the Creative Commons Attribution 4.0 License.
the Creative Commons Attribution 4.0 License.
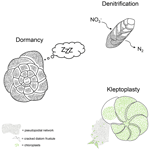
Benthic foraminifera and gromiids from oxygen-depleted environments – survival strategies, biogeochemistry and trophic interactions
Nicolaas Glock
The oceans are losing oxygen (O2), and oxygen minimum zones are expanding due to climate warming (lower O2 solubility) and eutrophication related to agriculture. This trend is challenging for most marine taxa that are not well adapted to O2 depletion. For other taxa this trend might be advantageous because they can withstand low O2 concentrations or thrive under O2-depleted or even anoxic conditions. Benthic foraminifera are a group of protists that include taxa with adaptations to partly extreme environmental conditions. Several species possess adaptations to O2 depletion that are rare amongst eukaryotes, and these species might benefit from ongoing ocean deoxygenation. In addition, since some foraminifera can calcify even under anoxic conditions, they are important archives for paleoceanographic reconstruction in O2-depleted environments. This paper reviews the current state of knowledge about foraminifera from low-O2 environments. Recent advances in our understanding of specific survival strategies of foraminifera to withstand O2 depletion are summarized and discussed. These adaptations include an anaerobic metabolism, heterotrophic denitrification, symbiosis with bacteria, kleptoplasty and dormancy and have a strong impact on their preferred microhabitat in the sediments, especially the ability of some benthic foraminiferal species to denitrify. Benthic foraminifera also differ regarding their trophic strategies, which has an additional impact on the selection of their microhabitat. For example, some species are strict herbivores that feed exclusively on fresh phytodetritus and live close to the sediment surface, while some species are non-selective detrivores that occupy intermediate to deep infaunal habitats. There is evidence that foraminifers have the capacity to undergo phagocytosis, even under anoxia, and some foraminiferal species which can withstand low-O2 conditions seem to prey on meiofauna. Also, due to their high abundances in O2-depleted environments and their metabolic adaptations, benthic foraminifera are key players in marine nutrient cycling, especially within the marine N and P cycles. This review summarizes the denitrification rates for the species that are known to denitrify and the intracellular nitrate concentrations of the species that are known to intracellularly store nitrate. Finally, equations are provided that can be used to estimate the intracellular nutrient storage and denitrification rates of foraminifera and might be integrated into biogeochemical models.
Please read the corrigendum first before continuing.
-
Notice on corrigendum
The requested paper has a corresponding corrigendum published. Please read the corrigendum first before downloading the article.
-
Article
(4252 KB)
-
The requested paper has a corresponding corrigendum published. Please read the corrigendum first before downloading the article.
- Article
(4252 KB) - Full-text XML
- Corrigendum
-
Supplement
(289 KB) - BibTeX
- EndNote
- Included in Encyclopedia of Geosciences
More than 2 decades have passed since Bernhard and Sen Gupta (1999) provided a comprehensive review about the history of research on foraminifera from O2-depleted environments. About a decade later, Koho and Piña-Ochoa (2012) published another overview about benthic foraminifera as inhabitants of low-O2 habitats, mainly focusing on the species distribution in different environments and the different depth layers in the sediment. They also summarized the early work on foraminiferal denitrification, kleptoplasty and evidence for bacterial symbiosis. Nevertheless, advances in methods to analyze the metabolic rates, intracellular nitrate storage and molecular genetics of foraminifera have changed our understanding of strategies such as an anaerobic metabolism that help them to withstand O2 depletion. This paper aims to summarize these developments, mainly focusing on benthic foraminifera. For the discussion about life in habitats where O2 is scarce or absent it is important to define the range of O2 concentration for terms such as anoxia, hypoxia, and suboxic and oxic conditions. The concentration range for these terms varies with the literature. To avoid confusion, this review only uses the following definitions from the literature:
-
Anoxia usually indicates the complete absence of O2 ([O2] = 0 µM; Diaz, 2016).
-
Suboxic conditions indicate habitats where O2 is low enough that denitrification and Mn and Fe reduction are present, but sulfide concentrations are still low due to the absence of sulfate reduction ([O2] ∼1–10 µM; Oakley et al., 2007).
-
Hypoxia in aquatic environments indicates habitats where O2 is present, but the O2 saturation is less than 30 %, since most fish cannot survive below 30 % saturation ([O2] < 62.5 µM Levin et al., 2009).
-
Low-O2 or O2-depleted habitats summarize all environments that fulfill one of the above definitions (i.e., every environment where [O2] is <62.5 µM).
Knowledge about planktic foraminifera from O2-depleted habitats is scarce compared to the knowledge about benthic foraminifera. Nonetheless, at least two species (Globorotaloides hexagonus and Hastigerina parapelagica) are known to live in pelagic oxygen minimum zones (OMZs) (Davis et al., 2021). As a result, G. hexagonus has proven to be a valuable paleo-indicator for the presence of pelagic OMZs during the Pliocene (Davis et al., 2023). Benthic foraminifera from low-O2 environments have also been established as an invaluable archive for paleoceanography. However, this review summarizes redox proxies based on benthic foraminifera only briefly, since there is work in progress to give a comprehensive review about proxies for O2 concentrations in paleoceanography (Hoogakker et al., 2023). Due to their ability to precipitate their calcitic tests even under anoxic conditions, fossil benthic foraminifera became routine tools in paleoceanography to reconstruct past redox conditions (Nardelli et al., 2014; Orsi et al., 2020). Some morphological adaptations are very common for benthic foraminifera that thrive in O2-depleted habitats. Small, more elongated and flattened morphologies are often characteristic for O2 depletion, while more spherical forms can indicate oxygenated conditions (Bernhard, 1986; Bernhard et al., 1997). In addition, high porosity and thin test walls seem to be characteristic for foraminifera that live in low-O2 environments (Kaiho, 1994). The porosity, including pore size and pore density, of foraminiferal tests recently received more attention as a possible paleoceanographic tool. Different foraminiferal species seem to adapt their pore characteristics in a different way to environmental conditions. Cibicides spp. for example mainly thrive in well-oxygenated environments (Mackensen et al., 1995), and the porosity in epifaunal Cibicides spp. and Planulina spp. is significantly negatively correlated with the O2 concentrations in the bottom water (Rathburn et al., 2018; Glock et al., 2022). If O2 is too depleted, these foraminifers increase their porosity to optimize the O2 uptake. Furthermore, the mechanism of biomineralization in foraminifera can preserve the chemical signature of ambient seawater in their test calcite. These species precipitate their test calcite directly from vacuolized seawater (Erez, 2003; de Nooijer et al., 2014; Toyofuku et al., 2017), and thus the chemical composition of the test calcite reflects the chemical composition of the surrounding water in their habitats. Different ratios are used as a proxy for various parameters. Over the past decades several redox-sensitive ratios in foraminiferal calcite were identified as potential O2 proxies, where (Reichart et al., 2003; Barras et al., 2018; Brinkmann et al., 2021) and (e.g., Zhou et al., 2014, 2022; Lu et al., 2016; Glock et al., 2019d; Winkelbauer et al., 2021; Cook et al., 2022) are amongst the most prominent examples. The offset of the stable-carbon-isotope fractionation (δ13C) between the tests of epifaunal and deep-infaunal benthic foraminifera can also be used as a quantitative [O2]BW proxy (e.g., McCorkle and Emerson, 1988; Schmiedl and Mackensen, 2006; Hoogakker et al., 2014, 2018). Finally, species compositions of benthic foraminifera assemblages are used to reconstruct past environmental conditions. Kaiho et al. (1994) developed the first benthic foraminifera O2 index (BFOI). Further development of this index is still ongoing, with recent developments by Tetard et al. (2021) and Kranner et al. (2022).
The first part of the present paper reviews recent advances in our understanding of the diverse strategies that foraminifera use to withstand O2 depletion, focusing mainly on denitrification, dormancy and kleptoplasty. The part about foraminiferal denitrification also incorporates denitrification into the conceptual TROX model of Jorissen et al. (1995). The TROX model explains the sediment microhabitats of benthic foraminifera in terms of an interplay between the supply of O2 and non-refractory organic matter that can be used as food. The next section briefly summarizes the knowledge about ecological and trophic interactions of foraminifera from O2-depleted environments. Finally, the role of foraminifera in marine biogeochemical cycling is discussed, with a focus on nitrogen and phosphorous cycling.
1.1 Survival strategies
Some benthic foraminiferal species have very specific adaptations that provide the opportunity either to thrive in anoxia or at least to survive periods of O2 depletion (see examples in Fig. 1).
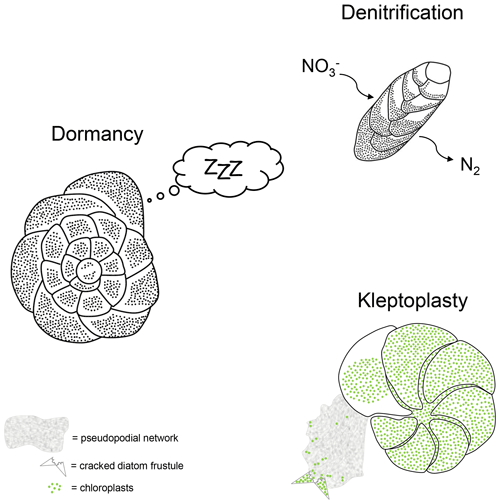
Figure 1Schematic representations for three survival strategy examples performed by benthic foraminifera under O2-depleted conditions.
1.1.1 Foraminiferal denitrification
More than a decade ago the first evidence emerged that some foraminifera from O2-depleted environments are able to perform complete denitrification (Risgaard-Petersen et al., 2006). Heterotrophic denitrification describes the step-by-step reduction of nitrate (NO to inert N2 gas (Reaction R1 according to Jorgensen, 2006, and Fig. 2).
where [CH2O] symbolizes organic matter of unspecified composition.
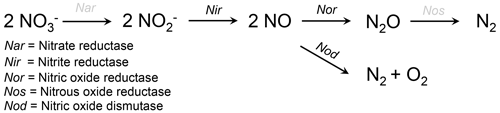
Figure 2Schematic view of two alternative pathways suggested for foraminiferal denitrification. Abbreviations above the reaction arrows indicate the enzymes that are catalyzing the respective step (see legend). Enzymes in black have been found transcribed by eukaryotic (foraminiferal) RNA (Woehle and Roy et al., 2018). Enzymes in gray are missing in the foraminiferal denitrification pathway and are likely performed by bacterial symbionts (Woehle and Roy et al., 2022). The straight pathway above describes the normal heterotrophic denitrification pathway. The junction, catalyzed by the nitric oxide dismutase, which produces O2, has been suggested as an alternative pathway for foraminiferal denitrification (Woehle and Roy et al., 2018).
Heterotrophic denitrification provides energy to an organism for oxidative phosphorylation in a similar way to O2 respiration. The ΔG0 for heterotrophic denitrification per mole of carbon at a pH of 7 is −453 kJ mol−1, which is slightly less efficient O2 respiration (ΔG kJ mol−1 according to Jorgensen, 2006).
The discovery of foraminiferal denitrification by Risgaard-Petersen et al. (2006) was also the first evidence for complete denitrification in eukaryotic cells in general, and it showed that they likely take up NO from the surrounding pore water and store it within intracellular seawater vacuoles. Nevertheless, no later study could actually prove a bona fide “complete” denitrification pathway in foraminifera, and the eukaryotic foraminiferal denitrification pathway is considered today to be incomplete (Woehle et al., 2018; Orsi et al., 2020; Gomaa et al., 2021; see discussion below). Other eukaryotes that are known to perform incomplete denitrification are the primitive eukaryote Loxodes (Finlay et al., 1983) and two species of fungi (Usuda et al., 1995).
Four years after the study by Risgaard-Petersen et al. (2006), Pina-Ochoa et al. (2010b) documented that intracellular NO storage and denitrification are not an exception, limited to a few specialized foraminiferal species, but actually a widespread phenomenon. Within a couple of years more studies either quantified denitrification rates or the intracellular NO storage of various foraminifera and gromiid species (Høgslund et al., 2008; Glud et al., 2009; Piña-Ochoa et al., 2010b, a; Koho et al., 2011; Bernhard et al., 2012b). The intracellular NO storage can reach concentrations up to 567 mM in gromiids (Piña-Ochoa et al., 2010b), and experiments with isotopically labeled NO showed that Globobulimina turgida takes up NO at a similar rate, independently of the presence or magnitude of the intracellular NO pool (Koho et al., 2011). It has been hypothesized that at least some denitrifying foraminifera seem to take up NO through the pores in their tests, and the pore density (number of pores per area) of some denitrifying species, such as Bolivina spissa, turned out to be a promising proxy for quantitative NO reconstructions (Glock et al., 2011, 2018). However, not all benthic foraminifera are able to denitrify, even if they live in environments that are periodically exposed to anoxia such as representatives of the intertidal species morphogroup Ammonia tepida (either Ammonia veneta, Ammonia aberdoveyensis or Ammonia confertitesta according to Hayward et al., 2021), which neither store NO nor show any denitrification activity (Piña-Ochoa et al., 2010b). Some foraminifera from the Bering Sea have been shown to store NO but did not denitrify in incubation experiments (Langlet et al., 2020). These species include Nonionella pulchella, Uvigerina peregrina and Bolivinellina pseudopunctata. Also, the NO storage in U. peregrina shows a high variability, depending on the environment. Individuals of U. peregrina from the Bay of Biscay lack significant NO storage, while U. peregrina from the North Sea and the Bering Sea both show intracellular NO enrichment (Piña-Ochoa et al., 2010b; Langlet et al., 2021). Other Uvigerina and Nonionella species have been shown to denitrify (Risgaard-Petersen et al., 2006; Høgslund et al., 2008; Piña-Ochoa et al., 2010b; Glock et al., 2019b; Gomaa et al., 2021). Many miliolids and allogromiids, several intertidal rotaliid species, and also some other rotaliids and textulariids completely lack intracellular NO storage (Piña-Ochoa et al., 2010b).
The observations that some species store NO and denitrify in some environments and not in others might have two reasons. One reason could be that these species belong to an opportunistic group of foraminifera that can adapt well to both oxygenated environments where they respire O2 and do not denitrify and O2-depleted environments where they switch to denitrification. The other reason could be that some of these foraminifera belong to morphogroups that are identified as a single species but indeed are a mixture of cryptic and pseudocryptic species that include denitrifying and non-denitrifying species. An example for such a morphogroup that has recently had a revision is A. tepida. This morphogroup includes three species (A. veneta, A. aberdoveyensis and A. confertitesta) that can now be morphologically distinguished (Richirt et al., 2019; Hayward et al., 2021). A similar case concerns the morphogroup Nonionella stella, where representatives have been found to denitrify (Høgslund et al., 2008; Choquel et al., 2021). The morphogroup N. stella also consists of several cryptic to pseudocryptic species (Deldicq et al., 2019). The situation might be similar with other Nonionella species and the widespread species U. peregrina.
There is strong evidence for symbiosis between foraminifera and prokaryotes in many hosts from O2-depleted environments, which is most likely an adaptation to survive within the steep geochemical gradients close to the oxic–anoxic boundary (Bernhard et al., 2000, 2006, 2018; Bernhard, 2003; Nomaki et al., 2014). Most of the observed prokaryotic associates are endobionts within the foraminiferal cytoplasm, but some are ectobionts that are often observed close to the pores in the foraminiferal shell (Bernhard et al., 2001, 2010a, 2018). For about a decade after the first discovery of foraminiferal denitrification it remained unclear if foraminifera indeed denitrify themselves or if the bacterial symbionts are responsible for the denitrification. Evidence came up for both hypotheses. Bernhard et al. (2012b) showed that Bolivina argentea consumed its intracellular NO storage in O2-free incubations even after a very harsh treatment with antibiotics, which indicates that this species can denitrify even when the activity of potential bacterial symbionts would be inhibited. Other studies showed that bacterial endobionts likely perform denitrification in some allogromiid foraminifera and gromiid species (Bernhard et al., 2012a; Høgslund et al., 2017). Gromiida are a separate group of protists within the Rhizaria and are closely related to foraminifera. With the recent advances in molecular biology, however, it became possible to analyze the transcriptome of denitrifying foraminifera, and Woehle and Roy et al. (2018) showed that the enzymes responsible for denitrification in Globobulimina spp. from an O2-depleted Swedish fjord basin are indeed transcribed by eukaryotic RNA. These enzymes are homologues of enzymes that are also used by bacteria for denitrification, which indicates an ancient prokaryotic origin of denitrification in foraminifera. Nevertheless, the homologues of the enzymes that catalyze the first and the last step of foraminiferal denitrification (reduction of NO to nitrite (NO and reduction of nitrous oxide (N2O) to N2 gas; Fig. 2) have not been identified yet. This indicates that foraminifera use other enzymes to catalyze these steps, that they rely on bacterial symbionts for these steps or that they use an alternative denitrification pathway in general.
One hypothesis, brought up by Woehle and Roy et al. (2018), is that the homologue of the nitric oxide reductase (Nor) is indeed a nitric oxide dismutase that has been proposed to catalyze the enzymatic reaction (alternative pathway in Fig. 2) (Ettwig et al., 2012). The presence of the eukaryotic denitrification pathway found in foraminifera (Woehle and Roy et al., 2018) has been confirmed through other analyses of foraminiferal transcriptomes (Orsi et al., 2020; Gomaa et al., 2021). Gomaa et al. (2021) also identified an enzyme of yet unknown functionality that might be responsible for the first step in the foraminiferal denitrification pathway. Recent metagenomics and transcriptomic results of denitrifying foraminifera indicate that bacterial symbionts might perform the missing steps in the foraminiferal denitrification pathway or that they at least partly contribute to the amount of NO that is denitrified within foraminiferal cells (Woehle and Roy et al., 2022). It has already been hypothesized before that the ectobionts, found on Bolivina pacifica from the Santa Barbara Basin, are either sulfate-reducing or sulfur-oxidizing bacteria (Bernhard et al., 2010a). The possible complementation of the foraminiferal denitrification with bacterial symbionts appears to be contradictory to the results of Bernhard et al. (2012b), who showed that B. argentea consumed its intracellular NO storage (likely for denitrification) even after the antibiotic treatment. Gomaa et al. (2021) confirmed that B. argentea also lacks the first and last denitrification step in its transcriptome, although it lacks intracellular bacterial symbionts (Bernhard et al., 2012b). Future studies might decipher if indeed bacteria are responsible for the missing denitrification step and are immune to such antibiotic treatment; if an oxygenic nitric oxide dismutase skips the last denitrification step, as discussed by Woehle and Roy et al. (2018); and/or if foraminifera have unknown enzymes that catalyze the missing steps, as suggested by Gomaa et al. (2021). The study by Woehle and Roy et al. (2022) also showed that the last common ancestor of denitrifying foraminifera likely has its origin during the Cretaceous, possibly related to the occurrence of the Cretaceous anoxic events. Since the foraminiferal denitrification pathway is incomplete, and the first and last steps might be performed by Desulfobacteraceae in their microbiome, the authors suggested that the acquisition of denitrification ability in foraminifera occurred in multiple stages (starting during the Cretaceous) but is not yet complete (Woehle and Roy et al., 2022).
It is noteworthy that denitrifying foraminifera from the Peruvian OMZ show a metabolic preference for NO over O2 as an electron acceptor (Glock et al., 2019b). These foraminifera show an increasing cell volume with increasing ambient NO and decreasing O2 concentrations. Similar observations have been made at the California Borderland, where some benthic foraminifera also increase their cell volume with decreasing ambient O2 concentrations (Keating-Bitonti and Payne, 2017). Additional evidence for the metabolic NO preference came from comparing denitrification and O2 respiration rates and scaling them to their cell volume (Glock et al., 2019b). The scaling is lower for O2 respiration than for denitrification, indicating that the NO metabolism during denitrification is more efficient than the O2 metabolism during aerobic respiration in foraminifera from the Peruvian OMZ. This might explain why some infaunal denitrifying foraminifera follow the oxycline within sediments (Linke and Lutze, 1993; Duijnstee et al., 2003). We have to keep in mind that O2 can be quite harmful for organisms that are not adapted to higher O2 concentrations due to its strong reactivity. Even trace amounts of O2 can inhibit denitrification, and O2 can repress the denitrifying enzyme synthesis (Smith and Tiedje, 1979; Knowles, 1981; Tiedje, 1988; Mckenney et al., 1994). Thus, if denitrifying foraminifera are exposed to small amounts of O2 they cannot denitrify but also do not have enough O2 to supply their demands for electron acceptors. Larger amounts of O2 might supply this demand but also harm the cell. For example, O2 can inhibit the growth of some obligate anaerobes' poison enzymes that are important for their metabolism (Lu and Imlay, 2021). Also, for aerobes, O2 can be harmful. “Hyperoxia”, an excess supply of O2, leads to damaging effects by highly reactive metabolic products of O2 (free O2 radicals) that inactivate enzymes in the cell, damage DNA and destroy lipid membranes (Frank and Massaro, 1980). Furthermore, foraminifera are able to store NO within vacuoles due to its lower reactivity and still have an electron acceptor reservoir if NO is depleted in their microhabitat. This is not possible for O2 due to its high reactivity (Auten and Davis, 2009). Finally, a review by Zimorski et al. (2019) addresses the common misconception that the presence of O2 improves the overall energetic state of the cell. It is a fact that the energy yield from remineralizing glucose or amino acids is higher in the presence of O2 (“O2 respiration”), but it is also a fact that the synthesis of biomass consumes 13 times more energy per cell if O2 is present compared to anoxic conditions. This is related to the chemical equilibrium between organic matter and CO2, which strongly shifts to the side of CO2 in the presence of O2 (Zimorski et al., 2019). All this might explain why the metabolism of at least some foraminifera is better adapted to denitrification than to O2 respiration.
The circumstance that some foraminifera have a metabolic preference for NO over O2 as an electron acceptor (Glock et al., 2019b) and that other species like U. peregrina denitrify in some environments but completely lack intracellular NO storage in others (Piña-Ochoa et al., 2010b) might partly explain the microhabitat selectivity of benthic foraminifera in the sediment. According to the conceptual TROX model, benthic foraminifera can be divided into groups due to their microhabitat preference: epifauna, shallow infauna, intermediate infauna and deep infauna (Jorissen et al., 1995). The presence of this species-specific microhabitat structure was first documented by Corliss (1985). These microhabitats are mainly controlled by bottom-water O2 concentrations and the supply of non-refractory organic matter (i.e., food; Jorissen et al., 1995). Due to our increasing understanding about the anaerobic metabolism of foraminifera we can now assume that NO availability is another controlling factor (Fig. 3). This is also indicated by a study that coupled early diagenetic modeling with foraminiferal ecology to model the microhabitats of benthic foraminifera (Jorissen et al., 2022). According to their metabolic preference for NO or O2 as electron acceptors, many benthic foraminifera species that typically occupy a certain microhabitat (epifauna, shallow infauna and deep infauna) might partly be assigned to three different attributes (aerobe, facultative anaerobe and facultative aerobe). There are most likely exceptions to these classifications, which are discussed below. Another controlling factor of the microhabitat is the specific trophic strategy of the foraminiferal species, which is further discussed in Sect. 3.
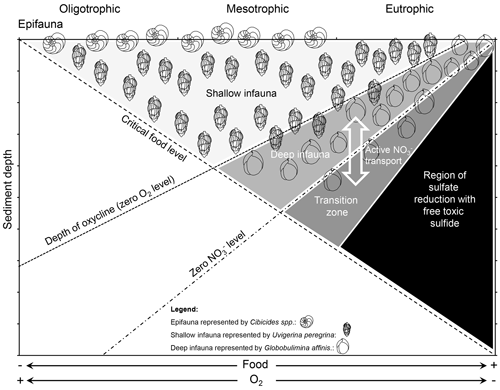
Figure 3TROX model modified after Jorissen et al. (1995) and Xu et al. (2021). The supply of organic matter and bottom-water O2 and NO concentrations in different environments control the penetration depth of O2 and NO into the sediment. Benthic foraminifera choose their microhabitat according to their metabolic preferences for O2 or NO as an electron acceptor and the availability of food. Intermediate infauna is not specifically schematized in the figure but peaks between the shallow and deep infauna with an overlap in both directions. Note that denitrifying foraminifera can actively transport intracellular NO below the NO penetration depth in the sediments. The deeper regions where production of free sulfide occurs will mainly be avoided. For further details see the text.
Deep-infaunal species can most likely be considered to be facultative aerobes that have a metabolic preference for NO over O2 (Glock et al., 2019b) and try to avoid trace amounts of O2. They cannot be counted as obligate anaerobes though since they can withstand periods of oxygenation. Many experiments show that denitrifying foraminifera can switch to O2 respiration if they are exposed to O2 (i.e., Piña-Ochoa et al., 2010b). Still, they follow the oxycline in the sediments to avoid the inhibition of denitrification by trace amounts of O2. The δ13C signature of shells of deep-infaunal globobuliminids indicates that they calcify in sediment depth where the pore water O2 level reaches zero or even deeper in the sediments. The offset between δ13C of Globobulimina spp. tests and δ13C of epifaunal foraminifera or of bottom-water dissolved inorganic carbon (DIC) is nearly equal to the offset between DIC at the zero-O2 layer and the bottom water (Schmiedl and Mackensen, 2006) and often can be even higher (Costa et al., 2023), indicating that many globobuliminids live even below the oxycline. Even though they can switch to O2 respiration (Piña-Ochoa et al., 2010b), these species would most likely try to avoid crossing the oxycline since denitrification would already be inhibited by O2 concentrations within the nanomolar range (Dalsgaard et al., 2014), and the O2 concentration slightly above the oxycline is not high enough to fulfill their metabolic demands. Indeed, the model by Jorissen et al. (2022) describes the distribution of deep infauna very well by using the presence of O2 as an inhibiting factor, which also suggests that they can be considered facultative aerobes instead of facultative anaerobes. Taxa belonging to the deep-infaunal group that might be considered to be facultative aerobes that prefer NO over O2 include, for example, Valvulineria inflata and bradyana, Bolivina seminuda, Globobulimina pyrula, Globobulimina affinis, and Cancris carmenensis (Jorissen et al., 1995; Schmiedl and Mackensen, 2006; Mojtahid et al., 2010; Glock et al., 2019b).
Shallow infauna can in many cases be considered to be facultative anaerobes that are well adapted to the presence of low O2 concentrations but can switch to denitrification if they are exposed to anoxic conditions or need to enter the deeper sediment parts to find food or avoid competitive stress. These species have the advantage that they can utilize both fresh phytodetritus from the top of the sediments and organic matter of lower quality from the deeper parts of the sediments. A good example for a shallow-infaunal–facultative anaerobe species is U. peregrina, which is well known for its shallow-infaunal lifestyle (Schmiedl and Mackensen, 2006) and has been found with and without intracellular NO storage in different environments (Piña-Ochoa et al., 2010b; Langlet et al., 2020). Of course, it cannot be generalized that all foraminifera from a shallow infaunal habitat are indeed facultative anaerobes. At least some species that can be considered shallow-infaunal have been shown neither to be able to store NO nor to denitrify. As mentioned above, all specimens from the Ammonia tepida morphogroup that have been analyzed so far lack intracellular NO storage and cannot denitrify (Piña-Ochoa et al., 2010b). Nevertheless, these taxa are often exposed to anoxia and can sometimes even be found alive in 4 to 26 cm sediment depth (Alve and Murray, 2001; Thibault de Chanvalon et al., 2015). It is possible that these foraminifera indeed only have an aerobe metabolism and just become dormant under exposure to anoxia (dormancy is discussed in another section). However, another possibility is that intertidal species such as A. veneta, A. aberdoveyensis and A. confertitesta have other adaptations to anoxia than denitrification. Recent studies revealed other possible anaerobic metabolic pathways in foraminifera such as fermentation and dephosphorylation of creatine phosphate, which are discussed in Sect. 2.1.3 (Orsi et al., 2020; Gomaa et al., 2021). Also, an Ammonia sp. has been shown to take up nitrogen from 15N-labeled NO under O2 depletion and to assimilate it within cell organelles known as electron dense bodies (Nomaki et al., 2016). Eventually, studies on the transcriptome of non-denitrifying species from infaunal environments might be able to show if some of these species can switch to an alternative anaerobe metabolism under exposure to anoxia.
Many epifaunal species can most likely be considered to be aerobes that typically occur at the sediment–water interface or on elevated surfaces. Typical epifaunal–aerobe taxa include Cibicides spp. and Planulina spp. (Corliss and Chen, 1988; Lutze and Thiel, 1989). These species have the advantage that they are well adapted to collect fresh food supply from above (Wollenburg et al., 2021) but usually cannot withstand longer O2-depleted periods (Mackensen et al., 1995). Nevertheless, recent genetic data indicate that Cibicidoides wuellerstorfi clusters very close to known denitrifying species in the phylogenetic tree, so it cannot be excluded that some Cibicides spp. may denitrify under certain circumstances (Woehle and Roy et al., 2022). In the same way as for the other microhabitats, not all species with an epifaunal lifestyle should be automatically considered to be aerobes. There are examples of epifaunal benthic foraminifera that have not been found in well-oxygenated environments but reach high abundances in O2-depleted environments. One example is Epistominella smithi, which has been described in low-O2 environments, such as the Santa Barbara Basin (Harman, 1964) or the Peruvian OMZ (Erdem and Schönfeld, 2017). Nevertheless, the morphology of E. smithi strongly suggests an epifaunal lifestyle. Another example is the epifaunal species Planulina limbata. This species is abundant only in O2-depleted environments on continental margins within the eastern Pacific (Natland, 1938; Erdem and Schönfeld, 2017; Glock et al., 2022). Recent P. limbata specimens are present in severely O2-depleted water masses within the Peruvian OMZ ([O2] = 3–12 µmol kg−1; Glock et al., 2022). Nevertheless, P. limbata also adapts its pore density to the availability of O2 (Glock et al., 2022), which might indicate that it has an aerobic metabolism, despite the fact that its presence appears to be limited to low-O2 environments. Another possibility is that species such as E. smithi or P. limbata may denitrify under certain circumstances and therefore can also be considered to be facultative anaerobes. Hopefully, measurements of metabolic rates, intracellular nutrient content and enzymatic activity might bring further evidence in the future if at least some epifaunal species can switch to an anaerobe metabolism when O2 is too depleted.
Table 1Summary of foraminiferal denitrification rates (individual and volume-specific), where Ind. refers to the number of individuals used for one incubation. Individual denitrification rates refer to average rates per individual, while specific denitrification rates refer to rates normalized to the biovolume of the foraminifers. Specimens earlier identified as A. tepida are likely either A. aberdoveyensis or A. confertitesta according to Hayward et al. (2021).
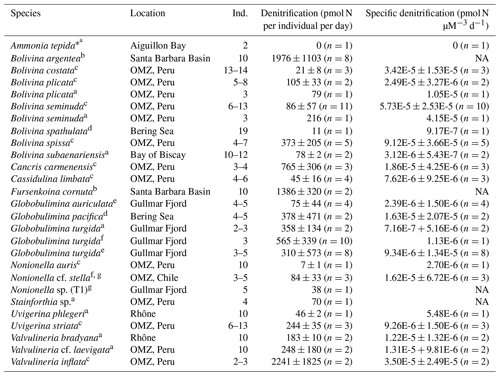
Errors are given as standard deviations (1 SD). a Data from Piña-Ochoa et al. (2010b), b data from Bernhard et al. (2012b), c data from Glock et al. (2019b), d data from Langlet et al. (2020), e data from Woehle and Roy et al. (2018), f data from Risgaard-Petersen et al. (2006), g data from Choquel et al. (2021), h data from Høgslund et al. (2008). ∗ Ammonia tepida is a morphogroup of pseudocryptic species that recently had a revision.
The intermediate infauna is somehow an exceptional case. Common representatives of intermediate-infaunal taxa are Melonis barleeanus and Pullenia spp. (Corliss, 1991). The typical example for intermediate-infaunal species, M. barleeanus, is interesting since it stores either no or only very small amounts of NO (see Tables 2 and 3). Still, several studies indicate that M. barleeanus lives deeper in the sediments than some Uvigerina spp. (Corliss, 1991; Ní Fhlaithearta et al., 2018), although many Uvigerina species have been shown to store NO and denitrify (Tables 1 and 2). This might give room to speculate if M. barleeanus has other metabolic adaptations to O2 depletion than denitrification or if it simply does not store large amounts of NO but denitrifies NO directly after the uptake from the seawater. Indeed, a recent study predicted the microhabitats of infaunal benthic foraminifera using an early diagenetic model and showed that the intermediate-infaunal clusters around the NO maximum in the pore water (Jorissen et al., 2022). Future perspectives on understanding the biology of intermediate infauna might include transcriptome analyses to decipher other anaerobe metabolic pathways and testing the denitrification capacity after incubation in NO-free and NO-containing seawater.
Note that the deep infauna can even migrate deeper into the sediments below the depth of NO penetration if they must due to their ability to intracellularly store NO as a reservoir (Fig. 3). The deeper boundary of the deep infauna might be controlled by the zone of sulfate reduction, where free sulfide is produced, which could be toxic for the foraminifers. Research to measure denitrification rates in different benthic foraminiferal species continues (Langlet et al., 2020; Choquel et al., 2021). This will add to the scarce available data and contribute to estimates of the role of foraminifera in oceanic N cycling. This topic is discussed a bit further in Sect. 4.
Table 2Summary of intracellular nitrate (NO storage in benthic foraminifera and gromiids from different environments. Only species where intracellular [NO] was at least 0.1 mM are listed. Species with intracellular [NO mM are listed in Table 3.
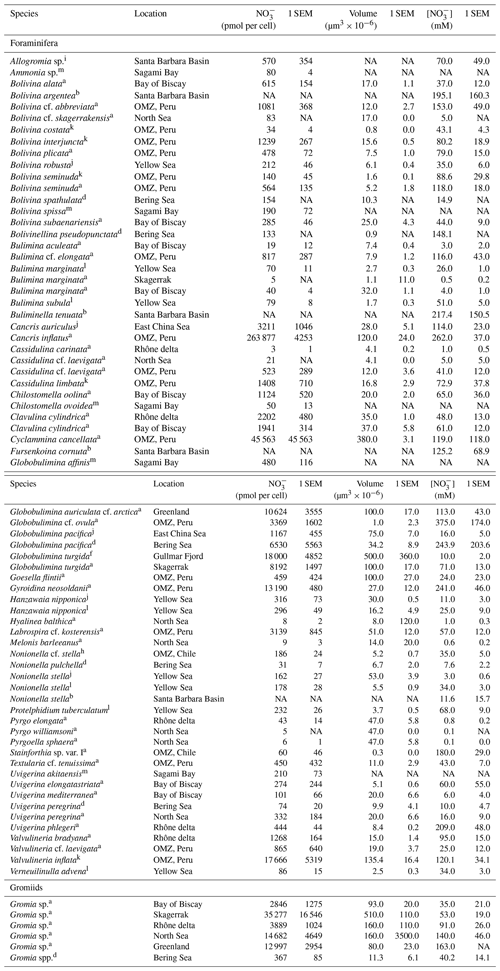
Errors are given as standard error of the mean (SEM). a Data from Piña-Ochoa et al. (2010b), b data from Bernhard et al. (2012b), d data from Langlet et al. (2020), f data from Risgaard-Petersen et al. (2006), h data from Høgslund et al. (2008), i data from Bernhard et al. (2012a), j data from Xu et al. (2017), k data from Glock et al. (2020), l data from Xu et al. (2021), m data from Nomaki et al. (2015). NA: not available.
1.1.2 Kleptoplasty
Kleptoplasty describes a symbiosis between algal chloroplasts and a host organism that sequesters the chloroplasts from algae (Clark et al., 1990). The word originates from the Greek word Kleptes, which means “thief”. Kleptoplasty in foraminifera is most extensively studied for shallow Elphidium and Haynesina species that often thrive within the photic zone, and this research originated in the 1970s (Lopez, 1979; Lee et al., 1988; Correia and Lee, 2000, 2002b, a; Goldstein et al., 2004; Pillet et al., 2011, 2013; Cevasco et al., 2015; Jauffrais et al., 2016, 2017, 2018; Cesbron et al., 2017; Goldstein and Richardson, 2018; Jesus et al., 2021). Several studies showed that the sequestered chloroplasts in the intertidal species Haynesina germanica are still capable of photosynthesis under light exposure (Lopez, 1979; Cesbron et al., 2017). H. germanica often shares a habitat with species from the Ammonia tepida morphogroup (Ammonia aberdoveyensis or Ammonia confertitesta according to Hayward et al., 2021), which also tend to ingest chloroplasts, but these chloroplasts do not show any photosynthetic activity anymore (Jauffrais et al., 2016).
Table 3Summary of benthic foraminifera from different environments that lack intracellular nitrate (NO storage. Only species with intracellular [NO mM are listed. Species in bold letters have been found to store NO in other environments (see Table 2). Specimens earlier identified as A. tepida are likely either A. aberdoveyensis or A. confertitesta according to Hayward et al. (2021).
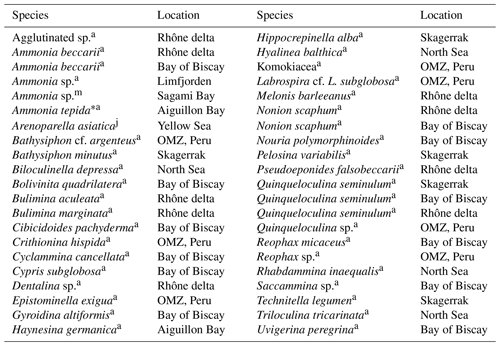
a Data from Piña-Ochoa et al. (2010b), j data from Xu et al. (2017), m data from Nomaki et al. (2015). ∗ Ammonia tepida is a morphogroup of pseudocryptic species that recently had a revision.
The kleptoplasts in foraminifera originate from diatoms, which has been confirmed on the basis of the chloroplast shape in transmission electron microscopy (TEM) observations and by sequencing the chloroplasts using molecular-biological methods (Lopez, 1979; Lee et al., 1988; Cedhagen, 1991; Lee and Anderson, 1991; Bernhard and Bowser, 1999; Grzymski et al., 2002; Goldstein et al., 2004). Austin et al. (2005) hypothesized that the tooth plates in H. germanica are morphological adaptations to crack diatom frustules for access to their chloroplasts. Recently, LeKieffre et al. (2018) showed in (aerated) incubation experiments with H13CO and 15NH during a light–dark cycle that Haynesina germanica is indeed able to fix inorganic carbon and nitrogen under light exposure. Intertidal foraminifera are often exposed to O2-depleted or even anoxic conditions when water stagnates during low tide or if they are transported to deeper anoxic sediment layers by bioturbation (Rybarczyk et al., 1996; Cesbron et al., 2017). Oxygen penetration depths in tidal flats can vary from a few millimeters during low tide to several centimeters during high tide (Jansen et al., 2009). Thus, intertidal foraminifera are often exposed to anoxia, even within the first centimeter of the sediment column. H. germanica is also supposed to occur in black sediments of the British salt marsh tide pools (Bernhard and Bowser, 1999), which likely become anoxic during tidal cycles (Rybarczyk et al., 1996), and it was among the first recolonizers of a fjord suffering from organic pollution (Cato et al., 1980; Bernhard and Bowser, 1999). Kleptoplasty might thus be an additional adaptation of foraminifera from photic environments to stay active during periods of O2 depletion, which has already been hypothesized by Cesbron et al. (2017).
Less well understood is the phenomenon of kleptoplasty, observed in the benthic foraminifers Nonionella stella, Virgulina fragilis and Nonionellina labradorica, which can thrive below the photic zone and often inhabit O2-depleted sediments (Cedhagen, 1991; Bernhard and Bowser, 1999; Grzymski et al., 2002; Bernhard, 2003; Tsuchiya et al., 2015; Jauffrais et al., 2019; Gomaa et al., 2021; Powers et al., 2022). Experiments to test if N. labradorica is able to photosynthesize with its sequestered chloroplasts have been inconclusive. While Cedhagen (1991) found active photosynthesis in N. labradorica specimens incubated with 14C, Jauffrais et al. (2019) showed an increased O2 respiration rate instead of O2 production and chloroplast degradation in specimens exposed to light. Recently, Gomaa et al. (2021) found chloroplast encoded in transcripts of N. stella, indicating that the kleptoplasts in this species are still active. Genetic analyses revealed that the kleptoplasts in N. stella and N. labradorica are also mainly sequestered from diatoms, most likely after ingestion and selective digestion of phytodetritus (Grzymski et al., 2002; Jauffrais et al., 2019; Gomaa et al., 2021). Grymzki et al. (2002) calculated that the required amount of light for N. stella specimens collected from aphotic depths at the Santa Barbara Basin is too low to sustain active photosynthesis. Instead, they suggested that the kleptoplasts in foraminifera from aphotic environments provide the ability to fix inorganic nitrogen via the glutamine synthetase and glutamate 2-oxoglutarate amidotransferase (GOGAT) pathway. Indeed, Jauffrais et al. (2019) showed that kleptoplastic N. labradorica are able to fix inorganic nitrogen, but coupled TEM–nanoscale secondary ion mass spectrometry (nanoSIMS) revealed that the assimilated nitrogen is associated with electron opaque bodies instead of sequestered chloroplasts. Analyses of the transcriptome of N. stella by Gomaa et al. (2021) support the observations by Grimzki et al. (2002), since N. stella appears to be able to fix ammonia by itself. They also found that the fucoxanthin–chlorophyll binding protein (FCP) was expressed in the transcriptome of N. stella and speculated that the ability to synthesize FCP was derived from the kleptoplasts by horizontal gene transfer. FCP is a pigment, commonly found in chloroplasts of brown algae, and allows a more efficient photosynthesis with a light absorption bandwidth, which is especially useful in aquatic environments (Papagiannakis et al., 2005; Premvardhan et al., 2008). The true function of the kleptoplasts in deep-sea benthic foraminifera from aphotic, often O2-depleted environments still remains enigmatic, though.
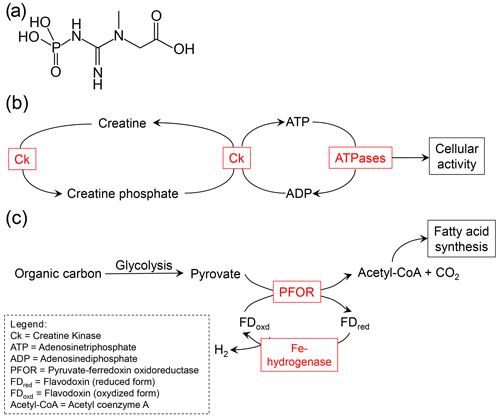
Figure 4Examples for molecules and processes that are relevant in the anaerobic metabolism of foraminifera. (a) Structural formula of creatine phosphate. (b) The role of creatine kinase (Ck) and creatine phosphate in the anaerobic metabolism. High-energy creatine phosphate is produced by phosphorylation of creatine. Creatine phosphate can rapidly recycle adenosine diphosphate (ADP) to adenosine triphosphate (ATP) to provide resources for rapid energy bursts. This pathway has been described by Orsi et al. (2020). (c) Fermentation has been found to be relevant in the anaerobic metabolism of foraminifera by both Orsi et al.(2020) and Gomaa et al. (2021). The possibility of a H2-producing fermentation pathway, catalyzed by Fe-hydrogenase, has been described by Gomaa et al. (2021).
1.1.3 Other strategies: fermentation, utilization of high-energy phosphates and peroxisome proliferation
Several recent publications based on advances in molecular-biological methods (e.g., next generation sequencing) have revealed some other metabolic adaptations of foraminifera that thrive under O2 depletion (see examples in Fig. 4) (Woehle and Roy et al., 2018, 2022; Orsi et al., 2020; Gomaa et al., 2021). In N. stella and Bolivina argentea, Gomaa et al. (2021) found evidence for the expression of proteins, including pyruvate:ferredoxin oxidoreductase (PFOR) and [FeFe]-hydrogenase, which are characteristic of anaerobic metabolism. These PFOR sequences were indeed eukaryotic and closely related to those of the facultative anaerobe polychaete Capitella teleta and the anaerobic protistan parasite Blastocystis. The [FeFe]-hydrogenase is very similar to those in the amoeba/flagellate Naegleria gruberi, which has experimentally been shown to be active and to produce molecular hydrogen even under aerobic conditions (Tsaousis et al., 2014). Due to these observations, Gomaa et al. (2021) suggested that N. stella and B. argentea might be able to produce H2 gas and have the capacity for an anaerobic energy metabolism.
Another important observation was made by Orsi et al. (2020). They used metatranscriptomics on sediments from the Namibian shelf, where the foraminiferal community is dominated by Bolivina and Stainforthia species. Presumably living foraminifera were present in the sediment column up to 28 cm depth in an anoxic habitat with high sulfide concentrations. The gene expression of those foraminifers increased under sulfidic conditions, which indicates that they not only survive but thrive under anoxic conditions. The anaerobic energy metabolism of these foraminifers seems to be sufficient enough to support calcification and phagocytosis even under anoxic conditions. Evidence for foraminiferal calcification under anoxia already came up in a study by Nardelli et al. (2014). Orsi et al. (2020) suggested that the Namibian foraminifera use phagocytosis (vacuolic ingestion of food particles) to ingest prey cells even under anoxic conditions. These processes (calcification and the ingestion of prey cells by phagocytosis) require bursts of high energy, which the authors suggest is generated by dephosphorylation of intracellular creatine phosphate storage to regenerate ATP from ADP. Evidence for the capacity for the dephosphorylation of creatine phosphate under anoxia was indicated by the metatranscriptomes. In addition, high intracellular dissolved inorganic phosphate storage has been found in benthic foraminifera from the Peruvian OMZ, which might serve as a reservoir to synthesize creatine phosphate and/or to synthesize polyphosphates that might be broken down to harvest energy (Glock et al., 2020). Orsi et al. (2020) and Gomaa et al. (2021) also found evidence for another anaerobic metabolism. Their data indicate that the foraminifers metabolize hydrolyzed organics to produce ATP using fermentation and fumarate reduction.
Most foraminifera species from O2-depleted habitats possess numerous peroxisomes that are usually associated with mitochondria and the endoplasmatic reticulum (Bernhard and Bowser, 2008). Bernhard and Bowser (2008) hypothesized that these peroxisome proliferations might be used to either metabolize H2O2 and other highly reactive oxygen species that are produced within the chemocline close to the oxic–anoxic boundary or to reduce the oxidative stress by these compounds. Indeed, they showed in an experiment that ATP concentrations in foraminifera increased proportionally to ambient H2O2 concentrations. A recent study on the transcriptome and metatranscriptome of N. stella and B. argentea from the Santa Barbara Basin revealed that these species utilize an adaptable mitochondrial and peroxisomal metabolism, depending on the chemical treatment in the experiment (Powers et al., 2022). The high plasticity of their peroxisomal and mitochondrial metabolism might be substantial for survival under the highly variable conditions at the chemocline in the sediments. The results of Powers et al. (2022) indicate that at least some processes that are involved in foraminiferal denitrification are associated with mitochondria. Interestingly, the expression of denitrification-related genes in both species was upregulated after incubation with elevated H2O2 but without NO and downregulated if they were incubated without H2O2 but with NO, compared to a control treatment with both H2O2 and NO. In the same way several peroxisomal processes were upregulated in the H2O2-only treatment. In addition, despite the fact that both species are able to denitrify, Powers et al. (2022) found distinct metabolic adaptations to anoxia in both species. For example, a quinol:fumarate oxidoreductase, which is considered to be an adaptive mechanism for anaerobic respiration in eukaryotic organisms, was present in N. stella but not in B. argentea. On the other hand, B. argentea has the capacity to digest food vacuole contents under O2 depletion, while N. stella was lacking food vacuoles (Powers et al., 2022).
1.1.4 Dormancy
Dormancy is another strategy to survive anoxia or extreme O2 depletion for some benthic foraminifera that cannot denitrify. Dormancy is defined as the reduced or suspended metabolic activity in response to exogenous factors (Ross and Hallock, 2016). Observations that indicate the potential of dormancy in foraminifera have been documented since the 1950s and are extensively reviewed by Ross and Hallock (2016). Nevertheless, many aspects of foraminiferal dormancy, such as its role in the foraminiferal life cycle or its role in structuring foraminiferal assemblages, remain unexplored (Ross and Hallock, 2016).
In the 1990s some studies suggested that some foraminifera may become dormant when exposed to anoxia. Bernhard and Alve (1996) observed that the ATP concentration ([ATP]) of the benthic foraminiferal species Bulimina marginata, Stainforthia fusiformis and Adercotryma glomerata flushed with N2 gas to drive out O2 was significantly lower than in specimens from well-aerated conditions. They interpreted this observation as an indication that dormancy is a survival strategy for some foraminiferal species when they are exposed to periods of anoxia. Linke and Lutze (1993) observed cysts of Elphidium incertum from putative anoxic habitats that might be interpreted as a sign for dormancy, and Hannah and Rogerson (1997) hypothesized that foraminifera transported to an anoxic sediment layer might become dormant until they return to aerated conditions by transport through bioturbation.
Recently, dormancy of foraminifera exposed to anoxia has gained more attention again. LeKieffre et al. (2017) did a feeding experiment with specimens from the Ammonia tepida morphogroup (A. confertitesta according to Koho et al., 2018, and Hayward et al., 2021) using a 13C-labeled diatom film as a food source. They compared the metabolic differences in Ammonia sp. between oxic and anoxic conditions by mapping the distribution of 13C within the cells using coupled TEM–nanoSIMS and by analyzing the carbon concentration and stable-carbon-isotope composition of the total organic matter and individual fatty acids in the foraminifer. Nearly the complete diatom biofilm was consumed, and the foraminiferal cytoplasm was strongly enriched in 13C under oxic conditions. Specimens from the anoxic incubation ingested only few of the diatoms, and those were neither assimilated nor metabolized further. In addition, the specimens from the oxic incubation produced a significant quantity of specific polyunsaturated fatty acids, which was not the case under anoxic conditions. A. confertitesta reacted to the induced anoxia with a severely reduced metabolic rate within less than 24 h. All these observations provide solid evidence that dormancy is a survival strategy of A. confertitesta under anoxia.
Koho et al. (2018) further analyzed cell structural changes in Ammonia spp. under exposure to anoxia collected from the field as well as from incubations. The specimens from anoxia showed an increase in lipid droplets and electron dense bodies within their cytoplasm. The cytoplasm itself was thinned out, which was interpreted as metabolization of the cytosol. In addition, while absent within the specimens from oxic environments, various bacteria were present within the cytoplasm of the specimens from anoxia. These were interpreted as endobionts but might also be parasites that could not be fended off due to the drastically reduced metabolism during dormancy under anoxia. A continuum of intracellular bacteria including prey in food vacuoles, endobionts, parasites and necrophages has been documented before in benthic foraminifera from cold seeps (Bernhard et al., 2010b). It has already been hypothesized by the authors that bacteria can switch their function from endobionts to predators, depending on the vitality of the host cell. Considering all the studies about dormancy, it is likely that dormancy is a common survival strategy for foraminiferal species whose supply of suitable electron acceptors is exhausted (i.e., O2 or NO or who are exposed to periods of extreme environmental conditions. Since there is evidence for dormancy in both S. fusiformis and B. marginata (Bernhard and Alve, 1996), it is likely that even denitrifying species can become dormant under unfavorable conditions. Another Stainforthia sp. has been shown to denitrify, and B. marginata stores NO in some environments (Piña-Ochoa et al., 2010b).
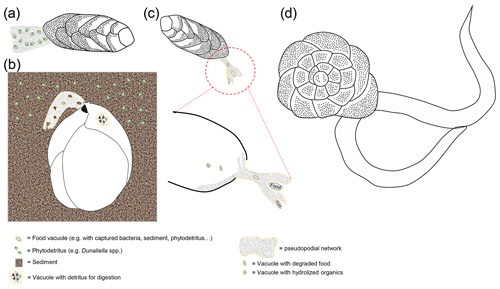
Figure 5(a) Schematic representation of a bolivinid ingesting bacterial cells. Recent studies have shown that benthic foraminifera from O2-depleted habitats have the capacity to undergo phagocytosis even under anoxia (Orsi et al., 2020). (b) Schematic representation of Ammonia sp. preying on a nematode. Some benthic foraminifera are known to prey on meiofauna (Dupuy et al., 2010), and there is evidence that even some globobuliminids that usually thrive under O2-depleted conditions might prey on nematodes (Glock et al., 2019a).
In general, benthic foraminifera show a wide range of trophic strategies. Gooday et al. (2008) suggested that they can be separated according to their main trophic types (see examples in Fig. 5): A – selective herbivores, which include phytophagous species that consume only phytodetritus; B – seasonal herbivores, which feed on fresh phytodetritus when available and consume sedimentary organic matter at other times; C – detrivores that non-selectively ingest sediment and consume the present degraded organic matter, bacteria and/or other organisms; D – selective bacterivores that consume only bacteria; and E – suspension feeders that either arise from the sediments or occur on elevated substrates. The latter two are not discussed in detail, since they mainly apply to abyssal species that inhabit more oxygenated environments. Nevertheless, some Cibicides and Planulina species can also inhabit environments with relatively low O2 concentrations (Erdem and Schönfeld, 2017; Rathburn et al., 2018; Hoogakker et al., 2018b; Glock et al., 2022), and at least some of these Cibicides species are certainly suspension feeders (Wollenburg et al., 2018, 2021). The trophic types that have been introduced above suggest that foraminifera mainly feed on a low trophic level, and it has been suggested that they constitute a trophic link to higher levels in the food chain (Lipps and Valentine, 1970; Gooday et al., 1992; Nomaki et al., 2008).
There are a few studies that specifically focused on trophic interactions of foraminifera in environments where O2 is scarce or absent. Early observations have been documented by Nomaki et al. (2006), who conducted an in situ feeding experiment at central Sagami Bay (1450 m), Japan, using 13C-labeled algae and bacteria. Bottom-water O2 concentration at this location is usually less than 60 µM, and O2 penetration depth into sediments varies between 3 and 10 mm, indicating that infaunal foraminifera in this habitat are regularly exposed to hypoxia and anoxia (Glud et al., 2005). Nomaki et al. (2006) described three different feeding strategies by benthic foraminifera in this environment. Since the bottom-water O2 concentrations at central Sagami Bay fluctuate and are not strictly hypoxic, these observations likely apply to more oxygenated environments as well, especially for the shallow-infaunal species. Uvigerina akitaensis, Bolivina spissa and Bolivina pacifica selectively ingest fresh phytodetritus and thus can be described as phytophagous species (selective herbivores). Bulimina aculeata, Textularia kattegatensis and Globobulimina affinis ingest fresh phytodetritus selectively but feed on sedimentary organic matter instead when fresh phytodetritus is unavailable (seasonal herbivores). The species Cyclammina cancellata and Chilostomella ovoidea ingest sedimentary organic matter at random and can thus be described as detrivores. A later study confirmed these trophic types for most of the species at Sagami Bay by measuring the nitrogen isotope fractionation (δ15N) of their amino acids, which is commonly used to trace the trophic position of an organism in the food chain (Nomaki et al., 2015). Another feeding experiment at Sagami Bay by Nomaki et al. (2011) revealed that all of the analyzed benthic species assimilated carbon from 13C-labeled glucose and thus can effectively also utilize dissolved organic carbon. The same study indicated that even the deep-infaunal detrivores can be selective regarding their food source. Four of the five analyzed species, excluding C. cancellata, incorporated proportionally more 13C-labeled organic matter from the green algae Dunaliella sp. than from other carbon sources, while C. cancellata preferentially incorporated carbon from Chlorella sp. (Nomaki et al., 2005, 2006, 2011). Additional feeding experiments have been conducted at the Arabian Sea OMZ, where benthic foraminifera from locations with different bottom-water O2 concentrations have been supplied with 13C- and 15N-labeled algae (Enge et al., 2014, 2016). Nine out of nine analyzed species took up labeled phytodetritus during the 4 d experimental phase (Enge et al., 2014). The foraminifera took up the highest amount of labeled carbon in the OMZ center, and the uptake decreased with distance from the OMZ (Enge et al., 2016). The authors hypothesized that either the foraminifera from the core OMZ have a higher carbon demand or that there was less food competition with macrofauna at the O2-depleted locations. Similar to the studies by Nomaki et al. (2005, 2006, 2011) at Sagami Bay, the experiments by Enge et al. (2014, 2016) showed a more or less selective ingestion at the Arabian Sea OMZ depending on the foraminiferal species. For example, several Uvigerina species took up large amounts of carbon from the labeled algae and are thus either selective or seasonal herbivores, while Globobulimina spp. took up either no or only small amounts of the labeled carbon, indicating their detritivore behavior (Enge et al., 2016). Further examples for selective herbivores, opportunistic omnivores (which include seasonal herbivores) and sediment detrivores are discussed by Gooday et al. (2008). It appears that many of the species that are considered to be selective herbivores (e.g., B. spissa, U. akitaensis, Eponides pusillus and Cassidulina carinata) have epifaunal or shallow-infaunal lifestyles, although the selective herbivore B. pacifica can also be considered to be intermediate infauna (Gooday et al., 2008). The seasonal herbivores (or opportunistic omnivores, e.g., U. peregrina, G. affinis and G. pacifica) can be found in a relatively wide range of microhabitats, from shallow to deep infauna (Gooday et al., 2008). Species that are considered to be sediment deposit feeders (or detrivores, e.g., C. ovoidea and M. barleeanum) are usually found in the deeper habitats and belong to intermediate to deep infauna (Gooday et al., 2008). This indicates that the selective herbivores must live closer to the source of fresh food supply, while the less selective species can also feed on degraded organic matter or bacteria deeper in the sediments. Thus, the specific trophic type is another control on the microhabitat of benthic foraminifera in addition to the availability of O2 and NO and the metabolic adaptations discussed in Sect. 2. Indeed, the coupled diagenetic and ecologic model of Jorissen et al. (2022) successfully uses different types of food particles as a controlling factor to simulate the microhabitats of benthic foraminifera.
Although benthic foraminifera feed mainly on detritus and minute organisms, there is also (less common) evidence for carnivorous behavior when foraminifera prey on meiofauna (e.g., Lee, 1980; Bowser et al., 1986, 1992; Hallock and Talge, 1994). These observations have mainly been made for species that usually live in oxygenated environments. Dupuy et al. (2010) also documented carnivorous behavior in a laboratory experiment for the Ammonia tepida morphogroup (A. aberdoveyensis or A. confertitesta according to Hayward et al., 2021), which is not uncommon in anoxic layers of tidal mudflats. A study on the trophic behavior of intertidal foraminifera using metabarcoding brought up evidence that A. confertitesta actively preys on small eukaryotes (e.g., nematodes), even in their natural environment (Panagiota-Chronopoulou et al., 2019). The intracellular eukaryotic community in A. confertitesta varies with sediment depth, but even up to 10 cm depth the metabarcoding indicates freshly ingested eukaryotic prey in this species (Panagiota-Chronopoulou et al., 2019). Still, the main eukaryotic prey of A. confertitesta appears to be diatoms (Panagiota-Chronopoulou et al., 2019). Similar results have been documented by Schweizer et al. (2022). Recently, new evidence came up indicating ingestion of nematodes by Globobulimina auriculata from the O2-depleted Alsbäck Deep in Gullmar Fjord, Sweden (Glock et al., 2019a). The species G. auriculata denitrifies and lives under O2-depleted conditions (Woehle and Roy et al., 2018). It is inconclusive though if the foraminifer preys on the nematode or vice versa, but the nematodes have most likely been ingested in the natural O2-depleted habitat (Glock et al., 2019a). Although predation is the main type of interaction in aerobic communities, it usually plays a much smaller role in anoxic communities (Fenchel and Finlay, 1995). This is related to the low growth yields associated with the anaerobic metabolism, which results in very short food chains. Thus, the decrease in energy flow along the anaerobic food chains is higher than along the aerobic food chain (Fenchel and Finlay, 1995). The predatory isopod Saduria entomon for example strongly reduces its predatory activity under hypoxia in comparison to aerobic conditions (Sandberg, 1994), and the predator prey biomass ratio has been shown to be 4 times lower in anoxic environments compared to oxic environments (Fenchel and Finlay, 1995). There is evidence that foraminifera from the Namibian shelf can perform phagocytosis (vacuolic ingestion of food particles) even under anoxic conditions, which usually requires bursts of energy (Orsi et al., 2020). This study provides further evidence that the Namibian foraminifera express enzymes for lysing digested prey cells inside food vacuoles after phagocytosis (schematic representations for phagocytosis and predation on meiofauna shown in Fig. 5). The evidence for phagotrophy and predation on or by benthic foraminifera under O2-depleted conditions, although it is rare, is thought-provoking, and future studies might shed more light on predator–prey interactions of benthic foraminifera in O2-depleted environments. In general, future metabarcoding studies to identify food sources of deep infauna or foraminifera that inhabit anoxic environments might shed more light on trophic strategies in O2-depleted environments.
Pina-Ochoa et al. (2010b) also suggested the possible importance of denitrifying foraminifera for the benthic N cycle due partly to their high abundances in O2-depleted environments. In some environments, such as certain habitats in the Peruvian OMZ, foraminifera even seem to be the key players in benthic denitrification (Glud et al., 2009; Glock et al., 2013, 2019b; Choquel et al., 2021). Complete heterotrophic denitrification produces non-reactive (i.e., not bioavailable) N2 gas. Denitrifying benthic foraminifera can thus be considered a sink for bioavailable N. Recent genetic studies on denitrifying benthic foraminifera did not find transcripts for homologues of enzymes that catalyze the last step of denitrification – the reduction of N2O to N2 (Woehle and Roy et al., 2018, 2022; Orsi et al., 2020; Gomaa et al., 2021). Some globobuliminids from the O2-depleted Alsbäck Deep in the Swedish Gullmar Fjord have been shown to produce N2O gas as a product of denitrification, although the rates were lower than their rates for complete denitrification (Piña-Ochoa et al., 2010a). The NO storage in denitrifying foraminifera, but also in some sulfur bacteria, such as Beggiatoa, is of greater importance for benthic biogeochemical cycling due to the potential of biological transport of these intracellular reservoirs (Dale et al., 2016). Most of the other diagenetic models that describe and calculate benthic N cycling are based on (and limited to) diffusive transport of the different N species in bottom and pore water. Active biological transport of different N species can thus efficiently influence the benthic fluxes of different N species (Dale et al., 2016).
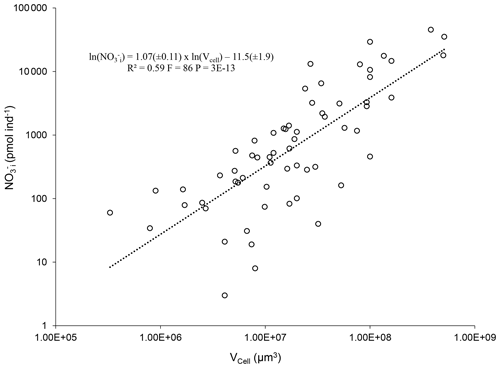
Figure 6Log–log plot and power regression of intracellular NO content (NO against the biovolume (Vcell) of benthic foraminifera from diverse environments (Table 2). Only species with an intracellular [NO mM where both NO and Vcell were published were considered for the power regression.
The estimates of total benthic foraminiferal denitrification rates are mainly based on upscaling individual species-specific denitrification rates by the living abundances of benthic foraminifera in different environments (Piña-Ochoa et al., 2010b; Glock et al., 2013, 2019b). This approach is limited by the availability of species-specific denitrification rates, although various approximations can be used to calculate estimated denitrification rates for species with unknown denitrification rates (Glock et al., 2013). A summary of all published benthic foraminiferal denitrification rates can be found in Table 1. Further data on species-specific foraminiferal denitrification rates will improve our estimates regarding the role of foraminifera in benthic N cycling and thus also models for benthic biogeochemical cycling.
Recently, it has been found that some benthic foraminifera not only store NO for denitrification but also larger amounts phosphate (Glock et al., 2020). The intracellular phosphate concentration can exceed the concentration in the surrounding pore waters by a factor of 10 to 100. The use of this intracellular phosphate storage is still under debate. Hypotheses include the synthesis of polyphosphates or a reservoir for the synthesis of phospholipids for the cell membranes (Glock et al., 2020). In addition, there is evidence that the intracellular phosphate storage in foraminifera facilitates phosphogenesis in some environments, similar to the intracellular polyphosphate enrichment in some sulfur bacteria (Schulz and Schulz, 2005). The release of phosphate after breakdown of these polyphosphates to harvest energy in times of electron acceptor depletion results in apatite supersaturation and initiates phosphogenesis (Schulz and Schulz, 2005). Sediments at the lower boundary of the Peruvian OMZ contain many small phosphorite grains with foraminifera of similar size and shape (Manheim et al., 1975; Glock et al., 2020). The sand fraction of the surface sediments in this region is a mixture of pristine living foraminifer shells with dead tests that show a transition from shells that are filled with phosphorites to small phosphorite grains that only retain the size and coarse shape of a foraminifer. It is likely that a postmortem release of the intracellular phosphate storage results in a supersaturated microenvironment within the shells that initiates apatite formation (Glock et al., 2020) in a similar way to that suggested for other organisms (Kulakovskaya, 2014). The recent evidence for the potential of benthic foraminifera to use dephosphorylation of intracellular creatine phosphate storage to regenerate ATP under anoxic conditions might be another explanation for the high intracellular phosphate storage (Orsi et al., 2020). It might be that this is an adaptation of foraminifera to enable phagocytosis even under anoxic conditions.
3.1 Estimating the contribution of foraminifera to benthic nutrient budgets and fluxes
The intracellular NO storage in benthic foraminifera from different environments shows a relatively wide concentration range (Table 2). In addition, species that lack intracellular NO storage are relatively widespread, and there are species that, depending on the environment, either have or lack intracellular NO (Tables 2 and 3). Most of the species that have been found both with and without intracellular NO in different environments (bold species in Table 3) are species that are typically shallow-infaunal. They belong to the group of foraminifera that might partly be considered facultative anaerobe and are likely opportunistic species that are well adapted to transitional environments with periodic O2 depletion, since they apparently can handle oxygenated and anoxic environments (see Sect. 2.1.1). In addition, the NO is most likely stored in seawater vacuoles, and the vacuole volume of foraminifera can have a large variability (LeKieffre et al., 2018).
Given this variation in NO storage capability, the reliability of estimates for the foraminiferal contribution to NO budgets depends crucially on the availability of data. The more data there are, the better we are able to calculate foraminiferal NO budgets. Nevertheless, there are thousands of benthic foraminiferal species, and a considerable number of these species inhabit O2-depleted environments and potentially store NO and denitrify. It will be unrealistic to measure the intracellular nutrient content and metabolic rates for all foraminifera. Thus, functions to estimate the contribution of species with unknown denitrification rates or intracellular NO will provide more data for better estimates of total foraminiferal budgets within the nitrogen cycle. Of course, it is not possible to strictly define which foraminiferal species are able to denitrify or to store NO without real measurements. If a foraminiferal species inhabits O2-depleted environments and belongs to a genus of the species listed in Tables 1 and 2, as a rule of thumb, they are good candidates for potential denitrifiers. In addition, if a species is known to inhabit well-oxygenated environments and/or belongs to a genus of the species shown in Table 3, use of the equations presented below to estimate NO storage or denitrification rates should be avoided. Considering this, an analysis of published data on intracellular NO content reveals a highly significant correlation between the intracellular NO and the cell volume of denitrifying benthic foraminifera (Fig. 6; power regression, R2=0.59, F=86, ).
Thus, the intracellular NO content of a potentially denitrifying foraminifer can be estimated from its biovolume according to the following equation:
where NO is the intracellular NO content in picomoles per individual and Vcell is the cell volume in cubic micrometers. Note that only species from Table 2 with an intracellular [NO mM were considered for the power regression. In addition, two extreme data points were discarded as outliers (see Supplement). Similar equations have been published to estimate foraminiferal denitrification rates (Glock et al., 2019b; Eq. 2 herein) and intracellular dissolved inorganic phosphorous content (Glock et al., 2020; Eq. 3 herein).
where Rden(ind) is the individual denitrification rate in picomoles per individual per day, and DIPi is the intracellular dissolved inorganic phosphorous content in picomoles per individual.
Further equations and principles for upscaling foraminiferal nitrogen and phosphorous budgets from abundances of living foraminifera can be found in Glock et al. (2013, 2019b and 2020) and Xu et al. (2021). Formulae to estimate the biovolume of many different common shapes of foraminifera have recently been published (de Freitas et al., 2021). Due to the high uncertainties related to the natural variability in metabolic rates and nutrient storage, a thorough error estimation is recommended (see Appendix B in Glock et al., 2020). With an increasing amount of data on metabolic rates and intracellular nutrient storage, more accurate models and equations might become available in the future that describe the role of benthic foramifera within marine biogeochemistry. Similar models and equations might also be very helpful for exploring the role of planktonic foraminifera in pelagic biogeochemistry.
No new data have been used for this paper. All original data can be found in the cited articles, and all data I compiled are available in the tables of the paper.
The supplement related to this article is available online at: https://doi.org/10.5194/bg-20-3423-2023-supplement.
The contact author has declared that neither of the authors has any competing interests.
Publisher’s note: Copernicus Publications remains neutral with regard to jurisdictional claims in published maps and institutional affiliations.
This article is part of the special issue “Low-oxygen environments and deoxygenation in open and coastal marine waters”. It is not associated with a conference.
I would like to thank Gerhard Schmiedl for providing constructive feedback on an early draft of this paper. In addition, I acknowledge the extensive and constructive feedback of Andrew Gooday, Frans Jorissen, another anonymous reviewer and the editor Lisa Levin, which significantly improved this paper. Funding was provided by the Deutsche Forschungsgemeinschaft (DFG) through Heisenberg Grant GL 999/3-1 to Nicolaas Glock. Finally, I would like to thank all the authors and co-authors that are cited in this review because of their pioneering research on benthic foraminifera from O2-depleted environments.
This research has been supported by the Deutsche Forschungsgemeinschaft (grant no. GL 999/3-1).
This paper was edited by Lisa Levin and reviewed by Andy Gooday and one anonymous referee.
Alve, E. and Murray, J.: Temporal variability in vertical distributions of live (stained) intertidal foraminifera, Southern England, J. Foramin. Res., 31, 12–24, https://doi.org/10.2113/0310012, 2001.
Austin, H. A., Austin, W. E. N., and Paterson, D. M.: Extracellular cracking and content removal of the benthic diatom Pleurosigma angulatum (Quekett) by the benthic foraminifera Haynesina germanica (Ehrenberg), Mar. Micropaleontol., 57, 68–73, https://doi.org/10.1016/J.MARMICRO.2005.07.002, 2005.
Auten, R. L. and Davis, J. M.: Oxygen toxicity and reactive oxygen species: The devil is in the details, Pediatr. Res., 66, 121–127, https://doi.org/10.1203/PDR.0b013e3181a9eafb, 2009.
Barras, C., Mouret, A., Nardelli, M. P., Metzger, E., Petersen, J., La, C., Filipsson, H. L., and Jorissen, F.: Experimental calibration of manganese incorporation in foraminiferal calcite, Geochim. Cosmochim. Ac., 237, 49–64, https://doi.org/10.1016/j.gca.2018.06.009, 2018.
Bernhard, J. M.: Characteristic assemblages and morphologies of benthic foraminifera from anoxic, organic-rich deposits; Jurassic through Holocene, J. Foraminifer. Res., 16, 207–215, https://doi.org/10.2113/GSJFR.16.3.207, 1986.
Bernhard, J. M.: Potential Symbionts in Bathyal Foraminifera, Science, 299, p. 861, https://doi.org/10.1126/science.1077314, 2003.
Bernhard, J. M. and Alve, E.: Survival, ATP pool, and ultrastructural characterization of benthic foraminifera from Drammensfjord (Norway): response to anoxia, Mar. Micropaleontol., 28, 5–17, https://doi.org/10.1016/0377-8398(95)00036-4, 1996.
Bernhard, J. M. and Bowser, S. S.: Benthic foraminifera of dysoxic sediments: chloroplast sequestration and functional morphology, Earth-Sci. Rev., 46, 149–165, https://doi.org/10.1016/S0012-8252(99)00017-3, 1999.
Bernhard, J. M. and Sen Gupta, B. K.: Foraminifera of oxygen-depleted environments, Mod. Foraminifera, 201–216, https://doi.org/10.1007/0-306-48104-9_ 12, 1999.
Bernhard, J. M. and Bowser, S. S.: Peroxisome proliferation in foraminifera inhabiting the chemocline: An adaptation to reactive oxygen species https://doi.org/10.1111/j.1550-7408.2008.00318.x, 2008.
Bernhard, J. M., Sen Gupta, B. K., and Borne, P. F.: Benthic foraminiferal proxy to estimate dysoxic bottom-water oxygen concentrations; Santa Barbara Basin, U.S. Pacific continental margin, J. Foraminifer. Res., 27, 301–310, https://doi.org/10.2113/GSJFR.27.4.301, 1997.
Bernhard, J. M., Buck, K. R., Farmer, M. A., and Bowser, S. S.: The Santa Barbara Basin is a symbiosis oasis, Nature, 403, 77–80, https://doi.org/10.1038/47476, 2000.
Bernhard, J. M., Buck, K. R., and Barry, J. P.: Monterey Bay cold-seep biota: Assemblages, abundance, and ultrastructure of living foraminifera, Deep. Res. Pt. I, 48, 2233–2249, 2001.
Bernhard, J. M., Ostermann, D. R., Williams, D. S., and Blanks, J. K.: Comparison of two methods to identify live benthic foraminifera: A test between Rose Bengal and CellTracker Green with implications for stable isotope paleoreconstructions, Paleoceanography, 21, https://doi.org/10.1029/2006PA001290, 2006.
Bernhard, J. M., Goldstein, S. T., and Bowser, S. S.: An ectobiont-bearing foraminiferan, Bolivina pacifica, that inhabits microxic pore waters: cell-biological and paleoceanographic insights, Environ. Microbiol., 12, 2107–2119, https://doi.org/10.1111/j.1462-2920.2009.02073.x, 2010a.
Bernhard, J. M., Martin, J. B., and Rathburn, A. E.: Combined carbonate carbon isotopic and cellular ultrastructural studies of individual benthic foraminifera: 2. Toward an understanding of apparent disequilibrium in hydrocarbon seeps, Paleoceanography, 25, https://doi.org/10.1029/2010PA001930, 2010b.
Bernhard, J. M., Edgcomb, V. P., Casciotti, K. L., McIlvin, M. R., and Beaudoin, D. J.: Denitrification likely catalyzed by endobionts in an allogromiid foraminifer., ISME J., 6, 951–60, https://doi.org/10.1038/ismej.2011.171, 2012a.
Bernhard, J. M., Casciotti, K. L., Mcilvin, M. R., Beaudoin, D. J., Visscher, P. T., and Edgcomb, V. P.: Potential importance of physiologically diverse benthic foraminifera in sedimentary nitrate storage and respiration, 117, 1–14, https://doi.org/10.1029/2012JG001949, 2012b.
Bernhard, J. M., Tsuchiya, M., and Nomaki, H.: Ultrastructural observations on prokaryotic associates of benthic foraminifera: Food, mutualistic symbionts, or parasites?, Mar. Micropaleontol., 138, 33–45, https://doi.org/10.1016/j.marmicro.2017.09.001, 2018.
Bowser, S. S., Delaca, T. E., and Rieder, C. L.: Novel extracellular matrix and microtubule cables associated with pseudopodia of Astrammina rara, a carnivorous antarctic foraminifer, J. Ultrastruct. Mol. Struct. Res., 94, 149–160, https://doi.org/10.1016/0889-1605(86)90061-3, 1986.
Bowser, S. S., Alexander, S. P., Stockton, W. L., and Delaca, T. E.: Extracellular matrix augments mechanical properties of pseudopodia in the carnivorous foraminiferan Astrammina rara: Role in prey capture, J. Protozool., 39, 724–732, https://doi.org/10.1111/j.1550-7408.1992.tb04455.x, 1992.
Brinkmann, I., Ni, S., Schweizer, M., Oldham, V. E., Quintana Krupinski, N. B., Medjoubi, K., Somogyi, A., Whitehouse, M. J., Hansel, C. M., Barras, C., Bernhard, J. M., and Filipsson, H. L.: Foraminiferal as bottom-water hypoxia proxy: An assessment of Nonionella stella in the Santa Barbara Basin, USA, Paleoceanogr. Paleoclimatol., 36, e2020PA004167, https://doi.org/10.1029/2020PA004167, 2021.
Cato, I., Olsson, I., and Rosenberg, R.: Recovery and decontamination of estuaries, in: Chemistry and Biogeochemistry of Estuaries, edited by: Olausson, E. and Cato, I., Wiley, London, 403–440, 1980.
Cedhagen, T.: Retention of chloroplasts and bathymetric distribution in the sublittoral foraminiferan Nonionellina labradorica, Ophelia, 33, 17–30, https://doi.org/10.1080/00785326.1991.10429739, 1991.
Cesbron, F., Geslin, E., Le Kieffre, C., Jauffrais, T., Nardelli, M. P., Langlet, D., Mabilleau, G., Jorissen, F. J., Jézéquel, D., and Metzger, E.: Sequestered chloroplasts in the benthic foraminifer Haynesina germanica: Cellular organization, oxygen fluxes and potential ecological implications, J. Foraminifer. Res., 47, 268–278, 2017.
Cevasco, M. E., Lechliter, S. M., Mosier, A. E., and Perez, J.: Initial observations of kleptoplasty in the foraminifera of Coastal South Carolina, Naturalist, 14, 361–372, https://doi.org/10.2307/26454495, 2015.
Choquel, C., Geslin, E., Metzger, E., Filipsson, H. L., Risgaard-Petersen, N., Launeau, P., Giraud, M., Jauffrais, T., Jesus, B., and Mouret, A.: Denitrification by benthic foraminifera and their contribution to N-loss from a fjord environment, Biogeosciences, 18, 327–341, https://doi.org/10.5194/bg-18-327-2021, 2021.
Clark, K., Jensen, K., and Stirts, H.: Survey for functional kleptoplasty among West Atlantic Ascoglossa (= Sacoglossa) (Mollusca: Opisthobranchia), Veliger, 334, 339–345, 1990.
Cook, M. K., Dial, A. R., and Hendy, I. L.: Iodine stability as a function of pH and its implications for simultaneous multi-element ICP-MS analysis of marine carbonates for paleoenvironmental reconstructions, Mar. Chem., 245, 104148, https://doi.org/10.1016/J.MARCHEM.2022.104148, 2022.
Corliss, B. H.: Microhabitats of benthic foraminifera within deep-sea sediments, Nature, 314, 435–438, https://doi.org/10.1038/314435a0, 1985.
Corliss, B. H.: Morphology and microhabitat preferences of benthic foraminifera from the northwest Atlantic Ocean, Mar. Micropaleontol., 17, 195–236, https://doi.org/10.1016/0377-8398(91)90014-W, 1991.
Corliss, B. H. and Chen, C.: Morphotype patterns of Norwegian Sea deep-sea benthic foraminifera and ecological implications, Geology, 16, 716–719, https://doi.org/10.1130/0091-7613(1988)016< 0716:MPONSD>2.3.CO;2, 1988.
Correia, M. J. and Lee, J. J.: Chloroplast retention by Elphidium excavatum (Terquem). Is it a selective process?, Symbiosis, 29, 343–355, 2000.
Correia, M. J. and Lee, J. J.: Fine structure of the plastids retained by the foraminifer Elphidium excavatum (Terquem), Symbiosis, 32, 15–26, 2002a.
Correia, M. J. and Lee, J. J.: How long do the plastids retained by Elphidium excavatum (Terquem) last in their host?, Symbiosis, 32, 27–38, 2002b.
Costa, K. M., Nielsen, S. G., Wang, Y., Lu, W., Hines, S. K. V, Jacobel, A. W., and Oppo, D. W.: Marine sedimentary uranium to barium ratios as a potential quantitative proxy for Pleistocene bottom water oxygen concentrations, Geochim. Cosmochim. Ac., 343, 1–16, https://doi.org/10.1016/j.gca.2022.12.022, 2023.
Dale, A. W., Sommer, S., Lomnitz, U., Bourbonnais, A., and Wallmann, K.: Biological nitrate transport in sediments on the Peruvian margin mitigates benthic sulfide emissions and drives pelagic N loss during stagnation events, Deep Sea Res. Pt. I, 112, 123–136, https://doi.org/10.1016/j.dsr.2016.02.013, 2016.
Dalsgaard, T., Frank, S. J., Thamdrup, B., De Brabandere, L., Revsbech, N. P., Ulloa, O., Canfield, D. E., and DeLong, E. F.: Oxygen at nanomolar levels reversibly suppresses process rates and gene expression in anammox and denitrification in the oxygen minimum Zone off Northern Chile, MBio, 5, e01966-14, https://doi.org/10.1128/mBio.01966-14, 2014.
Davis, C. V., Wishner, K., Renema, W., and Hull, P. M.: Vertical distribution of planktic foraminifera through an oxygen minimum zone: how assemblages and test morphology reflect oxygen concentrations, Biogeosciences, 18, 977–992, https://doi.org/10.5194/bg-18-977-2021, 2021.
Davis, C. V., Sibert, E. C., Jacobs, P. H., Burls, N., and Hull, P. M.: Intermediate water circulation drives distribution of Pliocene Oxygen Minimum Zones, Nat. Commun., 14, 1–11, https://doi.org/10.1038/s41467-022-35083-x, 2023.
de Freitas, T. R., Bacalhau, E. T., and Disaró, S. T.: Biovolume method for foraminiferal biomass assessment: Evaluation of geometric models and incorporation of species mean cell occupancy, J. Foraminifer. Res., 51, 249–266, https://doi.org/10.2113/GSJFR.51.4.249, 2021.
Deldicq, N., Alve, E., Schweizer, M., Asteman, I. P., Hess, S., Darling, K., and Bouchet, V. M. P.: History of the introduction of a species resembling the benthic foraminifera nonionella stella in the oslofjord (Norway): Morphological, molecular and paleo-ecological evidences, Aquat. Invasions, 14, 182–205, https://doi.org/10.3391/ai.2019.14.2.03, 2019.
Diaz, R. J.: Anoxia, hypoxia, and dead zones BT – Encyclopedia of Estuaries, edited by: Kennish, M. J., Springer Netherlands, Dordrecht, 19–29, https://doi.org/10.1007/978-94-017-8801-4_ 82, 2016.
Duijnstee, I. A. P., Ernst, S. R., and Van Der Zwaan, G. J.: Effect of anoxia on the vertical migration of benthic foraminifera, Mar. Ecol. Prog. Ser., 246, 85–94, https://doi.org/10.3354/MEPS246085, 2003.
Dupuy, C., Rossignol, L., Geslin, E., and Pascal, P. Y.: Predation of mudflat meio-macrofaunal metazoans by a calcareous foraminifer, Ammonia tepida (Cushman, 1926), J. Foraminifer. Res., 40, 305–312, https://doi.org/10.2113/GSJFR.40.4.305, 2010.
Enge, A. J., Witte, U., Kucera, M., and Heinz, P.: Uptake of phytodetritus by benthic foraminifera under oxygen depletion at the Indian margin (Arabian Sea), Biogeosciences, 11, 2017–2026, https://doi.org/10.5194/bg-11-2017-2014, 2014.
Enge, A. J., Wukovits, J., Wanek, W., Watzka, M., Witte, U. F. M., Hunter, W. R., and Heinz, P.: Carbon and Nitrogen Uptake of Calcareous Benthic Foraminifera along a Depth-Related Oxygen Gradient in the OMZ of the Arabian Sea, Sec. Aquatic Microbiology, 7, https://doi.org/10.3389/fmicb.2016.00071, 2016.
Erdem, Z. and Schönfeld, J.: Pleistocene to Holocene benthic foraminiferal assemblages from the Peruvian continental margin, Palaeontol. Electron., 1–32, https://doi.org/10.26879/764, 2017.
Erez, J.: The source of ions for biomineralization in foraminifera and their implications for paleoceanographic proxies, Rev. Mineral. Geochem., 54, 115–149, https://doi.org/10.2113/0540115, 2003.
Ettwig, K. F., Speth, D. R., Reimann, J., Wu, M. L., Jetten, M. S. M., and Keltjens, J. T.: Bacterial oxygen production in the dark, Front. Microbiol., 3, 273, https://doi.org/10.3389/FMICB.2012.00273/BIBTEX, 2012.
Fenchel, T. M. and Finlay, B. J.: Ecology and evolution in anoxic worlds, edited by: Fenchel, T. M. and Finlay, B. J., Oxford University Press Inc., Oxford, ISBN 0198548389, 1995.
Finlay, B. J., Span, A. S. W., and Harman, J. M. P.: Nitrate respiration in primitive eukaryotes, Nature, 303, 333–336, https://doi.org/10.1038/303333a0, 1983.
Frank, L. and Massaro, D.: Oxygen toxicity, Am. J. Med., 69, 117–126, https://doi.org/10.1016/0002-9343(80)90509-4, 1980.
Glock, N., Eisenhauer, A., Milker, Y., Liebetrau, V., Schonfeld, J., Mallon, J., Sommer, S., and Hensen, C.: Evironmental influences on the pore density of Bolivina spissa (Cushman), J. Foraminifer. Res., 41, 22–32, https://doi.org/10.2113/gsjfr.41.1.22, 2011.
Glock, N., Erdem, Z., Wallmann, K., Somes, C. J., Liebetrau, V., Schönfeld, J., Gorb, S., and Eisenhauer, A.: Coupling of oceanic carbon and nitrogen facilitates spatially resolved quantitative reconstruction of nitrate inventories, Nat. Commun., 9, 1217, https://doi.org/10.1038/s41467-018-03647-5, 2018.
Glock, N., Schönfeld, J., Eisenhauer, A., Hensen, C., Mallon, J., and Sommer, S.: The role of benthic foraminifera in the benthic nitrogen cycle of the Peruvian oxygen minimum zone, Biogeosciences, 10, 4767–4783, https://doi.org/10.5194/bg-10-4767-2013, 2013.
Glock, N., Wukovits, J., and Roy, A.-S.: Interactions of Globobulimina auriculata with nematodes: predator or prey?, J. Foraminifer. Res., 49, 66–75, https://doi.org/10.2113/gsjfr.49.1.66, 2019a.
Glock, N., Roy, A.-S., Romero, D., Wein, T., Weissenbach, J., Revsbech, N. P., Høgslund, S., Clemens, D., Sommer, S., and Dagan, T.: Metabolic preference of nitrate over oxygen as an electron acceptor in foraminifera from the Peruvian oxygen minimum zone, P. Natl. Acad. Sci. USA, 116, 2860–2865, https://doi.org/10.1073/pnas.1813887116, 2019b.
Glock, N., Liebetrau, V., Vogts, A., and Eisenhauer, A.: Organic heterogeneities in foraminiferal calcite traced through the distribution of N, S, and I measured with NanoSIMS: A new challenge for element-ratio-based paleoproxies?, Front. Earth Sci., 7, 175, https://doi.org/10.3389/feart.2019.00175, 2019c.
Glock, N., Romero, D., Roy, A. S., Woehle, C., Dale, A. W., Schönfeld, J., Wein, T., Weissenbach, J., and Dagan, T.: A hidden sedimentary phosphate pool inside benthic foraminifera from the Peruvian upwelling region might nucleate phosphogenesis, Geochim. Cosmochim. Ac., 289, 14–32, https://doi.org/10.1016/j.gca.2020.08.002, 2020.
Glock, N., Erdem, Z., and Schönfeld, J.: The Peruvian oxygen minimum zone was similar in extent but weaker during the Last Glacial Maximum than Late Holocene, Commun. Earth Environ., 3, 1–14, https://doi.org/10.1038/s43247-022-00635-y, 2022.
Glud, R. N., Wenzhöfer, F., Tengberg, A., Middelboe, M., Oguri, K., and Kitazato, H.: Distribution of oxygen in surface sediments from central Sagami Bay, Japan: In situ measurements by microelectrodes and planar optodes, Deep. Sea Res. Pt. I, 52, 1974–1987, https://doi.org/10.1016/J.DSR.2005.05.004, 2005.
Glud, R. N., Thamdrup, B., Stahl, H., Wenzhoefer, F., Glud, A., Nomaki, H., Oguri, K., Revsbech, N. P., and Kitazato, H.: Nitrogen cycling in a deep ocean margin sediment (Sagami Bay, Japan), Limnol. Oceanogr., 54, 723–734, https://doi.org/10.4319/lo.2009.54.3.0723, 2009.
Goldstein, S. T. and Richardson, E. A.: Fine structure of the foraminifer Haynesina germanica (Ehrenberg) and its sequestered chloroplasts, Mar. Micropaleontol., 138, 63–71, https://doi.org/10.1016/J.MARMICRO.2017.10.010, 2018.
Goldstein, S. T., Bernhard, J. M., and Richardson, E. A.: Chloroplast sequestration in the foraminifer Haynesina germanica: Application of high pressure freezing and freeze substitution, Microsc. Microanal., 10, 1458–1459, https://doi.org/10.1017/S1431927604885891, 2004.
Gomaa, F., Utter, D. R., Powers, C., Beaudoin, D. J., Edgcomb, V. P., Filipsson, H. L., Hansel, C. M., Wankel, S. D., Zhang, Y., and Bernhard, J. M.: Multiple integrated metabolic strategies allow foraminiferan protists to thrive in anoxic marine sediments, Sci. Adv., 7, eabf1586, https://doi.org/10.1126/sciadv.abf1586, 2021.
Gooday, A. J., Levin, L. A., Linke, P., and Heeger, T.: The Role of Benthic Foraminifera in Deep-Sea Food Webs and Carbon Cycling BT – Deep-sea food chains and the global carbon cycle, edited by: Rowe, G. T. and Pariente, V., Springer Netherlands, Dordrecht, 63–91, https://doi.org/10.1007/978-94-011-2452-2_ 5, 1992.
Gooday, A. J., Nomaki, H., and Kitazato, H.: Modern deep-sea benthic foraminifera: a brief review of their morphology-based biodiversity and trophic diversity, Geol. Soc. Spec. Publ., 303, 97–119, https://doi.org/10.1144/SP303.8, 2008.
Grzymski, J., Schofield, O. M., Falkowski, P. G., and Bernhard, J. M.: The function of plastids in the deep-sea benthic foraminifer, Nonionella stella, Limnol. Oceanogr., 47, 1569–1580, https://doi.org/10.4319/LO.2002.47.6.1569, 2002.
Hallock, P. and Talge, H. K.: A predatory foraminifer, Floresina amphiphaga, n. sp., from the Florida Keys, J. Foraminifer. Res., 24, 210–213, https://doi.org/10.2113/GSJFR.24.4.210, 1994.
Hannah, F. and Rogerson, A.: The temporal and spatial distribution of foraminiferans in marine benthic sediments of the Clyde Sea Area, Scotland, Estuar. Coast. Shelf Sci., 44, 377–383, https://doi.org/10.1006/ECSS.1996.0136, 1997.
Harman, R. A.: Distribution of foraminifera in the Santa Barbara Basin, California, Micropaleontology, 10, 81–96, https://doi.org/10.2307/1484628, 1964.
Hayward, B., Holzmann, M., Pawlowski, J., Parker, J., Kaushik, T., Toyofuku, M., and Tsuchiya, M.: Molecular and morphological taxonomy of living Ammonia and related taxa (Foraminifera) and their biogeography, Micropaleontology, 67, 109–313, https://doi.org/10.47894/mpal.67.2-3.01, 2021.
Høgslund, S., Revsbech, N. P., Cedhagen, T., Nielsen, L. P., and Gallardo, V. A.: Denitrification, nitrate turnover, and aerobic respiration by benthic foraminiferans in the oxygen minimum zone off Chile, J. Exp. Mar. Bio. Ecol., 359, 85–91, https://doi.org/10.1016/j.jembe.2008.02.015, 2008.
Høgslund, S., Cedhagen, T., Bowser, S. S., and Risgaard-Petersen, N.: Sinks and sources of intracellular nitrate in gromiids, Front. Microbiol., 8, 617, https://doi.org/10.3389/fmicb.2017.00617, 2017.
Hoogakker, B. and the PLO2P-Team: Review of proxies for low-oxygen paleo reconstructions, ID# 1273324, presented at AGU23, San Francisco CA, 11–15 Deember 2023.
Hoogakker, B. A. A., Elderfield, H., Schmiedl, G., McCave, I. N., and Rickaby, R. E. M.: Glacial–interglacial changes in bottom-water oxygen content on the Portuguese margin, Nat. Geosci., 8, 40–43, https://doi.org/10.1038/ngeo2317, 2014.
Hoogakker, B. A. A., Lu, Z., Umling, N., Jones, L., Zhou, X., Rickaby, R. E. M., Thunell, R., Cartapanis, O., and Galbraith, E.: Glacial expansion of oxygen-depleted seawater in the eastern tropical Pacific, Nature, 562, 410–413, https://doi.org/10.1038/s41586-018-0589-x, 2018a.
Hoogakker, B. A. A., Lu, Z., Umling, N., Jones, L., and Zhou, X.: Glacial expansion of oxygen-depleted seawater in the eastern tropical Pacific, Nature, 562, 410–413, https://doi.org/10.1038/s41586-018-0589-x, 2018b.
Jansen, S., Walpersdorf, E., Werner, U., Billerbeck, M., Böttcher, M. E., De Beer, D., Jansen, S., Walpersdorf, E., Werner, : U, Billerbeck, : M, Böttcher, M. E., De Beer, D., Werner, U., and Billerbeck, M.: Functioning of intertidal flats inferred from temporal and spatial dynamics of O2, H2S and pH in their surface sediment, Ocean Dynam., 59, 317–332, https://doi.org/10.1007/S10236-009-0179-4, 2009.
Jauffrais, T., Jesus, B., Metzger, E., Mouget, J.-L., Jorissen, F., and Geslin, E.: Effect of light on photosynthetic efficiency of sequestered chloroplasts in intertidal benthic foraminifera (Haynesina germanica and Ammonia tepida), Biogeosciences, 13, 2715–2726, https://doi.org/10.5194/bg-13-2715-2016, 2016.
Jauffrais, T., Jesus, B., Méléder, V., and Geslin, E.: Functional xanthophyll cycle and pigment content of a kleptoplastic benthic foraminifer: Haynesina germanica, PLoS One, 12, e0172678, https://doi.org/10.1371/JOURNAL.PONE.0172678, 2017.
Jauffrais, T., LeKieffre, C., Koho, K. A., Tsuchiya, M., Schweizer, M., Bernhard, J. M., Meibom, A., and Geslin, E.: Ultrastructure and distribution of kleptoplasts in benthic foraminifera from shallow-water (photic) habitats, Mar. Micropaleontol., 138, 46–62, https://doi.org/10.1016/J.MARMICRO.2017.10.003, 2018.
Jauffrais, T., LeKieffre, C., Schweizer, M., Geslin, E., Metzger, E., Bernhard, J. M., Jesus, B., Filipsson, H. L., Maire, O., and Meibom, A.: Kleptoplastidic benthic foraminifera from aphotic habitats: insights into assimilation of inorganic C, N and S studied with sub-cellular resolution, Environ. Microbiol., 21, 125–141, https://doi.org/10.1111/1462-2920.14433, 2019.
Jesus, B., Jauffrais, T., Trampe, E. C. L., Goessling, J. W., Lekieffre, C., Meibom, A., Kühl, M., and Geslin, E.: Kleptoplast distribution, photosynthetic efficiency and sequestration mechanisms in intertidal benthic foraminifera, ISME J., 16, 822–832, https://doi.org/10.1038/s41396-021-01128-0, 2021.
Jørgensen, B. B.: Bacteria and marine biogeochemistry, Mar. Geochem., 169–206, https://doi.org/10.1007/3-540-32144-6_ 5/COVER, 2006.
Jorissen, F. J., de Stigter, H. C., and Widmark, J. G. V: A conceptual model explaining benthic foraminiferal microhabitats, Mar. Micropaleontol., 26, 3–15, https://doi.org/10.1016/0377-8398(95)00047-X, 1995.
Jorissen, F. J., Meyers, S. R., Kelly-Gerreyn, B. A., Huchet, L., Mouret, A., and Anschutz, P.: The 4GFOR model – Coupling 4G early diagenesis and benthic foraminiferal ecology, Mar. Micropaleontol., 170, 102078, https://doi.org/10.1016/j.marmicro.2021.102078, 2022.
Kaiho, K.: Benthic foraminiferal dissolved-oxygen index and dissolved-oxygen levels in the modern ocean, Geology, 22, 719–722, https://doi.org/10.1130/0091-7613(1994)022< 0719:BFDOIA>2.3.CO;2, 1994.
Keating-Bitonti, C. R. and Payne, J. L.: Ecophenotypic responses of benthic foraminifera to oxygen availability along an oxygen gradient in the California Borderland, Mar. Ecol., 38, 1–16, https://doi.org/10.1111/maec.12430, 2017.
Knowles, R.: Denitrification, in: Terrestrial nitrogen cycles, Ecological Bulletin, Stockholm, 315–329, 1981.
Koho, K. A. and Piña-Ochoa, E.: Benthic foraminifera: Inhabitants of low-oxygen environments, in: Anoxia: Evidence for Eukaryote Survival and Paleontological Strategies, edited by: Altenbach, A. V, Bernhard, J. M., and Seckbach, J., Springer Netherlands, Dordrecht, 249–285, https://doi.org/10.1007/978-94-007-1896-8_ 14, 2012.
Koho, K. A., Piña-Ochoa, E., Geslin, E., and Risgaard-Petersen, N.: Vertical migration, nitrate uptake and denitrification: survival mechanisms of foraminifers (Globobulimina turgida) under low oxygen conditions, FEMS Microbiol. Ecol., 75, 273–283, https://doi.org/10.1111/j.1574-6941.2010.01010.x, 2011.
Koho, K. A., LeKieffre, C., Nomaki, H., Salonen, I., Geslin, E., Mabilleau, G., Søgaard Jensen, L. H., and Reichart, G. J.: Changes in ultrastructural features of the foraminifera Ammonia spp. in response to anoxic conditions: Field and laboratory observations, Mar. Micropaleontol., 138, 72–82, https://doi.org/10.1016/j.marmicro.2017.10.011, 2018.
Kranner, M., Harzhauser, M., Beer, C., Auer, G., and Piller, W. E.: Calculating dissolved marine oxygen values based on an enhanced Benthic Foraminifera Oxygen Index, Sci. Rep., 12, 1376, https://doi.org/10.1038/s41598-022-05295-8, 2022.
Kulakovskaya, T.: Phosphorus storage in microorganisms: Diversity and evolutionary insight, Biochem. Physiol. Open Access, 4, 1–4, https://doi.org/10.4172/2168-9652.1000e130, 2014.
Langlet, D., Bouchet, V. M. P., Riso, R., Matsui, Y., Suga, H., Fujiwara, Y., and Nomaki, H.: Foraminiferal ecology and role in nitrogen benthic cycle in the hypoxic Southeastern Bering Sea, Front. Mar. Sci., 7, 843, https://doi.org/10.3389/FMARS.2020.582818/BIBTEX, 2020.
Lee, J. J.: Nutrition and physiology of the foraminifera, in: Biochemistry and Physiology of Protozoa, edited by: Levandowsky, M. and Hutner, S., Academic Press, Inc., New York, ISBN-13 978-0124446014, 1980.
Lee, J. J. and Anderson, O. R.: Symbiosis in foraminfera, in: Biology of Foraminifera, edited by: Lee, J. J. and Anderson, O. R., Academic Press, London, 157–220, 1991.
Lee, J. J., Lanners, E., and Kuile, B. Ter: The retention of chloroplasts by the foraminifer Elphidium crispum, Symbiosis, 5, 45–59, 1988.
Lekieffre, C., Jauffrais, T., Geslin, E., Jesus, B., Bernhard, J. M., Giovani, M. E., and Meibom, A.: Inorganic carbon and nitrogen assimilation in cellular compartments of a benthic kleptoplastic foraminifer, Sci. Rep., 8, 1–12, https://doi.org/10.1038/s41598-018-28455-1, 2018.
LeKieffre, C., Spangenberg, J. E., Mabilleau, G., Escrig, S., Meibom, A., and Geslin, E.: Surviving anoxia in marine sediments: The metabolic response of ubiquitous benthic foraminifera (Ammonia tepida), PLoS One, 12, e0177604, https://doi.org/10.1371/journal.pone.0177604, 2017.
LeKieffre, C., Bernhard, J. M., Mabilleau, G., Filipsson, H. L., Meibom, A., and Geslin, E.: An overview of cellular ultrastructure in benthic foraminifera: New observations of rotalid species in the context of existing literature, Mar. Micropaleontol., 138, 12–32, https://doi.org/10.1016/j.marmicro.2017.10.005, 2018.
Levin, L. A., Ekau, W., Gooday, A. J., Jorissen, F., Middelburg, J. J., Naqvi, S. W. A., Neira, C., Rabalais, N. N., and Zhang, J.: Effects of natural and human-induced hypoxia on coastal benthos, Biogeosciences, 6, 2063–2098, https://doi.org/10.5194/bg-6-2063-2009, 2009.
Linke, P. and Lutze, G. F.: Microhabitat preferences of benthic foraminifera – a static concept or a dynamic adaptation to optimize food acquisition?, Mar. Micropaleontol., 20, 215–234, https://doi.org/10.1016/0377-8398(93)90034-U, 1993.
Lipps, J. H. and Valentine, J. W.: The role of foraminifera in the trophic structure of marine communities, Lethaia, 3, 279–286, https://doi.org/10.1111/j.1502-3931.1970.tb01271.x, 1970.
Lopez, E.: Algal chloroplasts in the protoplasm of three species of benthic foraminifera: taxonomic affinity, viability and persistence, Mar. Biol., 53, 201–211, https://doi.org/10.1007/BF00952427/METRICS, 1979.
Lu, Z. and Imlay, J. A.: When anaerobes encounter oxygen: mechanisms of oxygen toxicity, tolerance and defence, Nat. Rev. Microbiol., 19, 774, https://doi.org/10.1038/S41579-021-00583-Y, 2021.
Lu, Z., Hoogakker, B. A. A., Hillenbrand, C.-D., Zhou, X., Thomas, E., Gutchess, K. M., Lu, W., Jones, L., and Rickaby, R. E. M.: Oxygen depletion recorded in upper waters of the glacial Southern Ocean, Nat. Commun., 7, 11146, https://doi.org/10.1038/ncomms11146, 2016.
Lutze, G. F. and Thiel, H.: Epibenthic foraminifera from elevated microhabitats; Cibicidoides wuellerstorfi and Planulina ariminensis, J. Foraminifer. Res., 19, 153–158, https://doi.org/10.2113/gsjfr.19.2.153, 1989.
Mackensen, A., Schmiedl, G., Harloff, J., and Giese, M.: Deep-sea foraminifera in the South Atlantic Ocean; ecology and assemblage generation, Micropaleontology, 41, 342–358, 1995.
Manheim, F., Rowe, G. T., and Jipa, D.: Marine phosphorite formation off Peru, J. Sediment. Res., 45, 243–251, https://doi.org/10.1306/212F6D20-2B24-11D7-8648000102C1865D, 1975.
McCorkle, D. C. and Emerson, S. R.: The relationship between pore water carbon isotopic composition and bottom water oxygen concentration, Geochim. Cosmochim. Ac., 52, 1169–1178, https://doi.org/10.1016/0016-7037(88)90270-0, 1988.
Mckenney, D. J., Drury, C. F., Findlay, W. I., Mutus, B., McDonnell, T., and Gajda, C.: Kinetics of denitrification by Pseudomonas fluorescens: Oxygen effects, Soil Biol. Biochem., 26, 901–908, https://doi.org/10.1016/0038-0717(94)90306-9, 1994.
Mojtahid, M., Jorissen, F., Lansard, B., and Fontanier, C.: Microhabitat selection of benthic foraminifera in sediments off the Rhône river mouth (NW Mediterranean), J. Foraminifer. Res., 40, 231–246, https://doi.org/10.2113/GSJFR.40.3.231, 2010.
Nardelli, M. P., Barras, C., Metzger, E., Mouret, A., Filipsson, H. L., Jorissen, F., and Geslin, E.: Experimental evidence for foraminiferal calcification under anoxia, Biogeosciences, 11, 4029–4038, https://doi.org/10.5194/bg-11-4029-2014, 2014.
Natland, M. L.: New species of Foraminifera from off the west coast of North America and from the later Tertiary of the Los Angeles basin, Bull. Scripps Inst. Oceanogr. Univ. California, Tech. Ser., 1938.
Ní Fhlaithearta, S., Fontanier, C., Jorissen, F., Mouret, A., Dueñas-Bohórquez, A., Anschutz, P., Fricker, M. B., Günther, D., de Lange, G. J., and Reichart, G.-J.: Manganese incorporation in living (stained) benthic foraminiferal shells: a bathymetric and in-sediment study in the Gulf of Lions (NW Mediterranean), Biogeosciences, 15, 6315–6328, https://doi.org/10.5194/bg-15-6315-2018, 2018.
Nomaki, H., Heinz, P., Nakatsuka, T., Shimanaga, M., and Kitazato, H.: Species-specific ingestion of organic carbon by deep-sea benthic foraminifera and meiobenthos: In situ tracer experiments, Limnol. Oceanogr., 50, 134–146, https://doi.org/10.4319/lo.2005.50.1.0134, 2005.
Nomaki, H., Heinz, P., Nakatsuka, T., Shimanaga, M., Ohkouchi, N., Ogawa, N. O., Kogure, K., Ikemoto, E., and Kitazato, H.: Different ingestion patterns of 13C-labeled bacteria and algae by deep-sea benthic foraminifera, Mar. Ecol. Prog. Ser., 310, 95–108, https://doi.org/10.3354/MEPS310095, 2006.
Nomaki, H., Ogawa, N., Ohkouchi, N., Suga, H., Toyofuku, T., Shimanaga, M., Nakatsuka, T., and Kitazato, H.: Benthic foraminifera as trophic links between phytodetritus and benthic metazoans: carbon and nitrogen isotopic evidence, Mar. Ecol. Prog. Ser., 357, 153–164, https://doi.org/10.3354/meps07309, 2008.
Nomaki, H., Ogawa, N. O., Takano, Y., Suga, H., Ohkouchi, N., and Kitazato, H.: Differing utilization of glucose and algal particulate organic matter by deep-sea benthic organisms of Sagami Bay, Japan, Mar. Ecol. Prog. Ser., 431, 11–24, 2011.
Nomaki, H., Chikaraishi, Y., Tsuchiya, M., Toyofuku, T., Ohkouchi, N., Uematsu, K., Tame, A., and Kitazato, H.: Nitrate uptake by foraminifera and use in conjunction with endobionts under anoxic conditions, Limnol. Oceanogr., 59, 1879–1888, https://doi.org/10.4319/lo.2014.59.6.1879, 2014.
Nomaki, H., Chikaraishi, Y., Tsuchiya, M., Toyofuku, T., Suga, H., Sasaki, Y., Uematsu, K., Tame, A., and Ohkouchi, N.: Variation in the nitrogen isotopic composition of amino acids in benthic foraminifera: Implications for their adaptation to oxygen-depleted environments, Limnol. Oceanogr., 60, 1906–1916, https://doi.org/10.1002/lno.10140, 2015.
Nomaki, H., Bernhard, J.M., Ishida, A., Tsuchiya, M., Uematsu, K., Tame, A., Kitahashi, T., Takahata, N., Sano, Y. and Toyofuku, T.: Intracellular isotope localization in Ammonia sp. (Foraminifera) of oxygen-depleted environments: Results of nitrate and sulfate labeling experiments, Front. Microbiol., 7, 163, https://doi.org/10.3389/fmicb.2016.00163, 2016.
de Nooijer, L. J., Spero, H. J., Erez, J., Bijma, J., and Reichart, G. J.: Biomineralization in perforate foraminifera, Earth-Sci. Rev., 135, 48–58, https://doi.org/10.1016/j.earscirev.2014.03.013, 2014.
Oakley, B. B., Francis, C. A., Roberts, K. J., Fuchsman, C. A., Srinivasan, S., and Staley, J. T.: Analysis of nitrite reductase (nirK and nirS) genes and cultivation reveal depauperate community of denitrifying bacteria in the Black Sea suboxic zone, Environ. Microbiol., 9, 118–130, https://doi.org/10.1111/j.1462-2920.2006.01121.x, 2007.
Orsi, W. D., Morard, R., Vuillemin, A., Eitel, M., Wörheide, G., Milucka, J., and Kucera, M.: Anaerobic metabolism of Foraminifera thriving below the seafloor, ISME J., 14, 2580–2594, https://doi.org/10.1038/s41396-020-0708-1, 2020.
Panagiota-Chronopoulou, M., Salonen, I., Bird, C., Reichart, G. J., and Koho, K. A.: Metabarcoding insights into the trophic behavior and identity of intertidal benthic foraminifera, Front. Microbiol., 10, https://doi.org/10.3389/FMICB.2019.01169/FULL, 2019.
Papagiannakis, E., Van Stokkum, I. H. M., Fey, H., Büchel, C., and Van Grondelle, R.: Spectroscopic characterization of the excitation energy transfer in the fucoxanthin-chlorophyll protein of diatoms, Photosynth. Res., 86, 241–250, https://doi.org/10.1007/S11120-005-1003-8/METRICS, 2005.
Pillet, L., de Vargas, C., and Pawlowski, J.: Molecular identification of sequestered diatom chloroplasts and kleptoplastidy in foraminifera, Protist, 162, 394–404, https://doi.org/10.1016/j.protis.2010.10.001, 2011.
Pillet, L., Voltski, I., Korsun, S., and Pawlowski, J.: Molecular phylogeny of Elphidiidae (foraminifera), Mar. Micropaleontol., 103, 1–14, https://doi.org/10.1016/J.MARMICRO.2013.07.001, 2013.
Piña-Ochoa, E., Koho, K. A., Geslin, E., and Risgaard-Petersen, N.: Survival and life strategy of the foraminiferan Globobulimina turgida through nitrate storage and denitrification, Mar. Ecol. Prog. Ser., 417, 39–49, https://doi.org/10.3354/meps08805, 2010a.
Piña-Ochoa, E., Høgslund, S., Geslin, E., Cedhagen, T., Revsbech, N. P., Nielsen, L. P., Schweizer, M., Jorissen, F., Rysgaard, S., and Risgaard-Petersen, N.: Widespread occurrence of nitrate storage and denitrification among foraminifera and gromiida, P. Natl. Acad. Sci. USA, 107, 1148–1153, https://doi.org/10.1073/pnas.0908440107, 2010b.
Powers, C., Gomaa, F., Billings, E. B., Utter, D. R., Beaudoin, D. J., Edgcomb, V. P., Hansel, C. M., Wankel, S. D., Filipsson, H. L., Zhang, Y., and Bernhard, J. M.: Two canonically aerobic foraminifera express distinct peroxisomal and mitochondrial metabolisms, Front. Mar. Sci., 9, 2423, https://doi.org/10.3389/FMARS.2022.1010319/BIBTEX, 2022.
Premvardhan, L., Sandberg, D. J., Fey, H., Birge, R. R., Büchel, C., and Van Grondelle, R.: The charge-transfer properties of the S2 state of fucoxanthin in solution and in fucoxanthin chlorophyll-a/c2 protein (FCP) based on stark spectroscopy and molecular-orbital theory, J. Phys. Chem. B, 112, 11838–11853, https://doi.org/10.1021/JP802689P/SUPPL_ FILE/JP802689P-FILE003.PDF, 2008.
Rathburn, A. E., Willingham, J., Ziebis, W., Burkett, A. M., and Corliss, B. H.: A New biological proxy for deep-sea paleo-oxygen: Pores of epifaunal benthic foraminifera, Sci. Rep., 8, 9456, https://doi.org/10.1038/s41598-018-27793-4, 2018.
Reichart, G.-J., Jorissen, F., Anschutz, P., and Mason, P. R. D.: Single foraminiferal test chemistry records the marine environment, Geology, 3, 355–358, 2003.
Richirt, J., Schweizer, M., Bouchet, V. M. P., Mouret, A., Quinchard, S., and Jorissen, F. J.: Morphological distinction of three Ammonia phylotypes occurring along European Coasts, J. Foraminifer. Res., 49, 76–93, https://doi.org/10.2113/GSJFR.49.1.76, 2019.
Risgaard-Petersen, N., Langezaal, A. M., Ingvardsen, S., Schmid, M. C., Jetten, M. S. M., Op Den Camp, H. J. M., Derksen, J. W. M., Piña-Ochoa, E., Eriksson, S. P., Nielsen, L. P., Revsbech, N. P., Cedhagen, T., and Van Der Zwaan, G. J.: Evidence for complete denitrification in a benthic foraminifer, Nature, 443, 93–96, https://doi.org/10.1038/nature05070, 2006.
Ross, B. J. and Hallock, P.: Dormancy in the foraminifera: A review, J. Foraminifer. Res., 46, 358–368, https://doi.org/10.2113/GSJFR.46.4.358, 2016.
Rybarczyk, H., Elkaim, B., Wilson, J., and Loquet, N.: L'eutrophisation en Baie de Somme: mortalités des peuplements benthiques par anoxie, Oceanol. Ac., 19, 131–140, 1996.
Sandberg, E.: Does short-term oxygen depletion affect predator-prey relationships in zoobenthos?, Experiments with the isopod Saduria entomon, Mar. Ecol. Prog. Ser., 103, 73–80, 1994.
Schmiedl, G. and Mackensen, A.: Multispecies stable isotopes of benthic foraminifers reveal past changes of organic matter decomposition and deepwater oxygenation in the Arabian Sea, Paleoceanography, 21, 1–14, https://doi.org/10.1029/2006PA001284, 2006.
Schulz, H. N. and Schulz, H. D.: Large sulfur bacteria and the formation of phosphorite, Science, 307, 416–418, https://doi.org/10.1126/science.1103096, 2005.
Schweizer, M., Jauffrais, T., Choquel, C., Méléder, V., Quinchard, S., and Geslin, E.: Trophic strategies of intertidal foraminifera explored with single-cell microbiome metabarcoding and morphological methods: What is on the menu?, Ecol. Evol., 12, e9437, https://doi.org/10.1002/ece3.9437, 2022.
Smith, M. S. and Tiedje, J. M.: Phases of denitrification following oxygen depletion in soil, Soil Biol. Biochem., 11, 261–267, https://doi.org/10.1016/0038-0717(79)90071-3, 1979.
Tetard, M., Licari, L., Ovsepyan, E., Tachikawa, K., and Beaufort, L.: Toward a global calibration for quantifying past oxygenation in oxygen minimum zones using benthic Foraminifera, Biogeosciences, 18, 2827–2841, https://doi.org/10.5194/bg-18-2827-2021, 2021.
Thibault de Chanvalon, A., Metzger, E., Mouret, A., Cesbron, F., Knoery, J., Rozuel, E., Launeau, P., Nardelli, M. P., Jorissen, F. J., and Geslin, E.: Two-dimensional distribution of living benthic foraminifera in anoxic sediment layers of an estuarine mudflat (Loire estuary, France), Biogeosciences, 12, 6219–6234, https://doi.org/10.5194/bg-12-6219-2015, 2015.
Tiedje, J.: Ecology of denitrification and dissimilatory nitrate reduction to ammonium, in: Methods of Soil Analysis, Part 2. Chemical and Microbiological Properties, 717, 179–244, 1988.
Toyofuku, T., Matsuo, M. Y., de Nooijer, L. J., Nagai, Y., Kawada, S., Fujita, K., Reichart, G.-J., Nomaki, H., Tsuchiya, M., Sakaguchi, H., and Kitazato, H.: Proton pumping accompanies calcification in foraminifera, Nat. Commun., 8, 14145, https://doi.org/10.1038/ncomms14145, 2017.
Tsaousis, A. D., Nývltová, E., Šuták, R., Hrdý, I., and Tachezy, J.: A nonmitochondrial hydrogen production in Naegleria gruberi, Genome Biol. Evol., 6, 792–799, https://doi.org/10.1093/GBE/EVU065, 2014.
Tsuchiya, M., Toyofuku, T., Uematsu, K., Brüchert, V., Collen, J., Yamamoto, H., and Kitazato, H.: Cytologic and genetic characteristics of endobiotic bacteria and kleptoplasts of Virgulinella fragilis (Foraminifera), J. Eukaryot. Microbiol., 62, 454–469, https://doi.org/10.1111/jeu.12200, 2015.
Usuda, K., Toritsuka, N., Matsuo, Y., Kim, D. H., and Shoun, H.: Denitrification by the fungus Cylindrocarpon tonkinense: anaerobic cell growth and two isozyme forms of cytochrome P-450nor., Appl. Environ. Microbiol., 61, 883–889, 1995.
Winkelbauer, H., Cordova-Rodriguez, K., Reyes-Macaya, D., Scott, J., Glock, N., Lu, Z., Hamilton, E., Chenery, S., Holdship, P., Dormon, C., and Hoogakker, B.: Foraminifera iodine to calcium ratios: Approach and cleaning, Geochem. Geophys. Geosys., 22, e2021GC009811, https://doi.org/10.1029/2021GC009811, 2021.
Woehle, C., Roy, A. S., Glock, N., Wein, T., Weissenbach, J., Rosenstiel, P., Hiebenthal, C., Michels, J., Schönfeld, J., and Dagan, T.: A novel eukaryotic denitrification pathway in foraminifera, Curr. Biol., 28, 2536–2543, https://doi.org/10.1016/j.cub.2018.06.027, 2018.
Woehle, C., Roy, A.-S., Glock, N., Michels, J., Wein, T., Weissenbach, J., Romero, D., Hiebenthal, C., Gorb, S., Schönfeld, J., and Dagan, T.: Denitrification in foraminifera has an ancient origin and is complemented by associated bacteria, P. Natl. Acad. Sci. USA, 119, e2200198119, https://doi.org/10.1073/pnas.2200198119, 2022.
Wollenburg, J. E., Zittier, Z. M. C., and Bijma, J.: Insight into deep-sea life – Cibicidoides pachyderma substrate and pH-dependent behaviour following disturbance, Deep. Res. Pt. I, 138, 34–45, https://doi.org/10.1016/j.dsr.2018.07.006, 2018.
Wollenburg, J. E., Bijma, J., Cremer, C., Bickmeyer, U., and Zittier, Z. M. C.: Permanent ectoplasmic structures in deep-sea Cibicides and Cibicidoides taxa – long-term observations at in situ pressure, Biogeosciences, 18, 3903–3915, https://doi.org/10.5194/bg-18-3903-2021, 2021.
Xu, Z., Liu, S., Xiang, R., and Song, G.: Live benthic foraminifera in the Yellow Sea and the East China Sea: vertical distribution, nitrate storage, and potential denitrification, Mar. Ecol. Prog. Ser., 571, 65–81, https://doi.org/10.3354/meps12135, 2017.
Xu, Z., Liu, S., and Ning, X.: Potential foraminiferal nitrate transport in sediments in contact with oxic overlying water, Limnol. Oceanogr., 66, 1510–1530, https://doi.org/10.1002/lno.11701, 2021.
Zhou, X., Thomas, E., Rickaby, R. E. M., Winguth, A. M. E., and Lu, Z.: evidence for upper ocean deoxygenation during the PETM, Paleoceanography, 29, 964–975, https://doi.org/10.1002/2014PA002702, 2014.
Zhou, X., Hess, A. V., Bu, K., Sagawa, T., and Rosenthal, Y.: Simultaneous determination of and other elemental ratios in foraminifera: Comparing results from acidic and basic solutions, Geochem., Geophys. Geosys., 23, e2022GC010660, https://doi.org/10.1029/2022GC010660, 2022.
Zimorski, V., Mentel, M., Tielens, A. G. M., and Martin, W. F.: Energy metabolism in anaerobic eukaryotes and Earth's late oxygenation, Free Radic. Biol. Med., 140, 279–294, https://doi.org/10.1016/j.freeradbiomed.2019.03.030, 2019.
The requested paper has a corresponding corrigendum published. Please read the corrigendum first before downloading the article.
- Article
(4252 KB) - Full-text XML