the Creative Commons Attribution 4.0 License.
the Creative Commons Attribution 4.0 License.
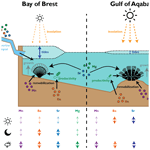
Ultradian rhythms in shell composition of photosymbiotic and non-photosymbiotic mollusks
Daniel Killam
Lukas Fröhlich
Lennart de Nooijer
Wim Boer
Bernd R. Schöne
Julien Thébault
Gert-Jan Reichart
The chemical composition of mollusk shells is a useful tool in (paleo)climatology since it captures inter- and intra-annual variability in environmental conditions. Trace element and stable isotope analysis with improved sampling resolution now allows in situ determination of the composition of mollusk shell volumes precipitated at daily to sub-daily time intervals. Here, we discuss hourly resolved Mg Ca, Mn Ca, Sr Ca, and Ba Ca profiles measured by laser ablation inductively coupled plasma – mass spectrometry (ICP-MS) through shells of the photosymbiotic giant clams (Tridacna maxima, T. squamosa, and T. squamosina) and the non-photosymbiotic scallop Pecten maximus. Precise sclerochronological age models and spectral analysis allowed us to extract daily and tidal rhythms in the trace element composition of these shells. We find weak but statistically significant expressions of these periods and conclude that this cyclicity explains less than 10 % of the sub-annual variance in trace element profiles. Tidal and diurnal rhythms explain variability of, at most, 0.2 mmol mol−1 (∼ 10 % of mean value) in Mg Ca and Sr Ca, while ultradian Mn Ca and Ba Ca cyclicity has a median amplitude of less than 2 µmol mol−1 mol mol−1 (∼ 40 % and 80 % of the mean of Mn Ca and Ba Ca, respectively). Daily periodicity in Sr Ca and Ba Ca is stronger in Tridacna than in Pecten, with Pecten showing stronger tidal periodicity. One T. squamosa specimen which grew under a sunshade exhibits among the strongest diurnal cyclicity. Daily cycles in the trace element composition of giant clams are therefore unlikely to be driven by variations in direct insolation but rather reflect an inherent biological rhythmic process affecting element incorporation. Finally, the large amount of short-term trace element variability unexplained by tidal and daily rhythms highlights the dominance of aperiodic processes in mollusk physiology and/or environmental conditions over shell composition at the sub-daily scale. Future studies should aim to investigate whether this remaining variability in shell chemistry reliably records weather patterns or circulation changes in the animals' environment.
- Article
(3480 KB) - Full-text XML
- BibTeX
- EndNote
Patterns in the growth increments, microstructure, and chemical composition of accretionary carbonate bioarchives yield detailed information about the environmental conditions and biological rhythm of carbonate-producing animals (Dunbar and Wellington, 1981; Jones, 1983; Witbaard et al., 1994; Klein et al., 1996; Surge et al., 2001; Schöne et al., 2005a; Ivany, 2012; Schöne and Gillikin, 2013; DeCarlo and Cohen, 2017; Killam and Clapham, 2018). These characteristics have spurred the development of a multitude of techniques for extracting information about life history (Jones and Quitmyer, 1996; Schöne et al., 2005b; Eggins et al., 2003; Anand and Elderfield, 2005; Goodwin et al., 2009; Mahé et al., 2010; Comboul et al., 2014; DeCarlo and Cohen, 2017; Judd et al., 2018; de Winter et al., 2023), carbonate chemistry (Sinclair et al., 1998; Lazareth et al., 2003; Schöne et al., 2010; de Winter and Claeys, 2017; Warter and Müller, 2017; Huyghe et al., 2021; de Winter et al., 2021b), and microstructure (Lazier et al., 1999; Checa et al., 2007; Popov, 2014; Gilbert et al., 2017; Crippa et al., 2020; Höche et al., 2020, 2021; Wichern et al., 2023) from carbonate shells and skeletons. As a result, (fossil) carbonate skeletons have gained much attention as archives of past environmental and climate change (Lough, 2010; Schöne and Gillikin, 2013; Ivany and Judd, 2022, and references therein).
Three characteristics make the shells of marine mollusks especially valuable as climate archives: (1) nearly all marine mollusks precipitate their shells in equilibrium with the stable oxygen isotope composition of ambient seawater, except for juvenile oysters, some mollusks growing near hydrothermal vents, and some deep-burrowing species (Schöne et al., 2004; Hallmann et al., 2008; Wisshak et al., 2009; Huyghe et al., 2021; de Winter et al., 2022); (2) mollusk shells have a high fossilization potential and long geological history, dating back to the beginning of the Phanerozoic (Al-Aasm and Veizer, 1986a, b; Jablonski et al., 2003; Cochran et al., 2010; Jablonski et al., 2017; de Winter et al., 2017, 2018; Coimbra et al., 2020); (3) the incremental growth of mollusk shells allows internal dating within the shell, yielding chronologies of shell growth with sub-annual precision (Richardson et al., 1980; Jones, 1983; Schöne et al., 2005b; Goodwin et al., 2009; Huyghe et al., 2019). These advantages enable mollusk shells to record important information about climate and ambient water chemistry on the seasonal scale. Thereby, reconstructions from mollusk shells are highly complementary to other, less highly resolved but longer-term climate and environmental reconstructions like sedimentary records, tree rings, and ice cores (Black, 2009; Bougeois et al., 2014; Petersen et al., 2016; Tierney et al., 2020; de Winter et al., 2021a).
The resolution of the mollusk shell archive is not limited to seasonal variability. Studies monitoring the behavior of mollusks during growth experiments show that their activity varies as a function of environmental conditions (e.g., temperature and food availability) and follows ultradian rhythms which may contain daily to hourly periodicities, probably linked to diurnal and tidal cycles, or which lack periodic behavior altogether (Rodland et al., 2006; García-March et al., 2008; Tran et al., 2011; Ballesta-Artero et al., 2017; Xing et al., 2019; Tran et al., 2020). Analyses of the growth patterns and, more recently, the composition of shell carbonate deposited at these short time intervals show that these ultradian rhythms can be recorded in mollusk shells (Pannella, 1976; Richardson et al., 1980; Sano et al., 2012; Warter et al., 2018; de Winter et al., 2020). This raises the question of whether mollusk shells reliably record behavioral changes and/or high-frequency (paleo-)weather or circulation patterns (e.g., Komagoe et al., 2018; Yan et al., 2020; Poitevin et al., 2020). Alternatively, the presence of daily cyclicity in shell chemistry may yield information about the paleobiology of extinct mollusks, such as the use of photosymbiosis (e.g., Sano et al., 2012; Warter et al., 2018; de Winter et al., 2020). The latter seems plausible given the effect of photosymbiosis on shell mineralization in modern tridacnids (Ip and Chew, 2021) and on the trace element composition of aragonite in modern photosymbiotic scleractinian corals (Cohen et al., 2002; Meibom et al., 2003; Inoue et al., 2018). If proven true, daily variability in bivalve shells may serve as a proxy for photosymbiosis in the fossil record (e.g., de Winter et al., 2020). This is of interest because photosymbiosis is a derived adaptation of some tropical bivalve species (e.g., tridacnids), and its prevalence in the fossil record has important implications for the ecological niche of fossil mollusks (e.g., Vermeij, 2013). In addition, photosymbiosis can affect mollusk shell composition, and understanding it is therefore critical for the interpretation of chemical proxies in mollusk shells for environmental reconstructions (Killam et al., 2020). Finally, improving our understanding of photosymbiosis in tropical ecosystems sheds light on the resilience of photosymbiotic organisms to environmental change, now and in the geological past. The latter is of special interest in light of the ongoing climate and biodiversity crises, which are profoundly affecting these sensitive ecosystems (Pandolfi and Kiessling, 2014).
In this study, we investigate the shell growth patterns and shell chemistry of the photosymbiotic bivalves Tridacna maxima, T. squamosa, and T. squamosina and of the non-photosymbiotic scallop Pecten maximus. P. maximus was chosen as a non-photosymbiotic counterpart in comparison to the tridacnids because of its comparatively high growth rate and the presence of daily striae visible on the outer surface of its shell, which make it possible to construct accurate shell chronologies (Chauvaud et al., 2005). We combine ultra-high-resolution (micrometer-scale) Mg Ca, Sr Ca, Mn Ca, and Ba Ca measurements in the shells with detailed sclerochronology to investigate the variability in these trace elements over time in all four species. The aims of this study are to investigate (1) whether the shells record high-frequency variability in shell chemistry that can be linked to environmental and/or circadian rhythms and (2) whether the presence of photosymbiosis influences the expression of this variability in the shell composition.
2.1 Preparation of P. maximus specimens
Three specimens of the king scallop P. maximus (labeled PM2, PM3, and PM4) were collected alive by scuba divers on 15 November 2019 on the southern coast of the Bay of Brest near Lanvéoc, France (48∘17′ N, 4∘30′ W), from a depth of approximately 8 m with permission from the Prefect of the Brittany Region (see Fröhlich et al., 2022; Fig. 1). Note that water depth in the Bay of Brest varies significantly due to the macrotidal regime, with a mean tidal range of 2.8–5.9 m and with extreme ranges up to 7.2 m (Guillaume Olivier et al., 2021; Service Hydrographique et Océanographique de la Marine – Géoportail, 2022). Collected specimens contained at least 1 full year of growth based on the visibility of one winter growth line on the outside of the shell (age class 1; see Thébault et al., 2022; Figs. 1f and S1 in de Winter, 2023). Specimens were frozen at −20 ∘C immediately after collection. Soft body parts and epibionts were removed from the shells before further treatment. Shells were superficially cleaned using a plastic brush, and adhering sediment was removed by ultrasonication in deionized water. The flat, left valves were used for elemental and sclerochronological analysis following previous studies on P. maximus (Thébault et al., 2022; Fröhlich et al., 2022).
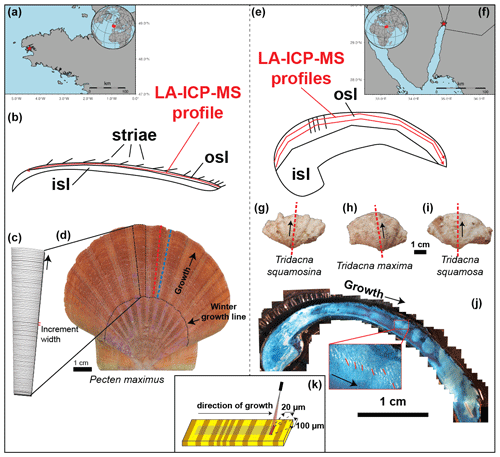
Figure 1Overview of sample locations and preparation steps. (a) Location of the Bay of Brest, with the red star indicating the sampling location. (b) Schematic cross section through P. maximus showing how the LA-ICP-MS line scan (red line) was positioned within the outer shell layer (OSL). (c) Schematic representation of a segment through the shell of P. maximus showing the striae which are deposited daily and which were counted to establish age models (see also b). (d) Left valve of P. maximus (PM2) used in this study, with dashed lines showing the position of cross sections through a rib (red) and valley (blue) in the shell. Black arrow indicates growth direction away from the shell hinge. The dotted black line highlights a winter growth stop. (e) Schematic cross section through a tridacnid, illustrating the positions of parallel LA-ICP-MS line scans (red lines) through these shells within the OSL. (f) Position of the Gulf of Aqaba, with the red star indicating the sample location for tridacnids. (g–i) Pictures of (from left to right) T. squamosa (specimen TSFRS1), T. maxima (specimen TM29), and T. squamosina (specimen SQSA1), with dashed red lines indicating the positions of the cross sections used for LA-ICP-MS analysis (see c) and black arrows indicating the direction of growth. (j) Example of Mutvei-stained cross section through a T. maxima specimen used to visualize and count growth lines, with the insert showing part of the OSL where growth lines were counted (red lines) to establish age models for the tridacnids. Black arrows indicate the direction of growth. (k) Schematic representation of the LA-ICP-MS line-scanning setup with the rectangular spot size (100 × 20 µm; see S11 in de Winter, 2023) that was positioned parallel to the growth layers in the shell.
High-resolution color photos were made of the outside of the left valve of the shell using a mirror-reflex camera (Canon EOS 600 DSLR camera connected to a Wild Heerbrugg binocular microscope equipped with a Schott VisiLED MC 1000 sectoral dark-field light source) aimed downward perpendicular to the working surface. Overlapping images of the shells were stitched together using Image Composite Editor v2.0.3.0 (Microsoft Research Computational Photography Group, Redmond, WA, USA). The stitched images were used to count and measure daily striae on the shell surface (see Fig. 1 and S1 in de Winter, 2023). To obtain a fully focused composite of the complete shell, dynamic focusing was applied to allow all parts of the slightly curved surface of the shell to come into focus. Dynamic-focus images were later stitched together using focus stacking in Helicon Focus (Helicon Focus 7.7.5; HeliconSoft, Kharkiv, Ukraine; see S1 in de Winter, 2023).
Cross sections were cut through all three P. maximus shells perpendicular to the daily growth lines (striae) from the ventral margin of the shell to the shell hinge (see Fig. 1b–d and S1 in de Winter, 2023) along the axis of maximal growth. Shells were fortified with a protective layer of metal epoxy (GLUETEC WIKO Epofix 05) before sectioning using a Buehler Isomet 1000 low-speed precision saw (Buehler Inc., Lake Bluff, IL, USA) equipped with a diamond-coated wafering thin blade (0.4 mm thickness; number 15LC 11–4255) at 200 rpm. Parallel cuts were made to allow shell sections to be glued to glass plates for grinding (down to F1200 grit SiC powder) and high-grade polishing (1 µm Al2O3 suspension on Buhler polishing cloth, MasterTex). Two cross sections were made through specimens PM2 and PM3: one through a rib of the shell (i.e., radial segment that protrudes away from the interior, named PM2_1 and PM3_1) and one through a valley (i.e., radial segment between two ribs that lies deeper towards the interior, named PM2_2 and PM3_2; see Fig. 1 and S1 in de Winter, 2023). The dual sections were cut to compare shell chemistry between the ribs and valleys of the shell. Specimen PM4 was only sectioned once, through a valley in the shell, yielding a total of five cross sections through the P. maximus specimens.
2.2 Preparation of Tridacna specimens
A total of five tridacnid specimens, two T. maxima specimens (named TM29 and TM84), two T. squamosa specimens (named TS85 and TSFRS1), and one T. squamosina (SQSA1) specimen, were collected in the summer of 2016 from beach death assemblages on the coast of the Gulf of Aqaba with permission from the Israeli National Parks Authority (Fig. 1; see details in Killam et al., 2020). One cultured Tridacna squamosa shell (TSM1) was obtained from the National Center for Mariculture, Eilat. Species were determined following shell characteristics of the local population as cited in Roa-Quiaoit (2005).
All shells were sectioned along the axis of maximum growth after removing epibionts using a metal brush (see Fig. 1g–i). The original microstructure and preservation of the original aragonite mineralogy of all specimens were confirmed using scanning electron microscopy and X-ray diffraction spectroscopy following Gannon et al. (2017) and Kontoyannis and Vagenas (2000) (see details in Killam et al., 2020). Shell segments were partially embedded in Araldite 2020 epoxy resin (Huntsman Corp., Woodlands, TX, USA) before being sectioned in the direction of maximum growth using a slow-rotating saw equipped with a thin wafered saw blade (thickness < 1 mm). Parallel cross sections produced 5–10 mm thick sections that were polished using progressively finer SiC polishing disks.
2.3 Microscopy and photography
Polished surfaces of all 11 cross sections (5 Pecten and 6 Tridacna) were imaged using an Epson® 1850 flatbed scanner (Seiko Epson Corp., Nagano, Japan) at a pixel resolution of 6400 dpi (±4 µm pixel size), as well as by stitching micrographs made using a KEYENCE VHX-5000 digital microscope using × 250 magnification together into composite images (see S1 in de Winter, 2023). Cross sections were imaged both before and after trace element analyses to allow the trace element profiles to be referenced relative to the cross sections.
2.4 LA-ICP-MS analyses
Elemental ratios were based on measuring ratios of the isotopes 25Mg, 87Sr, 55Mn, and 137Ba to 43Ca along profiles through all shell cross sections using laser ablation inductively coupled plasma mass spectrometry (LA-ICP-MS). Measurements were carried out on a laser ablation system (ESI NWR193UC; Elemental Scientific, Omaha, NE, USA) coupled to a quadrupole ICP-MS (iCap-Q, Thermo Fisher Scientific, Waltham, MA, USA) at the Royal Netherlands Institute for Sea Research (NIOZ). Operation parameters are provided in S11 in de Winter (2023).
Scan lines were programmed on the polished-shell cross sections in the direction of growth as close as possible to the outer edge of the shell, with the rectangular LA-ICP-MS spot oriented parallel to the growth lines (with a width of 20 µm in scanning direction; see Fig. 1j; S11 in de Winter, 2023). For the pectinids, care was taken to target the outer portion of the outer shell layer (oOSL) and t6o avoid sampling of the inner portion of the outer shell layer (iOSL) or the inner shell layer (ISL), which was demonstrated to have a different chemical composition (see Freitas et al., 2009). For the tridacnids, profiles were placed within the OSL close to (within 100 µm of) the outer edge of the shell in a first analytical session. However, since spikes of high Mg Ca and Mn Ca ratios were observed in these results, parallel transects placed approximately 100 µm further towards the inside of the shell were measured through all tridacnid shells to verify whether these spikes in Mg and Mn were reproducible further inward (see S2 in de Winter, 2023). The combination of laser scan speed (4 µm s−1) and ICP-MS run cycle time (sweep time; 109 ms) yielded a mean spatial sampling resolution of 0.44 µm, determined as the arithmetic mean without considering the shape of the laser spot. However, note that the width of the LA-ICP-MS spot size in the scanning direction (20 µm) caused smoothing of the LA-ICP-MS signal, reducing the effective spatial sampling resolution. As a result, each 0.44 µm wide segment of the shells in the growth direction is sampled 45 times while the spot moves across the shell. We tested the effect of this smoothing on a virtual record with characteristics similar to the Sr Ca record obtained by Sano et al. (2012; see Sect. 3.3 and S13 in de Winter, 2023). All scan lines in pectinids and tridacnids were repeated a second time at the exact same location using a faster scan rate of 10 µm s−1 to assess the repeatability of the elemental signals (see S2 in de Winter, 2023).
Data reduction was performed using an adapted version of the data reduction software SILLS (Signal Integration for Laboratory Laser Systems; Guillong et al., 2008) in MATLAB. Raw LA-ICP-MS data were calibrated using NIST610 (National Institute of Standards and Technologies, Gaithersburg, MD, USA) using the reference values reported in the GeoReM database (Jochum et al., 2005, 2011). Quality control reference materials BAS CRM 393 (Bureau of Analyzed Samples, Middlesbrough, UK) and RS3 and one matrix-matched carbonate standard (MACS-3; United States Geological Survey, Reston, VA, USA; Wilson et al., 2008) were used to monitor the quality of the measurement. Details on the accuracy of the LA-ICP-MS trace element results relative to preferred values for the check standards are provided in S12 in de Winter (2023).
To increase the stability of the ICP-MS signal and to correct for drift, 43Ca was used as an internal standard. Calcium concentrations were assumed to be constant (38 wt %) throughout the LA-ICP-MS profiles within the same shell layer. External drift correction using repeated measurements on the JCp1 standard was applied. The element Ca drift was less than 5 % during the analytical sequence. Drift during a single transect was found to be negligible.
2.5 Age models
Trace element profiles in P. maximus shells were internally dated using daily striae visible on the outer shell surface (Fig. 1d). Daily increment widths (perpendicular distances between successive striae) were counted and measured multiple times by different persons, both on the outside of the shell using the focus-stacked images (see Sect. 2.3) and by counting and measuring the distance between growth layers in cross sections through the valleys of the shells (PM2_2 and PM3_2; see S3 in de Winter, 2023). Positions of daily striae on the outside of the shell were plotted relative to distance along the LA-ICP-MS scan line using manual alignment of striae and the LA-ICP-MS path on microscope composites of cross sections through the shells, taking into account the curvature of growth lines with distance away from the outer shell surface (see S3 in de Winter, 2023). The timing of shell formation was determined by backdating the daily striae from the ventral margin (last visible stria mineralized on the date of shell collection, i.e., 15 November 2019) and by linearly interpolating the timing of measurements located between daily growth lines based on their distance from daily striae positions (S5 in de Winter, 2023).
Trace element profiles from Tridacna shells were also dated using layer counting. Polished cross sections through all tridacnids were imaged using UV luminescence (see Fig. 1j and S4 in de Winter, 2023) and stained with Mutvei solution (Schöne et al., 2005c; Killam et al., 2021) to facilitate this counting. However, despite staining and luminescence techniques, the expressions of daily and semi-diurnal (half-daily or 12 h rhythmic) growth markings were insufficiently clear to count individual growth lines along the full (multi-year) growth period recorded in all the shells. Therefore, we opted for a hybrid method in which we measured the width of daily and semi-diurnal increments in parts of the cross sections of each shell where they were well developed and used these measurements in combination with the annual growth breaks (which are easily recognized on the shell) to create age models for all specimens. The distinction between diurnal (24 h) and tidal (∼ 12 h) pacing of growth increments was made based on the width of small-scale increments relative to the width of annual increments in the shell. A von Bertalanffy growth model (von Bertalanffy, 1957) was constructed for each specimen based on the annual growth (ΔL) inferred from (semi-)diurnal growth line counting and the maximum shell height (Linf) known for these species in the Red Sea from the literature (Roa-Quiaoit, 2005; Mohammed et al., 2019):
with
In this formula, Lt is the shell height at time t, and k is the growth constant (Brody growth coefficient; Munro, 1982). Since cross sections through the tridacnids were made through the shell hinge (in direction of the shell height) and since literature values for Linf are reported with reference to shell length (measured parallel to the shell hinge), allometric data on T. maxima, T. squamosa, and T. squamosina from the literature were used to convert Linf values (which are commonly reported as shell length) to shell height and to make them relevant for the direction in which the trace element profiles were measured on the cross sections (Roa-Quiaoit, 2005; Richter et al., 2008; Mohammed et al., 2019). Uncertainties regarding the annual growth increment widths (ΔL) were calculated from the standard error of the mean width of daily and semi-diurnal growth increments on which ΔL is based, and uncertainties regarding the values for Linf were taken from the variability in the values in the literature. Both sources of uncertainty were propagated through the growth model using the variance formula (Ku, 1966) to obtain error envelopes on the age–distance relationships (growth curves) of tridacnids (see S5 in de Winter, 2023). All data-processing steps described in this paper are carried out using the open-source computational software package R (R Core Team, 2013), and scripts detailing these calculations are provided in S6 in de Winter (2023) and deposited on the open-access software repository GitHub (https://doi.org/10.5281/zenodo.6603175, de Winter, 2022).
2.6 Spectral analysis
Spectral analysis on the LA-ICP-MS data was used to isolate trace element variability at the sub-annual scale. All trace element profiles were first detrended using a locally estimated scatterplot smoothing (LOESS) filter with a span of 0.2 times the length of the record to remove longer-term (i.e., seasonal to multi-annual) trends. The detrended series were linearly resampled in the time domain before applying the multi-taper method (MTM; Thomson, 1982) to extract dominant frequencies from the data. Spectral analysis was carried out using the Astrochron package (Meyers, 2014) in R (R Core Team, 2013; see script in S6). The significance of relevant periodicities was tested using a combination of red-noise estimation and a harmonic F test (see Meyers, 2012). To visualize the evolution of periodic behavior across the shells, wavelet analysis was applied on all trace element profiles using the dplR package in R (see S6 in de Winter, 2023).
2.7 Extracting high-resolution variability
After detrending and spectral analysis, all trace element profiles were smoothed using a Savitzky–Golay filter with a width of 21 data points (8.4 µm; equivalent to a time span of ∼ 1–5 h; S6 in de Winter, 2023) to remove high-frequency measurement noise within our LA-ICP-MS spot size. The Savitzky–Golay filter was used because it preserves the tendency of the trace element profile and therefore retains a larger fraction of the amplitude of periodicity close to the width of the filter compared to simpler smoothing techniques (e.g., moving average; Savitzky and Golay, 1964). The 21-point window size was chosen to smooth out the maximum amount of instrumental noise without losing periodicity larger than half the width of the laser spot (0.22 µm; equivalent to 23 data points). Statistically significant (see Sect. 2.6) variability in daily (∼ 22–36 h; centered on the 24 h diurnal cycle) and semi-diurnal (∼ 8–14 h; centered on the 12.4 h tidal cycle) frequency bands was extracted from the trace element records using a combination of bandpass filtering (using the bandpass function in the Astrochron R package) and stacking (see S6 in de Winter, 2023). Trace element data were stacked along bandpass filters using the following procedure: maxima and minima in the bandpass filter were used as tie points to reference each data point of the smoothed dataset relative to its position within the cycle on a scale from 0 to 1. These relative positions were then used to divide the data into 10 bins (bin 1 contains positions 0–0.1, bin 2 contains data from positions 0.1–0.2, etc.), providing stacked data a resolution of 0.1 times the length of the cycle under investigation. The full breakdown of variability within and between bins created in the stacking routine is provided in S7 in de Winter (2023). Different sources of variance in the trace element records were isolated by sequentially determining the variance left in the trace element records after each of the data treatment steps explained above (see example in S7 in de Winter, 2023). This procedure allowed us to quantify the amount of variance in each trace element profile explained by either diurnal or semi-diurnal variability.
3.1 Trace element data
LA-ICP-MS line scans yielded profiles of Sr Ca, Mg Ca, Mn Ca, and Ba Ca in growth direction on 11 cross sections through shells of P. maximus, T. maxima, T. squamosa, and T. squamosina (Fig. 2; see S0 in de Winter, 2023, for raw data of all scans). Sub-millimeter-scale patterns in Sr Ca, Mg Ca, Mn Ca, and Ba Ca are highly reproducible between consecutive line scans on the same LA-ICP-MS transect: R2 values typically exceed 0.8, and mean element ratio differences between time-equivalent samples in different line scans are typically < 0.05 mmol mol−1 for Mg Ca (< 5 % relative to mean value), < 0.02 mmol mol−1 for Sr Ca (< 2 % relative to mean value), < 0.5 µmol mol−1 for Mn Ca (< 10 % relative to mean value), and < 0.2 µmol mol−1 for Ba Ca (< 8 % relative to mean value; see Table S2 in de Winter, 2023). Remeasured transects further away from the outer shell surface in tridacnids (see Sect. 2.4) differ more from the original transects than those measured on the exact same locality in the shell: R2 values between parallel lines in different localities are 0.3–0.5 for Mg Ca and Sr Ca and < 0.3 for Mn Ca and Ba Ca, reflecting intra-shell variability in trace element composition in the tridacnids (S2 in de Winter, 2023). Overall, sub-millimeter-scale patterns in trace element composition are reproduced in parallel line scans. Finally, rib and valley segments through the same specimen of P. maximus show similar patterns in trace elements, but element-to-calcium ratios (especially Ba Ca and Mn Ca) can be quite different, highlighting heterogeneity within the shells of P. maximus (Fig. 2).
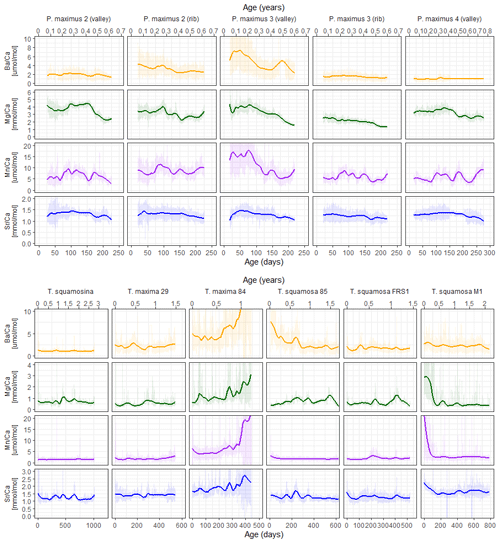
Figure 2Overview of LA-ICP-MS results of Sr Ca (blue), Mg Ca (green), Mn Ca (purple), and Ba Ca (orange) in pectinid (upper panel) and tridacnid (lower panel) specimens. Vertical axes are equal for plots positioned next to each other (but different for the two groups of tridacnid and pectinid plots). Shaded lines show raw LA-ICP-MS data, while solid lines indicate 0.2 span LOESS fits through the data, highlighting monthly scale variability. A direct comparison of trace elemental ratios between specimens is provided in S8 in de Winter (2023).
Trace element profiles in pectinids, reflecting only one growing season, show Sr Ca and Mg Ca maxima in the middle of the profile, while Mn Ca and Ba Ca are more variable, showing multiple peaks in the same growth year. Peaks in Mn Ca and Ba Ca are synchronous between profiles through the same specimen but not between specimens. Tridacnid Mg Ca and Sr Ca ratios show one or two distinct cycles per growth year. Mn Ca and Ba Ca ratios in tridacnids show more regular annual or biannual variability than pectinids (most notably specimen SQSA1). It must be noted, however, that P. maximus shells only recorded one growth season, limiting the interpretation of seasonal growth patterns.
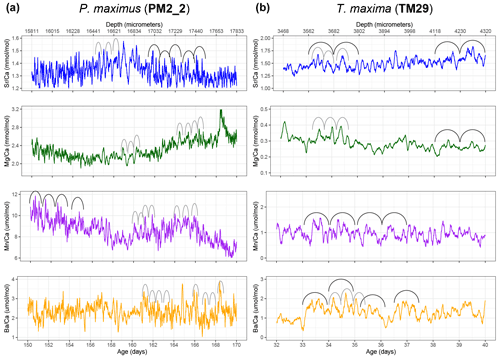
Figure 3Plots showing samples of semi-diurnal and daily variability in Savitzky–Golay-filtered trace element ratios in selected intervals through P. maximus specimen PM2_2 (a; 51-point filter) and T. maxima specimen TM29 (b; 21-point filter). Filters isolate hourly to daily scale variability while filtering out the higher-order measurement noise. Arches indicate examples of the expression of daily (black) and semi-diurnal (gray) cycles in the records.
Plots of trace element variability zoomed in on smaller sections of the record reveal dominant high-frequency variability superimposed on seasonal-scale patterns (Fig. 3). Both tridacnid and pectinid LA-ICP-MS records show distinct high-frequency cyclicity in all studied trace element-to-Ca ratios, with amplitudes on nearly the same order of magnitude as the longer-term variability described by the records on a seasonal to multi-annual scale (Fig. 2).
3.2 Age models
Growth line counting in the P. maximus shells was repeated multiple times both on the outer shell surface and in cross sections through the shell by different persons (Table 1; Fig. S3 in de Winter, 2023). The variability in counting results shows that the growth lines were not always equally easy to distinguish. In PM2 and PM3, the most likely number of increments (228 and 220, respectively) was confirmed both in cross sections and on the outside of the shell. In PM4, the count in the cross section (278 increments) was chosen over the slightly lower count on the outside of the shell (272–273 increments) since the LA-ICP-MS profile was measured on the same cross section and could be directly linked to the counted increments. Note that, while the consistency in daily counts between specimens from the same year and environment is encouraging, inter-specimen variability with the onset of spring growth or with the onset of the winter stop (potentially prior to the collection date of 15 November 2019) places some uncertainty on the absolute date assigned to P. maximus shell sections (Chauvaud et al., 2005). The age–distance relationships (growth curves) resulting from the sclerochronology are shown in S5 in de Winter (2023).
Table 1Growth increment counting in P. maximus. Values separated by commas refer to different counts at the same location of the same specimen. Values in bold indicate counts closely corresponding between cross section and outer shell surface.
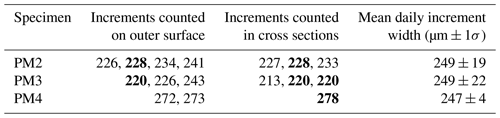
Table 2Growth line counting in Tridacna shells. Column 3 shows the total number of increments counted in the specimen, column 4 shows their median width, and column 6 shows the width of an annual increment in the specimen. Note that increments could not be counted over the entire growth period of the shells so the numbers in column 3 represent representative numbers of increments counted in those parts of the shells where they were distinct enough for counting (see Sect. 2.5). Increment timing (semi-diurnal vs. diurnal) was established based on the relative difference between increment width and the distance between annual growth breaks measured on the outside of the shells (see S4 in de Winter, 2023, for details).
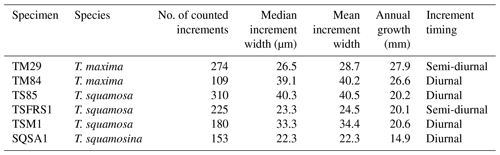
Layer counting in tridacnid shells yielded estimates of semi-diurnal, daily, and annual growth (Table 2; S4 in de Winter, 2023). Annual growth rates calculated from layer counting are highly consistent between specimens from the same species from the same environment (within 1 mm yr−1; Table 2), lending confidence to the growth-line-counting results. The von Bertalanffy growth models based on these growth line counts are plotted in S5 in de Winter (2023). Statistics of the parameters (Linf and k) of these growth models and their uncertainty are provided in S4 in de Winter (2023).
3.3 Growth rates and temporal sampling resolution
Growth rates are highly similar between specimens of the same species (Tables 1 and 2; S3–S5 in de Winter, 2023), with P. maximus achieving the highest growth rates (∼ 220 growth days × ∼ 250 µm d−1 ≈ 55 mm yr−1; Table 1), followed by T. maxima (∼ 27 mm yr−1; Table 2), T. squamosa (∼ 20 mm yr−1; Table 2), and T. squamosina (15 mm yr−1; Table 2). Dividing the width of the daily increments (e.g., 250 µm for P. maximus) by a spatial LA-ICP-MS sampling resolution of 0.44 µm (see Sect. 2.4), these growth rates would seem to translate to temporal resolutions of 0.04, 0.24, 0.44, and 0.27 h for P. maximus, T. maxima, T. squamosa, and T. squamosina, respectively. However, our LA-ICP-MS spot size (20 µm in sampling direction) causes significant smoothing, reducing the frequency that can be resolved. Nevertheless, our test applying the LA-ICP-MS settings on a virtual Sr Ca dataset with the characteristics of the diurnal record by Sano et al. (2012) and growth rates equivalent to those of our specimens (see S13 in de Winter, 2023) reveals that our analytical methodology can easily resolve daily variability even in our slowest-growing specimen (T. squamosina SQSA1), conserving at least 75 % of the signal amplitude. The LA-ICP-MS profiles record trace element variability during growth periods ranging between 220 d (for PM3) and 1041 d (for SQSA1).
3.4 Spectral analysis
Normalized power spectra and the significance level of daily and tidal periodicities in pectinid and tridacnid records are shown in Figs. 4 and 5, respectively. Full spectral analysis results for all trace element records in all specimens are provided in S9. All P. maximus power spectra (Fig. 4) reveal semi-diurnal (12 h) periodicity in Sr Ca and Ba Ca, with > 86 % statistical significance. Only sections through the ribs of the shells (PM2_1 and PM3_1) show semi-diurnal periodicity in Mg Ca and Mn Ca (> 90 % significance). Daily periodicity is present in some, but not all, pectinid profiles. Most pectinid trace element records also contain multi-day periodicities, including strong 7 d periodicities and weaker cyclicity associated with 14 and 28 d cycles. The latter is partly suppressed by the 0.2 span LOESS filter (equivalent to a 44–56 d period depending on the length of the record) applied on the records to remove the seasonal trend from the records. However, these lower-frequency cycles are clearly visible in the wavelets (see S9 in de Winter, 2023).
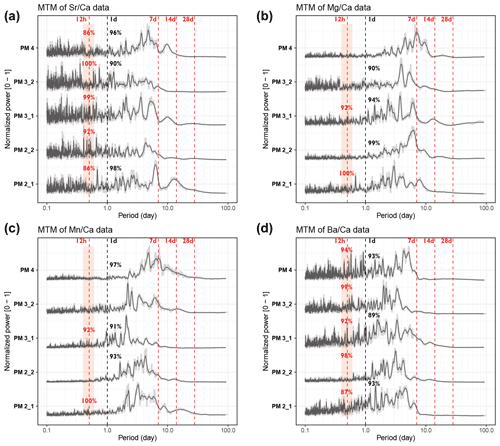
Figure 4Multi-taper-method spectrograms of Sr Ca (a), Mg Ca (b), Mn Ca (c), and Ba Ca (d) records through the five pectinid cross sections after detrending (see Sect. 2.6). All spectra are normalized by dividing by the highest power peak and are plotted on the same horizontal axis. Gray-shaded lines show raw data, while solid black lines plot 21-point moving-average-smoothed curves. Vertical dashed red lines highlight the expected periods of tidal variability, while vertical dashed black lines indicate 1 d periodicities. Significance levels of peaks in these periods (see Sect. 2.6 and Meyers, 2012) are rounded to the nearest whole percentage point.
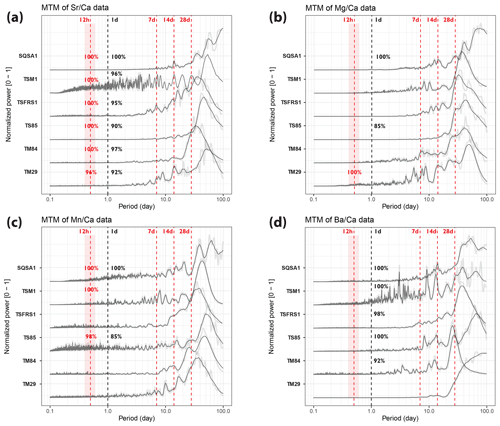
Figure 5Multi-taper-method spectrograms of Sr Ca (a), Mg Ca (b), Mn Ca (c), and Ba Ca (d) records through the six tridacnid cross sections after detrending (see Sect. 2.6). All spectra are normalized by dividing by the highest power peak and are plotted on the same horizontal axis. Gray-shaded lines show raw data, while solid black lines plot 21-point moving-average-smoothed curves. Vertical dashed red lines highlight the expected periods of tidal variability, while vertical dashed black lines indicate 1 d periodicities. Significance levels of peaks in these periods (see Sect. 2.6 and Meyers, 2012) are rounded to the nearest whole percentage point.
Tridacnid trace element profiles show more consistent daily periodicity than pectinid records (Fig. 5). In particular, Sr Ca and Ba Ca records through nearly all tridacnid specimens exhibit strong (> 90 % confidence level) power in the daily period, while daily cyclicity is weaker in Mn Ca and Mg Ca records. Sr Ca records in the tridacnids also contain a significant (> 96 %) semi-diurnal component whose tidal origin seems clear in most specimens by peaks in power in the longer (7, 14, and 28 d) tidal components.
3.5 Variance decomposition
Variability at the daily (24 h) and semi-diurnal (12 h) scale in all trace element records through all specimens was extracted using bandpass filtering (Sect. 2.7; see S9 and S10 in de Winter, 2023). The median amplitude of variability within these stacks was plotted against the median period of the variability per element and per specimen to highlight dominant periodicities in the trace element data (Fig. 6). As noted in the spectral analysis results (Sect. 3.4), trace element composition in tridacnid shells is more strongly controlled by daily variability than in pectinid shells (Fig. 6; S10 in de Winter, 2023). The difference is especially noticeable in Sr Ca and Ba Ca ratios, which show a clear divide between daily periodicity in tridacnid shells and tidal periodicity in pectinids (see Fig. 6). The differences in Mg Ca and Mn Ca ratios are less obvious.
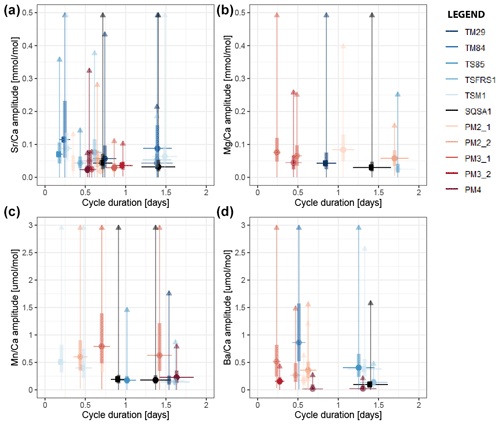
Figure 6Cross plot showing the amplitude of variability of dominant spectral periods in Sr Ca (a), Mg Ca (b), Mn Ca (c), and Ba Ca (d) against the period (duration) of the cycle. Round symbols indicate the median amplitude of the cycle, while vertical bars and lines show interquartile differences and ranges in the amplitude over the record. Horizontal bars indicate the width of the bandpass filter used to extract periodic variability. Colors highlight different specimens (see legend).
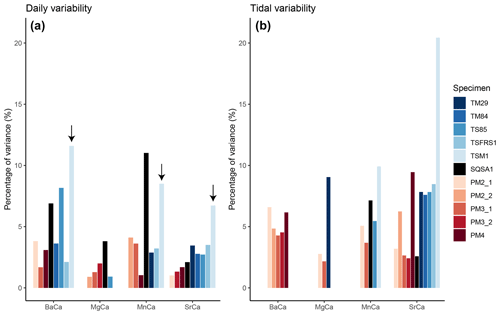
Figure 7Summary of relative variance (in %) of significant daily (a) and tidal (b) variability extracted from trace element records. Colors highlight different specimens (see legend). Note that the T. squamosa specimen TSM1, which grew under a sunshade, is highlighted with a black arrow.
Table 3Overview of the relative (in %) variance associated with daily and tidal variability in all trace element records through all specimens. Empty cells represent records for which no significant tidal or daily periodicity was found (see Figs. 4–5).
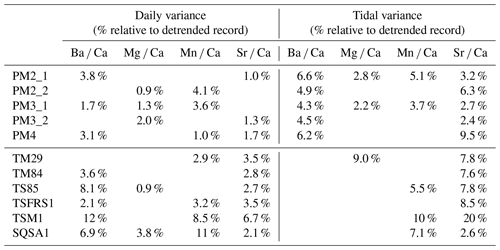
An example of the distribution of normalized variability within the trace element records after each data-processing step is shown in S7 in de Winter (2023). This example shows that a large fraction of the variance in the records (73 % in this record after trimming outliers) is explained by low-frequency (seasonal-scale) variability (S7 in de Winter, 2023). Of the remaining smoothed and detrended dataset, at most, 20 % of the variance is explained by daily and tidal (semi-diurnal) periodicity (see Fig. 7 and Table 3). A full decomposition of variance in all trace element records through all specimens is provided in S7 in de Winter (2023). Figures 6 and 7a show that, overall, the variance explained by daily periodicity is higher in tridacnids than in pectinids (Wilcoxon signed rank test; W = 44; p = 0.009). The difference between species is smaller for tidal variability (Fig. 7b). There is no clear difference in the relative dominance of semi-diurnal variability between trace element records, but daily variability is more strongly expressed in Ba Ca and Mn Ca records, especially in tridacnid shells. Finally, T. squamosa specimen TSM1, which grew under a sunshade, does not exhibit significantly lower daily periodicity compared to the other tridacnid specimens.
4.1 Compositional differences between pectinids and tridacnids
Pectinid and tridacnid shells are characterized by similar mean Sr Ca and Ba Ca ratios (Sr Ca of 1.3 ± 0.3 and 1.5 ± 0.6 mmol mol−1, respectively; Ba Ca of 2.8 ± 2.5 and 3.0 ± 5.1 µmol mol−1, respectively; uncertainty is calculated as 1σ). Mean Mg Ca and Mn Ca ratios are higher in P. maximus than in Tridacna species (Mg Ca = 3.1 ± 0.9 and 0.7 ± 0.9 mmol mol−1, Mn Ca = 7.8 ± 4.7 and 2.7 ± 7.8 µmol mol−1; 1σ; Fig. 2; S4 in de Winter, 2023). The incorporation of higher concentrations of the elements Mg and Mn, which have a smaller ionic radius than Sr and Ba, in calcitic pectinid shells compared to in aragonitic tridacnid shells makes sense given the higher partition coefficient of these elements in calcite than in aragonite (Day and Henderson, 2013; Wassenburg et al., 2016).
Differences between tridacnid specimens generally exceed the differences between tridacnids and pectinids (1σ of Ba Ca among all tridacnid specimens = 2.1 µmol mol−1), suggesting large inter-species differences. However, individual records such as those in TM84 and PM3_1 show large variability (especially in Ba Ca and Mn Ca) compared to other specimens of the same species. Inter-specimen variability is higher in tridacnid shells than in pectinids (inter-specimen relative standard deviations as a fraction of mean ratio for Ba Ca: 0.74 vs. 0.64 µmol mol−1, for Mg Ca: 0.37 vs. 0.20 mmol mol−1, for Sr Ca: 0.19 vs. 0.03 mmol mol−1, and for Mn Ca: 0.78 vs. 0.33 µmol mol−1 for tridacnids and pectinids, respectively). Figure 2 shows that this variability between tridacnids is not readily explained by differences between species but mostly reflects differences in the trends within the records, with some specimens (e.g., TM84, TSM1, and TS85) showing trends in composition towards the end of the record (see also S8 in de Winter, 2023). It thus seems that inter-specimen differences, perhaps driven by growth stress, are dominant drivers of trace element variability in giant clams. This interpretation is corroborated by the observation that trace element compositions in tridacnid shells are significantly more skewed towards higher values than in pectinids (mean skewness per element and per specimen is 9.7 for tridacnids and 0.9 for pectinids). This skewness reflects the high peaks in trace element composition observed in tridacnid profiles, especially near the ventral margin (e.g., specimens TM84, TSM1, and TS85; see Sect. 2.4; Fig. 2; S8 in de Winter, 2023), which hint towards growth disturbances at the individual level.
4.2 Trace element variability in P. maximus
4.2.1 Comparison with previous studies
Trace element concentrations in P. maximus analyzed in this study are in close agreement with concentrations found in wild (live-collected) pectinid shells in the literature (Lorrain et al., 2005; Barats et al., 2008; Poitevin et al., 2020; Fröhlich et al., 2022). In these studies, Sr Ca shows a strong link with calcification rate (as measured by the width of daily shell increments; Lorrain et al., 2005), although previous studies did not assess variability on the (sub-)daily scale. The long-term trends in our Sr Ca records seem to confirm this correlation, with higher values being recorded in the middle of the growing season (day 50–150; Fig. 2) when growth rates are highest (see S5 in de Winter, 2023). Previous studies demonstrate that Mg Ca ratios in pectinid shells are at most partially related to temperature and/or salinity (Lorrain et al., 2005; Poitevin et al., 2020). The fact that the studied P. maximus specimens, which all grew during the same year in the same environment, do not show a synchronous Mg Ca pattern (Fig. 2) corroborates this previous work and argues against a straightforward temperature dependence for Mg Ca in P. maximus. In addition, the lack of strict coherence between profiles of Mg Ca (and other elements) in parallel transects through P. maximus shells (e.g., PM2_1 and PM2_2; Fig. 2) hints at compositional heterogeneity within the shells, in agreement with findings by Lorrain et al. (2005). Low correlations between profiles through the same shell at the daily scale are also partly driven by small misalignments in the timing of shell formation between the transects at the sub-millimeter scale and variations in the height of trace element peaks, especially those of Mn Ca and Ba Ca, which are higher further towards the outside of the shell (S2 in de Winter, 2023).
There is evidence suggesting that Mg content varies in mollusk shells as a function of the amount of organic matter in the biomineral (Dauphin et al., 2003; Richard, 2009; Tanaka et al., 2019). Contrarily, Mn is known to be taken up in thermodynamic equilibrium in the mineral fraction of bivalve shells (Onuma et al., 1979; Soldati et al., 2016), and Mn Ca ratios in P. maximus have been shown to faithfully record fluctuations of dissolved Mn in the coastal environment driven by riverine input and redox conditions (Barats et al., 2008). Similarly, there is strong evidence that Ba Ca ratios in P. maximus (and other mollusks) record primary-productivity-related changes in Ba available in the environment (e.g., Gillikin et al., 2008; Thébault et al., 2009; Fröhlich et al., 2022). Interestingly, our results (Fig. 2) show that background Ba Ca values are not equal in the shells of P. maximus and tridacnid specimens grown in the same environment. This suggests that the findings by Gillikin et al. (2005) that background Ba Ca concentrations are a function of environmental conditions and can be consistently subtracted from Ba Ca records to separate peak from background values may not be generally valid. The suggested relationship between Ba Ca and primary productivity would explain the skewed (skewness > 1; S8 in de Winter, 2023) character of the Ba Ca records and the correlation between Ba Ca and Mn Ca in the studied P. maximus specimens as reducing conditions following peaks in primary productivity favor the remobilization of Mn into the water column, causing short-lived simultaneous increases in Ba Ca and Mn Ca in the shells (Dehairs et al., 1989; Barats et al., 2008, 2009).
4.2.2 Short-term changes in shell composition in P. maximus
In the context of the high-resolution trace element variability central to this study, it seems plausible that short-term changes in the environment of the Bay of Brest were drivers of Mn Ca and Ba Ca variability in P. maximus shells, while Mg Ca and Sr Ca compositions are mostly driven by changes in calcification rate. This would suggest that the significant tidal (12 h) component in Ba Ca and Mn Ca records through P. maximus (Fig. 4) is driven directly by redox changes over the strong tidal cycle in the Bay of Brest (see Polsenaere et al., 2021) and resuspension of Ba and Mn due to tidal currents (Hily et al., 1992), while tidal rhythms in Mg Ca and Sr Ca may be a consequence of the scallop's calcification response to changes in its environment (e.g., temperature, salinity, and light availability) through the large (up to 7 m range) tidal cycle (Fig. 8). The latter corroborates previous studies in other calcitic mollusk shells which demonstrated that Mg incorporation on short timescales is driven by the metabolic response to subtle changes in the environment (Lazareth et al., 2007). Finally, care must be taken to interpret trace element variability in P. maximus shells since large intra-shell gradients in Mg Ca, Sr Ca, and Mn Ca have previously been observed in this species, making trace element composition highly dependent on the location of measurements relative to the outer shell surface or positioning relative to striae on the shell surface (Freitas et al., 2009). Even though the LA-ICP-MS line scans in this study targeted exclusively the oOSL of P. maximus specimens, variability in elemental ratios resulting from small changes in the distance of the line scan from the outer edge of the shell cannot be fully excluded (Richard, 2009).
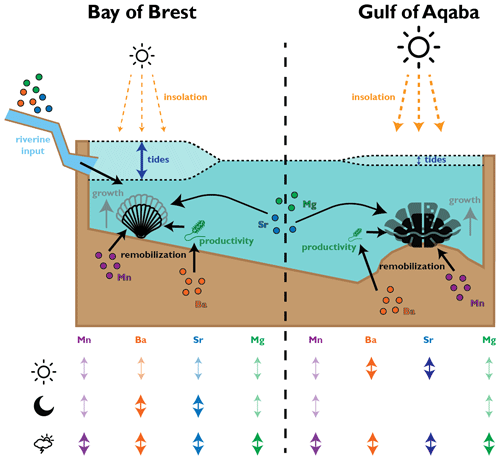
Figure 8Schematic overview of environmental parameters interpreted to affect shell growth and composition of pectinids in the Bay of Brest and tridacnids in the Gulf of Aqaba. The table at the bottom provides a schematic qualitative overview of the amount of variance in the trace element records of the taxa, explained by daily (sun symbol), tidal (moon symbol), or aperiodic (cloud symbol) variability in the environment.
4.3 Trace element variability in tridacnids
4.3.1 Comparison with previous studies
Results for Sr Ca, Mg Ca, and Ba Ca in our tridacnid specimens broadly corroborate trace element results in other tridacnid studies (e.g., Elliot et al., 2009; Sano et al., 2012; Yan et al., 2013; Warter et al., 2018). While data on Mn Ca in the OSL of tridacnids are scarce, the Mn Ca ratios in tridacnids in this study (mean Mn Ca = 7.8 ± 4.7 µmol mol−1) are similar to LA-ICP-MS Mn Ca data available in the literature (Warter et al., 2015; 4–10 µmol mol−1) but are significantly lower than Mn Ca values measured using total digestion atomic absorption spectrometry (Madkour, 2005; ∼ 30 µmol mol−1). The main difference between the techniques is that LA-ICP-MS (both in this study and in Warter et al., 2015) sampled shell layers where growth lines were visible and did not include pre-treatment, while the total-digestion study (Madkour, 2005) removed organic matter by roasting the shells at 200 ∘C prior to bulk shell analysis. Given that Mn in bivalve shells is typically associated with the mineral fraction of the shell (Soldati et al., 2016), the difference in results may therefore hint at differences between shell layers within tridacnids or differences in Mn concentration between the organic and mineral fractions in the shells. Bivalve shells typically contain between 1 % and 5 % organic matter (Marin and Luquet, 2004), with tridacnid shells being notable for their low organic matter content (< 1 %; Taylor and Layman, 1972; Agbaje et al., 2017). It thus seems unlikely that the differences in Mn Ca ratio between bulk analyses (Madkour, 2005) and in situ analysis (Warter et al., 2015; this study) could originate from variations within the organic matrix, which only constitutes a small fraction of the shell. Therefore, we consider a difference in Mn concentration between shell layers in tridacnids to be more likely. The lack of consistent trace element offsets between the tridacnid species under study here (T. maxima, T. squamosa, and T. squamosina) confirms the chemical similarities between shells from various tridacnid species found in previous studies (e.g., T. gigas – Elliot et al., 2009; Yan et al., 2013; T. crocea – Warter et al., 2018; T. derasa – Sano et al., 2012).
4.3.2 Short-term variability in Sr Ca paced to the day–night cycle
Sr Ca in tridacnids is hypothesized to be strongly controlled by light intensity through a circadian rhythm linked to the day–night cycle (Sano et al., 2012; Warter et al., 2018). This would explain the strong daily periodicity in Sr Ca records through all tridacnids in this study. This daily periodicity may be caused by the ctenidium in tridacnids working in a daily rhythm to keep the acid-base balance in the hemolymph of the clams to offset the CO2 depletion by photosymbionts (which is paced to the day–night cycle of light availability). In the process, Ca2+ channels and Na+ H+ exchangers work to keep the charge balance in the internal fluid and provide nutrients and ions for shell mineralization, letting in compatible trace elements such as Sr2+ (Ip and Chew, 2021). This mechanism follows the biomineralization model by Carré et al. (2006) and is supported by the high affinity of Sr2+ with Ca channels (Hagiwara and Byerly, 1981) and the high ionic fluxes supported by this pathway, allowing enough membrane permeability to support the fast shell formation in tridacnids (Coimbra et al., 1988; Sather and McCleskey, 2003). Following this line of reasoning, the preconcentration of Sr2+ in the extrapallial fluid by Ca channels should have a larger effect on shell Sr Ca ratios in tridacnids than the discrimination against Sr2+ (or other trace elements) by the shell organic matrix during mineralization of the shell from this fluid (as proposed in Gillikin et al., 2005). This model could explain the indirect link between trace element incorporation into the shell of tridacnids and the day–night cycle without a direct causal relationship between trace element concentration and light availability (as demonstrated by the strong daily cycle in trace elements in the shaded TSM1 specimen). It is worth noting that experiments on freshwater bivalves (e.g., Corbicula fluminea; Zhao et al., 2017) revealed that a closure of the Ca2+ channels did not influence Sr concentrations in the shell, arguing against a kinetic effect on Sr partitioning into the shell.
4.3.3 Tidal vs. diurnal variability
Our spectral analysis does not allow us to distinguish between the expression of the solar day (24 h) and the lunar day (∼ 24.8 h) because the width of the bandpass filters used to extract periodicities (∼ 22–36 h) encompass both frequencies. While we cannot exclude the possibility that some of the daily (24 h frequency band) periodicity in tridacnid records is an expression of the lunar cycle, it seems unlikely for most records except Sr Ca because the expression of the other lunar periodicities (most notably the ∼ 12 h cycle) is much weaker in tridacnids compared to in the pectinids (see Figs. 6–7). Nevertheless, it remains possible that the diurnal cycle in Sr Ca in tridacnids, previously interpreted as a response to the day–night cycle, is in fact caused by a circadian rhythm paced to the lunar day. Additionally, vertical mixing, a major driver of sea surface temperature changes in the northern Gulf of Aqaba, is shown to be driven by a combination of surface wind intensity (which has strong daily variability) and the presence of tidal currents (Carlson et al., 2014). It is therefore possible that changes in local surface water temperature partly control the observed (semi-)diurnal variability in trace element concentrations in Red Sea tridacnids.
4.3.4 Seasonal variability and temperature relationships
On longer (seasonal) timescales, Sr Ca in tridacnids has been suggested as a temperature proxy similar to the well-known Sr Ca–sea-surface-temperature relationship in tropical corals (Lough, 2010; Yan et al., 2013). However, significantly lower Sr Ca ratios in tridacnids compared to in coral aragonite (1.5–2.0 mmol mol−1 vs. 7.5–9.5 mmol mol−1 in corals; Elliot et al., 2009; Fig. 2) suggest that tridacnids exert a large degree of biological control on the Sr concentration in their shells, either through the light-sensitive photosymbiosis–calcification relationship outlined above (Sect. 4.3.2) or otherwise through active Sr removal from the biomineralization site by Sr-binding organic molecules (following the model proposed by Gillikin et al., 2005). Similarly, Mg Ca ratios in tridacnids were previously thought to primarily record water temperature (e.g., Batenburg et al., 2011), but our detailed investigation shows large differences in Mg concentration within tridacnid shells, and a strong anticorrelation of Mg with sulfur compounds associated with the organic matrix in the shell (see Sect. 4.2.1; Dauphin et al., 2003) has been put forward as evidence for a strong control of calcification and microstructure over Mg composition in tridacnid shells (Yoshimura et al., 2014). However, evidence from studies on foraminifera calcification demonstrate that the sulfur in biogenic carbonates is not organically bound and that the covariation with Mg might instead be caused by lattice distortion due to incorporation of Mg favoring simultaneous S incorporation (van Dijk et al., 2017). If this is also the case in tridacnids, the Mg Ca–S Ca covariance observed in previous studies might not preclude the use of Mg Ca as a temperature proxy. In that case, more detailed calibration studies (e.g., using controlled-growth experiments; Warter et al., 2018) are required to establish this proxy.
4.3.5 Ba Ca and Mn Ca in tridacnids
As in the pectinids, Ba Ca ratios in tridacnids likely reflect changes in Ba concentration in the environment, which can be caused by upwelling of comparatively nutrient-rich waters or blooms of Ba-rich phytoplankton (Vander Putten et al., 2000; Elliot et al., 2009). Given that Mn is mostly associated with the mineral fraction of bivalve shells and seems to fractionate into the shell close to equilibrium with seawater (Onuma et al., 1979; Soldati et al., 2016), Mn Ca ratios in tridacnids likely reflect the availability of dissolved Mn in the water column, as in other mollusk taxa (e.g., Barats et al., 2009; see Sect. 4.2). This assumption is supported by the correlation between Mn Ca and Ba Ca measured in this study (Fig. 2), suggesting that both records are influenced on seasonal timescales by variability in nutrient availability and redox conditions (sensu Dehairs et al., 1989). Part of this correlation between Mn Ca and Ba Ca is driven by synchronous increases in both elements near the start and end of the profiles through tridacnid shells (Fig. 2). These changes may reflect a decrease of control over shell composition during periods of stress or may alternatively reflect periods of slower growth, which cause more primitive microstructures (characterized by higher concentrations of trace elements) to be formed (Warter et al., 2018).
4.3.6 Environmental changes in the Gulf of Aqaba
Given that the Gulf of Aqaba is oligotrophic and seasonally stratified and lacks significant riverine inputs (Nassar et al., 2014; Manasrah et al., 2019), the variability in nutrient concentrations and redox conditions driving Mn Ca and Ba Ca variability in tridacnids is likely predominantly driven by convective overturning. The tidal amplitude is much smaller than in the Bay of Brest (< 1 m; Manasrah et al., 2019) and is unlikely to drive significant short-term fluctuations in seawater chemistry. This may explain the lack of tidal (12 h) periodicity in Ba Ca and Mn Ca in tridacnids (Figs. 5–6). Nevertheless, tidal rhythms have been observed in the behavior and growth of deep-sea bivalves living far below the direct influence of tides on the environment, proving that such patterns can be recorded by the animals through their circadian rhythm (Schöne and Giere, 2005; Nedoncelle et al., 2013; Mat et al., 2020). In this case, the daily cycle seems to have been more important for Ba Ca in tridacnids, plausibly by driving diurnal changes in primary productivity in the Gulf of Aqaba. Alternatively, the daily periodicity found in tridacnid shell chemistry could be a response to the lunar day (∼ 24.8 h) cycle, which is imprinted in the shell's chemical composition through periodic exposure of the clams to extreme heat or air (subaerial exposure) in their shallow-water environment during exceptionally low tides. The stress induced from this exposure could have affected calcification and incorporation of trace elements (see Sect. 4.3.5).
Interestingly, Sr Ca ratios in tridacnids do exhibit tidal periodicity (Table 2; Fig. 3), perhaps driven by a circadian rhythm in calcification linked to the tidal cycle or by subtle changes in water temperature driven by tidal currents (Carlson et al., 2014). This 12 h periodic behavior is not observed in previous studies of Sr Ca ratios in tridacnids (Sano et al., 2012; Warter et al., 2018). A recent valvometric study on tridacnids found a 12 h period of activity, which supports the hypothesis that a circadian rhythm paced to the tidal cycle could influence shell calcification (Killam et al., 2023). Significant daily fluctuations in solar radiation (up to 1500 W m−2; Manasrah et al., 2019) likely exert control on the calcification of tridacnids in the Red Sea, explaining the strong diurnal periodicity in Sr Ca and Ba Ca records in this study (see Figs. 6 and 8). As in the (non-symbiotic) pectinids, it seems likely that the majority of Mn Ca and Ba Ca variability in tridacnids directly reflects changes in the chemistry of the seawater and its constituents (e.g., particulate organic matter), while Mg Ca and Sr Ca variations are driven by changes in calcification and microstructure. The latter may be indirectly influenced by light intensity through photosynthesis by the symbionts or by circadian-rhythm paces to the diurnal or tidal cycle.
4.4 Role of photosymbiosis in high-frequency chemical variability
4.4.1 Effect of symbiosis on shell formation
While the amplitude of diurnal variability in trace element concentrations does not vary much between the symbiotic tridacnids and the non-symbiotic pectinids (Fig. 6), the amount of variance in the trace element records explained by daily cyclicity is up to twice as high in tridacnids (Fig. 7; Table 3). This suggests that the 24 h cycle has a much larger relative influence on trace element composition (especially Sr Ca and Ba Ca) in tridacnids than in pectinids. This seems to point towards a role of the photosymbionts in calcification by tridacnids, such as through symbiont-mediated diurnal variation in the pH of the extrapallial fluid (Ip et al., 2006), as well as active transport of HCO for calcification (Chew et al., 2019) and as a C supply to the symbionts from the host (Boo et al., 2021). Given the differences in elemental ratios between these two groups of bivalves, comparing variance yields a more robust assessment of the relative importance of tidal or diurnal variability to shell composition than looking at the absolute size (amplitude) of the chemical cycle. While the difference in variance is clear, the importance of diurnal cyclicity to the photosymbiotic tridacnids is not as big as one might expect. It is rare that more than 10 % of the variance is explained by day–night variability (Table 3). This seems to contradict the large daily Sr Ca amplitudes found in Warter et al. (2018) and the trace element fluctuations found in de Winter et al. (2020), which rival the seasonal cycle in these trace element ratios in terms of amplitude. However, the percentages in Table 3 relate to the amount of variation in the complete records through these individuals and therefore also contain areas of the shell where daily cyclicity is less pronounced, while values in previous studies often reflect maximum amplitudes recorded in parts of the shell with exceptionally clear daily increments.
4.4.2 Effect of differences in the environment
It seems plausible that part of the difference in diurnal variability between pectinids and tridacnids is explained by a difference in the environment between the Gulf of Aqaba and the Bay of Brest rather than by the presence of photosymbionts. The diurnal insolation cycle in the Gulf of Aqaba is larger than in the Bay of Brest (1500 vs. 546 W m−2 maximum summer irradiance, respectively; Roberts et al., 2018; Manasrah et al., 2019). If calcification in pectinids were equally sensitive to sunlight, this difference may explain much of the difference between the species. In this scenario, part of the strong tidal component in the pectinid trace element data may be explained by the influence of differences in water depth on the penetration of sunlight through the murky waters of the Bay of Brest (Roberts et al., 2018). Tidal movement can cause strong non-linear amplification or reduction of the solar irradiance at the sea floor of the Bay of Brest by factors exceeding 10, especially outside the summer months, which in turn has a significant effect on primary productivity in the water column (Roberts et al., 2018). This tidal effect is likely to be much weaker in the Gulf of Aqaba, given its comparatively low tidal amplitude, clear oligotrophic waters, and much stronger and less seasonal day–night cycle (Manasrah et al., 2019). Indeed, even in non-photosymbiotic bivalves, light and food availability are demonstrated to be major drivers of the animal's behavior (e.g., Ballesta-Artero et al., 2017). The combination of the effect of daily and tidal cycles on solar irradiance at depth and photosynthesis in the Bay of Brest may therefore pose an alternative pathway for strong tidal cyclicity in the trace element composition of pectinids in this study and account for part of the 2-fold increase in daily variability in tridacnids compared to in the pectinids (Figs. 7–8; Table 3).
4.4.3 Effect of direct insolation
Specimen TSM1 poses an interesting case study for investigating the link between sunlight and calcification in tridacnids since it grew under a sunshade and therefore experienced a dampened diurnal variability in insolation compared to other giant clams in the area. The fact that this specimen exhibits similar or even higher diurnal variability in shell chemistry (Fig. 7) argues against the direct influence of the rate of photosynthesis itself on calcification. Instead, it seems that daily chemical variability is mostly an expression of circadian rhythm in tridacnids, which is strongly (evolutionarily) coupled to the day–night cycle to optimize the symbiosis with primary producers in its mantle, possibly through respiration rhythms carried out by the ctenidium (see Sect. 4.3; Ip and Chew, 2021). Symbionts have been shown to directly aid in calcification through proton pumping (Armstrong et al., 2018), influencing internal acid-base chemistry (Ip et al., 2006), and valvometric studies show the clams bask in sunlight in daylight hours and close partially at night when symbiosis is likely reduced (Schwartzmann et al., 2011; Killam et al., 2023). This conclusion is further supported by the lack of a clear difference in diurnal cyclicity between trace element records in T. maxima, T. squamosa, and T. squamosina (Fig. 7; Table 3) even though the degree of reliance on photosymbiosis is demonstrated to be highly variable between these species (Killam et al., 2020). Therefore, it seems unlikely that sub-daily resolved trace element records in tridacnids can be used as quantitative recorders of paleo-insolation, as was originally suggested by Sano et al. (2012). While the degree of symbiotic activity may not be clearly recorded in the daily amplitude of trace element oscillations, the consistency of daily periodic signal in the studied giant clams could relate to the direct biological control exerted by the symbionts on the hosts' rhythms of calcification. Light exposure in giant clams promotes the expression of gene coding for proteins involved in Ca2+, H+, and HCO transport in the mantles of giant clams (Ip et al., 2017; Chew et al., 2019), with the expression proposed to be at least partially mediated by photosensing on the part of the symbionts themselves (Ip et al., 2017). Differences between the daily consistency (spectral power) of photosymbiotic and non-photosymbiotic trace element profiles might still allow paleontologists to use the presence of strong daily periodicity as a proxy for photosymbiosis in the fossil record (as suggested by de Winter et al., 2020). However, the small differences found between pectinids and tridacnids in this study and the comparatively large influence of environmental variability show that such records should be interpreted with caution. Future studies could measure photosynthetic activity of the symbionts in tridacnids and attempt to relate this to the trace element composition of the shell to isolate the direct effect of photosymbiosis on shell composition.
4.5 Aperiodic drivers of shell chemistry
4.5.1 Circadian and behavioral changes
Even after controlling for instrumental noise, most (∼ 90 %) of the variance observed in our trace element records is not directly related to the diurnal or tidal cycle. This suggests that aperiodic events at the scale of hours to days play an important role in the calcification of pectinids and tridacnids. Given the large difference in ecological niche (e.g., photosymbiotic versus non-symbiotic) between these taxa and the difference between the environments in which they grew, this observation suggests that calcification of bivalves at the (sub-)daily scale is generally dominated by aperiodic variability in calcification or in the environment. Part of this unaccounted variability may be caused by variability in the animal's behavior, as documented by observations of siphon and valve gape activity in cultured or monitored specimens of a variety of bivalve taxa (Rodland et al., 2006; Ballesta-Artero et al., 2017). While these experiments revealed quasi-periodic (3–7 and 60–90 min periods) behavior unassociated with the tidal or daily cycle, records of activity of the bivalves also reveal less regular patterns on the scale of 2–24 h, which may contribute to the aperiodic variance in trace element records (Rodland et al., 2006). Another example of aperiodic behavior potentially influencing shell chemistry is rapid valve adduction or coughing observed in both pectinids and tridacnids, which serves as a mechanism for expelling respiratory CO2 and faeces from the pallial cavity or to evade predation attempts (Robson et al., 2012; Soo and Todd, 2014). This behavior could resuspend sediment and produce pulses of Mn and Ba at the sediment–water interface, which are recorded as short-term, aperiodic variability in these elements in the shell. The temporal sampling resolution (several hours) of our trace element records after smoothing out measurement noise does not allow us to resolve the types of periodic variability at the sub-hourly scale cited in these previous studies, meaning that aperiodic variability in behavior and aliasing of these ultradian patterns likely contribute to the aperiodic variability in our trace element records. On longer (sub-)seasonal timescales, activity in bivalves is shown to be highly dependent on food and light availability (Ballesta-Artero et al., 2017), suggesting that aperiodic, short-term changes in these environmental factors could be a main driver of shell growth and composition and could explain a large part of the variance in the trace element records which is not explained by ultradian changes in the animal's behavior.
4.5.2 Short-term environmental changes and paleoweather
Outside of regular fluctuations caused by tidal, daily, and seasonal cycles, changes in light and food availability at the hourly to daily scale are probably linked to circulation and weather phenomena. Previous studies show that enhanced vertical mixing during weather events such as storms, algal bloom events after wind-driven upwelling, and pseudo-periodic dust deposition can temporarily increase the concentration of dissolved metals in surface waters, resuspend organic matter, and temporarily increase primary productivity (Lin et al., 2003; Al-Najjar et al., 2007; Iluz et al., 2009; Al-Taani et al., 2015; Komagoe et al., 2018). This will in turn lead to a shallowing of the redoxcline through increased organic matter load at the sediment–water interface, which can be recorded in the composition of giant clam shells (Yan et al., 2020). Interestingly, data in Yan et al. (2020) suggest that recording an extreme weather event in Tridacna requires wind speeds exceeding 20 km h−1, a threshold which is almost never reached in the comparatively quiet Gulf of Aqaba (Manasrah et al., 2019), while such events are common in the stormier Bay of Brest (Hily et al., 1992; Chauvaud et al., 2005). This difference is also reflected in the periodicity of shell composition, with the tridacnids having overall higher percentages of their variance explained by daily and tidal variability than pectinids (Figs. 3 and 7), showing that aperiodic (potentially weather-controlled) variability in shell composition has a stronger influence on the pectinids which grew in the stormier Bay of Brest. A plausible scenario therefore emerges in which aperiodic weather events cause short-term variability in both the chemistry and physical properties of the water column. These changes are subsequently recorded in bivalve shells, either directly because the weather events resuspend, remobilize, or deliver trace elements like Mn and Ba (e.g., Dehairs et al., 1989; Gillikin et al., 2008; Mahé et al., 2010) or indirectly because environmental stress associated with the event affects behavior and shell calcification, resulting in a change in the incorporation of alkali group cations (e.g., Mg and Sr) into the shell biomineral (Carré et al., 2006; Takesue et al., 2008; Fig. 8). Our results therefore highlight the potential of high-resolution trace element records in bivalve shells to record short-term circulation changes and weather events while prescribing caution in interpreting such records until the effect of true environmental changes on the sub-daily scale can be separated from aperiodic ultradian or behavioral patterns.
Our high-resolution trace element records reveal that short-term variability on the tidal and daily scale is recorded in the Mg, Sr, Mn, and Ba composition of shells of fast-growing mollusk species. The application of spectral analysis and variance decomposition to these trace element records is a useful tool to assess the influence of periodicity in the shallow marine environment over calcification in mollusk shells. Our statistical analysis reveals that tidal and daily variability, on average, account for less than 10 % of trace element variance in pectinids and tridacnids. In photosymbiotic giant clam shells, the amount of variance in Sr and Ba paced to the daily cycle is 2 times higher than in the non-photosymbiotic pectinids, suggesting that photosymbiosis in giant clams exerts some control over trace element composition in their shells. However, since only ∼ 10 % of the trace element variability in tridacnids is explained by diurnal variability, the recognition of photosymbiosis in the fossil record from diurnal variability in fossil shell composition will be complicated. In addition, differences between the mid-latitude environment of the pectinids and the tropical environment of the tridacnids likely account for part of the difference in trace element composition between the taxa.
We propose that Ba and Mn compositions in pectinids and tridacnids reflect short-term variability in primary productivity and seawater chemistry, which control the mobility of these elements. Concentrations of Mg and Sr are likely controlled by short-term changes in growth and metabolic rate of the mollusks, which may be indirectly controlled by changes in their environment through circadian rhythms or behavior, explaining the pacing of trace element composition to the tidal and diurnal cycle. Most of the variance in trace element records in both taxa is not related to periodic behavior at the 12 or 24 h scale, likely recording aperiodic events in the environment related to weather-scale phenomena or circadian patterns. We thus conclude that mollusk shell carbonate is a promising archive for recording weather-scale variability in shallow marine environments across latitudes and potentially for recording weather-scale phenomena in deep time as long as these environmental effects can be separated by the influence of the behavior of the animal.
Scripts used for data processing and to create figures in this paper were uploaded to an open-access repository on GitHub (https://github.com/nielsjdewinter/TE_circadian, last access: 19 July 2023) and linked through Zenodo (https://doi.org/10.5281/zenodo.6603175, de Winter, 2022).
Supplementary data and figures referenced in this contribution were uploaded to the online open-access repository Zenodo (de Winter, 2023; https://doi.org/10.5281/zenodo.8058451).
NJdW designed the experiment after discussion with BRS, DK, and LF. LF, DK, BRS, and JT collected the samples. LF, DK, and NJdW together prepared samples for analyses and constructed shell chronologies using growth line counting. WB, LdN, GJR, and NJdW carried out the LA-ICP-MS analyses and data processing. NJdW designed and carried out the statistical analyses and wrote the R scripts, guided by feedback from LF, DK, LdN, WB, and GJR. NJdW wrote the first draft of the paper. All the authors contributed to the writing process towards the final version of the paper.
The contact author has declared that none of the authors has any competing interests.
Publisher's note: Copernicus Publications remains neutral with regard to jurisdictional claims in published maps and institutional affiliations.
The authors would like to thank Leonard Bik for his help with the sample preparation and Maarten Zeilmans for his help with the high-resolution imaging of the samples at Utrecht University. This study is part of the UNBIAS project, jointly funded by the Flemish Research Foundation (FWO; grant no. 12ZB220N) post-doctoral fellowship (Niels J. de Winter) and a Marie Skłodowska-Curie Actions (MSCA) Individual Fellowship (grant nos. H2020-MSCA-IF-2018, 843011 – UNBIAS; awarded to Niels J. de Winter). Gert-Jan Reichart and Lennart de Nooijer acknowledge funding from the Netherlands Earth System Science Center (NESSC; grant no. 024.002.001) from the Dutch Ministry for Education, Culture and Science (gravitation grant no. NWO 024.002.001). Bernd R. Schöne acknowledges funding from the Deutsche Forschungsgemeinschaft (DFG; grant nos. SCHO 793/21 (HIPPO) and SCHO 793/23). Julien Thébault was funded by the French National Research Agency (ANR; grant no. ANR-18-CE92-0036-01) awarded within the framework of the French-German collaborative project HIPPO (HIgh-resolution Primary Production multiprOxy archives).
This research has been supported by the European Commission, Horizon 2020 Framework Programme (UNBIAS (grant nos. H2020-MSCA-IF-2018, 843011)); the Fonds Wetenschappelijk Onderzoek (grant no. 12ZB220N); the Aard- en Levenswetenschappen, Nederlandse Organisatie voor Wetenschappelijk Onderzoek (grant no. 024.002.001); the Deutsche Forschungsgemeinschaft (grant nos. SCHO/793/21 and SCHO 793/23); and the Agence Nationale de la Recherche (grant no. ANR-18-CE92-0036-01).
This paper was edited by Steven Bouillon and reviewed by two anonymous referees.
Agbaje, O. B. A., Wirth, R., Morales, L. F. G., Shirai, K., Kosnik, M., Watanabe, T., and Jacob, D. E.: Architecture of crossed-lamellar bivalve shells: the southern giant clam (Tridacna derasa, Röding, 1798), R. Soc. Open Sci., 4, 170622, https://doi.org/10.1098/rsos.170622, 2017.
Al-Aasm, I. S. and Veizer, J.: Diagenetic stabilization of aragonite and low-Mg calcite, I. Trace elements in rudists, J. Sediment. Res., 56, 138–152, 1986a.
Al-Aasm, I. S. and Veizer, J.: Diagenetic stabilization of aragonite and low-Mg calcite, II. Stable isotopes in rudists, J. Sediment. Res., 56, 763–770, 1986b.
Al-Najjar, T., Badran, M. I., Richter, C., Meyerhoefer, M., and Sommer, U.: Seasonal dynamics of phytoplankton in the Gulf of Aqaba, Red Sea, Hydrobiologia, 579, 69–83, https://doi.org/10.1007/s10750-006-0365-z, 2007.
Al-Taani, A. A., Rashdan, M., and Khashashneh, S.: Atmospheric dry deposition of mineral dust to the Gulf of Aqaba, Red Sea: Rate and trace elements, Mar. Pollut. Bull., 92, 252–258, https://doi.org/10.1016/j.marpolbul.2014.11.047, 2015.
Anand, P. and Elderfield, H.: Variability of Mg Ca and Sr Ca between and within the planktonic foraminifers Globigerina bulloides and Globorotalia truncatulinoides, Geochem. Geophy. Geosy., 6, Q11D15, https://doi.org/10.1029/2004GC000811, 2005.
Armstrong, E. J., Roa, J. N., Stillman, J. H., and Tresguerres, M.: Symbiont photosynthesis in giant clams is promoted by V-type H+-ATPase from host cells, J. Exp. Biol., 221, jeb177220, https://doi.org/10.1242/jeb.177220, 2018.
Ballesta-Artero, I., Witbaard, R., Carroll, M. L., and van der Meer, J.: Environmental factors regulating gaping activity of the bivalve Arctica islandica in Northern Norway, Mar. Biol., 164, 116, https://doi.org/10.1007/s00227-017-3144-7, 2017.
Barats, A., Amouroux, D., Pécheyran, C., Chauvaud, L., and Donard, O. F. X.: High-Frequency Archives of Manganese Inputs To Coastal Waters (Bay of Seine, France) Resolved by the LA-ICP-MS Analysis of Calcitic Growth Layers along Scallop Shells (Pecten maximus), Environ. Sci. Technol., 42, 86–92, https://doi.org/10.1021/es0701210, 2008.
Barats, A., Amouroux, D., Chauvaud, L., Pécheyran, C., Lorrain, A., Thébault, J., Church, T. M., and Donard, O. F. X.: High frequency Barium profiles in shells of the Great Scallop Pecten maximus: a methodical long-term and multi-site survey in Western Europe, Biogeosciences, 6, 157–170, https://doi.org/10.5194/bg-6-157-2009, 2009.
Batenburg, S. J., Reichart, G.-J., Jilbert, T., Janse, M., Wesselingh, F. P., and Renema, W.: Interannual climate variability in the Miocene: High resolution trace element and stable isotope ratios in giant clams, Palaeogeogr. Palaeoclimatol. Palaeoecol., 306, 75–81, 2011.
Black, B. A.: Climate-driven synchrony across tree, bivalve, and rockfish growth-increment chronologies of the northeast Pacific, Mar. Ecol. Prog. Ser., 378, 37–46, 2009.
Boo, M. V., Chew, S. F., and Ip, Y. K.: The colorful mantle of the giant clam Tridacna squamosa expresses a homolog of electrogenic sodium: Bicarbonate cotransporter 2 that mediates the supply of inorganic carbon to photosynthesizing symbionts, PloS One, 16, e0258519, https://doi.org/10.1371/journal.pone.0258519, 2021.
Bougeois, L., De Rafélis, M., Reichart, G.-J., De Nooijer, L. J., Nicollin, F., and Dupont-Nivet, G.: A high resolution study of trace elements and stable isotopes in oyster shells to estimate Central Asian Middle Eocene seasonality, Chem. Geol., 363, 200–212, 2014.
Carlson, D. F., Fredj, E., and Gildor, H.: The annual cycle of vertical mixing and restratification in the Northern Gulf of Eilat/Aqaba (Red Sea) based on high temporal and vertical resolution observations, Deep-Sea Res., 84, 1–17, https://doi.org/10.1016/j.dsr.2013.10.004, 2014.
Carré, M., Bentaleb, I., Bruguier, O., Ordinola, E., Barrett, N. T., and Fontugne, M.: Calcification rate influence on trace element concentrations in aragonitic bivalve shells: Evidences and mechanisms, Geochim. Cosmochim. Acta, 70, 4906–4920, https://doi.org/10.1016/j.gca.2006.07.019, 2006.
Chauvaud, L., Lorrain, A., Dunbar, R. B., Paulet, Y.-M., Thouzeau, G., Jean, F., Guarini, J.-M., and Mucciarone, D.: Shell of the Great Scallop Pecten maximus as a high-frequency archive of paleoenvironmental changes, Geochem. Geophy. Geosy., 6, Q08001, https://doi.org/10.1029/2004GC000890, 2005.
Checa, A. G., Esteban-Delgado, F. J., and Rodríguez-Navarro, A. B.: Crystallographic structure of the foliated calcite of bivalves, J. Struct. Biol., 157, 393–402, 2007.
Chew, S. F., Koh, C. Z., Hiong, K. C., Choo, C. Y., Wong, W. P., Neo, M. L., and Ip, Y. K.: Light-enhanced expression of Carbonic Anhydrase 4-like supports shell formation in the fluted giant clam Tridacna squamosa, Gene, 683, 101–112, 2019.
Cochran, J. K., Kallenberg, K., Landman, N. H., Harries, P. J., Weinreb, D., Turekian, K. K., Beck, A. J., and Cobban, W. A.: Effect of diagenesis on the Sr, O, and C isotope composition of late Cretaceous mollusks from the Western Interior Seaway of North America, Am. J. Sci., 310, 69–88, https://doi.org/10.2475/02.2010.01, 2010.
Cohen, A. L., Owens, K. E., Layne, G. D., and Shimizu, N.: The Effect of Algal Symbionts on the Accuracy of Sr Ca Paleotemperatures from Coral, Science, 296, 331–333, https://doi.org/10.1126/science.1069330, 2002.
Coimbra, J., Machado, J., Fernandes, P. L., Ferreira, H. G., and Ferreira, K. G.: Electrophysiology of the Mantle of Anodonta Cygnea, J. Exp. Biol., 140, 65–88, https://doi.org/10.1242/jeb.140.1.65, 1988.
Coimbra, R., Huck, S., de Winter, N. J., Heimhofer, U., and Claeys, P.: Improving the detection of shell alteration: Implications for sclerochronology, Palaeogeogr. Palaeoclimatol. Palaeoecol., 559, 109968, https://doi.org/10.1016/j.palaeo.2020.109968, 2020.
Comboul, M., Emile-Geay, J., Evans, M. N., Mirnateghi, N., Cobb, K. M., and Thompson, D. M.: A probabilistic model of chronological errors in layer-counted climate proxies: applications to annually banded coral archives, Clim. Past, 10, 825–841, https://doi.org/10.5194/cp-10-825-2014, 2014.
Crippa, G., Griesshaber, E., Checa, A. G., Harper, E. M., Roda, M. S., and Schmahl, W. W.: Orientation patterns of aragonitic crossed-lamellar, fibrous prismatic and myostracal microstructures of modern Glycymeris shells, J. Struct. Biol., 212, 107653, https://doi.org/10.1016/j.jsb.2020.107653, 2020.
Dauphin, Y., Cuif, J., Doucet, J., Salomé, M., Susini, J., and Williams, C.: In situ mapping of growth lines in the calcitic prismatic layers of mollusc shells using X-ray absorption near-edge structure (XANES) spectroscopy at the sulphur K-edge, Mar. Biol., 142, 299–304, 2003.
Day, C. C. and Henderson, G. M.: Controls on trace-element partitioning in cave-analogue calcite, Geochim. Cosmochim. Acta, 120, 612–627, https://doi.org/10.1016/j.gca.2013.05.044, 2013.
DeCarlo, T. M. and Cohen, A. L.: Dissepiments, density bands and signatures of thermal stress in Porites skeletons, Coral Reefs, 36, 749–761, https://doi.org/10.1007/s00338-017-1566-9, 2017.
Dehairs, F., Baeyens, W., and Van Gansbeke, D.: Tight coupling between enrichment of iron and manganese in North Sea suspended matter and sedimentary redox processes: Evidence for seasonal variability, Estuar. Coast. Shelf Sci., 29, 457–471, https://doi.org/10.1016/0272-7714(89)90080-2, 1989.
de Winter, N. J.: nielsjdewinter/TE_circadian: First beta (v1.0), Zenodo [code], https://doi.org/10.5281/zenodo.6603175, 2022.
de Winter, N. J.: Supplementary information for: “Ultradian rhythms in shell compositions of photosymbiotic and non-photosymbiotic mollusks”, Zenodo [data set], https://doi.org/10.5281/zenodo.8058451, 2023.
de Winter, N. J. and Claeys, P.: Micro X-ray fluorescence (μXRF) line scanning on Cretaceous rudist bivalves: A new method for reproducible trace element profiles in bivalve calcite, Sedimentology, 64, 231–251, https://doi.org/10.1111/sed.12299, 2017.
de Winter, N. J., Goderis, S., Dehairs, F., Jagt, J. W., Fraaije, R. H., Van Malderen, S. J., Vanhaecke, F., and Claeys, P.: Tropical seasonality in the late Campanian (late Cretaceous): Comparison between multiproxy records from three bivalve taxa from Oman, Palaeogeogr. Palaeoclimatol. Palaeoecol., 485, 740–760, 2017.
de Winter, N. J., Vellekoop, J., Vorsselmans, R., Golreihan, A., Soete, J., Petersen, S. V., Meyer, K. W., Casadio, S., Speijer, R. P., and Claeys, P.: An assessment of latest Cretaceous Pycnodonte vesicularis (Lamarck, 1806) shells as records for palaeoseasonality: a multi-proxy investigation, Clim. Past, 14, 725–749, https://doi.org/10.5194/cp-14-725-2018, 2018.
de Winter, N. J., Goderis, S., Malderen, S. J. M. V., Sinnesael, M., Vansteenberge, S., Snoeck, C., Belza, J., Vanhaecke, F., and Claeys, P.: Subdaily-Scale Chemical Variability in a Torreites Sanchezi Rudist Shell: Implications for Rudist Paleobiology and the Cretaceous Day-Night Cycle, Paleoceanogr. Paleoclimatology, 35, e2019PA003723, https://doi.org/10.1029/2019PA003723, 2020.
de Winter, N. J., Müller, I. A., Kocken, I. J., Thibault, N., Ullmann, C. V., Farnsworth, A., Lunt, D. J., Claeys, P., and Ziegler, M.: Absolute seasonal temperature estimates from clumped isotopes in bivalve shells suggest warm and variable greenhouse climate, Commun. Earth Environ., 2, 1–8, https://doi.org/10.1038/s43247-021-00193-9, 2021a.
de Winter, N. J., Agterhuis, T., and Ziegler, M.: Optimizing sampling strategies in high-resolution paleoclimate records, Clim. Past, 17, 1315–1340, https://doi.org/10.5194/cp-17-1315-2021, 2021b.
de Winter, N. J., Witbaard, R., Kocken, I. J., Müller, I. A., Guo, J., Goudsmit, B., and Ziegler, M.: Temperature Dependence of Clumped Isotopes (Δ47) in Aragonite, Geophys. Res. Lett., 49, e2022GL099479, https://doi.org/10.1029/2022GL099479, 2022.
de Winter, N. J., van Sikkeleras, S., Goudsmit-Harzevoort, B., Boer, W., de Nooijer, L., Reichart, G.-J., Claeys, P., and Witbaard, R.: Tracing timing of growth in cultured molluscs using strontium spiking, Frontiers in Marine Science, 10, 1157929, https://doi.org/10.3389/fmars.2023.1157929, 2023.
Dunbar, R. B. and Wellington, G. M.: Stable isotopes in a branching coral monitor seasonal temperature variation, Nature, 293, 453–455, 1981.
Eggins, S., De Deckker, P., and Marshall, J.: Mg Ca variation in planktonic foraminifera tests: implications for reconstructing palaeo-seawater temperature and habitat migration, Earth Planet. Sc. Lett., 212, 291–306, https://doi.org/10.1016/S0012-821X(03)00283-8, 2003.
Elliot, M., Welsh, K., Chilcott, C., McCulloch, M., Chappell, J., and Ayling, B.: Profiles of trace elements and stable isotopes derived from giant long-lived Tridacna gigas bivalves: potential applications in paleoclimate studies, Palaeogeogr. Palaeoclimatol. Palaeoecol., 280, 132–142, 2009.
Freitas, P. S., Clarke, L. J., Kennedy, H., and Richardson, C. A.: Ion microprobe assessment of the heterogeneity of Mg Ca, Sr Ca and Mn Ca ratios in Pecten maximus and Mytilus edulis (bivalvia) shell calcite precipitated at constant temperature, Biogeosciences, 6, 1209–1227, https://doi.org/10.5194/bg-6-1209-2009, 2009.
Fröhlich, L., Siebert, V., Walliser, E. O., Thébault, J., Jochum, K. P., Chauvaud, L., and Schöne, B. R.: Ba Ca profiles in shells of Pecten maximus – A proxy for specific primary producers rather than bulk phytoplankton, Chem. Geol., 593, 120743, https://doi.org/10.1016/j.chemgeo.2022.120743, 2022.
Gannon, M. E., Pérez-Huerta, A., Aharon, P., and Street, S. C.: A biomineralization study of the Indo-Pacific giant clam Tridacna gigas, Coral Reefs, 36, 503–517, https://doi.org/10.1007/s00338-016-1538-5, 2017.
García-March, J. R., Sanchís Solsona, M. Á., and García-Carrascosa, A. M.: Shell gaping behaviour of Pinna nobilis L., 1758: circadian and circalunar rhythms revealed by in situ monitoring, Mar. Biol., 153, 689–698, https://doi.org/10.1007/s00227-007-0842-6, 2008.
Gilbert, P. U., Bergmann, K. D., Myers, C. E., Marcus, M. A., DeVol, R. T., Sun, C.-Y., Blonsky, A. Z., Tamre, E., Zhao, J., and Karan, E. A.: Nacre tablet thickness records formation temperature in modern and fossil shells, Earth Planet. Sc. Lett., 460, 281–292, 2017.
Gillikin, D. P., Lorrain, A., Navez, J., Taylor, J. W., André, L., Keppens, E., Baeyens, W., and Dehairs, F.: Strong biological controls on Sr Ca ratios in aragonitic marine bivalve shells, Geochem. Geophy. Geosy., 6, Q05009, https://doi.org/10.1029/2004GC000874, 2005.
Gillikin, D. P., Lorrain, A., Paulet, Y.-M., André, L., and Dehairs, F.: Synchronous barium peaks in high-resolution profiles of calcite and aragonite marine bivalve shells, Geo-Mar. Lett., 28, 351–358, 2008.
Goodwin, D. H., Paul, P., and Wissink, C. L.: MoGroFunGen: A numerical model for reconstructing intra-annual growth rates of bivalve molluscs, Palaeogeogr. Palaeoclimatol. Palaeoecol., 276, 47–55, https://doi.org/10.1016/j.palaeo.2009.02.026, 2009.
Guillaume Olivier, M., Leroux, E., Rabineau, M., Le Hir, P., Granjeon, D., Chataigner, T., Beudin, A., and Muller, H.: Numerical modelling of a Macrotidal Bay over the last 9000 years: An interdisciplinary methodology to understand the influence of sea-level variations on tidal currents in the Bay of Brest, Cont. Shelf Res., 231, 104595, https://doi.org/10.1016/j.csr.2021.104595, 2021.
Guillong, M., Meier, D. L., Allan, M. M., Heinrich, C. A., and Yardley, B. W. D.: SILLS: A Matlab-based program for the reduction of laser ablation ICP–MS data of homogenous materials and inclusions, Mineral. Assoc. Can. Short Course, 40, 328–333, 2008.
Hagiwara, S. and Byerly, L.: Calcium channel, Annu. Rev. Neurosci., 4, 69–125, 1981.
Hallmann, N., Schöne, B. R., Strom, A., and Fiebig, J.: An intractable climate archive – Sclerochronological and shell oxygen isotope analyses of the Pacific geoduck, Panopea abrupta (bivalve mollusk) from Protection Island (Washington State, USA), Palaeogeogr. Palaeoclimatol. Palaeoecol., 269, 115–126, 2008.
Hily, C., Potin, P., and Floc'h, J.-Y.: Structure of subtidal algal assemblages on soft-bottom sediments: fauna/flora interactions and role of disburbances in the Bay of Brest, France, Mar. Ecol. Prog. Ser., 85, 115–130, 1992.
Höche, N., Peharda, M., Walliser, E. O., and Schöne, B. R.: Morphological variations of crossed-lamellar ultrastructures of Glycymeris bimaculata (Bivalvia) serve as a marine temperature proxy, Estuar. Coast. Shelf Sci., 237, 106658, https://doi.org/10.1016/j.ecss.2020.106658, 2020.
Höche, N., Walliser, E. O., de Winter, N. J., Witbaard, R., and Schöne, B. R.: Temperature-induced microstructural changes in shells of laboratory-grown Arctica islandica (Bivalvia), PloS One, 16, e0247968, https://doi.org/10.1371/journal.pone.0247968, 2021.
Huyghe, D., de Rafelis, M., Ropert, M., Mouchi, V., Emmanuel, L., Renard, M., and Lartaud, F.: New insights into oyster high-resolution hinge growth patterns, Mar. Biol., 166, 48, https://doi.org/10.1007/s00227-019-3496-2, 2019.
Huyghe, D., Daëron, M., de Rafelis, M., Blamart, D., Sébilo, M., Paulet, Y.-M., and Lartaud, F.: Clumped isotopes in modern marine bivalves, Geochim. Cosmochim. Acta, 316, 41–58, https://doi.org/10.1016/j.gca.2021.09.019, 2021.
Iluz, D., Dishon, G., Capuzzo, E., Meeder, E., Astoreca, R., Montecino, V., Znachor, P., Ediger, D., and Marra, J.: Short-term variability in primary productivity during a wind-driven diatom bloom in the Gulf of Eilat (Aqaba), Aquat. Microb. Ecol., 56, 205–215, https://doi.org/10.3354/ame01321, 2009.
Inoue, M., Nakamura, T., Tanaka, Y., Suzuki, A., Yokoyama, Y., Kawahata, H., Sakai, K., and Gussone, N.: A simple role of coral-algal symbiosis in coral calcification based on multiple geochemical tracers, Geochim. Cosmochim. Acta, 235, 76–88, https://doi.org/10.1016/j.gca.2018.05.016, 2018.
Ip, Y. K. and Chew, S. F.: Light-Dependent Phenomena and Related Molecular Mechanisms in Giant Clam-Dinoflagellate Associations: A Review, Front. Mar. Sci., 8, 627722, https://doi.org/10.3389/fmars.2021.627722, 2021.
Ip, Y. K., Loong, A. M., Hiong, K. C., Wong, W. P., Chew, S. F., Reddy, K., Sivaloganathan, B., and Ballantyne, J. S.: Light induces an increase in the pH of and a decrease in the ammonia concentration in the extrapallial fluid of the giant clam Tridacna squamosa, Physiol. Biochem. Zool., 79, 656–664, 2006.
Ip, Y. K., Koh, C. Z., Hiong, K. C., Choo, C. Y., Boo, M. V., Wong, W. P., Neo, M. L., and Chew, S. F.: Carbonic anhydrase 2-like in the giant clam, Tridacna squamosa: characterization, localization, response to light, and possible role in the transport of inorganic carbon from the host to its symbionts, Physiol. Rep., 5, e13494, https://doi.org/10.14814/phy2.13494, 2017.
Ivany, L. C.: Reconstructing paleoseasonality from accretionary skeletal carbonates – challenges and opportunities, Paleontol. Soc. Pap., 18, 133–166, 2012.
Ivany, L. C. and Judd, E. J.: Deciphering Temperature Seasonality in Earth's Ancient Oceans, Annu. Rev. Earth Planet. Sci., 50, 123–152, https://doi.org/10.1146/annurev-earth-032320-095156, 2022.
Jablonski, D., Roy, K., Valentine, J. W., Price, R. M., and Anderson, P. S.: The Impact of the Pull of the Recent on the History of Marine Diversity, Science, 300, 1133–1135, https://doi.org/10.1126/science.1083246, 2003.
Jablonski, D., Huang, S., Roy, K., and Valentine, J. W.: Shaping the latitudinal diversity gradient: new perspectives from a synthesis of paleobiology and biogeography, Am. Nat., 189, 1–12, 2017.
Jochum, K. P., Willbold, M., Raczek, I., Stoll, B., and Herwig, K.: Chemical Characterisation of the USGS Reference Glasses GSA-1G, GSC-1G, GSD-1G, GSE-1G, BCR-2G, BHVO-2G and BIR-1G Using EPMA, ID-TIMS, ID-ICP-MS and LA-ICP-MS, Geostand. Geoanal. Res., 29, 285–302, https://doi.org/10.1111/j.1751-908X.2005.tb00901.x, 2005.
Jochum, K. P., Weis, U., Stoll, B., Kuzmin, D., Yang, Q., Raczek, I., Jacob, D. E., Stracke, A., Birbaum, K., and Frick, D. A.: Determination of reference values for NIST SRM 610–617 glasses following ISO guidelines, Geostand. Geoanal. Res., 35, 397–429, 2011.
Jones, D. S.: Sclerochronology: reading the record of the molluscan shell: annual growth increments in the shells of bivalve molluscs record marine climatic changes and reveal surprising longevity, Am. Sci., 71, 384–391, 1983.
Jones, D. S. and Quitmyer, I. R.: Marking Time with Bivalve Shells: Oxygen Isotopes and Season of Annual Increment Formation, Palaios, 11, 340–346, https://doi.org/10.2307/3515244, 1996.
Judd, E. J., Wilkinson, B. H., and Ivany, L. C.: The life and time of clams: Derivation of intra-annual growth rates from high-resolution oxygen isotope profiles, Palaeogeogr. Palaeoclimatol. Palaeoecol., 490, 70–83, 2018.
Killam, D., Thomas, R., Al-Najjar, T., and Clapham, M.: Interspecific and Intrashell Stable Isotope Variation Among the Red Sea Giant Clams, Geochem. Geophy. Geosy., 21, e2019GC008669, https://doi.org/10.1029/2019GC008669, 2020.
Killam, D., Al-Najjar, T., and Clapham, M.: Giant clam growth in the Gulf of Aqaba is accelerated compared to fossil populations, Proc. R. Soc. B Biol. Sci., 288, 20210991, https://doi.org/10.1098/rspb.2021.0991, 2021.
Killam, D., Thompson, D., Morgan, K., and Russell, M.: Giant clams as open-source, scalable reef environmental biomonitors, Plos one, 18, e0278752, https://doi.org/10.1371/journal.pone.0278752, 2023.
Killam, D. E. and Clapham, M. E.: Identifying the ticks of bivalve shell clocks: Seasonal growth in relation to temperature and food supply, Palaios, 33, 228–236, https://doi.org/10.2110/palo.2017.072, 2018.
Klein, R. T., Lohmann, K. C., and Thayer, C. W.: Bivalve skeletons record sea-surface temperature and δ18O via Mg Ca and 18O 16O ratios, Geology, 24, 415–418, 1996.
Komagoe, T., Watanabe, T., Shirai, K., Yamazaki, A., and Uematu, M.: Geochemical and Microstructural Signals in Giant Clam Tridacna maxima Recorded Typhoon Events at Okinotori Island, Japan, J. Geophys. Res.-Biogeo., 123, 1460–1474, https://doi.org/10.1029/2017JG004082, 2018.
Kontoyannis, C. G. and Vagenas, N. V.: Calcium carbonate phase analysis using XRD and FT-Raman spectroscopy, Analyst, 125, 251–255, https://doi.org/10.1039/A908609I, 2000.
Ku, H. H.: Notes on the use of propagation of error formulas, J. Res. Natl. Bur. Stand., 70, 263–273, 1966.
Lazareth, C. E., Vander Putten, E., André, L., and Dehairs, F.: High-resolution trace element profiles in shells of the mangrove bivalve Isognomon ephippium: a record of environmental spatio-temporal variations?, Estuar. Coast. Shelf Sci., 57, 1103–1114, 2003.
Lazareth, C. E., Guzman, N., Poitrasson, F., Candaudap, F., and Ortlieb, L.: Nyctemeral variations of magnesium intake in the calcitic layer of a Chilean mollusk shell (Concholepas concholepas, Gastropoda), Geochim. Cosmochim. Acta, 71, 5369–5383, 2007.
Lazier, A. V., Smith, J. E., Risk, M. J., and Schwarcz, H. P.: The skeletal structure of Desmophyllum cristagalli: the use of deep-water corals in sclerochronology, Lethaia, 32, 119–130, 1999.
Lin, I., Liu, W. T., Wu, C.-C., Wong, G. T. F., Hu, C., Chen, Z., Liang, W.-D., Yang, Y., and Liu, K.-K.: New evidence for enhanced ocean primary production triggered by tropical cyclone, Geophys. Res. Lett., 30, 1718, https://doi.org/10.1029/2003GL017141, 2003.
Lorrain, A., Gillikin, D. P., Paulet, Y.-M., Chauvaud, L., Le Mercier, A., Navez, J., and André, L.: Strong kinetic effects on Sr Ca ratios in the calcitic bivalve Pecten maximus, Geology, 33, 965–968, 2005.
Lough, J. M.: Climate records from corals, WIREs Clim. Change, 1, 318–331, https://doi.org/10.1002/wcc.39, 2010.
Madkour, H. A.: Distribution and relationships of heavy metals in the giant clam (Tridacna maxima) and associated sediments from different sites in the Egyptian Red Sea Coast, Egypt. J. Aquat. Res., 31, 45–59, 2005.
Mahé, K., Bellamy, E., Lartaud, F., and de Rafélis, M.: Calcein and manganese experiments for marking the shell of the common cockle (Cerastoderma edule): tidal rhythm validation of increments formation, Aquat. Living Resour., 23, 239–245, https://doi.org/10.1051/alr/2010025, 2010.
Manasrah, R., Abu-Hilal, A., and Rasheed, M.: Physical and Chemical Properties of Seawater in the Gulf of Aqaba and Red Sea, in: Oceanographic and Biological Aspects of the Red Sea, edited by: Rasul, N. M. A. and Stewart, I. C. F., Springer International Publishing, Cham, 41–73, https://doi.org/10.1007/978-3-319-99417-8_3, 2019.
Marin, F. and Luquet, G.: Molluscan shell proteins, C. R. Palevol., 3, 469–492, https://doi.org/10.1016/j.crpv.2004.07.009, 2004.
Mat, A. M., Sarrazin, J., Markov, G. V., Apremont, V., Dubreuil, C., Eché, C., Fabioux, C., Klopp, C., Sarradin, P.-M., Tanguy, A., Huvet, A., and Matabos, M.: Biological rhythms in the deep-sea hydrothermal mussel Bathymodiolus azoricus, Nat. Commun., 11, 3454, https://doi.org/10.1038/s41467-020-17284-4, 2020.
Meibom, A., Stage, M., Wooden, J., Constantz, B. R., Dunbar, R. B., Owen, A., Grumet, N., Bacon, C. R., and Chamberlain, C. P.: Monthly Strontium/Calcium oscillations in symbiotic coral aragonite: Biological effects limiting the precision of the paleotemperature proxy, Geophys. Res. Lett., 30, 1418, https://doi.org/10.1029/2002GL016864, 2003.
Meyers, S. R.: Seeing red in cyclic stratigraphy: Spectral noise estimation for astrochronology, Paleoceanography, 27, PA3228, https://doi.org/10.1029/2012PA002307, 2012.
Meyers, S. R.: Astrochron: An R package for astrochronology, The Comprehensive R Archive Network, http://cran.r-project.org/package=astrochron (last access: 19 July 2023), 2014.
Mohammed, T. A. A., Mohamed, M. H., Zamzamy, R. M., and Mahmoud, M. A. M.: Growth rates of the giant clam Tridacna maxima (Röding, 1798) reared in cages in the Egyptian Red Sea, Egypt. J. Aquat. Res., 45, 67–73, https://doi.org/10.1016/j.ejar.2019.02.003, 2019.
Munro, J. L.: Estimation of the parameters of the von Bertalanffy growth equation from recapture data at variable time intervals, ICES J. Mar. Sci., 40, 199–200, https://doi.org/10.1093/icesjms/40.2.199, 1982.
Nassar, M. Z., Mohamed, H. R., Khiray, H. M., and Rashedy, S. H.: Seasonal fluctuations of phytoplankton community and physico-chemical parameters of the north western part of the Red Sea, Egypt, Egypt. J. Aquat. Res., 40, 395–403, https://doi.org/10.1016/j.ejar.2014.11.002, 2014.
Nedoncelle, K., Lartaud, F., de Rafelis, M., Boulila, S., and Le Bris, N.: A new method for high-resolution bivalve growth rate studies in hydrothermal environments, Mar. Biol., 160, 1427–1439, https://doi.org/10.1007/s00227-013-2195-7, 2013.
Onuma, N., Masuda, F., Hirano, M., and Wada, K.: Crystal structure control on trace element partition in molluscan shell formation, Geochem. J., 13, 187–189, 1979.
Pandolfi, J. M. and Kiessling, W.: Gaining insights from past reefs to inform understanding of coral reef response to global climate change, Curr. Opin. Environ. Sustain., 7, 52–58, https://doi.org/10.1016/j.cosust.2013.11.020, 2014.
Pannella, G.: Tidal growth patterns in recent and fossil mollusc bivalve shells: a tool for the reconstruction of paleotides, Naturwissenschaften, 63, 539–543, 1976.
Petersen, S. V., Tabor, C. R., Lohmann, K. C., Poulsen, C. J., Meyer, K. W., Carpenter, S. J., Erickson, J. M., Matsunaga, K. K., Smith, S. Y., and Sheldon, N. D.: Temperature and salinity of the Late Cretaceous western interior seaway, Geology, 44, 903–906, 2016.
Poitevin, P., Chauvaud, L., Pécheyran, C., Lazure, P., Jolivet, A., and Thébault, J.: Does trace element composition of bivalve shells record utra-high frequency environmental variations?, Mar. Environ. Res., 158, 104943, https://doi.org/10.1016/j.marenvres.2020.104943, 2020.
Polsenaere, P., Deflandre, B., Thouzeau, G., Rigaud, S., Cox, T., Amice, E., Bec, T. L., Bihannic, I., and Maire, O.: Comparison of benthic oxygen exchange measured by aquatic Eddy Covariance and Benthic Chambers in two contrasting coastal biotopes (Bay of Brest, France), Reg. Stud. Mar. Sci., 43, 101668, https://doi.org/10.1016/j.rsma.2021.101668, 2021.
Popov, S. V.: Formation of bivalve shells and their microstructure, Paleontol. J., 48, 1519–1531, https://doi.org/10.1134/S003103011414010X, 2014.
R Core Team: R: A language and environment for statistical computing, R Foundation for Statistical Computing, https://www.r-project.org/ (last access: 19 July 2023), 2013.
Richard, M.: Analyse de la composition élémentaire de Pecten maximus par HR-ICP-MS Element 2: développements méthodologiques et interprétations écologiques, PhD Thesis, Université de Bretagne occidentale-Brest, https://www.theses.fr/2009BRES2031 (last access: 19 July 2023), 2009.
Richardson, C. A., Crisp, D. J., Runham, N. W., and Gruffydd, L. D.: The use of tidal growth bands in the shell of Cerastoderma edule to measure seasonal growth rates under cool temperate and sub-arctic conditions, J. Mar. Biol. Assoc. UK, 60, 977–989, https://doi.org/10.1017/S002531540004203X, 1980.
Richter, C., Roa-Quiaoit, H., Jantzen, C., Al-Zibdah, M., and Kochzius, M.: Collapse of a new living species of giant clam in the Red Sea, Curr. Biol., 18, 1349–1354, 2008.
Roa-Quiaoit, H.: Ecology and culture of giant clams (Tridacnidae) in the Jordanian sector of the Gulf of Aqaba, Red Sea, Universität Bremen, https://elib.suub.uni-bremen.de/diss/docs/E-Diss1340_PHDROAQ.pdf (last access: 19 July 2023), 2005.
Roberts, E. M., Bowers, D. G., and Davies, A. J.: Tidal modulation of seabed light and its implications for benthic algae, Limnol. Oceanogr., 63, 91–106, https://doi.org/10.1002/lno.10616, 2018.
Robson, A. A., Chauvaud, L., Wilson, R. P., and Halsey, L. G.: Small actions, big costs: the behavioural energetics of a commercially important invertebrate, J. R. Soc. Interface, 9, 1486–1498, https://doi.org/10.1098/rsif.2011.0713, 2012.
Rodland, D. L., Schöne, B. R., Helama, S., Nielsen, J. K., and Baier, S.: A clockwork mollusc: Ultradian rhythms in bivalve activity revealed by digital photography, J. Exp. Mar. Biol. Ecol., 334, 316–323, https://doi.org/10.1016/j.jembe.2006.02.012, 2006.
Sano, Y., Kobayashi, S., Shirai, K., Takahata, N., Matsumoto, K., Watanabe, T., Sowa, K., and Iwai, K.: Past daily light cycle recorded in the strontium/calcium ratios of giant clam shells, Nat. Commun., 3, 761, https://doi.org/10.1038/ncomms1763, 2012.
Sather, W. A. and McCleskey, E. W.: Permeation and selectivity in calcium channels, Annu. Rev. Physiol., 65, 133–159, 2003.
Savitzky, A. and Golay, M. J.: Smoothing and differentiation of data by simplified least squares procedures, Anal. Chem., 36, 1627–1639, 1964.
Schöne, B. R. and Giere, O.: Growth increments and stable isotope variation in shells of the deep-sea hydrothermal vent bivalve mollusk Bathymodiolus brevior from the North Fiji Basin, Pacific Ocean, Deep-Sea Res., 52, 1896–1910, 2005.
Schöne, B. R. and Gillikin, D. P.: Unraveling environmental histories from skeletal diaries – Advances in sclerochronology, Palaeogeogr. Palaeoclimatol. Palaeoecol., 373, 1–5, https://doi.org/10.1016/j.palaeo.2012.11.026, 2013.
Schöne, B. R., Castro, A. D. F., Fiebig, J., Houk, S. D., Oschmann, W., and Kröncke, I.: Sea surface water temperatures over the period 1884–1983 reconstructed from oxygen isotope ratios of a bivalve mollusk shell (Arctica islandica, southern North Sea), Palaeogeogr. Palaeoclimatol. Palaeoecol., 212, 215–232, 2004.
Schöne, B. R., Fiebig, J., Pfeiffer, M., Gleß, R., Hickson, J., Johnson, A. L., Dreyer, W., and Oschmann, W.: Climate records from a bivalved Methuselah (Arctica islandica, Mollusca; Iceland), Palaeogeogr. Palaeoclimatol. Palaeoecol., 228, 130–148, 2005a.
Schöne, B. R., Houk, S. D., Castro, A. D. F., Fiebig, J., Oschmann, W., Kröncke, I., Dreyer, W., and Gosselck, F.: Daily growth rates in shells of Arctica islandica: assessing sub-seasonal environmental controls on a long-lived bivalve mollusk, Palaios, 20, 78–92, 2005b.
Schöne, B. R., Dunca, E., Fiebig, J., and Pfeiffer, M.: Mutvei's solution: An ideal agent for resolving microgrowth structures of biogenic carbonates, Palaeogeogr. Palaeoclimatol. Palaeoecol., 228, 149–166, https://doi.org/10.1016/j.palaeo.2005.03.054, 2005c.
Schöne, B. R., Zhang, Z., Jacob, D., Gillikin, D. P., Tütken, T., Garbe-Schönberg, D., and Soldati, A.: Effect of organic matrices on the determination of the trace element chemistry (Mg, Sr, Mg Ca, Sr Ca) of aragonitic bivalve shells (Arctica islandica) – Comparison of ICP-OES and LA-ICP-MS data, Geochem. J., 44, 23–37, 2010.
Schwartzmann, C., Durrieu, G., Sow, M., Ciret, P., Lazareth, C. E., and Massabuau, J.-C.: In situ giant clam growth rate behavior in relation to temperature: A one-year coupled study of high-frequency noninvasive valvometry and sclerochronology, Limnol. Oceanogr., 56, 1940–1951, https://doi.org/10.4319/lo.2011.56.5.1940, 2011.
Service Hydrographique et Océanographique de la Marine – Géoportail: https://www.geoportail.gouv.fr/, last access: 28 June 2022.
Sinclair, D. J., Kinsley, L. P. J., and McCulloch, M. T.: High resolution analysis of trace elements in corals by laser ablation ICP-MS, Geochim. Cosmochim. Acta, 62, 1889–1901, https://doi.org/10.1016/S0016-7037(98)00112-4, 1998.
Soldati, A. L., Jacob, D. E., Glatzel, P., Swarbrick, J. C., and Geck, J.: Element substitution by living organisms: the case of manganese in mollusc shell aragonite, Sci. Rep., 6, 1–9, 2016.
Soo, P. and Todd, P. A.: The behaviour of giant clams (Bivalvia: Cardiidae: Tridacninae), Mar. Biol., 161, 2699–2717, https://doi.org/10.1007/s00227-014-2545-0, 2014.
Surge, D., Lohmann, K. C., and Dettman, D. L.: Controls on isotopic chemistry of the American oyster, Crassostrea virginica: implications for growth patterns, Palaeogeogr. Palaeoclimatol. Palaeoecol., 172, 283–296, 2001.
Takesue, R. K., Bacon, C. R., and Thompson, J. K.: Influences of organic matter and calcification rate on trace elements in aragonitic estuarine bivalve shells, Geochim. Cosmochim. Acta, 72, 5431–5445, 2008.
Tanaka, K., Okaniwa, N., Miyaji, T., Murakami-Sugihara, N., Zhao, L., Tanabe, K., Schöne, B. R., and Shirai, K.: Microscale magnesium distribution in shell of the Mediterranean mussel Mytilus galloprovincialis: An example of multiple factors controlling Mg Ca in biogenic calcite, Chem. Geol., 511, 521–532, https://doi.org/10.1016/j.chemgeo.2018.10.025, 2019.
Taylor, J. D. and Layman, M.: The mechanical properties of bivalve (Mollusca) shell structures, Palaeontology, 15, 73–87, 1972.
Thébault, J., Chauvaud, L., L'Helguen, S., Clavier, J., Barats, A., Jacquet, Sé., PÉcheyran, C., and Amouroux, D.: Barium and molybdenum records in bivalve shells: Geochemical proxies for phytoplankton dynamics in coastal environments?, Limnol. Oceanogr., 54, 1002–1014, https://doi.org/10.4319/lo.2009.54.3.1002, 2009.
Thébault, J., Jolivet, A., Waeles, M., Tabouret, H., Sabarot, S., Pécheyran, C., Leynaert, A., Jochum, K. P., Schöne, B. R., Fröhlich, L., Siebert, V., Amice, E., and Chauvaud, L.: Scallop shells as geochemical archives of phytoplankton-related ecological processes in a temperate coastal ecosystem, Limnol. Oceanogr., 67, 187–202, https://doi.org/10.1002/lno.11985, 2022.
Thomson, D. J.: Spectrum estimation and harmonic analysis, Proc. IEEE, 70, 1055–1096, 1982.
Tierney, J. E., Poulsen, C. J., Montañez, I. P., Bhattacharya, T., Feng, R., Ford, H. L., Hönisch, B., Inglis, G. N., Petersen, S. V., Sagoo, N., Tabor, C. R., Thirumalai, K., Zhu, J., Burls, N. J., Foster, G. L., Goddéris, Y., Huber, B. T., Ivany, L. C., Turner, S. K., Lunt, D. J., McElwain, J. C., Mills, B. J. W., Otto-Bliesner, B. L., Ridgwell, A., and Zhang, Y. G.: Past climates inform our future, Science, 370, eaay3701, https://doi.org/10.1126/science.aay3701, 2020.
Tran, D., Nadau, A., Durrieu, G., Ciret, P., Parisot, J.-P., and Massabuau, J.-C.: Field chronobiology of a molluscan bivalve: how the moon and sun cycles interact to drive oyster activity rhythms, Chronobiol. Int., 28, 307–317, 2011.
Tran, D., Perrigault, M., Ciret, P., and Payton, L.: Bivalve mollusc circadian clock genes can run at tidal frequency, P. R. Soc. B Biol. Sci., 287, 20192440, https://doi.org/10.1098/rspb.2019.2440, 2020.
Vander Putten, E., Dehairs, F., Keppens, E., and Baeyens, W.: High resolution distribution of trace elements in the calcite shell layer of modern Mytilus edulis: Environmental and biological controls, Geochim. Cosmochim. Acta, 64, 997–1011, 2000.
van Dijk, I., de Nooijer, L. J., Boer, W., and Reichart, G.-J.: Sulfur in foraminiferal calcite as a potential proxy for seawater carbonate ion concentration, Earth Planet. Sc. Lett., 470, 64–72, 2017.
Vermeij, G. J.: The evolution of molluscan photosymbioses: a critical appraisal, Biol. J. Linn. Soc., 109, 497–511, 2013.
Von Bertalanffy, L.: Quantitative laws in metabolism and growth, Q. Rev. Biol., 32, 217–231, 1957.
Warter, V. and Müller, W.: Daily growth and tidal rhythms in Miocene and modern giant clams revealed via ultra-high resolution LA-ICPMS analysis – A novel methodological approach towards improved sclerochemistry, Palaeogeogr. Palaeoclimatol. Palaeoecol., 465, 362–375, 2017.
Warter, V., Müller, W., Wesselingh, F. P., Todd, J. A., and Renema, W.: Late Miocene Seasonal To Subdecadal Climate Variability In The Indo-West Pacific (East Kalimantan, Indonesia) Preserved In Giant Clams, Palaios, 30, 66–82, https://doi.org/10.2110/palo.2013.061, 2015.
Warter, V., Erez, J., and Müller, W.: Environmental and physiological controls on daily trace element incorporation in Tridacna crocea from combined laboratory culturing and ultra-high resolution LA-ICP-MS analysis, Palaeogeogr. Palaeoclimatol. Palaeoecol., 496, 32–47, https://doi.org/10.1016/j.palaeo.2017.12.038, 2018.
Wassenburg, J. A., Scholz, D., Jochum, K. P., Cheng, H., Oster, J., Immenhauser, A., Richter, D. K., Häger, T., Jamieson, R. A., Baldini, J. U. L., Hoffmann, D., and Breitenbach, S. F. M.: Determination of aragonite trace element distribution coefficients from speleothem calcite–aragonite transitions, Geochim. Cosmochim. Ac., 190, 347–367, https://doi.org/10.1016/j.gca.2016.06.036, 2016.
Wichern, N. M. A., de Winter, N. J., Johnson, A. L. A., Goolaerts, S., Wesselingh, F., Hamers, M. F., Kaskes, P., Claeys, P., and Ziegler, M.: The fossil bivalve Angulus benedeni benedeni: a potential seasonally resolved stable-isotope-based climate archive to investigate Pliocene temperatures in the southern North Sea basin, Biogeosciences, 20, 2317–2345, https://doi.org/10.5194/bg-20-2317-2023, 2023.
Wilson, S. A., Koenig, A. E., and Orklid, R.: Development of microanalytical reference material (MACS-3) for LA-ICP-MS analysis of carbonate samples, Geochim. Cosmochim. Acta Suppl., 72, A1025, https://ui.adsabs.harvard.edu/abs/2008GeCAS..72R1025W/abstract (last access: 19 July 2023), 2008.
Wisshak, M., Correa, M. L., Gofas, S., Salas, C., Taviani, M., Jakobsen, J., and Freiwald, A.: Shell architecture, element composition, and stable isotope signature of the giant deep-sea oyster Neopycnodonte zibrowii sp. n. from the NE Atlantic, Deep-Sea Res., 56, 374–407, 2009.
Witbaard, R., Jenness, M. I., Van Der Borg, K., and Ganssen, G.: Verification of annual growth increments in Arctica islandica L. from the North Sea by means of oxygen and carbon isotopes, Neth. J. Sea Res., 33, 91–101, https://doi.org/10.1016/0077-7579(94)90054-X, 1994.
Xing, Q., Zhang, L., Li, Y., Zhu, X., Li, Y., Guo, H., Bao, Z., and Wang, S.: Development of Novel Cardiac Indices and Assessment of Factors Affecting Cardiac Activity in a Bivalve Mollusc Chlamys farreri, Front. Physiol., 10, 293, https://doi.org/10.3389/fphys.2019.00293, 2019.
Yan, H., Shao, D., Wang, Y., and Sun, L.: Sr Ca profile of long-lived Tridacna gigas bivalves from South China Sea: A new high-resolution SST proxy, Geochim. Cosmochim. Acta, 112, 52–65, https://doi.org/10.1016/j.gca.2013.03.007, 2013.
Yan, H., Liu, C., An, Z., Yang, W., Yang, Y., Huang, P., Qiu, S., Zhou, P., Zhao, N., Fei, H., Ma, X., Shi, G., Dodson, J., Hao, J., Yu, K., Wei, G., Yang, Y., Jin, Z., and Zhou, W.: Extreme weather events recorded by daily to hourly resolution biogeochemical proxies of marine giant clam shells, P. Natl. Acad. Sci. USA, 117, 7038–7043, https://doi.org/10.1073/pnas.1916784117, 2020.
Yoshimura, T., Suzuki, A., Tamenori, Y., and Kawahata, H.: Micro-X-ray fluorescence-based comparison of skeletal structure and P, Mg, Sr, O and Fe in a fossil of the cold-water coral Desmophyllum sp., NW Pacific, Geo-Mar. Lett., 34, 1–9, 2014.
Zhao, L., Schöne, B. R., and Mertz-Kraus, R.: Controls on strontium and barium incorporation into freshwater bivalve shells (Corbicula fluminea), Palaeogeogr. Palaeoclimatol. Palaeoecol., 465, 386–394, https://doi.org/10.1016/j.palaeo.2015.11.040, 2017.