Lindo, 2019), vascular plants also have a faster initial decomposition rate (0.2 yr−1) than bryophytes (0.05–0.08 yr−1) (Frolking et al., 2001), leading to a rapid Hg release to the soil. Boreal peatlands are experiencing rising temperatures due to climate change (IPCC, 2018), which is likely to both increase aboveground biomass in vascular-plant-dominated peatlands (Tian et al., 2020) and promote a shift from moss-dominated to more vascular-plant-dominated plant communities (Buttler et al., 2015; Dieleman et al., 2015; Weltzin et al., 2000), further affecting Hg deposition (Zhang et al., 2016). To date, the amount of atmospheric Hg accumulated in dominant plants in the vascular-plant-dominated (i.e., graminoid plants and shrubs) peatlands, an important type of boreal wetland (Rydin and Jeglum, 2013), is unknown.
Foliar Hg eventually enters peat soils via litterfall and is expected to follow the ensuing sequence: (1) wash-off of aerosols, particles, and gases from leaf surfaces; (2) leaching of water-soluble components; and (3) incorporation into SOM after the microbial decomposition of litter. Leaching is the initial phase of litter breakdown in aquatic environments and can rapidly release up to 30 % dissolved matter, primarily dissolved organic matter (DOM) within 24 h after immersion of litter (Gessner et al., 1999). It has been established that DOM is closely related to Hg mobility in terrestrial and aquatic ecosystems (Haitzer et al., 2002; Ravichandran, 2004; Kneer et al., 2020), given the strong affinity between Hg and reduced sulfur groups (i.e., thiols) in DOM (Xia et al., 1999). DOM with higher aromaticity has more thiol ligands and has a stronger correlation with Hg (Dittman et al., 2009). The rapid and abundant leaching of DOM, especially those with higher aromaticity from litterfall, may lead to large amounts of Hg leaching. The amount of rapidly released Hg during litter leaching is unknown and needs to be elucidated because more recently deposited Hg appears to be more readily methylated than “old” Hg in peat soils (Branfireun et al., 2005; Hintelmann et al., 2002). Despite previous studies showing that Hg mass in live leaf leachate is insignificant compared to that on leaf surfaces and in SOM (Rea et al., 2000, 2001), litterfall generally lacks structural integrity and likely leaches more Hg compared to live leaves.
The overall objective of this study is to link the vascular plant community (i.e., sedges and shrubs) to the peatland Hg cycle in a vascular-plant-dominated fen-type peatland. We use “sedge-dominated fen” instead of “vascular-plant-dominated fen-type peatland” hereafter, given that sedges are the primarily dominant plants in this study site (Webster and McLaughlin, 2010). The specific objectives of this study are to
-
quantify the mass accumulation of atmospherically derived Hg in leaves of dominant plant species in a sedge-dominated fen over a growing season
-
estimate the Hg input from the litter of different plant species and through litter leaching to peat soils
-
clarify the role of DOM characteristics in controlling Hg leaching
-
estimate the annual areal loading of foliar Hg of different plant species to peat soils.
2.1 Study site
Samples were collected from a sedge-dominated fen (10.2 ha) located in an 817 ha sub-watershed of the Lake Superior basin near White River, Ontario, Canada (48∘21′ N, 85∘21′ W). The growing season is roughly from June to September. The sedge-dominated fen is mostly open, and the vegetation community is dominated by three sedge species: few-seeded sedge (Carex oligosperma Michx.), wire sedge (Carex lasiocarpa Ehrh), and tussock sedge (Carex stricta Lamb.) (Lyons and Lindo, 2019). Sweet gale (Myrica gale L.) is the dominant shrub at this site (Lyons and Lindo, 2019; Palozzi and Lindo, 2017). The mean species percent cover of few-seeded sedge, wire sedge, tussock sedge, and sweet gale from the sedge-dominated fen is 35.0 ± 21.79 %, 0.3 ± 0.12 %, 73.0 ± 18.81 %, 44.8 ± 10.63 % (average ± standard error (SE)), respectively (Palozzi and Lindo, 2017). Details of the study site and the characteristics of these plants are provided in the Supplement. In this study, few-seeded sedges and wire sedges were mixed during plant sample collection as they are indistinguishable in size and form from one another when not in flower/seed, and they frequently co-occur.
2.2 Sample collection and analysis
Five locations several hundred meters apart were selected in the sedge-dominated fen to serve as within-site replicates to account for potential local-scale variability. These five locations were roughly evenly distributed over this study area. Approximately fifty whole leaves of each few-seeded sedge/wire sedge, tussock sedge, and sweet gale were collected from each location using a clean blade in the middle of June, July, August, and after senescence at the beginning of October 2018 in each location, totaling 60 samples. For the October sampling event, the sedge leaves were still standing with the lower sections green, and although senesced, shrub leaves were sampled from the branch to ensure that there was no mixing with previous years' fallen leaves. Disposable nitrile gloves were worn during the sample collection. All samples were double bagged with two polyethylene bags and transported to the lab using a clean cooler. Leaves of each species that were collected from each location in October 2018 were divided for foliar total Hg (THg) analyses and a foliar Hg leaching experiment. Leaves were stored frozen until they were returned to the university laboratory.
For estimation of annual biomass of senesced leaves, seven 0.25 m2 (0.5 m × 0.5 m) plots several hundred meters apart were selected at the end of August 2019 during senescence and before leaf off. All aboveground biomass of few-seeded sedge/wire sedge and tussock sedge and all aboveground leaf biomass of sweet gale were collected separately using a clean blade from each 0.25 m2 plot. All vegetation samples were stored by species in paper bags, transported to the lab, and then oven-dried at 60 ∘C for a minimum of 48 h. Dried leaves of each species in each plot were sorted and weighed to estimate senesced leaf biomass of each species for each plot. The senesced leaf biomass of each species per hectare per year was calculated and expressed as milligrams per hectare per year (mg ha−1 yr−1).
Foliar total mercury, C content, and N content. In the laboratory, leaf samples for chemical analyses were rinsed three times with deionized water (18.2 MΩ cm), freeze-dried for 48 h, ground and homogenized, and then analyzed using a Milestone™ DMA-80 (EPA method 7473). Leaf C content (% C; ) and N content (% N; ) before and after the foliar Hg leaching experiment were analyzed using a CNSH analyzer (vario ISOTOPE cube; Elementar). The ratio of leaf C content and N content (C : N) was calculated. Detailed information concerning analytical methods are described in the Supplement, including analysis of foliar total Hg, % C, and % N.
Foliar mercury leaching experiment. The foliar leaching experimental procedure followed the design of Rea et al. (2000) and Del Giudice and Lindo (2017). Senesced leaves of sedges and sweet gale collected in October 2018 were rinsed twice with 100 mL of deionized water (18.2 MΩ cm) to quantify particulate or loosely bound Hg and DOM that can be easily removed/leached from the leaf surface. This water was reserved for subsequent analysis. After rinsing, the leaves were oven-dried at a low temperature (40 ∘C) for 48 h, and then the leaves of each species from each location were relatively evenly separated into three groups and weighed, totaling 45 groups. These oven-dried senesced leaf samples were immersed in 150 mL of deionized water in clean 250 mL PETG bottles. All PETG bottles were capped, double bagged, and incubated in the dark at room temperature (∼ 21 ∘C) for 48 h. Senesced leaf materials were gently swirled at the beginning of the leaching experiment to ensure complete wetting. Following the leaching, the leachate was vacuum filtered through a 0.45 µm glass fiber filter into clean 250 mL PETG bottles. Leachate from each sample was split into two aliquots. One was preserved by acidifying to 0.5 % (vol vol) with high-purity HCl for dissolved total Hg (THgaq) analysis and stored in 250 mL PETG bottles; the other was stored in the clean 60 mL Amber glass bottles and analyzed within 2 d for the quantity and characteristics of DOM. All samples were stored in the dark at 4 ∘C for further analysis. Method blanks of the leaching experiment were performed at the same time following the same procedure.
Senesced leaf material was taken out of each PETG bottle, oven-dried at 40 ∘C for 48 h, and re-weighed after leaching. The dry leaf weight before and after the leaching process was used to calculate the mass loss. These re-dried senesced leaf samples after leaching were ground and homogenized before the measurement for % C and % N as described above.
The dissolved total Hg (THgaq) concentrations in the rinse water and leachate were analyzed using Environmental Protection Agency (EPA) method 1631. Dissolved organic matter is quantified analytically as dissolved organic carbon (DOC). DOC concentrations in rinse water and leachate were measured using an iTOC Aurora 1030 (OI Analytical, College Station, TX, USA) using the persulfate wet oxidation method. Details on the analytical procedures and quality assurance and quality control (QA/QC) data for concentrations of THgaq and DOC are provided in the Supplement.
DOM in leachate was characterized as specific ultraviolet absorbance at a wavelength of 254 nm (SUVA254), an indicator of the molecular weight (or size) and aromaticity (the content of aromatic molecules) of DOM (Weishaar et al., 2003). Higher SUVA254 values suggest that DOM contains more high-molecular-weight and aromatic molecules (Weishaar et al., 2003). Fluorescence excitation–emission matrices (EEMs) were also collected for calculating informative optical indices that reflect differences in DOM characteristics in leachate. The reported EEMs were then converted to optical indices using R Software (R Core Team, 2012). Three common indices were chosen in this study: the fluorescence index (FI), the humification index (HIXEM), and the biological index or “freshness” index (BIX). Lower FI values (< 1.2) indicate that DOM is terrestrially derived (resulting from decomposition and leaching of plant and soil organic matter) and has higher aromaticity, while higher FI values (> 1.8) indicate that DOM is microbially derived (originating from processes as extracellular release and leachate of algae and bacteria) and has lower aromaticity (Fellman et al., 2010; McKnight et al., 2001). High HIXEM (> 1.0) values reflect the high humification of DOM and that DOM is composed of more highly condensed and higher-molecular-weight molecules (Fellman et al., 2010; Huguet et al., 2009). Higher BIX values (> 1.0) reflect that more low-molecular-weight DOM was recently produced by microbes (Fellman et al., 2010; Huguet et al., 2009). Details on the analytical procedures and QA/QC data for SUVA254, FI, HIXEM, and BIX are provided in the Supplement.
Results were analyzed using IBM SPSS statistics software (IBM SPSS Inc. 24.0). The repeated-measures ANOVA was performed to compare the difference in foliar THg concentrations among different plant species over the growing season and to analyze the effect of leaf age on foliar Hg concentrations. Linear regressions were analyzed to examine the relationship between foliar THg accumulation and leaf age. Differences in foliage quality (% C, % N, and C : N) were analyzed using a multivariate ANOVA. One-way ANOVA was used to determine the effects of plant species on concentrations of THgaq and DOM quantity and characteristics in leachate. The repeated-measures ANOVA, multivariate ANOVA, and one-way ANOVA were followed by a post hoc test (Bonferroni's significant difference; honestly significant difference at the 95 % confidence interval). Weighed least squares regression was used to examine the nature of the relationship between THgaq concentrations and SUVA254 in leachate. Data are presented as the mean ± standard deviation (SD). Coefficient of determination (R2) and significant p values (p) are presented for linear regression fits, and p<0.05 was considered significant.
4.1 Foliar mercury accumulation in peatland plants
Foliar THg concentrations were related to time/leaf age (, p<0.001) and plant species (, p<0.001) (Fig. 1). Based on post hoc tests, foliar THg concentrations were significantly different between plant species and between the sampling months, except that there was no significant difference in foliar THg concentrations between June and August. The mean foliar THg concentrations (n=5) in June followed the sequence few-seeded sedge/wire sedge < tussock sedge < sweet gale. In July foliar THg concentrations decreased by 30 % (few-seeded sedge/wire sedge), 40 % (tussock sedge), and 47 % (sweet gale). The decrease in THg concentrations is likely because of leaf growth dilution, although changes in leaf biomass were not quantified as part of this study. Foliar THg concentrations were positively related to time after July (few-seeded sedge/wire sedge: , p<0.001, R2=0.93; tussock sedge: , p<0.001, R2=0.94; sweet gale: , p<0.001, R2=0.84). The mean foliar THg concentrations in October of few-seeded sedge/wire sedge, tussock sedge, and sweet gale were 1.7, 1.3, and 2.0 times higher than the initial concentrations in June. This result showed a clear pattern of continuous THg accumulation in foliage in boreal peatland plant species over time as has been shown for forests (Laacouri et al., 2013; Rea et al., 2002), which can be attributed to foliar Hg accumulation from the air, given that plant roots act as a barrier to Hg transport from soils to shoots (Wang et al., 2015). Further studies are needed to quantify the contribution of atmospheric and soil Hg to foliar Hg.
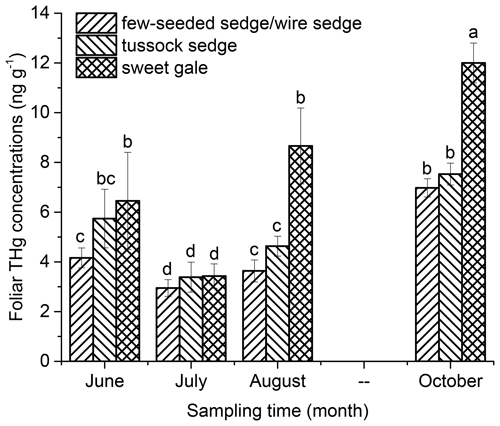
Figure 1The intraseasonal trend in foliar total mercury (THg) concentrations (ng g−1) of few-seeded sedge/wire sedge, tussock sedge, and sweet gale (ng g−1). All concentrations are expressed in dry weight. Error bars are ± SD (n=5 for each species for each time interval). The same letters above bars denote that values of foliar THg concentrations are not significantly different at the 0.05 levels.
Mercury accumulation in leaves is affected by many factors, such as atmospheric Hg concentration, environmental conditions (e.g., solar radiation and temperature), and biological factors (e.g., leaf age, plant species, leaf area, and leaf placement) (Blackwell and Driscoll, 2015; Ericksen et al., 2003; Ericksen and Gustin, 2004; Laacouri et al., 2013). Since all samples were collected in the same location, factors such as atmospheric Hg concentration and environmental conditions were deemed the same, leaving only biological factors as an explanation for differences.
Leaf age. Leaf age is an important biological factor in controlling foliar concentrations (Ericksen et al., 2003; Laacouri et al., 2013). The positive relationship between foliar THg concentrations and time after July suggests that leaves of all species here continued to assimilate atmospheric Hg over the growing season right up to senescence. Some studies have found that the rate of foliar Hg uptake decreased toward the end of the growing season (Ericksen et al., 2003; Laacouri et al., 2013; Poissant et al., 2008), which appears to be because of the decrease in photosynthetic activity at the end of the growing season. In this study, foliar Hg concentrations continue to increase right up to senescence. Although foliar Hg can transport to other plant organs, such as tree rings (Arnold et al., 2018; McLagan et al., 2022), and/or can be re-emitted into the atmosphere (Zheng et al., 2016; Yu et al., 2016; Yuan et al., 2019), the majority of foliar Hg by mass is generally incorporated into leaf tissue (Laacouri et al., 2013; Lodenius et al., 2003; Stamenkovic and Gustin, 2009). In addition, it is likely that less than 10 % of Hg in roots was transported to the leaves (Ericksen et al., 2003; Mao et al., 2013).
Plant species. Plant photosynthesis, transpiration, growth rates, and leaf area are different among plant species (Antúnez et al., 2001; Laacouri et al., 2013), and given that these are important controls on Hg accumulation, the differences among species found in this study are not surprising. The mean foliar THg concentrations in tussock sedge were 1.2 times higher than that in few-seeded sedge/wire sedge, and although not measured as part of this study, tussock sedge has a larger leaf area than few-seeded sedge/wire sedge (Newmaster et al., 1997). A larger leaf has a higher density of stomata and thus more leaf accumulation of atmospheric Hg (Laacouri et al., 2013; Stamenkovic and Gustin, 2009). A larger leaf area may also provide more adsorption sites for non-stomatal Hg uptake. Increased biomass corresponding with a bigger leaf area can offset the effects of stomate number on atmospheric Hg accumulation by leaves to a certain degree. A plausible explanation is that leaf biomass does not proportionally increase with leaf area and stomata, leading to a higher absolute Hg concentration in tussock sedge leaves than in few-seeded sedge/wire sedge leaves. Kozlowski and Pallardy (1997) reported that leaves near the top of the canopy generally have higher rates of photosynthesis and stomatal conductance than those near the bottom of the canopy due to light saturation. Sweet gale had potentially higher stomatal conductance due to higher incident radiation and vapor pressure deficits than sedges that are lower to the saturated ground with tightly packed vertical leaves.
Concentrations of Hg in senesced leaves of few-seeded sedge/wire sedge, tussock sedge, and sweet gale (6.58–12.77 ng g−1) were lower than that reported in tree litter (21–78 ng g−1) in North America and Europe (Laacouri et al., 2013; Obrist et al., 2021; Poissant et al., 2008; Rea et al., 2002; Wang et al., 2016) but similar to that previously reported for grasses and herbaceous plants (∼ 10 ng g−1) (Moore et al., 1995). The foliar Hg concentrations for plant species in this study increased 1.3–2.0 times over the growing season, which was a smaller increase than that (3–11 fold) reported for trees (Laacouri et al., 2013; Poissant et al., 2008; Rea et al., 2002). The above results further confirm that foliar Hg concentrations differ among vegetation types (Demers et al., 2007; Moore et al., 1995; Obrist et al., 2012; Richardson and Friedland, 2015). It has been suggested that Hg previously retained in leaves can be photo-reduced to Hg0 that is re-emitted to the atmosphere, and consistent Hg0 re-emission from the foliage is positively related to photosynthetically active radiation (PAR) (Yuan et al., 2019). The plants in open boreal peatlands lacking a tree overstorey like that in this study would receive very high exposure to ultraviolet (UV) light, which may result in a greater photoreduction of Hg previously retained in leaves – and then Hg loss – than tree leaves that are more often shaded. Moreover, despite angiosperms having higher stomatal conductance due to fewer stomata but more numbers (de Boer et al., 2016; Jordan et al., 2015), stomatal opening in dark-adapted leaves after light exposure was generally faster in gymnosperms than in angiosperms, but stomatal closing upon the darkness of light-adapted leaves was faster in angiosperms than in gymnosperms (Xiong et al., 2018). This phenomenon may lead to a higher Hg concentration in trees (a type of gymnosperm) than in sedges and sweet gales (two types of angiosperm). More studies are needed to elucidate this mechanism of foliar Hg accumulation by different plant types.
Leaf carbon, nitrogen, and mercury. Leaf % C, % N, and C : N were significantly different among plant species (, p<0.001) over the growing season (, p<0.001) (Fig. 2). Based on post hoc tests, foliar % C, % N, and C : N were significantly different between sweet gale and sedges (few-seeded sedge/wire sedge and tussock sedge) but not between few-seeded sedge/wire sedge and tussock sedge. Foliar % C and % N were much lower in these sedges than sweet gale, which agrees well with a previous study that deciduous shrubs (i.e., sweet gale) generally have a higher foliar % C and % N than grasses (Wright et al., 2004). The fixation of nitrogen in sweet gale is in part attributed to sweet gale root nodules containing symbiotic nitrogen fixing (Newmaster et al., 1997; Vitousek et al., 2002), with this greater amount of available N leading to higher photosynthetic capacity (Wright et al., 2004); thus, species containing a higher foliar % N are usually accompanied by a higher % C.
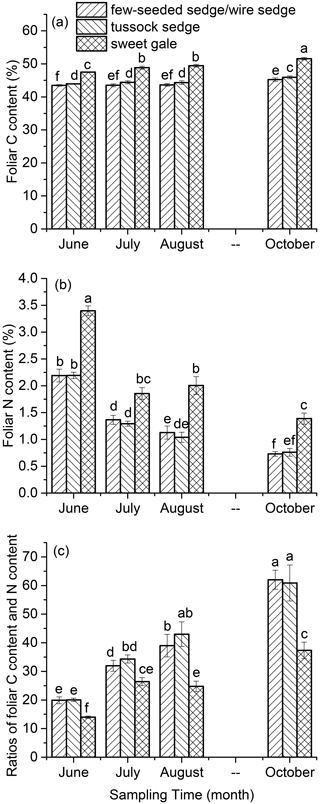
Figure 2The carbon content (% C) (a), nitrogen content (% N) (b), and the ratio of carbon content to nitrogen content (C : N) (c) over the 2018 growing season. Vertical bars are mean ± SD (n=5). The same letters above bars denote that values of foliar THg concentrations are not significantly different at the 0.05 levels.
There were significant increases in foliar % C (few-seeded sedge/wire sedge: , p<0.001; tussock sedge: , p<0.001; sweet gale: , p<0.001) but sharp decreases in foliar % N (few-seeded sedge/wire sedge: , p<0.001; tussock sedge: , p<0.001; sweet gale: , p<0.001) over the growing season (Fig. 2). The strong decreases in foliar % N with leaf age can be attributed to the translocation of N from senescing leaves to new leaves (Wang et al., 2003). A study found that approximately 77 % N, 57 % phosphorus (P), and 44 % potassium (K) were translocated out of senescing leaves during mangrove leaf senescence (Wang et al., 2003). Foliar C is sequestrating continuously over the growing season (Kueh et al., 2013). The element re-translocation and C sequestration in leaves may lead to the foliar % C increase with time. The values of foliar C : N increased with time, which is a function of the decreases in foliar % N and the increases in foliar % C.
Senesced leaf tissue with higher foliar % C and % N had higher foliar THg concentrations (% C and Hg: , p<0.05, , R2=0.94; % N and Hg: , p<0.05, , R2=0.93) (Fig. 3a and b). THg concentrations were negatively related to foliar C : N during senescence (, p<0.05, , R2=0.86; Fig. 3c). A previous study found soil Hg concentrations were positively related to soil organic C and N, giving a possible explanation that high C and N levels in soil reflect high vegetation productivity corresponding with high atmospheric Hg deposition via litterfall (Obrist et al., 2009). Although the mechanism of these relationships between Hg concentrations and contents of C and N in senesced leaf materials is still unclear, this study shows that Hg input via litterfall to soils can be affected by C and N content in senesced leaves. More studies and data are needed to draw predictive conclusions.
4.2 Mercury leaching from senesced leaves
Surface-rinsable mercury. The mean mass of Hg from the surface rinse of senesced leaf material (expressed per gram of dry senesced leaves) was 0.02 ± 0.01 and 0.01 ± 0.00 ng g−1(or 3.27 ± 1.68 and 1.39 ± 0.83 ng L−1, expressed per liter of rinse water (18.2 MΩ cm)), respectively, indicating that the mass of Hg that was loosely bound on the leaf surface was small relative to the total senesced leaf tissue Hg concentration (8.83 ± 2.38 ng g−1) representing on average only 0.4 % Hg (tussock sedge: 0.6 %; few-seeded sedge/wire sedge: 0.3 %; sweet gale: 0.3 %) of total THg mass.
Leachable mercury. The mean THgaq mass per gram of senesced leaves had significant differences between plant species (, p<0.001; Fig. 4). Based on post hoc tests, there were significant differences in THgaq mass per gram of senesced leaves between sweet gale and sedges (few-seeded sedge/wire sedge and tussock sedge) but not between few-seeded sedge/wire sedge and tussock sedge. The senesced leaves of sweet gale leached the least Hg among these plant species, which is likely due to their hydrophobic waxy cuticle that may both retain Hg and protect the inner leaf material from leaching. Another plausible explanation is that N was more easily released from sedges than C and it was the opposite for sweet gale, based on changes in foliar % C and % N between before and after leaching (Table 1), whereas N groups in litter hinder the leaching of foliar Hg (Obrist et al., 2009). Foliar % N of sweet gale increased after leaching, which is likely attributed to a large amount of loss of other elements, such as K, Mg, and P, although they were not part of this experiment. Bessaad and Korboulewsky (2020) found that 60 %–79 % of K, 19 %–50 % of Mg, 22 %–30 % of P, and < 16 % of Ca and N were leached out from fully developed broadleaves (collected in summer) during rainfall.
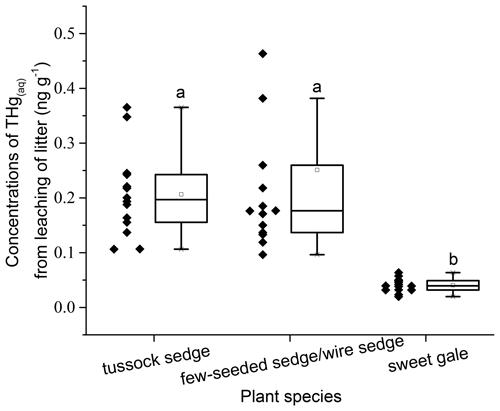
Figure 4Mass of mercury leached per gram of senesced leaf material (ng g−1). Boxplot displays median (50th percentile; the inside line of the box), first quartile (25th percentile; lower bound of the box), third quartile (75th percentile; upper bound of the box), whiskers (all measures between 5th percentile and 25th percentile and between 75th percentile and 95th percentile; the straight line below and above the box), and outliers (individual points outside of the 5th and 95th percentiles). n=15.
Table 1Changes in foliar carbon content (% C) and nitrogen content (% N) during leaching of litterfall. n=15.
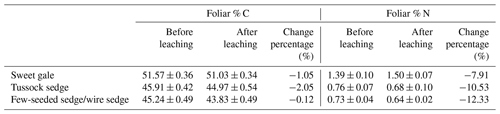
During experimental leaching, 3.0 %, 2.9 %, and 0.3 % of the total THg mass present in tussock sedge, few-seeded sedge/wire sedge, and sweet gale senesced leaves were leached, respectively. The percentages of Hg that leached from tussock sedge and few-seeded sedge/wire sedge leaves were 5.5 and 10.6 times higher than that from rinses, while the percentage of Hg that leached from sweet gale senesced leaves was similar to that from rinse water (0.3 %). Rea et al. (2000) reported that surface washoff of loosely bound and particulate Hg was a rapid and larger source of Hg in forest throughfall compared to continuously foliar Hg leaching from live leaves. It is likely because dry leaves lack structural integrity compared to live leaves in Rea et al.'s (2000) study, leading to more rapid leaching of soluble constituents (Gessner et al., 1999), including Hg, so the results of these prior studies are not directly comparable to this one. Further, although Hg leached from senesced leaf material was a small (< 5 % of foliar tissue Hg) but a measurable contributor to the mass balance, it is one that would be completely missed if material had been collected from a litter trap that had been exposed to rainfall for any period.
4.3 The roles of dissolved organic matter properties in Hg mobility during litter leaching phase
The quantity and characteristics of DOM in leachate. The mean mass of DOC leached per gram of senesced leaf material and the mass loss during senesced leaf material leaching was significantly different between plant species (leached DOC mass: , p<0.001; mass loss: , p<0.05) with the same sequence as follows: few-seeded sedge/wire sedge < tussock sedge < sweet gale (Fig. 5). The same sequence is in part because the loss of soluble carbons accounted for the majority of the mass loss during litter leaching (Del Giudice and Lindo, 2017). Mass loss of sweet gale (17.7 %) was significantly larger than sedges (few-seeded sedge/wire sedge (8.1 %) and tussock sedge (11.5 %)). The released DOC accounted for 22.96 ± 14.85 %, 23.73 ± 12.95 %, and 17.03 ± 6.68 % of mass loss during senesced leaf material leaching for few-seeded sedge/wire sedge, tussock sedge, and sweet gale, respectively. Loss of other nutrients, such as dissolved organic nitrogen (DON) and dissolved organic phosphorus (DOP) (Ong et al., 2017; Liu et al., 2018; Hensgens et al., 2020), as well as the inorganic components and other elemental organic matter (Lavery et al., 2013; Jiménez et al., 2017), also contributes to the mass loss, despite these nutrients not being measured.
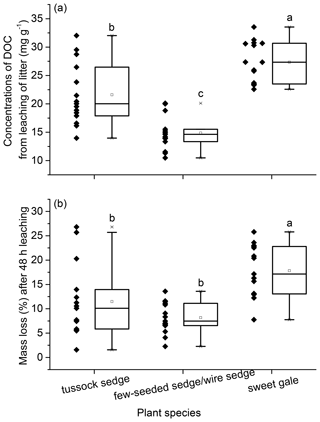
Figure 5Mass of dissolved organic carbon (DOC) leached per gram of senesced leaf material (mg g−1) (a) and mass loss after 48 h leaching (b). Boxplot displays median (50th percentile; the inside line of the box), first quartile (25th percentile; lower bound of the box), third quartile (75th percentile; upper bound of the box), whiskers (all measures between 5th percentile and 25th percentile and between 75th percentile and 95th percentile; the straight line below and above the box), and outliers (individual points outside of the 5th and 95th percentiles). n=15.
Characteristics of DOM also varied among plant species (SUVA254: , p<0.001; FI: , p<0.001; HIXEM: , p<0.05; BIX: , p<0.001) (Fig. 6 and Table 2). Based on post hoc tests, there were significant differences in SUVA254 and FI between sweet gale and sedges (few-seeded sedge/wire sedge) only and in BIX among all plant species. FI (1.2–1.8) and BIX (< 1.0) reflected that DOM in leachate was generally of plant origin, suggesting that the microbially derived OM was a smaller component. The mean value of SUVA254 in leachate followed the sequence tussock sedge ≈ few-seeded sedge/wire sedge > sweet gale leaves, indicating that leached DOM from tussock sedge and few-seeded sedge/wire sedge leaves had higher aromaticity and higher molecular weights than that from the sweet gale leaves. SUVA254 was negatively related to DOM concentrations (, p<0.001, , R2=0.53) when all plant species were considered, suggesting that sweet gale prefers to release a greater amount of lower aromatic DOM.
Previous studies have found that characteristics of DOM controlled Hg mobility and methylation (Cui et al., 2022; Jiang et al., 2018; Ravichandran 2004; Xin et al., 2022; Wang et al., 2022). Hg is tightly and readily bound to reduced sulfur groups (i.e., thiols) in DOM (Ravichandran, 2004; Xia et al., 1999). Mercury weakly binds to carboxyl and phenol functional groups in DOM after all thiol groups are occupied at relatively high Hg concentrations (Drexel et al., 2002; Graham et al., 2012), which is atypical in most natural environments in which Hg concentrations are relatively low. Higher terrestrial (plant-derived) DOM had a greater DOM–Hg affinity (Wang et al., 2022). Additionally, DOM with higher aromaticity and molecular weight strongly bonded with Hg(II), potentially because these types of DOM provide more sulfidic groups such as thiols (Dittman et al., 2009; Wang et al., 2022). Therefore, terrestrial DOM and/or DOM with higher aromaticity and molecular weight may transport more Hg into peat soils during the litter leaching phase.
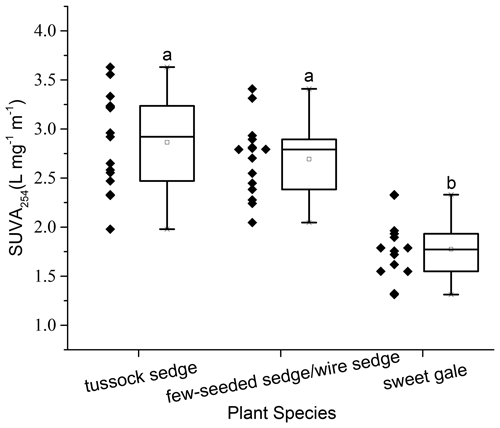
Figure 6Dissolved organic matter characteristics as measured by specific ultraviolet absorbance at the wavelength of 254 nm (SUVA254), n=15. Boxplot displays median (50th percentile; the inside line of the box), first quartile (25th percentile; lower bound of the box), third quartile (75th percentile; upper bound of the box), whiskers (all measures between 5th percentile and 25th percentile and between 75th percentile and 95th percentile; the straight line below and above the box), and outliers (individual points outside of the 5th and 95th percentiles).
Table 2The mean fluorescence indices of dissolved organic matter characteristics*.
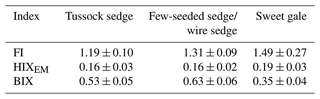
* Lower values of the FI (< 1.2) suggest dissolved organic matter (DOM) has higher aromaticity and is primarily composed of high-molecular-weight DOM, while high FI values (> 1.8) indicate that DOM has lower aromaticity and is mainly composed of low-molecular-weight DOM. DOM with high HIXEM (> 1) values is composed of more highly condensed and higher-molecular-weight molecules. In contrast, higher BIX (> 1.0) values reflect that more low-molecular-weight DOM was recently produced, generally, by microbes. All indices are unitless, n=15.
Table 3Annual input of senesced leaves and senesced leaf Hg, surficial Hg, and leached Hg during leaching into peat soils per hectare and per year in the sedge-dominated fen (mg ha−1 yr−1).
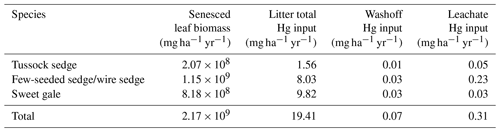
Correlation between THgaq concentrations and SUVA254 values in leachate. The concentrations of soluble THgaq were significantly related to SUVA254 values (, p<0.001, , R2=0.55; Fig. 7). This result suggested that DOM with higher aromaticity plays an important role in controlling Hg mobility (Ravichandran, 2004). The value of R2 was only 0.55, which can be attributed to the fact that the number of reduced sulfur groups in DOM far exceeds the amount of Hg in natural environments and other factors, such as pH and sulfide maybe affecting the binding between DOM and Hg (Ravichandran, 2004). In this study, DOM with higher aromaticity may transport more Hg from litter to soils, and senesced leaves of sedges had a higher potential in leaching Hg into peatland soils than the senesced leaves of sweet gales in this study.
4.4 Estimation of annual input of Hg via senesced leaves and rapid Leaching to peat soils
The annual input of leaf biomass (mg ha−1 yr−1) of few-seeded sedge/wire sedge into peat soils was 5.55 and 1.41 fold higher than tussock sedge and sweet gale, while the annual inputs of Hg (mg ha−1 yr−1) via sweet gale leaves were 6.29 and 1.22 fold higher than via tussock sedge and few-seeded/wire sedge leaves in the sedge-dominated fen (Table 3). Annual total Hg inputs through senesced leaves to peat soils were 1.56, 8.03, and 9.82 mg ha−1 yr−1 for tussock sedge, few-seeded sedge/wire sedge, and sweet gale, respectively. The input of surficial Hg and leachable Hg accounted for 0.64 % and 0.37 %, 0.31 % and 3.20 %, and 2.86 % and 0.30 % of total foliar Hg input to peat soils annually for tussock sedge, few-seeded sedge/wire sedge, and sweet gale, respectively. The majority of Hg in senesced leaves (> 96 %) was from the deposition of solid plant tissues in litter.
Based on the data from the study growing season, the annual total input of Hg via senesced leaves (19.40 mg ha−1 yr−1) was 5 %–22 % of litterfall in forest ecosystems (e.g., jack pine, black spruce, and balsam fir forest; red maple and birch forest; Norway spruce forest; 86–372 mg ha−1 yr−1) (St Louis et al., 2001; Graydon et al., 2008; Shanley and Bishop, 2012), which can be attributed to those forest ecosystems having both higher mean foliar Hg concentrations (21–51 ng g−1) (Zhou and Obrist, 2021) and much greater aboveground biomass and litterfall inputs (2000–3488 kg ha−1 yr−1) (Graydon et al., 2008) than plants in this study. The overall annual Hg inputs via these senesced leaves to peat soils in this sedge-dominated fen were 59 % of that via wet deposition using the mean precipitation Hg input estimates from the Experimental Lakes Area (33 mg ha−1 yr−1) for the years 2001–2010, which is in the same general geographic region of Ontario (St Louis et al., 2019).
This study shows that the widely observed pattern of foliar Hg accumulation from the atmosphere and changes in foliar Hg concentrations over time are the same in peatland vascular plants as they are for forest trees and that the patterns are related to time/leaf age and plant species. The THg concentrations in senesced leaves in this study are relatively lower than that in the forest litterfall. Hg released from ubiquitous sedge litter during leaching is relatively quicker than the much slower release of tissue-associated Hg through the decomposition of plant tissues. Thus, the supply of inorganic Hg to sites of methylation in peatlands has both fast and slow pathways that may shift under climate change, given that peatland plant species composition and biomass will certainly change under climate change.
Hg | Mercury |
MeHg | Methylmercury |
GEM | Gaseous elemental mercury |
RGM | Reactive gaseous mercury |
PBM | Particulate-bound mercury |
THg | Total mercury |
THgaq | Dissolved total mercury |
% C | Carbon content |
% N | Nitrogen content |
C : N | The ratio of leaf C content and N content |
DOM | Dissolved organic matter |
DOC | Dissolved organic carbon |
SUVA254 | Specific ultraviolet absorbance at awavelength of 254 nm |
EEMs | Fluorescence excitation–emission matrices |
FI | Fluorescence index |
HIXEM | Humification index |
BIX | Biological index soil organic matter (SOM) |
CRM | Certified reference material |
RSD | Relative standard deviation |
All data generated or analyzed during this study are included in this published article and its Supplement.
The supplement related to this article is available online at: https://doi.org/10.5194/bg-20-2971-2023-supplement.
TS carried the project out, collected all samples, performed the leaching experiment, analyzed samples and data, and wrote the manuscript. BAB designed the experiments, provided supervision, and edited the manuscript.
The contact author has declared that neither of the authors has any competing interests.
Publisher’s note: Copernicus Publications remains neutral with regard to jurisdictional claims in published maps and institutional affiliations.
The research is supported by the Natural Sciences and Engineering Research Council of Canada (NSERC) Strategic Partnership grant (STPGP/479026-2015). We thank all members of Brian A. Branfireun and Zoë Lindo's lab group for their help in the field.
This research has been supported by the Natural Sciences and Engineering Research Council of Canada (grant no. STPGP/479026-2015).
This paper was edited by Jianming Xu and reviewed by two anonymous referees.
Antúnez, I., Retamosa, E. C., and Villar, R.: Relative growth rate in phylogenetically related deciduous and evergreen woody species, Oecologia, 128, 172–180, https://doi.org/10.1007/s004420100645, 2001.
Arnold, J., Gustin, M. S., and Weisberg, P. J.: Evidence for nonstomatal uptake of Hg by aspen and translocation of Hg from foliage to tree rings in Austrian pine, Environ. Sci. Technol., 52, 1174–1182, https://doi.org/10.1021/acs.est.7b04468, 2018.
Bessaad, A. and Korboulewsky, N.: How much does leaf leaching matter during the pre-drying period in a whole-tree harvesting system?, Forest Ecol. Manag., 477, 118492, https://doi.org/10.1016/j.foreco.2020.118492, 2020.
Blackwell, B. D. and Driscoll, C. T.: Deposition of mercury in forests along a montane elevation gradient, Environ. Sci. Technol., 49, 5363–5370, https://doi.org/10.1021/es505928w, 2015.
Blodau, C., Mayer, B., Peiffer, S., Moore, T. R.: Support for an anaerobic sulfur cycle in two Canadian peatland soils, J. Geophy. Res.-Biogeo., 112, G02004, https://doi.org/10.1029/2006JG000364, 2007.
Brahmstedt, E. S., Crespo, C. N. A., Holsen, T. M., and Twiss, M. R.: Mercury distribution in an Upper St. Lawrence River wetland dominated by cattail (Typha angustifolia), Wetlands, 41, 119, https://doi.org/10.1007/s13157-021-01511-9, 2021.
Branfireun, B. A., Heyes, A., and Roulet, N. T.: The hydrology and methylmercury dynamics of a Precambrian Shield headwater peatland, Water Resour. Res., 32, 1785–1794, https://doi.org/10.1029/96WR00790, 1996.
Branfireun, B. A., Krabbenhoft, D. P., Hintelmann, H., Hunt, R. J., Hurley, J. P., and Rudd, J. W. M.: Speciation and transport of newly deposited mercury in a boreal forest wetland: A stable mercury isotope approach, Water Resour. Res., 41, W06016, https://doi.org/10.1029/2004WR003219, 2005.
Buttler, A., Robroek, B. J., Laggoun-Défarge, F., Jassey, V. E., Pochelon, C., Bernard, G., Delarue, F., Gogo, S., Mariotte, P., and Mitchell, E. A. D.: Experimental warming interacts with soil moisture to discriminate plant responses in an ombrotrophic peatland, J. Veg. Sci., 26, 964–974, https://doi.org/10.1111/jvs.12296, 2015.
Cabrita, M. T., Duarte, B., Cesario, R., Mendes, R., Hintelmann, H., Eckey, K., Dimock, B., Cacador, I., and Canário, J.: Mercury mobility and effects in the salt-marsh plant Halimione portulacoides: Uptake, transport, and toxicity and tolerance mechanisms, Sci. Total Environ., 650, 111–120, https://doi.org/10.1016/j.scitotenv.2018.08.335, 2019.
Canário, J., Poissant, L., Pilote, M., Caetano, M., Hintelmann, H., and O'Driscoll, N. J.: Salt-marsh plants as potential sources of Hg-0 into the atmosphere, Atmos. Environ., 152, 458–464, https://doi.org/10.1016/j.atmosenv.2017.01.011, 2017.
Cui, H. Y., Zhao, Y., Zhao., L., and Wei, Z. M.: Characterization of mercury binding to different molecular weight fractions of dissolved organic matter, J. Hazard. Mater., 431, 128593, https://doi.org/10.1016/j.jhazmat.2022.128593, 2022.
de Boer, H. J., Price, C. A., Wagner-Cremer, F., Dekker, S. C., Franks, P. J., and Veneklaas, E. J.: Optimal allocation of leaf epidermal area for gas exchange, New Phytol., 210, 1219–1228, https://doi.org/10.1111/nph.13929, 2016.
Demers, J. D., Driscoll, C. T., Fahey, T. J., and Yavitt, J. B.: Mercury cycling in litter and soil in different forest types in the Adirondack region, New York, USA, Ecol. Appl., 17, 1341–1351, https://doi.org/10.1890/06-1697.1, 2007.
Del Giudice, R. and Lindo, Z.: Short-term leaching dynamics of three peatland plant species reveals how shifts in plant communities may affect decomposition processes, Geoderma, 285, 110–116, https://doi.org/10.1016/j.geoderma.2016.09.028, 2017.
Dieleman, C. M., Branfireun, B. A., McLaughlin, J. W., and Lindo, Z.: Climate change drives a shift in peatland ecosystem plant community: implications for ecosystem function and stability, Glob. Change Biol., 21, 388–395, https://doi.org/10.1111/gcb.12643, 2015.
Dittman, J. A., Shanley, J. B., Driscoll, C. T., Aiken, G. R., Chalmers, A. T., and Towse, J. E.: Ultraviolet absorbance as a proxy for total dissolved mercury in streams, Environ. Pollut., 157, 1953–1956, https://doi.org/10.1016/j.envpol.2009.01.031, 2009.
Drexel, R. T., Haitzer, M., Ryan, J. N., Aiken, G. R., and Nagy, K. L.: Mercury (II) sorption to two Florida Everglades peats: evidence for strong and weak binding and competition by dissolved organic matter released from the peat, Environ. Sci. Technol., 36, 4058–4064, https://doi.org/10.1021/es0114005, 2002.
Enrico, M., Le Roux, G., Marusczak, N., Heimburger, L. E., Claustres, A., Fu, X. W., Sun, R. Y., and Sonke, J. E.: Atmospheric mercury transfer to peat bogs dominated by gaseous elemental mercury dry deposition, Environ. Sci. Technol., 50, 2405–2412, https://doi.org/10.1021/acs.est.5b06058, 2016.
Ericksen, J., Gustin, M., Schorran, D., Johnson, D., Lindberg, S., and Coleman, J.: Accumulation of atmospheric mercury in forest foliage, Atmos. Environ., 37, 1613–1622, https://doi.org/10.1016/S1352-2310(03)00008-6, 2003.
Ericksen, J. A. and Gustin, M.: Foliar exchange of mercury as a function of soil and air mercury concentrations, Sci. Total Environ., 324, 271–279, https://doi.org/10.1016/j.scitotenv.2003.10.034, 2004.
Fay, L. and Gustin, M. S.: Investigation of mercury accumulation in cattails growing in constructed wetland mesocosms, Wetlands, 27, 1056–1065, https://doi.org/10.1672/0277-5212(2007)27[1056:IOMAIC]2.0.CO;2, 2007.
Fellman, J. B., Hood, E., and Spencer, R. G.: Fluorescence spectroscopy opens new windows into dissolved organic matter dynamics in freshwater ecosystems: A review, Limnol. Oceanogr., 55, 2452–2462, https://doi.org/10.4319/lo.2010.55.6.2452, 2010.
Frolking, S., Roulet, N. T., Moore, T. R., Richard, P. J., Lavoie, M., and Muller, S. D.: Modeling northern peatland decomposition and peat accumulation, Ecosystems 4, 479–498, https://doi.org/10.1007/s10021-001-0105-1, 2001.
Gessner, M. O., Chauvet, E., and Dobson, M.: A perspective on leaf litter breakdown in streams, Oikos 85, 377–384, https://doi.org/10.2307/3546505, 1999.
Gorham, E.: Northern peatlands: Role in the carbon cycle and probable responses to climatic warming, Ecol. Appl., 1, 182–195, https://doi.org/10.2307/1941811, 1991.
Graham, A. M., Aiken, G. R., and Gilmour, C. C.: Dissolved organic matter enhances microbial mercury methylation under sulfidic conditions, Environ. Sci. Technol., 46, 2715–2723, https://doi.org/10.1021/es203658f, 2012.
Graydon, J. A., St Louis, V. L., Hintelmann, H., Lindberg, S. E., Sandilands, K. A., Rudd, J. W., Kelly, C. A., Hall, B. D., and Mowat, L. D.: Long-term wet and dry deposition of total and methyl mercury in the remote boreal ecoregion of Canada, Environ. Sci. Technol., 42, 8345–8351, https://doi.org/10.1021/es801056j, 2008.
Grigal, D. F.: Mercury sequestration in forests and peatlands: A review, J. Environ. Qual., 32, 393–405, https://doi.org/10.2134/jeq2003.0393, 2003.
Haitzer, M., Aiken, G. R., and Ryan, J. N.: Binding of mercury (II) to dissolved organic matter: The role of the mercury-to-DOM concentration ratio, Environ. Sci. Technol., 36, 3564–3570, https://doi.org/10.1021/es025699i, 2002.
Hensgens, G., Laudon, H., Peichl, M., Gil, I. A., Zhou, Q., and Berggren, M.: The role of the understory in litter DOC and nutrient leaching in boreal forests, Biogeochemistry, 149, 87–103, https://doi.org/10.1007/s10533-020-00668-5, 2020.
Hintelmann, H., Harris, R., Heyes, A., Hurley, J. P., Kelly, C. A., Krabbenhoft, D. P., Lindberg, S., Rudd, J. W., Scott, K. J., and St Louis, V. L.: Reactivity and mobility of new and old mercury deposition in a boreal forest ecosystem during the first year of the METAALICUS study, Environ. Sci. Technol., 36, 5034–5040, https://doi.org/10.1021/es025572t, 2002.
Hobbie, S. E.: Temperature and plant species control over litter decomposition in Alaskan tundra, Ecol. Monogr., 66, 503–522, https://doi.org/10.2307/2963492, 1996.
Huguet, A., Vacher, L., Relexans, S., Saubusse, S., Froidefond, J. M., and Parlanti, E.: Properties of fluorescent dissolved organic matter in the Gironde Estuary, Org. Geochem., 40, 706–719, https://doi.org/10.1016/j.orggeochem.2009.03.002, 2009.
IPCC: Summary for policymakers, in: Global warming of 1.5 ∘C, an IPCC special report on the impacts of global warming of 1.5 ∘C above pre-industrial levels and related global greenhouse gas emission pathways, in the context of strengthening the global response to the threat of climate change, sustainable development, and efforts to eradicate poverty, edited by: Masson-Delmotte, V. P., Zhai, H.-O., Portner, D., Roberts, J., Skea, P. R., Shukla, A., et al., Geneva, Switzerland: World Meteorological Organization, 32, 2018.
Jiang, T., Bravo, A. G., Skyllberg, U., Bjorn, E., Wang, D. Y., Yan, H. Y., and Green, N. W.: Influence of dissolved organic matter (DOM) characteristics on dissolved mercury (Hg) species composition in sediment porewater of lakes from southwest China, Water Res., 146, 146–158, https://doi.org/10.1016/j.watres.2018.08.054, 2018.
Jiménez, M. A., Beltran, R., Traveset, A., Calleja, M. L., Delgado-Huertas, A., and Marba, N.: Aeolian transport of seagrass (Posidonia oceanica) beach-cast to terrestrial systems, Estuar. Coast. Shelf S., 196, 31–44, https://doi.org/10.1016/j.ecss.2017.06.035, 2017.
Jiskra, M., Sonke, J. E., Obrist, D., Bieser, J., Ebinghaus, R., Myhre, C. L., Pfaffhuber, K. A., Wängberg, I., Kyllönen, K., and Worthy, D.: A vegetation control on seasonal variations in global atmospheric mercury concentrations, Nat. Geosc., 11, 244–250, https://doi.org/10.1038/s41561-018-0078-8, 2018.
Jordan, G. J., Carpenter, R. J., Koutoulis, A., Price, A., and Brodribb, T. J.: Environmental adaptation in stomatal size independent of the effects of genome size, New Phytol., 205, 608–617, https://doi.org/10.1111/nph.13076, 2015.
Kneer, M. L., White, A., Rolfhus, K. R., Jeremiason, J. D., Johnson, N. W., and Ginder-Vogel, M.: Impact of dissolved organic matter on porewater Hg and MeHg concentrations in St Louis River estuary sediments, ACS Earch Space Chem., 4, 1386–1397, https://doi.org/10.1021/acsearthspacechem.0c00134, 2020.
Kozlowski, T. T. and Pallardy, S. G.: Physiology of Woody Plants, Academic Press, New York, 1997.
Kueh, J. H. R., Ab Majid, N. M., Seca, G., and Ahmed, O. H.: Above ground biomass-carbon partitioning, storage and sequestration in a Rehabilitated forest, Bintulu, Sarawak, Malaysia, Sains Malays., 42, 1041–1050, 2013.
Laacouri, A., Nater, E. A., and Kolka, R. K.: Distribution and uptake dynamics of mercury in leaves of common deciduous tree species in Minnesota, USA, Environ. Sci. Technol., 47, 10462–10470, https://doi.org/10.1021/es401357z, 2013.
Lavery, P. S., McMahon, K., Weyers, J., Boyce, M. C., and Oldham, C. E.: Release of dissolved organic carbon from seagrass wrack and its implications for trophic connectivity, Mar. Ecol.-Prog. Ser., 494, 121–133, https://doi.org/10.3354/meps10554, 2013.
Lindberg, S., Bullock, R., Ebinghaus, R., Engstrom, D., Feng, X., Fitzgerald, W., Pirrone, N., Prestbo, E., and Seigneur, C.: A synthesis of progress and uncertainties in attributing the sources of mercury in deposition, AMBIO, 36, 19–33, https://doi.org/10.1579/0044-7447(2007)36[19:ASOPAU]2.0.CO;2, 2007.
Lindberg, S. E., Meyers, T. P., Taylor, G. E., Turner, R. R., and Schroeder, W. H.: Atmosphere surface exchange of mercury in a forest: results of modeling and gradient approaches, J. Geophys. Res.-Atmos., 97, 2519–2528, https://doi.org/10.1029/91JD02831, 1992.
Liu, S. L., Jiang, Z. J., Zhou, C. Y., Wu, Y. C., Arbi, I., Zhang, J. P., and Huang, X. P., and Trevathan-Tackett, S. M.: Leaching of dissolved organic matter from seagrass leaf litter and its biogeochemical implications, Acta Oceanol. Sin., 37, 84–90, https://doi.org/10.1007/s13131-018-1233-1, 2018.
Lodenius, M., Tulisalo, E., and Soltanpour-Gargari, A.: Exchange of mercury between atmosphere and vegetation under contaminated conditions, Sci. Total Environ., 304, 169–174, https://doi.org/10.1016/S0048-9697(02)00566-1, 2003.
Lyons, C. L. and Lindon, Z.: Above-and belowground community linkages in boreal peatlands, Plant Ecol., 221, 615–632, https://doi.org/10.1007/s11258-020-01037-w, 2019.
Mao, Y. X., Li, Y. B., Richards, J., and Cai, Y.: Investigating uptake and translocation of mercury species by Sawgrass (Cladium jamaicense) using a stable isotope tracer technique, Environ. Sci. Technol., 47, 9678–9684, https://doi.org/10.1021/es400546s, 2013.
McKnight, D. M., Boyer, E. W., Westerhoff, P. K., Doran, P. T., Kulbe, T., and Andersen, D. T.: Spectrofluorometric characterization of dissolved organic matter for indication of precursor organic material and aromaticity, Limnol. Oceanogr., 46, 38–48, https://doi.org/10.4319/lo.2001.46.1.0038, 2001.
McLagan, D. S., Biester, H., Navratil, T., Kraemer, S. M., and Schwab, L.: Internal tree cycling and atmospheric archiving of mercury: examination with concentration and stable isotope analyses, Biogeosciences, 19, 4415–4429, https://doi.org/10.5194/bg-19-4415-2022, 2022.
Mitchell, C. P. J., Branfireun, B. A., and Kolka, R. K.: Spatial characteristics of net methylmercury production hot spots in peatlands, Environ. Sci. Technol., 42, 1010–1016, https://doi.org/10.1021/es0704986, 2008.
Moore, T., Bubier, J., Heyes, A., and Flett, R.: Methyl and total mercury in boreal wetland plants, Experimental Lakes Area, Northwestern Ontario, J. Environ. Qual., 24, 845–850, https://doi.org/10.2134/jeq1995.00472425002400050007x, 1995.
Morel, F. M., Kraepiel, A. M., and Amyot, M.: The chemical cycle and bioaccumulation of mercury, Annu. Rev. Ecol. Syst., 29, 543–566, https://doi.org/10.1146/annurev.ecolsys.29.1.543, 1998.
Newmaster, S. G., Harris, A. G., and Kershaw, L. J.: Wetland plants of Ontario, Lone Pine Publishing, Edmonton, Canada, ISBN 10: 1551050595, ISBN 13: 9781551050591, 1997.
Obrist, D., Johnson, D., and Lindberg, S.: Mercury concentrations and pools in four Sierra Nevada forest sites, and relationships to organic carbon and nitrogen, Biogeosciences, 6, 765–777, https://doi.org/10.5194/bg-6-765-2009, 2009.
Obrist, D., Johnson, D. W., and Edmonds, R. L.: Effects of vegetation type on mercury concentrations and pools in two adjacent coniferous and deciduous forests, J. Plant Nutr. Soil Sc., 175, 68–77, https://doi.org/10.1002/jpln.201000415, 2012.
Obrist, D., Agnan, Y., Jiskra, M., Olson, C., Colegrove, D., Hueber, J., Moore, C., Sonke, J., and Helmig, D.: Tundra uptake of atmospheric elemental mercury drives Arctic mercury pollution, Nature, 547, 201–204, https://doi.org/10.1038/nature22997, 2017.
Obrist, D., Roy, E. M., Harrison, J. L., Kwong, C. F., Munger, J. W., Moosmuller, H., Romero, C. D., Sun, S.W., Zhou, J., and Commane, R.: Previously unaccounted atmospheric mercury deposition in a midlatitude deciduous forest, P. Natl. Acad. Sci. USA, 118, e2105477118, https://doi.org/10.1073/pnas.2105477118, 2021.
Olson, C. L., Jiskra, M., Sonke, J. E., and Obrist, D.: Mercury in tudra vegetation of Alaska: Spatial and temporal dynamics and stable isotope patterns, Sci. Total Environ., 660, 1502–1512, https://doi.org/10.1016/j.scitotenv.2019.01.058, 2019.
Palozzi, J. E. and Lindo, Z.: Boreal peat properties link to plant functional traits of ecosystem engineers, Plant Soil, 418, 277–291, https://doi.org/10.1007/s11104-017-3291-0, 2017.
Pech, P., Wojtun, B., Samecka-Cymerman, A., Polechonska, L., and Kempers, A. J.: Metals in plant functional types of ombrotrophic peatlands in the Sudetes (SW Poland), Arch. Environ. Contam. Toxicol., 82, 506–519, https://doi.org/10.1007/s00244-022-00928-5, 2022.
Poissant, L., Pilote, M., Yumvihoze, E., and Lean, D.: Mercury concentrations and foliage/atmosphere fluxes in a maple forest ecosystem in Quebec, Canada, J. Geophys. Res-Atmos., 113, D10307, https://doi.org/10.1029/2007JD009510, 2008.
R Core Team: R: A Language and Environment for Statistical Computing. R Foundation for Statistical Computing, Vienna, Austria, http://www.r-project.org/, 2012.
Ravichandran, M.: Interactions between mercury and dissolved organic matter: A review, Chemosphere, 55, 319–331, https://doi.org/10.1016/j.chemosphere.2003.11.011, 2004.
Rea, A., Lindberg, S., Scherbatskoy, T., and Keeler, G. J.: Mercury accumulation in foliage over time in two northern mixed-hardwood forests, Water Air Soil Poll., 133, 49–67, https://doi.org/10.1023/A:1012919731598, 2002.
Rea, A. W., Lindberg, S. E., and Keeler, G. J.: Assessment of dry deposition and foliar leaching of mercury and selected trace elements based on washed foliar and surrogate surfaces, Environ. Sci. Technol., 34, 2418–2425, https://doi.org/10.1021/es991305k, 2000.
Redjala, T., Zelko, I., Sterckeman, T., Legue, V., and Lux, A.: Relationship between root structure and root cadmium uptake in maize, Environ. Exp. Bot., 71, 241–248, https://doi.org/10.1016/j.envexpbot.2010.12.010, 2011.
Richardson, J. and Friedland, A.: Mercury in coniferous and deciduous upland forests in northern New England, USA: implications of climate change, Biogeosciences, 12, 6737–6749, https://doi.org/10.5194/bg-12-6737-2015, 2015.
Risch, M. R., DeWild, J. F., Krabbenhoft, D. P., Kolka, R. K., and Zhang, L. M.: Litterfall mercury dry deposition in the eastern USA, Environ. Pollut., 161, 284–290, https://doi.org/10.1016/j.envpol.2011.06.005, 2012.
Risch, M. R., DeWild, J. F., Gay, D. A., Zhang, L., Boyer, E. W., and Krabbenhoft, D. P.: Atmospheric mercury deposition to forests in the eastern USA, Environ. Pollut., 228, 8–18, https://doi.org/10.1016/j.envpol.2017.05.004, 2017.
Rydin, H. and Jeglum, J. K.: The biology of peatlands, 2nd Edn., Oxford, United Kingdom, Oxford University Press, https://doi.org/10.1093/acprof:osobl/9780199602995.001.0001, 2013.
Schmalenberger, A., Drake, H. L., and Küsel, K.: High unique diversity of sulfate-reducing prokaryotes characterized in a depth gradient in an acidic fen, Environ. Microbiol., 9, 1317–1328, https://doi.org/10.1111/j.1462-2920.2007.01251.x, 2007.
Schroeder, W. H. and Munthe, J.: Atmospheric mercury: An overview, Atmos. Environ., 32, 809–822, https://doi.org/10.1016/S1352-2310(97)00293-8, 1998.
Shanley, J. B. and Bishop, K.: Mercury cycling in terrestrial watersheds, in: Mercury in the Environment: pattern and process, edited by: Banks, M. S., University of California Press, 119–141, https://doi.org/10.1525/california/9780520271630.003.0008, 2012.
Stamenkovic, J. and Gustin, M. S.: Nonstomatal versus stomatal uptake of atmospheric mercury, Environ. Sci. Technol., 43, 1367–1372, https://doi.org/10.1021/es801583a, 2009.
St Louis, V. L., Rudd, J. W., Kelly, C. A., Beaty, K. G., Bloom, N. S., and Flett, R. J.: Importance of wetlands as sources of methyl mercury to boreal forest ecosystems, Can. J. Fish. Aquat. Sci., 51, 1065–1076, https://doi.org/10.1139/f94-106, 1994.
St Louis, V. L., Rudd, J. W., Kelly, C. A., Hall, B. D., Rolfhus, K. R., Scott, K. J., Lindberg, S. E., and Dong, W.: Importance of the forest canopy to fluxes of methyl mercury and total mercury to boreal ecosystems, Environ. Sci. Technol., 35, 3089–3098, https://doi.org/10.1021/es001924p, 2001.
St Louis, V. L., Graydon, J. A., Lehnherr, I., Amos, H. M., Sunderland, E. M., St. Pierre, K. A., Emmerton, C. A., Sandilands, K., Tate, M., Steffen, A., and Humphreys, E. R.: Atmospheric concentrations and wet/dry loadings of mercury at the remote Experimental Lakes Area, Northwestern Ontario, Canada, Environ. Sci. Technol., 53, 8017–8026, https://doi.org/10.1021/acs.est.9b01338, 2019.
Streets, D. G., Devane, M. K., Lu, Z., Bond, T. C., Sunderland, E. M., and Jacob, D. J.: All-time releases of mercury to the atmosphere from human activities, Environ. Sci. Technol., 45, 10485–10491, https://doi.org/10.1021/es202765m, 2011.
Tian, J., Branfireun, B. A., and Lindo, Z.: Global change alters peatland carbon cycling through plant biomass allocation, Plant Soil, 455, 53–64, https://doi.org/10.1007/s11104-020-04664-4, 2020.
Vitousek, P. M., Cassman, K., Cleveland, C., Crews, T., Field, C. B., Grimm, N. B., Howarth, R. W., Marino, R., Martinelli, L., Rastetter, E. B., and Sprent, J. I.: Towards an ecological understanding of biological nitrogen fixation, Biogeochemistry, 57, 1–45, https://doi.org/10.1023/A:1015798428743, 2002.
Wang, W. Q., Wang, M., and Lin, P.: Seasonal changes in element contents in mangrove element retranslocation during leaf senescene, Plant Soil, 252, 187–193, https://doi.org/10.1023/A:1024704204037, 2003.
Wang, X., Tam, N. F., He, H. D., and Ye, Z. H.: The role of root anatomy, organic acids and iron plaque on mercury accumulation in rice, Plant Soil, 394, 301–313, https://doi.org/10.1007/s11104-015-2537-y, 2015.
Wang, X., Bao, Z., Lin, C. J., Yuan, W., and Feng, X.: Assessment of global mercury deposition through litterfall, Environ. Sci. Technol., 50, 8548–8557, https://doi.org/10.1021/acs.est.5b06351, 2016.
Wang, Y. Q., Liu, J., Van, L. N., Tian, S. Y., Zhang, S. Q., Wang, D. Y., and Jiang, T.: Binding strength of mercury (II) to different dissolved organic matter: The roles of DOM properties and sources, Sci. Total Environ., 807, 150979, https://doi.org/10.1016/j.scitotenv.2021.150979, 2022.
Webster, K. L. and McLaughlin, J. W.: Importance of the water table in controlling dissolved carbon along a fen nutrient gradient, Soil Sci. Soc. Am. J., 74, 2254–2266, https://doi.org/10.2136/sssaj2009.0111, 2010.
Weis, J. S. and Weis, P.: Metal uptake, transport and release by wetland plants: Implications for phytoremediation and restoration, Environ. Int., 30, 685–700, https://doi.org/10.1016/j.envint.2003.11.002, 2004.
Weishaar, J. L., Aiken, G. R., Bergamaschi, B. A., Fram, M. S., Fujii, R., and Mopper, K.: Evaluation of specific ultraviolet absorbance as an indicator of the chemical composition and reactivity of dissolved organic carbon, Environ. Sci. Technol., 37, 4702–4708, https://doi.org/10.1021/es030360x, 2003.
Weltzin, J. F., Pastor, J., Harth, C., Bridgham, S. D., Updegraff, K., and Chapin, C. T.: Response of bog and fen plant communities to warming and water-table manipulations, Ecology, 81, 3464–3478, https://doi.org/10.1890/0012-9658(2000)081[3464:ROBAFP]2.0.CO;2, 2000.
Wright, I. J., Reich, P. B., Westoby, M., Ackerly, D. D., Baruch, Z., Bongers, F., Cavender-Bares, J., Chapin, T., Cornelissen, J. H. C., Diemer, M., Flexas, J., Garnier, E., Groom, P. K., Gulias, J., Hikosaka, K., Lamont, B. B., Lee, T., Lee, W., Lusk, C., Midgley, J. J., Navas, M. L., Niinemets, U., Oleksyn, J., Osada, N., Poorter, H., Poot, P., Prior, L., Pyankov, V. I., Roumet, C., Thomas, S. C., Tjoelker, M. G., Veneklaas, E. J., and Villar, R.: The worldwide leaf economics spectrum, Nature, 428, 821–827, https://doi.org/10.1038/nature02403, 2004.
Xia, K., Skyllberg, U., Bleam, W., Bloom, P., Nater, E., and Helmke, P.: X-ray absorption spectroscopic evidence for the complexation of Hg (II) by reduced sulfur in soil humic substances, Environ. Sci. Technol., 33, 257–261, https://doi.org/10.1021/es980433q, 1999.
Xin, Y., Zhang, X. H., Zheng, D. M., Zhang, Z. S., and Jiang, M.: Impacts of spectral characteristics of dissolved organic matter on methylmercury contents in peatlands, Northeast China, Environ. Geochem. Hlth., 45, 913–923, https://doi.org/10.1007/s10653-022-01257-1, 2022.
Xiong, D. L., Douthe, C., and Flexas, J.: Differential coordination of stomatal conductance, mesophyll conductance, and leaf hydraulic conductance in response to changing light across species, Plant Cell Environ., 41, 436–450, https://doi.org/10.1111/pce.13111, 2018.
Yu, B., Fu, X. W., Yin, R. S., Zhang, H., Wang, X., Lin, C. J., Wu, C. S., Zhang, Y. P., He, N. N., Fu, P. Q., Wang, Z. F., Shang, L. H., Sommar, J., Sonke, J. E., Maurice, L., Guinot, B., and Feng, X. B.: Isotopic composition of atmospheric mercury in China: new evidence for sources and transformation processes in air and in vegetation, Environ. Sci. Technol., 50, 9262–9269, https://doi.org/10.1021/acs.est.6b01782, 2016.
Yuan, W., Sommar, J., Lin, C. J., Wang, X., Li, Kai, Liu, Y., Zhang, H., Lu, Z. Y., Wu, C. S., and Feng, X. B.: Stable isotope evidence shows re-emission of elemental mercury vapor occurring after reductive loss from foliage, Environ. Sci. Technol., 53, 651–660, https://doi.org/10.1021/acs.est.8b04865, 2019.
Zhang, H., Holmes, C., and Wu, S.: Impacts of changes in climate, land use and land cover on atmospheric mercury, Atmos. Environ., 141, 230–244, https://doi.org/10.1016/j.atmosenv.2016.06.056, 2016.
Zhang, L., Wright, L. P., and Blanchard, P.: A review of current knowledge concerning dry deposition of atmospheric mercury, Atmos. Environ., 43, 5853–5864, https://doi.org/10.1016/j.atmosenv.2009.08.019, 2009.
Zheng, W., Obrist, D., Weis, D., and Bergquist, B. A.: Mercury isotope compositions across North American forests, Global Biogeochem. Cy., 30, 1475–1492, https://doi.org/10.1002/2015GB005323, 2016.
Zhou, J. and Obrist, D.: Global mercury assimilation by vegetation, Environ. Sci. Technol., 55, 14245–14257, https://doi.org/10.1021/acs.est.1c03530, 2021.