the Creative Commons Attribution 4.0 License.
the Creative Commons Attribution 4.0 License.
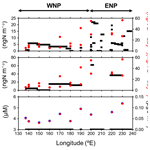
Marine nitrogen fixation as a possible source of atmospheric water-soluble organic nitrogen aerosols in the subtropical North Pacific
Tsukasa Dobashi
Eri Tachibana
Kazutaka Takahashi
Sachiko Horii
Fuminori Hashihama
Saori Yasui-Tamura
Yoko Iwamoto
Shu-Kuan Wong
Koji Hamasaki
Water-soluble organic nitrogen (WSON) in marine atmospheric aerosols affect the water solubility, acidity, and light-absorbing properties of aerosol particles, which are important parameters in assessing both the climate impact and the biogeochemical cycling of bioelements. Size-segregated aerosol and surface seawater (SSW) samples were simultaneously collected over the subtropical North Pacific to investigate the origin of WSON in the marine atmosphere. The fine-mode WSON concentration (7.5 ± 6.6 ngN m−3) at 200–240∘ E along 23∘ N, defined as the eastern North Pacific (ENP), was significantly higher than that (2.4 ± 1.9 ngN m−3) at 135–200∘ E, defined as the western North Pacific (WNP). Analysis of the stable carbon isotope ratio of water-soluble organic carbon (WSOC; δ13CWSOC) together with backward trajectory indicated that most of the observed WSON in the fine particles in the ENP originated from the ocean surface. We found positive relations among nitrogen-fixation rate, dissolved organic nitrogen (DON) in SSW, and the WSON concentrations. The result suggests that reactive nitrogen (DON and ammonium), produced and exuded by nitrogen-fixing microorganisms in SSW, contributed to the formation of WSON aerosols. This study provides new insights into the role of ocean-derived reactive nitrogen aerosols associated with marine microbial activity.
- Article
(1139 KB) -
Supplement
(1250 KB) - BibTeX
- EndNote
Ocean-derived atmospheric organic aerosols (OAs) play an essential role in cloud formation processes and subsequently impact radiation over the open ocean (Rosenfeld et al., 2019). Therefore, it is essential to understand the formation process, composition, and emission flux of marine aerosols to assess the ocean-to-cloud relationship and its impact on climate (Oreopoulos et al., 2008; Salter et al., 2008). In addition to primary emissions of OAs (POAs), the origin and formation processes of secondary OAs (SOAs) from the ocean surface remain unclear. Brüggemann et al. (2018) indicated that additional SOAs from the oxidation of photochemically active precursors, such as volatile organic compounds (VOCs) from the ocean surface, are important, especially in tropical and/or subtropical regions with low POA concentrations; such SOAs contribute up to 60 % of additional OA mass. However, field measurements of marine SOAs have been limited.
Regarding the chemical components found in marine organic aerosols, water-soluble organic nitrogen (WSON) can affect the hygroscopicity, light-absorbing properties, and acidity of OAs, as well as the global biogeochemical cycling of nitrogen (Mohr et al., 2013; van Pinxteren et al., 2017; Facchini et al., 2008; Nehir and Koçak, 2018). Miyazaki et al. (2011) demonstrated that marine organic aerosols are enriched in ON from marine biological origins in the western North Pacific. Altieri et al. (2016) also suggested the importance of a marine biogenic sources of aerosol WSON in the western North Atlantic, leading to the conclusion that only 27 % of total nitrogen (TN) deposition to the global ocean is anthropogenic, which is significantly less than the previously estimated values of 48 %–80 % (Duce et al., 2008; Kanakidou et al., 2012). The ocean has been recognized to act as a source of atmospheric ON (e.g., alkyl nitrates, aliphatic amines, and urea) and ammonia (NH3); the chemical compositions of atmospheric ON include a wide variety of functional groups (Altieri et al., 2016). However, the origin and formation process of WSON aerosols, particularly those of marine atmospheric aerosols, remain unclear.
There is also substantial uncertainty in our understanding of the linkage between the formation of aerosol WSON and marine phytoplankton at the sea surface. Among the major groups of marine phytoplankton, cyanobacteria are the dominant primary producers in subtropical gyres (Rousseaux et al., 2012; Ottesen et al., 2014), which occupy one-third of Earth's surface. In particular, N2-fixing microorganisms, including cyanobacteria, are widely distributed in the subtropical North Pacific (Cheung et al., 2020; Zehr et al., 2020), which is a primarily oligotrophic area with a weak vertical supply of nitrate from below the euphotic layer (Shiozaki et al., 2017; Yamaguchi et al., 2021). In this oceanic region, N2 fixation by marine microorganisms such as Trichodesmium, a genus of filamentous cyanobacteria, is expected to be a possible source of reactive nitrogen in the atmosphere (Frischkorn et al., 2018). However, the effects of N2-fixing microorganisms on the sea-to-air emissions of reactive nitrogen species, including WSON, have not yet been investigated.
The present study aimed to elucidate the origin and formation process of WSON aerosols in the oligotrophic subtropical ocean, particularly their linkage with N2-fixing microorganisms in surface seawater. We measured WSON and other reactive nitrogen species in size-segregated aerosols collected on board a research vessel sailing over the subtropical North Pacific along the longitudinal transect in summer 2017. The discussion is extended to comparisons of the observed nitrogen aerosols with N2-fixation rates in surface seawater (SSW) to investigate possible sources of WSON over the oceanic region.
2.1 Aerosol sampling
Ambient aerosol sampling was conducted from 12 August to 5 October 2017 on board the research vessel Hakuho Maru (Hashihama et al., 2020; Yamaguchi et al., 2021). Sampling was carried out during cruise KH–17–4 from Vancouver to Tokyo via Honolulu in the subtropical North Pacific (Fig. 1). The aerosol samples were collected using a high-volume air sampler (HVAS; model 120SL, Kimoto Electric, Osaka, Japan) located on the deck above the bridge of the ship. A cascade impactor (CI; model TE-234, Tisch Environmental, Cleves, OH, USA) was attached to the HVAS to collect size-segregated particles at a flow rate of ∼1130 L min−1, without temperature or humidity control. In the present study, we used analytical results obtained from the one bottom stage and four upper stages of the impactor, which collected particles with aerodynamic diameter (Dp)<0.95 µm every 12 or 24 h and Dp>0.95 µm every 96 h, respectively. Aerosol particles collected at the bottom and upper stages were referred to as fine and coarse particles, respectively. To avoid possible contamination from the ship exhaust during aerosol sampling, the sampling pump of the HVAS was shut off when the relative wind direction was out of ±60∘ to the bow and/or when the relative wind speed was low (<5 m s−1).
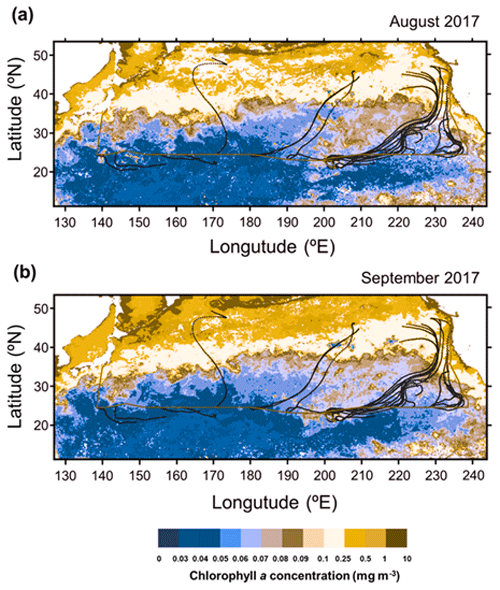
Figure 1R/V Hakuho Maru cruise track in the subtropical North Pacific between 12 August and 5 October 2017 (red), together with typical 5 d back trajectories (black). Also shown are monthly averaged concentrations of Chl a for (a) August and (b) September 2017, derived from the MODIS-Aqua (https://neo.gsfc.nasa.gov/view.php?datasetId=MY1DMM_CHLORA, last access: 28 June 2022). Area in which data are missing is shown in white.
The aerosol samples were collected on quartz fiber filters (25 cm × 20 cm), which were precombusted at 450 ∘C for 6 h to remove any contaminants. Each collected filter was stored in a glass jar with a Teflon-lined screwed cap at −20 ∘C to limit chemical reactions on the filter and losses of volatile compounds. In this study, aerosol samples collected with a total air volume <100 m3 were not used. In total, 51 and 9 samples are presented for the fine and coarse particles, respectively. These numbers accounted for 88 % and 82 % of the total numbers of fine and coarse aerosol samples collected, respectively.
2.2 Surface seawater (SSW) sampling and chlorophyll (Chl) a analysis
SSW samples were collected overboard at the depth of 0 m using an acid-cleaned bucket during each aerosol-sampling duration. The average temperature of SSW at each sampling point was 26.1 ± 2.1 ∘C. In total, analytical results of 12 SSW samples obtained along 23∘ N were shown in the present study. The SSW samples for Chl a analysis were filtered using 25 mm Whatman GF/F filters (GE Healthcare, Buckinghamshire, UK). Chl a concentrations were further measured fluorometrically using 10-AU™ field and laboratory fluorometers (Turner Designs, Sunnyvale, CA) after extraction with N′, N′-dimethylformamide (Suzuki and Ishimaru, 1990).
2.3 Chemical analysis of water-soluble aerosols and elemental carbon
To measure the concentrations of WSOC and water-soluble total nitrogen (WSTN) in the aerosol filter samples, a filter cut of 19.63 cm2 was extracted with 15 mL of ultrapure water under ultrasonication and filtered using a disk filter (Millex-GV, 0.22 µm, Millipore, Billerica, MA, USA). The concentrations of WSOC and WSTN were determined using a total organic carbon (TOC) analyzer with a TN unit (model TOC-LCHP+TNM-L, SHIMADZU). Additionally, another cut of the filter (7.07 cm2) was extracted with 10 mL of ultrapure water to measure the concentrations of Na+, NH, NO, NO, and methanesulfinic acid (MSA). The same syringe filter type as described above was used before the extract was injected into an ion chromatograph (model 761 compact IC; Metrohm). Here, the WSON concentration was defined as the difference between the WSTN and inorganic nitrogen (NH, NO, NO) concentrations. As an anthropogenic tracer, mass concentrations of elemental carbon (EC) were measured using a Sunset Lab carbon analyzer. A filter punch of 1.54 cm2 was used for the analysis of EC.
2.4 Stable carbon isotopic characterization of water-soluble organic aerosols
To determine the δ13C of WSOC (δ13CWSOC) in the collected aerosols, a portion of the filter (9.08 cm2) was extracted with 20 mL of ultrapure water. The extracted samples were then concentrated via rotary evaporation, and 40 µL of each sample were transferred to be absorbed onto 15 mg of precombusted Chromosorb in a precleaned tin cup. The 13CWSOC was then measured using an elemental analyzer (Flash EA 1112) and continuous flow carrier gas system (ConFlo)–isotope ratio mass spectrometer (Delta V, Thermo Finnigan) to determine the δ13C of WSOC (δ13CWSOC) (Miyazaki et al., 2012). The 13C data were reported relative to an established reference of carbon Vienna PeeDee Belemnite (VPDB).
2.5 Estimation of nitrogen-fixation rate
Primary production and N2-fixation rates were determined using methods previously reported (Shiozaki et al., 2017), which followed Mohr et al. (2010) and Hama et al. (1983), respectively. The SSW samples were collected directly from an acid-cleaned bucket into acid-cleaned 4.5 L polycarbonate bottles. The SSW samples to estimate the initial 15N and 13C enrichment of particulate organic matter (POM) were filtered immediately onto precombusted GF/F filters. For incubation, 13C-labeled sodium bicarbonate (99 atom % 13C; Cambridge Isotope Laboratories, Inc.) was added to each duplicate bottle at a final concentration of 200 µmol L−1. The 110 mL of 15N2-enriched SSW, in which 1.1 mL of 15N2 gas (>99 atom % 15N, Shoko Science) was dissolved using a Sterapore membrane unit (20M1500A: Mitsubishi Rayon Co., Ltd.), was added to each bottle. The samples were then incubated for 24 h using an on-deck incubator with surface SSW running continuously. The incubation was terminated by gentle vacuum filtration of the SSW samples through precombusted GF/F filters. The filters were then frozen at −20 ∘C until the post-measurement on the ground. The filter samples were dried at 50 ∘C in an oven and were exposed to hydrogen chloride (HCl) fumes for 2 h to remove inorganic carbon, followed by being dried at 50 ∘C again. The N and C contents and their stable isotope ratios were then determined using a DELTA V advantage mass spectrometer (Thermo Electron, USA) connected to an elemental analyzer. N2-fixation activity was regarded to be significant when the atom % of 15N for each incubated bottle was higher than that for the initial sample by 0.00146 atom % (Montoya et al., 1996). Primary production and N2-fixation rates were determined twice at each sampling station.
2.6 Determination of dissolved inorganic and organic nitrogen
Surface seawater samples for the determination of dissolved inorganic and organic nitrogen were collected at 10 m depth using a conductivity–temperature–depth (CTD) system (Sea-Bird Electronics) equipped with acid-cleaned Niskin-X bottles (General Oceanics; Hashihama et al., 2020). Total dissolved nitrogen (TDN) was measured by a persulfate oxidation method with a gas-segmented continuous flow analyzer (Yasui-Tamura et al., 2020). Nanomolar concentrations of NH and NO NO in seawater were determined by using sensitive liquid waveguide spectrophotometry (Hashihama et al., 2009, 2015). Dissolved organic nitrogen (DON) concentration was determined as the difference between the TDN and dissolved inorganic nitrogen (DIN = NH NO NO) concentrations in surface seawater.
Figure 2a and b show the longitudinal distribution of the concentrations of WSON in fine particles (WSONF) with particle diameter (Dp) <0.95 µm and in coarse particles (WSONC) with Dp>0.95 µm from samples collected at 23∘ N in the subtropical North Pacific (Fig. 1). The average concentrations of WSONF and WSONC were 6.1 and 22 ± 21 ngN m−3, respectively, during the cruise measurement. The sum of these values is within range of the WSON concentrations in total suspended particles (TSP) collected over the western North Pacific (3.0–35 ngN m−3; Duce et al., 2008). Here, along the cruise track at 23∘ N, the oceanic region at 200–240∘ E is defined as the eastern North Pacific (ENP), whereas the region at 135–200∘ E is defined as the western North Pacific (WNP). Concentrations of WSON in both the fine and coarse particles showed a distinct longitudinal gradient, with substantially higher concentrations in ENP. The average concentrations of WSONF and WSONC in ENP were approximately 3–6 times as large as those in the WNP (Table 1).
Table 1Average concentrations of nitrogen species and stable carbon isotope ratios in the fine (Dp<0.95 µm) and coarse (Dp>0.95 µm) aerosol particles collected along 23∘ N in each oceanic region.
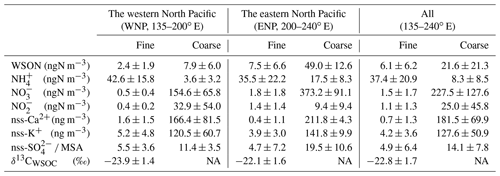
NA: not available.
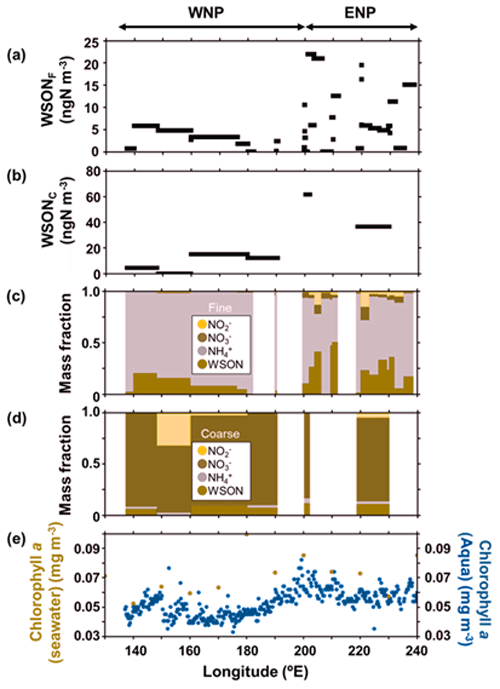
Figure 2Longitudinal distributions of each parameter of the atmospheric aerosols and surface seawater along 23∘ N; the mass concentrations of WSON in (a) fine particles (WSONF) and (b) coarse particles (WSONC); the chemical mass fractions of nitrogen species in (c) WSONF and (d) WSONC; (e) Chl a concentrations in the surface seawater samples during the cruise (ocher) and the average Chl a concentrations from August–September 2017, as measured by MODIS-Aqua (blue). WNP and ENP denote the oceanic regions of the western North Pacific and eastern North Pacific, respectively, defined in this study.
The longitudinal distributions of the mass concentrations of inorganic nitrogen (NH, NO, and NO) generally showed similar patterns to those of WSON (Fig. S1). The sum of the mass concentrations of the inorganic nitrogen in ENP was approximately twice as large as those in WNP. Overall, NH-N was the dominant component of water-soluble total nitrogen (WSTN) (∼ 78 %) in the fine-mode aerosols, followed by WSON (16 %) (Fig. 2c). In contrast, NO-N was the most abundant WSTN component of the coarse-mode aerosols, which accounted for 83 % of the WSTN mass on average. Notably, the mass fraction of WSON in ENP was significant, accounting for up to ∼ 50 % (average: 19 ± 15 %) and ∼ 11 % of the WSTN in the fine- and coarse-particle masses, respectively (Fig. 2c and d). This result indicates the importance of the abundance of WSON, particularly in the fine particles in ENP.
Figure 2e presents the concentrations of chlorophyll (Chl) a in surface seawater (SSW) samples obtained during the cruise, as well as the average concentrations of Chl a during August–September 2017 derived from the MODIS-Aqua ocean color data (https://neo.gsfc.nasa.gov/view.php?datasetId=MY1DMM_CHLORA, last access: 28 June 2022). The two different measurements of Chl a generally showed similar longitudinal distributions, which resemble the longitudinal trend of the WSON concentrations. Moreover, 5 d back trajectories, calculated by HYSPLIT (https://www.ready.noaa.gov/HYSPLIT_traj.php, last access: 28 June 2022), showed that the sampled air masses were transported primarily over the oceanic regions in the Pacific (Fig. 1). These results suggest that most of the observed aerosols were transported within the marine boundary layer with less influence from terrestrial sources prior to sampling. This is supported by the concentrations of nss-Ca2+ and nss-K+, as well as of nss-SO methanesulfonic acid (MSA) ratios which did not show significant differences between WNP and ENP (Table 1). The overall results suggested that the observed aerosols, particularly those in ENP, were largely influenced by marine sources associated with phytoplankton.
Previously, a method using stable carbon isotope ratios (δ13C) of aerosol organic carbon was successfully used to determine the contributions of marine and terrestrial sources to organic aerosols in the marine atmosphere (Cachier et al., 1986; Miyazaki et al., 2016). The δ13C of water-soluble organic carbon (WSOC; δ13CWSOC) as a function of the WSONF concentrations is shown in Fig. 3. The average δ13CWSOC observed in this study was −22.8 ± 1.7 ‰, with 71 % of the data (12 out of 17 data points) in the range of typical marine origin (between −24 ‰ and −18 ‰; Miyazaki et al., 2016), while that of terrestrial origin is between −27 ‰ and −25 ‰ (Cachier et al., 1986; Dasari et al., 2019). This isotopic characterization supports that most of the organic carbon fractions of the observed aerosols were of marine origin.
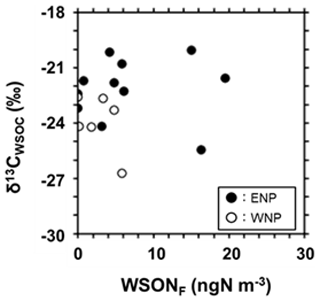
Figure 3δ13C of WSOC (δ13CWSOC) as a function of the concentrations of WSONF. Solid and open circles indicate the data of the eastern North Pacific (ENP; 200–240∘ E) and the western North Pacific (WNP; 135–200∘ E), respectively.
To elucidate the possible formation processes of the observed WSON, we used several tracers of marine origins. Figure 4a and b show scatter plots between WSON and Na+ concentrations. The concentrations of Na+, used as a tracer of marine primary emissions, did not show any significant positive correlations with those of WSONF (R2=0.04) or WSONC (R2=0.03) in the study area. Specifically, the R2 value between WSONF and Na was 0.003 in ENP, while WSONF and Na concentrations even showed a negative correlation (R2=0.49) in WNP (Fig. 4a). The correlation cannot be statistically discussed for the coarse mode separately in ENP and WNP due to the limited number of data points. Nevertheless, the WSON concentration was higher in the ENP than in the WNP for the same Na+ concentration level in the coarse particles (Fig. 4b), suggesting that WSON was more abundant relative to Na+ in coarse particles in ENP. Moreover, concentrations of glucose, a molecular tracer of marine primary aerosols (Miyazaki et al., 2018), were below the lower detection limit for most of the samples during the cruise. These results suggest insignificant contributions of direct emissions from the sea surface to the observed WSON.
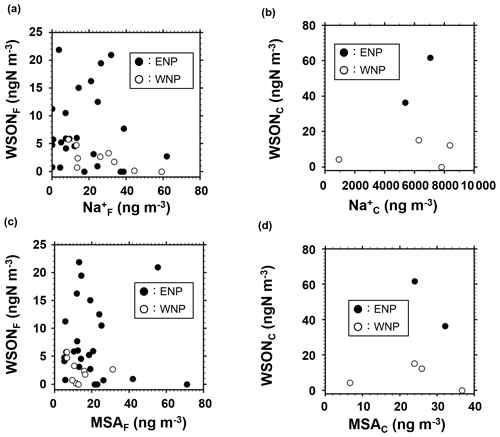
Figure 4Concentrations of WSON as functions of those of sodium (a, b) and MSA (c, d), where (a) and (c) are for the fine particles and (b) and (d) are for the coarse particles. Solid and open circles represent the data of the eastern North Pacific (ENP) and western North Pacific (WNP), respectively.
The above results also imply that secondary formation was likely the dominant process underlying the formation of WSON observed in this study. The process includes accommodation of secondary WSON onto sea salt particles. Figure 4c and d present scatter plots between WSON and MSA concentrations in each particle size category. MSA has been widely used as a tracer of marine SOAs because it is an oxidation product of dimethyl sulfate (DMS). MSA is either produced by gas-phase MSA directly scavenged by aerosols or rapidly produced in the aqueous phase from scavenged dimethylsulfoxide (DMSO) and methanesulfonic acid (MSIA) (Zhu et al., 2006). The concentrations of WSON did not show any significant correlations with those of MSA in each size range regardless of the oceanic region. The insignificant correlation suggests that the origin of the observed WSON aerosols differed from that of DMS or that the formation pathways of WSON were different from the oxidation processes of DMS. It is also possible that dependence of temperature and/or OH levels in the subtropics on the relative yields of MSA to sulfate in the oxidation of DMS (e.g., Mungall et al., 2018) might also partly affect the insignificant correlations between WSON and MSA. In fact, no relations were found between sulfate, known as an oxidation product of DMS, and WSON concentrations (R2<0.02) in fine particles (data not shown). On the other hand, sulfate and WSON concentrations in coarse particles showed some positive relationships, although the number of data is limited and the relation is not statistically significant (p=0.103). This may reflect overlapping processes of biogenic sulfate and WSON on a longer timescale (i.e., several days of sampling of coarse particles) even though the exact origins are different.
To further explore the origin and possible formation process of the observed WSON aerosols associated with phytoplankton, we focused on N2 fixation in SSW as a possible source of atmospheric reactive nitrogen. N2 fixation is the biological conversion of N2 to NH or dissolved ON (DON), which represents the main external source of bioavailable nitrogen in marine environments. A significant fraction of fixed N2 can be directly released by N2-fixing microorganisms as dissolved inorganic nitrogen (NH, NO, and NO) and DON in the ocean. Data obtained from Station ALOHA in the subtropical North Pacific have shown enrichment of nitrogen pools (i.e., NH, NO, DON) within Trichodesmium blooms (Karl et al., 1992; Letelier and Karl, 1994). It has been shown that the majority of the recently fixed N2 is released directly as DON during the growth of Trichodesmium, primarily as dissolved free amino acids (Capone et al, 1994; Glibert and Bronk, 1994). Luo et al. (2014) estimated that, on average, spatially integrated N2 fixation over the Pacific accounted for ∼ 50 % of that in the global ocean, pointing to the importance of the Pacific in terms of this process.
In the subtropical North Pacific, previously measured nitrogen isotope ratios (δ15N) in particulate organic matter (POM) from seawater suggested that POM was significantly affected by nitrogen supplied from N2-fixing microorganisms (Horii et al., 2018). Indeed, in the current study, the average δ15N of POM in the SSW samples was −0.3 ± 1.0 ‰ (data not shown), which is within the range of δ15N values of diazotrophic cyanobacteria, typically ranging from −2 ‰ to 0 ‰ (Horii et al., 2018). This indicates that the organic matter in SSW collected in the study region is derived from nitrogen supplied by N2-fixing microorganisms such as cyanobacteria.
Figure 5 shows the latitudinal distributions of the N2-fixation rate and concentrations of DON and TDN in the SSW samples compared with those of aerosol WSON concentrations in each size category. The average N2-fixation rate in the SSW samples collected in ENP was 38.6 ± 13.3 ngN L−1 d−1, which was significantly higher than that in the WNP (11.5 ± 10.6 ngN L−1 d−1). The observed range of the N2-fixation rate in SSW is similar to those reported for the same oceanic region in previous studies (Montoya et al., 2004; Bonnet et al., 2009; Yamaguchi et al., 2019). Moreover, the longitudinal gradient of the N2-fixation rate is similar to that along 23∘ N in the tropical Pacific, as shown by previous field measurements and model simulations (Luo et al., 2014; Dutheil et al., 2018; Wang et al., 2019; Shiozaki et al., 2018; Hashihama et al., 2020). The higher N2-fixation rate in ENP compared to that in WNP could be attributed to the greater abundance of N2-fixing microorganisms such as Trichodesmium and a symbiotic unicellular cyanobacterium (UCYN-A) measured by quantitative polymerase chain reaction (qPCR) of nifH. In particular, the number of the nifH copies of UCYN-A in ENP was 3 orders of magnitude larger than that in WNP (data not shown).
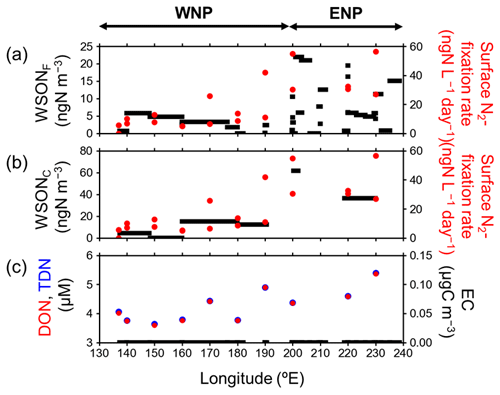
Figure 5Longitudinal distributions of mass concentrations of (a) WSONF (black) and (b) WSONC (black), together with N2-fixation rate in the SSW samples (red solid circle), and (c) DON (red solid circle) and TDN (blue solid circle) concentrations in surface seawater and aerosol EC concentrations (black) during the entire cruise.
The longitudinal distributions of DON clearly showed that DON concentrations in SSW of ENP (4.77 ± 0.53 µM) were larger than those of WNP (4.03 ± 0.47 µM; Fig. 5c). Furthermore, N2-fixation rate and DON concentrations in SSW showed a positive correlation with R2 of 0.63, where DON concentration accounted for >98 % of TDN concentration, which showed the dominance of DON in TDN during the cruise. These results suggest that the majority of DON in SSW was released in association with the recently fixed N2 during the growth of N2-fixing microorganisms.
The WSON concentrations in both fine and coarse particles showed positive correlations with the N2-fixation rates in the SSW samples (Figs. 5 and 6), with R2 values of 0.27 (p<0.05) and 0.60 (p<0.05) in the fine and coarse modes, respectively. Additionally, concentrations of WSON and DON were positively correlated (R2=0.36, p<0.05). Meanwhile, the R2 values for the WSON concentrations and primary productivity in SSW were 0.08 (p=0.24) and 0.58 (p<0.05) in the fine and coarse modes, respectively (Figs. S2 and S3), which were lower than those for WSON and the N2-fixation rate. The positive relation between the WSON mass concentrations and N2-fixation rate in SSW suggests that reactive nitrogen produced by N2-fixing microorganisms in SSW significantly contributed to the formation of WSON aerosols.
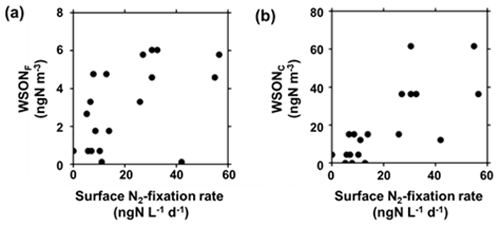
Figure 6Mass concentrations of (a) WSONF and (b) WSONC as a function of N2-fixation rate in SSW. The data of N2-fixation rate in SSW were merged into the duration of each corresponding aerosol sampling. For each one aerosol sample, one or more corresponding measurement data of N2-fixation rate in SSW were obtained, so that the number of the data points in the panels is more than that of the aerosol samples.
Previous laboratory experiments also showed that DON and NH are released in seawater through N2 fixation by microorganisms known as diazotrophs (Wannicke et al., 2009; Berthelot et al., 2015). Here, we discuss mass-based N:C ratios in seawater and atmospheric aerosols. The average WSTN:WSOC ratio in all the size ranges of the observed aerosols was 1.70 ± 0.94, while those in ENP and WNP were 1.61 ± 0.99 and 1.87 ± 0.82, respectively. It is noted that there may be bias in the WSTN:WSOC ratios, particularly in WNP, because the WSOC concentrations were below the detection limit in many samples (Table S1). Yvon-Durocher et al. (2015) reported that the N:C ratios of marine algal assemblages over the subtropical Pacific ranged between 0.10 and 0.13, while Wannicke et al. (2009) obtained N:C ratios of Trichodesmium (0.21 ± 0.02) through a laboratory experiment. Furthermore, Berthelot et al. (2015) reported that the ratio of dissolved nitrogen (DN) to DOC released during N2 fixation was 0.07 ± 0.48. The currently observed WSTN:WSOC ratio in the aerosols was much higher than the N:C ratios of microbes and the DN:DOC ratios in seawater affected by N2 fixation. The higher WSTN:WSOC ratios in aerosols relative to the typical DN:DOC ratios in seawater suggest that nitrogen-containing aerosols are preferentially produced relative to organic carbon in the atmosphere.
It is possible to argue that anthropogenic sources might contribute to the observed WSTN, including WSON and NH, as well as WSTN:WSOC ratios in the aerosols shown above. Concentrations of EC as an anthropogenic tracer were below the lower detection limit (∼ 0.1 µgC m−3) in all the aerosol samples, where they did not show any statistically significant differences between WNP and ENP (Fig. 5). This result suggests that the effects of anthropogenic sources on the observed aerosols in ENP were likely small. This is consistent with the stable carbon isotope analysis, which suggested that most of the observed aerosols were of marine origin rather than terrestrial sources, including anthropogenic origin. Although measurement data of gas species were not available in this study, it is unlikely that only gas-phase precursors were of anthropogenic origin, explaining the secondary formation of WSON in spite of the fact that most of the observed aerosols originated from the ocean surface.
Previous studies showed that oceanic regions at low latitudes, including the subtropics, act as a source of NH3 (the net flux of NH3 is out of the ocean to the atmosphere; Jickells et al., 2003; Johnson et al., 2008). Paulot et al. (2015) used two global ocean biogeochemical models to show evidence for a missing source of atmospheric ammonia (NH3 and NH) over the equatorial Pacific that was attributable to photolysis of marine ON at the ocean surface or in the atmosphere. In fact, DON was the dominant component of TDN (>98 %) in surface seawater, the longitudinal distribution of which was similar to that of WSON in our study (Fig. 5). Furthermore, the observed NH concentration levels in our study (avg 40–50 ng m−3) agree well with those predicted by Paulot et al. (2015) in the same oceanic region. Indeed, NH was the dominant component of the aerosol reactive nitrogen in the fine particles (Fig. 2c) in this study, whereas the correlations between NH concentrations and N2-fixation rates were insignificant (R2=0.06). This insignificant correlation is partially attributable to phase partitioning of ammonia into the gas phase in the subtropical region. A possible source of NO is not necessarily anthropogenic in the equatorial Pacific, while the contribution of an oceanic source in the open ocean cannot be ruled out. Several recent field studies suggested oceanic sources of aerosol NO in the eastern equatorial Pacific, with evidence of extremely low values of δ15N of NO (e.g., lower than −5 ‰; Kamezaki et al., 2019; Carter et al. 2021). In this oceanic region, alkyl nitrates are suggested to contribute to nitrate production, where relatively high concentrations of alkyl nitrates have been observed over the equatorial Pacific (e.g., Blake et al., 2003). The low concentrations of EC and stable carbon isotope ratios in aerosols, together with the higher concentrations of DON in ENP, partly supported the oceanic source of aerosol NO in this oceanic region, although δ15N of NO was not available in this study.
The secondary formation processes of WSON include emissions of gas-phase ON from the ocean and/or marine VOCs reacting with NH3 (Paulot et al., 2015; Altieri et al., 2021). Specifically, aliphatic amines by gas-to-particle conversion are one candidate for WSON species observed in this study, as those amines of marine origin were observed in gas and aerosol phases in tropical and/or subtropical open oceans (e.g., Miyazaki et al., 2010; van Pinxteren et al., 2019). Although the exact mechanism of the WSON formation is not apparent in this study, the current results of the shipboard measurements suggest that N2 fixation in SSW could partly explain one of the missing sources of atmospheric WSON and ammonia indicated by previous modeling studies.
To summarize, the current result suggests that N2-fixing microorganisms in the SSW likely contributed to the formation of aerosol WSON and possibly to other reactive nitrogen species, such as NH3 NH, in the oceanic region of this study. Further field studies are required to elucidate the effect of N2 fixation in surface seawater on the emission of atmospheric reactive nitrogen in different oceanic regions. This includes, for example, simultaneous measurements of gas and particle phases of organic and inorganic nitrogen species together with measurements of biological and chemical parameters relevant to N2-fixing microorganisms in surface seawater by shipboard observations. Additional laboratory studies are needed to evaluate the factors controlling the atmospheric emissions of reactive nitrogen associated with N2 fixation in surface sea water. Jiang et al. (2018) predicted that global ocean warming in the future would result in large increases in growth and N2 fixation by Trichodesmium. Consequently, the formation process and the amount of atmospheric WSON associated with N2 fixation in surface seawater are expected to change, which should be important from the viewpoints of the climate effect of marine atmospheric aerosols and of the air–sea exchange of nitrogen.
This study investigated the origin and formation process of WSON aerosols in the oligotrophic subtropical North Pacific in terms of their linkage with N2-fixing microorganisms in SSW based on the cruise measurements. The average concentration of WSON in fine-mode aerosols along 23∘ N in the eastern North Pacific (7.5 ± 6.6 ngN m−3) was much higher than that in the western North Pacific (2.4 ± 1.9 ngN m−3) during the research cruise. The stable carbon isotope ratio of WSOC together with backward trajectories indicated that most of the observed WSON in the fine particles in the eastern North Pacific originated from the ocean surface. Relations of the concentrations of WSON with those of Na+ and MSA imply that secondary formation, which differed from the oxidation processes of DMS, was likely the dominant process underlying the formation of WSON observed in this study. Instead, significant positive correlations were found among nitrogen-fixation rate, DON concentrations in SSW, and the aerosol WSON concentrations. Meanwhile, the EC concentrations in all the samples were below the lower detection limit and did not show any statistically significant differences between WNP and ENP, suggesting that the effects of anthropogenic sources on the observed aerosols were likely small in this study. The overall results suggest that reactive nitrogen, such as dissolved organic nitrogen and ammonium, produced and exuded by nitrogen-fixing microorganisms in SSW, likely contributed to the formation of WSON aerosols over the oceanic region. This study provides new implications for the role of marine microbial activity in the formation of reactive nitrogen aerosols in the ocean surface.
All data used in this study are provided in the Supplement.
The supplement related to this article is available online at: https://doi.org/10.5194/bg-20-439-2023-supplement.
YM designed and led the overall research. TD and YM wrote the paper. TD, ET, and YM performed the measurements of chemical parameters in the aerosol samples. KT, SH, YI, and KH collected the samples. SKW and KH measured chlorophyll a concentrations. SH and KT measured nitrogen-fixation rate and δ15N of POM. FH measured nanomolar DIN, and SYT measured TDN in seawater. TD, YM, and ET analyzed the data.
The contact author has declared that none of the authors has any competing interests.
Publisher’s note: Copernicus Publications remains neutral with regard to jurisdictional claims in published maps and institutional affiliations.
We thank the researchers and crew of the R/V Hakuho Maru for their help with the observations. Ryohei Yamaguchi is acknowledged for his help with the aerosol sampling. This study was also supported by the Grant for Joint Research Program of the Institute of Low Temperature Science, Hokkaido University.
This research has been supported by the Ministry of Education, Culture, Sports, Science and Technology (grant nos. 16H02931, 19H04233, and 24121005).
This paper was edited by Steven Bouillon and reviewed by Darius Ceburnis and one anonymous referee.
Altieri, K. E., Fawcett, S. E., Peters, A. J., Sigman, D. M., and Hastings, M. G.: Marine biogenic source of atmospheric organic nitrogen in the subtropical North Atlantic, P. Natl. Acad. Sci. USA, 113, 925–930, https://doi.org/10.1073/pnas.1516847113, 2016.
Altieri, K. E., Fawcett, S. E., and Hastings, M. G.: Reactive Nitrogen Cycling in the Atmosphere and Ocean, Annu. Rev. Earth Planet. Sci., 49, 523–550, https://doi.org/10.1146/annurev-earth-083120-052147, 2021.
Berthelot, H., Bonnet, S., Camps, M., Grosso, O., and Moutin, T.: Assessment of the dinitrogen released as ammonium and dissolved organic nitrogen by unicellular and filamentous marine diazotrophic cyanobacteria grown in culture, Front. Mar. Sci., 2, 80, https://doi.org/10.3389/fmars.2015.00080, 2015.
Blake, N. J., Blake, D. R., Swanson, A. L., Atlas, E., Flocke, F., and Rowland, F. S.: Latitudinal, vertical, and seasonal variations of C1-C4 alkyl nitrates in the troposphere over the Pacific Ocean during PEM-Tropics A and B: Oceanic and continental sources, J. Geophys. Res., 108, 8242, https://doi.org/10.1029/2001JD001444, 2003.
Bonnet, S., Biegala, I. C., Dutrieux, P., Slemons, L. O., and Capone, D. G.: Nitrogen fixation in the western equatorial Pacific: rates, diazotrophic cyanobacterial size class distribution, and biogeochemical significance, Global Biogeochem. Cy., 23, GB3012, https://doi.org/10.1029/2008GB003439, 2009.
Brüggemann, M., Hayeck, N., and George, C.: Interfacial photochemistry at the ocean surface is a global source of organic vapors and aerosols, Nat. Commun., 9, 1–8, https://doi.org/10.1038/s41467-018-04528-7, 2018.
Cachier, H., Buat-Ménard, M. P., Fontugne, M., and Chesselet, R.: Long-range transport of continentally derived particulate carbon in the marine atmosphere: evidence from stable isotope studies, Tellus, 38, 161–177, https://doi.org/10.1111/j.1600-0889.1986.tb00184.x, 1986.
Capone, D. G., Ferrier, M. D., and Carpenter, E. J.: Cycling and release of glutamate and glutamine in colonies of the marine planktonic cyanobacterium, Trichodesmium thiebautii, Appl. Environ. Microbiol., 60, 3989–3995, 1994.
Carter, T. S., Joyce, E. E., and Hastings, M. G.: Quantifying Nitrate Formation Pathways in the Equatorial Pacific Atmosphere from the GEOTRACES Peru-Tahiti Transect, ACS Earth Space Chem., 5, 2638–2651, 2021.
Cheung, S., Nitanai, R., Tsurumoto, C., Endo, H., Nakaoka, S., Cheah, W., Lorda, J. F., Xia, X., Liu, H., and Suzuki, K.: Physical forcing controls the basin-scale occurrence of nitrogen-fixing organisms in the North Pacific Ocean, Global Biogeochem. Cy., 34, e2019GB006452, https://doi.org/10.1029/2019GB006452, 2020.
Dasari, S., Andersson, A., Bikkina, S., Holmstrand, H., Budhavant, K., Satheesh, S., Asmi, E., Kesti, J., Backman, J., Salam, A., Bisht, D. S., Tiwari, S., Hameed, Z., and Gustafsson, Ö.: Photochemical degradation affects the light absorption of water-soluble brown carbon in the South Asian outflow, Sci. Adv., 5, eaau8066, https://doi.org/10.1126/sciadv.aau8066, 2019.
Duce, R. A., LaRoche, J., Altieri, K., Arrigo, K. R., Baker, A. R., Capone, D. G., Cornell, S., Dentener, F., Galloway, J., Ganeshram, R. S., Geider, R. J., Jickells, T., Kuypers, M. M., Langlois, R., Liss, P. S., Liu, S. M., Middelburg, J. J., Moore, C. M., Nickovic, S., Oschlies, A., Pedersen, T., Prospero, J., Schlitzer, R., Seitzinger, S., Sorensen, L. L., Uematsu, M., Ulloa, O., Voss, M., Ward, B., and Zamora, L.: Impacts of atmospheric anthropogenic nitrogen on the open ocean, Science, 320, 893–897, https://doi.org/10.1126/science.1150369, 2008.
Dutheil, C., Aumont, O., Gorguès, T., Lorrain, A., Bonnet, S., Rodier, M., Dupouy, C., Shiozaki, T., and Menkes, C.: Modelling N2 fixation related to Trichodesmium sp.: driving processes and impacts on primary production in the tropical Pacific Ocean, Biogeosciences, 15, 4333–4352, https://doi.org/10.5194/bg-15-4333-2018, 2018.
Facchini, M. C., Decesari, S., Rinaldi, M., Carbone, C., Finessi, E., Mircea, M., Fuzzi, S., Moretti, F., Tagliavini, E., Ceburnis, D., and O'Dowd, C. D.: Important Source of Marine Secondary Organic Aerosol from Biogenic Amines, Environ. Sci. Technol., 42, 9116–9121, https://doi.org/10.1021/es8018385, 2008.
Frischkorn, K. R., Haley, S. T., and Dyhrman, S. T.: Coordinated gene expression between Trichodesmium and its microbiome over day–night cycles in the North Pacific Subtropical Gyre, ISME Journal, 12, 997–1007, https://doi.org/10.1038/s41396-017-0041-5, 2018.
Glibert, P. M. and Bronk, D. A.: Release of dissolved organicnitrogen by marine diazotrophic cyanobacteria, Trichodesmium spp., Appl. Environ. Microbiol., 60, 3996–4000, 1994.
Hama, T., Miyazaki, T., Ogawa, Y., Iwakuma, T., Takahashi, M., Otsuki, A., and Ichimura, S.: Measurement of photosynthetic production of a marine phytoplankton population using a stable 13C isotope, Mar. Biol., 73, 31–36, https://doi.org/10.1007/BF00396282, 1983.
Hashihama, F., Furuya, K., Kitajima, S., Takeda, S., Takemura, T., and Kanda, J.: Macro-scale exhaustion of surface phosphate by dinitrogen fixation in the western North Pacific, Geophys. Res. Lett., 36, L03610, https://doi.org/10.1029/2008GL036866, 2009.
Hashihama, F., Kanda, J., Tauchi, A., Kodama, T., Saito, H., and Furuya, K.: Liquid waveguide spectrophotometric measurement of nanomolar ammonium in seawater based on the indophenol reaction with o-phenylphenol (OPP), Talanta, 143, 374–380, 2015.
Hashihama, F., Saito, H., Shiozaki, T., Ehama, M., Suwa, S., Sugiyama, T., Kato, H., Kanda, J., Sato, M., Kodama, T., Yamaguchi, T., Horii, S., Tanita., I, Takino, S., Takahashi, K., and Ogawa, H.: Biogeochemical controls of particulate phosphorus distribution across the oligotrophic subtropical Pacific ocean, Global Biogeochem. Cy., 34, e2020GB006669, https://doi.org/10.1029/2020GB006669, 2020.
Horii, S., Takahashi, K., Shiozaki, T., Hashihama, F., and Furuya, K.: Stable isotopic evidence for differential contribution of diazotrophs to the epipelagic grazing food chain in the mid-Pacific Ocean, Global Ecol. Biogeogr., 27, 1467–1480, https://doi.org/10.1111/geb.12823, 2018.
Jiang, H. B., Fu, F. X., Rivero-Calle, S., Levine, N. M., Sañudo-Wilhelmy, S. A., Qu, P. P., Wang, X. W., Pinedo-Gonzalez, P., Zhu, Z., and Hutchins, D. A.: Ocean warming alleviates iron limitation of marine nitrogen fixation, Nature Climate Change, 8, 709–712, https://doi.org/10.1038/s41558-018-0216-8, 2018.
Jickells, T. D., Kelly, S. D., Baker, A. R., Biswas, K., Dennis, P. F., Spokes, L. J., Witt, M., and Yeatman, S. G.: Isotopic evidence for a marine ammonia source, Geophys. Res. Lett., 30, 1374, https://doi.org/10.1029/2002GL016728, 2003.
Johnson, M. T., Liss, P. S., Bell, T. G., Lesworth, T. J., Baker, A. R., Hind, A. J., Jickells, T. D., Biswas, K. F., Woodward, E. M. S., and Gibb, S. W.: Field observations of the ocean-atmosphere exchange of ammonia: Fundamental importance of temperature as revealed by a comparison of high and low latitudes, Global Biogeochem. Cy., 22, GB1019, https://doi.org/10.1029/2007GB003039, 2008.
Kamezaki, K., Hattori, S., Iwamoto, Y., Ishino, S., Furutani, H., Miki, Y., Uematsu, M., Miura, K., and Yoshida, N.: Tracing the sources and formation pathways of atmospheric particulate nitrate over the Pacific Ocean using stable isotopes, Atmos. Environ., 209, 152–166, https://doi.org/10.1016/J.ATMOSENV.2019.04.026, 2019.
Kanakidou, M., Duce, R. A., Prospero, J. M., Baker, A. R., Benitez-Nelson, C., Dentener, F. J., Hunter, K. A., Liss, P. S., Mahowald, N., Okin, G. S., Sarin, M., Tsigaridis, K., Uematsu, M., Zamora, L. M., and Zhu, T.: Atmospheric fluxes of organic N and P to the global ocean, Global Biogeochem. Cy., 26, GB3026, https://doi.org/10.1029/2011GB004277, 2012.
Karl, D. M., Letelier, R., Hebel, D. V., Bird, D. F., and Winn, C. D.: Trichodesmium blooms and new nitrogen in the north Pacific gyre, Marine pelagic cyanobacteria: Trichodesmium and other diazotrophs, Kluwer Academic Publishers, Dordrecht, The Netherlands, 219–237, https://doi.org/10.1007/978-94-015-7977-3_14, 1992.
Letelier, R. M. and Karl, D. M: The role of Trichodesmium spp. In the productivity of the subtropical North Pacific Ocean, Mar. Ecol. Prog. Ser., 133, 263–273, 1994.
Luo, Y.-W., Lima, I. D., Karl, D. M., Deutsch, C. A., and Doney, S. C.: Data-based assessment of environmental controls on global marine nitrogen fixation, Biogeosciences, 11, 691–708, https://doi.org/10.5194/bg-11-691-2014, 2014.
Miyazaki, Y., Kawamura, K., Jung, J., Furutani, H., and Uematsu, M.: Latitudinal distributions of organic nitrogen and organic carbon in marine aerosols over the western North Pacific, Atmos. Chem. Phys., 11, 3037–3049, https://doi.org/10.5194/acp-11-3037-2011, 2011.
Miyazaki, Y., Fu, P. Q., Kawamura, K., Mizoguchi, Y., and Yamanoi, K.: Seasonal variations of stable carbon isotopic composition and biogenic tracer compounds of water-soluble organic aerosols in a deciduous forest, Atmos. Chem. Phys., 12, 1367–1376, https://doi.org/10.5194/acp-12-1367-2012, 2012.
Miyazaki, Y., Coburn, S., Ono, K., Ho, D. T., Pierce, R. B., Kawamura, K., and Volkamer, R.: Contribution of dissolved organic matter to submicron water-soluble organic aerosols in the marine boundary layer over the eastern equatorial Pacific, Atmos. Chem. Phys., 16, 7695–7707, https://doi.org/10.5194/acp-16-7695-2016, 2016.
Miyazaki, Y., Yamashita, Y., Kawana, K., Tachibana, E., Kagami,S., Mochida, M., Suzuki, K., and Nishioka, J.: Chemical transfer of dissolved organic matter from surface seawater to sea spray water-soluble organic aerosol in the marine atmosphere, Sci. Rep., 8, 14861, https://doi.org/10.1038/s41598-018-32864-7, 2018.
Mohr, W., Großkopf, T., Wallace, D. W. R., and Laroche, J.: Methodological underestimation of oceanic nitrogen fixation rates, PLoS ONE, 5, e12583, https://doi.org/10.1371/journal.pone.0012583, 2010.
Mohr, C., Lopez-Hilfiker, F. D., Zotter, P., Prévôt, A. S. H., Xu, L., Ng, N. L., Herndon, S. C., Williams, L. R., Franklin J. P., Zahniser, M. S., Worsnop, D. R., Knighton, W. B., Aiken, A. C., Gorkowski, K. J., Dubey M. K., Allan J. D., and Thornton, J. A.: Contribution of nitrated phenols to wood burning brown carbon light absorption in Detling, United Kingdom during wintertime, Environ. Sci. Technol., 47, 6316–6324, https://doi.org/10.1021/es400683v, 2013.
Montoya, J. P., Voss, M., Kaehler, P., and Capone, D. G.: A simple, high precision, high sensitivity tracer assay for dinitrogen fixation, Appl. Environ. Microbiol., 62, 986–993, https://doi.org/10.1128/AEM.62.3.986-993.1996, 1996.
Montoya, J. P., Holl, C. M., Zehr, J. P., Hansen, A., Villareal, T. A., and Capone, D. G.: High rates of N2 fixation by unicellular diazotrophs in the oligotrophic Pacific Ocean, Nature, 430, 1027–1032, https://doi.org/10.1038/nature02824, 2004.
Mungall, E. L., Wong, J. P. S., and Abbatt, P. D.: Heterogeneous oxidation of particulate methanesulfonic acid by the hydroxyl radical: kinetics and atmospheric implications, ACS Earth Space Chem., 2, 48–55, 2018.
Nehir, M. and Koçak, M.: Atmospheric water-soluble organic nitrogen (WSON) in the eastern Mediterranean: origin and ramifications regarding marine productivity, Atmos. Chem. Phys., 18, 3603–3618, https://doi.org/10.5194/acp-18-3603-2018, 2018.
Oreopoulos, L. and Platnick, S.: Radiative susceptibility of cloudy atmospheres to droplet number perturbations. II. Global analysis from MODIS, J. Geophys. Res., 113, D14S21, https://doi.org/10.1029/2007JD009655, 2008.
Ottesen, E. A., Young, C. R., Gifford, S. M., Eppley, J. M., Marin, R. 3rd, Schuster, S. C., Scholin, C. A., and DeLong, E. F.: Ocean microbes. Multispecies diel transcriptional oscillations in open ocean heterotrophic bacterial assemblages, Science, 345, 207–212, https://doi.org/10.1126/science.1252476, 2014.
Paulot, F., Jacob, D. J., Johnson, M. T., Bell, T. G., Baker, A. R., Keene, W. C., Lima, I. D., Doney, S. C., and Stock, C. A.: Global oceanic emission of ammonia: Constraints from seawater and atmospheric observations, Global Biogeochem. Cy., 29, 1165–1178, https://doi.org/10.1002/2015GB005106, 2015.
Rosenfeld, D., Zhu, Y. N., Wang, M. H., Zheng, Y. T., Goren, T., and Yu, S. C.: Aerosol-driven droplet concentrations dominate coverage and water of oceanic low level clouds, Science, 363, eaav0566, https://doi.org/10.1126/science.aav0566, 2019.
Rousseaux, C. S. and Gregg, W. W.: Climate variability and phytoplankton composition in the Pacific Ocean, J. Geophys. Res., 117, C10006, https://doi.org/10.1029/2012JC008083, 2012.
Salter, S., Sortino, G., and Latham, J.: Sea-going hardware for the cloud albedo method of reversing global warming, Philos. T. Roy. Soc. A, 366, 3989–4006, https://doi.org/10.1098/rsta.2008.0136, 2008.
Shiozaki, T., Bombar, D., Riemann, L., Hashihama, F., Takeda, S., Yamaguchi, T., Ehoma, M., Hamasaki, K., and Furuya, K.: Basin scale variablity of active diazotrophs and nitrogen fixation in the North Pacific, from the tropics to the subarctic Bering Sea, Global Biogeochem. Cy., 31, 996–1009, https://doi.org/10.1002/2017GB005681, 2017.
Shiozaki, T., Bombar, D., Riemann, L., Sato, M., Hashihama, F., Kodama, T., Tanita, I., Takeda, S., Saito, H., Hamasaki, K., and Furuya, K.: Linkage between dinitrogen fixation and primary production in the oligotrophic South Pacific Ocean, Global Biogeochem. Cy., 32, 1028–1044, https://doi.org/10.1029/2017GB005869, 2018.
Suzuki, R. and Ishimaru, T.: An improved method for the determination of phytoplankton chlorophyll using N, N-dimethylformamide, J. Oceanog., 46, 190–194, 1990.
van Pinxteren, M., Barthel, S., Fomba, K. W., Müller, K., von Tümpling, W., and Herrmann, H.: The influence of environmental drivers on the enrichment of organic carbon in the sea surface microlayer and in submicron aerosol particles-Measurements from the Atlantic Ocean, Elementa Science of the Anthropocene, 5, 35, https://doi.org/10.1525/elementa.225, 2017.
Wang, W. L., Moore, J. K., Martiny, A. C., and Primeau, F. W.: Convergent estimates of marine nitrogen fixation, Nature, 566, 205–211, https://doi.org/10.1038/s41586-019-0911-2, 2019.
Wannicke, N., Koch, B. P., and Voss, M.: Release of fixed N2 and C as dissolved compounds by Trichodesmium erythreum and Nodularia spumigena under the influence of high light and high nutrient (P), Aquat. Microb. Ecol., 57, 175–189, https://doi.org/10.3354/ame01343, 2009.
Yamaguchi, T., Sato, M., Hashihama, F., Ehama, M., Shiozaki, T., Takahashi, K., and Furuya, K.: Basin-scale variations in labile dissolved phosphoric monoesters and diesters in the central North Pacific Ocean, J. Geophys. Res.-Oceans, 124, 3058–3072, https://doi.org/10.1029/2018jc014763, 2019.
Yamaguchi, T., Sato, M., Hashihama, F., Kato, H., Sugiyama, T., Ogawa, H., Takahashi, K., and Furuya, K.: Longitudinal and vertical variations of dissolved labile phosphoric monoesters and diesters in the subtropical North Pacific, Front. Microbiol., 11, 570081, https://doi.org/10.3389/fmicb.2020.570081, 2021.
Yasui-Tamura, S., Hashihama, F., Ogawa, H., Nishimura, T., and Kanda, J.: Automated simultaneous determination of total dissolved nitrogen and phosphorus in seawater by persulfate oxidation method, Talanta Open, 2, 100016, https://doi.org/10.1016/j.talo.2020.100016, 2020.
Yvon-Durocher, G., Dossena, M., Trimmer, M., Woodward, G., and Allen, A. P.: Temperature and the biogeography of algal stoichiometry, Global Ecology and Biogeography, 24, 562–570, https://doi.org/10.1111/geb.12280, 2015.
Zehr, J. P. and Capone, D. G.: Changing perspectives on nitrogen fixation, Science, 368, eaay9514, https://doi.org/10.1126/science.aay9514, 2020.
Zhu, L., Nenes, A., Wine, P. H., and Nicovich, J. M.: Effects of aqueous organosulfur chemistry on particulate methanesulfonate to nonsea salt sulfate ratios in the marine atmosphere, J. Geophys. Res., 111, D05316, https://doi.org/10.1029/2005JD006326, 2006.
- Abstract
- Introduction
- Experimental
- Longitudinal distributions of WSON
- Isotopic characterization of aerosol organic carbon and formation processes of WSON
- Distributions of nitrogen-fixation rate, dissolved organic nitrogen, and aerosol WSON
- Discussion on nitrogen fixation as a possible source of WSON in marine aerosols
- Conclusions
- Data availability
- Author contributions
- Competing interests
- Disclaimer
- Acknowledgements
- Financial support
- Review statement
- References
- Supplement
- Abstract
- Introduction
- Experimental
- Longitudinal distributions of WSON
- Isotopic characterization of aerosol organic carbon and formation processes of WSON
- Distributions of nitrogen-fixation rate, dissolved organic nitrogen, and aerosol WSON
- Discussion on nitrogen fixation as a possible source of WSON in marine aerosols
- Conclusions
- Data availability
- Author contributions
- Competing interests
- Disclaimer
- Acknowledgements
- Financial support
- Review statement
- References
- Supplement