the Creative Commons Attribution 4.0 License.
the Creative Commons Attribution 4.0 License.
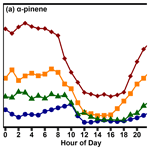
Minor contributions of daytime monoterpenes are major contributors to atmospheric reactivity
Deborah F. McGlynn
Graham Frazier
Laura E. R. Barry
Manuel T. Lerdau
Sally E. Pusede
Emissions from natural sources are driven by various external stimuli such as sunlight, temperature, and soil moisture. Once biogenic volatile organic compounds (BVOCs) are emitted into the atmosphere, they rapidly react with atmospheric oxidants, which has significant impacts on ozone and aerosol budgets. However, diurnal, seasonal, and interannual variability in these species are poorly captured in emissions models due to a lack of long-term, chemically speciated measurements. Therefore, increasing the monitoring of these emissions will improve the modeling of ozone and secondary organic aerosol concentrations. Using 2 years of speciated hourly BVOC data collected at the Virginia Forest Research Lab (VFRL) in Fluvanna County, Virginia, USA, we examine how minor changes in the composition of monoterpenes between seasons are found to have profound impacts on ozone and OH reactivity. The concentrations of a range of BVOCs in the summer were found to have two different diurnal profiles, which, we demonstrate, appear to be driven by light-dependent versus light-independent emissions. Factor analysis was used to separate the two observed diurnal profiles and determine the contribution from each emission type. Highly reactive BVOCs were found to have a large influence on atmospheric reactivity in the summer, particularly during the daytime. These findings reveal the need to monitor species with high atmospheric reactivity, even though they have low concentrations, to more accurately capture their emission trends in models.
- Article
(2265 KB) - Full-text XML
-
Supplement
(1728 KB) - BibTeX
- EndNote
Biogenic volatile organic compounds (BVOCs) are important chemical sinks for atmospheric oxidants and precursors for secondary organic aerosol (SOA) and ozone formation (Atkinson and Arey, 2003a; Guenther et al., 1995, 2000; Kroll and Seinfeld, 2008). Their emissions are primarily driven by the species of plants present and by changes in temperature and light, with secondary effects from other factors such as meteorology and deposition. Light-dependent or de novo biosynthesis emissions are produced within the leaves of plants and emitted shortly after formation through plant stomata (Niinemets and Monson, 2013). These emissions tend to increase with temperature (Guenther et al., 2006; Guenther, 1997) but are also linked to photosynthesis and therefore require photosynthetically active radiation (PAR). The dominant de novo BVOC emitted is isoprene, though some monoterpenes can be emitted in this manner (Staudt and Seufert, 1995; Tingey et al., 1979; Ghirardo et al., 2010; Taipale et al., 2011). In contrast, other emissions occur independently of light, are driven by temperature from a wide variety of vegetation, and therefore occur year-round (Niinemets and Monson, 2013; Ghirardo et al., 2010; Guenther et al., 1991). Monoterpenes, sesquiterpenes, and diterpenes are largely emitted in a temperature-dependent manner through volatilization from storage pools or resin ducts from within the plant (Zimmerman, 1979; Lerdau et al., 1997; Lerdau and Gray, 2003). The rate of volatilization is determined by the compound's vapor pressure.
The diurnal concentration profile of individual species (i.e., the observed average variability within a 24 h period) is a function of the drivers of emissions, the concentrations of atmospheric oxidants, and meteorology. For isoprene, which is emitted from plants in a light-dependent manner, the diurnal profile is well established and relatively consistent across environments (Rinne et al., 2002; Guenther et al., 2000; Delwiche and Sharkey, 1993; Niinemets and Monson, 2013; Guenther et al., 1991; Bouvier-Brown et al., 2009). Due to strong daytime emissions, concentrations peak around midday to late afternoon, when incoming solar radiation and temperatures are greatest. Nighttime emissions of de novo emitted BVOCs drop to near zero due to the lack of photosynthetically active radiation (Niinemets and Monson, 2013; Ghirardo et al., 2010; Panopoulou et al., 2020; Guenther et al., 1996; Rinne et al., 2002). Concentrations of de novo emitted species concomitantly drop as suspended gases are depleted by atmospheric oxidation, deposited to surfaces, and diluted through dispersion.
The diurnal variation in monoterpenes is substantially more variable and complex. Because their emissions are predominantly temperature dependent, emissions peak in the afternoon but continue throughout the night. Consequently, monoterpene concentrations are often greatest during the nighttime hours, when oxidation by photochemically formed hydroxyl radicals is minimal and boundary height is reduced, decreasing dilution through atmospheric mixing (Bouvier-Brown et al., 2009; Haapanala et al., 2007; Panopoulou et al., 2020; Hakola et al., 2012). However, some plants do produce and emit monoterpenes in a light-dependent manner (Staudt et al., 1999; Staudt and Seufert, 1995; Harley et al., 2014; Yu et al., 2017; Taipale et al., 2011; Guenther et al., 2012). Despite these findings, light-dependent monoterpene emissions have largely been deemed to contribute minimally to total monoterpene emissions. (Bouvier-Brown et al., 2009; Lerdau and Gray, 2003). Some studies suggest that this lack of contribution to total flux occurs because they are emitted from only a handful of plant taxa, and the emission rates themselves have not been shown to be significant (Staudt et al., 1999; Loreto et al., 1998; Staudt and Seufert, 1995). However, a few studies find that many trees emit low levels of monoterpenes in a light-dependent manner and that these emissions are seasonal and change with phenological patterns (Fischbach et al., 2002; Ghirardo et al., 2010; Taipale et al., 2011; Steinbrecher et al., 1999). Overall, understanding of the scale and seasonality of de novo monoterpene emissions is limited and highly variable in the literature. A major goal of the present work is to understand the potential role that the minor contribution of light-dependent emissions and/or individual compounds with differing temporal variability may play in the atmosphere. Certain monoterpenes that are often emitted at low levels and/or in a light-dependent manner have extremely high reactivities, raising the question of whether or not chemical impact may be disproportionate to flux magnitude.
A lack of understanding of how individual compounds are emitted from vegetative sources makes emission modeling difficult and more uncertain. This is largely due to the impact the structure of a BVOC has on its aerosol formation potential and its reaction rates with atmospheric oxidants, particularly for reactions involving ozone. For example, endocyclic monoterpenes (e.g., limonene and 3-carene) and sesquiterpenes (e.g., α-humulene and β-caryophyllene) have a greater aerosol formation potential and tend to react faster than compounds with exocyclic double bonds (e.g., α-pinene and α-cedrene). Consequently, long-term measurements of speciated BVOCs can assist in modeling BVOC emissions and in understanding their contribution to ozone modulation and SOA formation (Porter et al., 2017). These impacts extend further to the importance of individual fast-reacting isomers, which can represent substantial fractions of total reactivity even at low concentrations (Yee et al., 2018). In this context, a detailed understanding of the different drivers of isomer emissions and the temporal variability in composition is critical for interpreting such data.
Using 2 years of chemically resolved concentration measurements of in-canopy, biogenic volatile organic compound (BVOC) concentration data, we examine the contribution of individual monoterpene compounds to ozone and OH reactivity on diurnal, seasonal, and interannual timescales. We elucidate the impact of temporal variability on ozone and OH reactivity on scales from hours to years by identifying two varying components in the data, which we identify as arising from light-dependent and light-independent emissions and quantifying their chemical impacts on each timescale. Factor analysis is used to quantitatively separate these observed profiles and their contributions to total monoterpene concentration and ozone and OH reactivity. Our findings highlight the need to better understand the drivers of emissions with isomer-level chemical resolution and improve their representation in emissions models, as they have significant atmospheric impact.
2.1 Data collection and preparation
We measured in-canopy BVOC concentrations at the Virginia Forest Research Lab (VFRL) 37.9229∘ N, 78.2739∘ W) in Fluvanna County, Virginia. The VFRL sits on the eastern side of the Blue Ridge Mountains and is about 25 km east–southeast of Charlottesville, VA, USA. The forest is largely composed of oak, maple, and pine trees; oak predominantly emits isoprene, while pine is a major source of monoterpenes and sesquiterpenes. Additional information pertaining to the measurement site can be found in McGlynn et al. (2021), and information on the forest can be found in Chan (2011). The site houses a 40 m meteorological tower, with a climate-controlled, internet-connected lab at the bottom that is supplied by line power. The BVOC concentrations were measured using a gas chromatograph with flame ionization detection (GC-FID) adapted for automated collection and analysis of air samples from the mid-canopy (∼ 20 m) of the VFRL. In brief, air is pulled from the mid-canopy (∼ 20 m above ground level) through an insulated and heated Teflon tube. Ozone is removed from the sample using a sodium-thiosulfate-infused quartz fiber filter (Pollmann et al., 2005) at the front of the inlet. Samples were collected mid-canopy in order to more closely represent the in-canopy environment for co-located studies seeking to understand ozone loss processes. A subsample of air is concentrated onto a multi-bed adsorbent trap, the details of which can be found in McGlynn et al. (2021). A custom LabVIEW program (National Instruments) operates the instrument for hourly automated sample collection and analysis. Following sample collection, the trap is thermally desorbed to transfer the sample to the head of the GC column; details pertaining to the GC run methods, column, and gas flow rates can be found in McGlynn et al. (2021).
The instrument is calibrated using a multicomponent calibrant (Apel Riemer Environmental Inc.) optionally mixed at one of four different flows to generate four different mixing ratios. A calibration sample occurs once every 7 h, rotating between zero-air only, a calibrant at a fixed tracking mixing ratio, and a calibrant at one of three other mixing ratios. Details pertaining to calibrant composition, concentrations, peak integration, and data uncertainty can be found in McGlynn et al. (2021).
To identify analytes in the samples, a mass spectrometer (MS; Agilent 5977) was deployed in October 2019, September 2020, and June 2021 in parallel with the FID. Retention times of analytes detected by the two detectors were aligned using the retention time of known analytes. Analytes were identified by mass spectral matching with the 2011 NIST (National Institute of Standards and Technology) MS Library and reported retention indices (Mass Spectrometry Data Center, NIST, 2022). The chromatographic data were analyzed using the freely available TERN software package by Isaacman-VanWertz et al. (2017) within the Igor Pro 8 programming environment (WaveMetrics, Inc.). The measurement period included in this work extends from 15 September 2019 to 14 September 2021. This work presents all isoprene and monoterpene data collected during the measurement period but focuses largely on the monoterpenes between May and September (2020 primarily shown in the main text, with 2021 provided in the Supplement).
2.2 Positive matrix factorization
Positive matrix factorization (PMF) has been widely used for source apportionment problems (Norris and Duvall, 2014; Ulbrich et al., 2009; Kuang et al., 2015). A large number of variables can be reduced by the PMF algorithm to the main sources or factors that drive the observed variability (Norris and Duvall, 2014). Application of PMF to multi-variable data generates two matrices, the factor contributions, and factor profiles (Norris and Duvall, 2014), which, for environmental data, represent a time series as a set of covarying variables (e.g., chemical species).
This work employed the United States Environmental Protection Agency's (U.S. EPA) PMF 5.0 program to support the identification in the observational data of two apparent sources or drivers of BVOC concentration variability. Specifically, a two-factor PMF solution was examined to better understand and quantify the profiles and temporal variability in each observed factor. The 2 years of monoterpene data were run separately (2020 refers to 15 September 2019–14 September 2020, and 2021 refers to 15 September 2020–14 September 2021), with uncertainty, u, in the data calculated using the equation provided by Norris and Duvall (2014):
The method detection limit, MDL, is 2.2 ppt (parts per thousand) for monoterpenes (McGlynn et al., 2021). Values below the method detection limit were substituted with MDL 2 in both the concentration and uncertainty file. Missing data are excluded from the data processing. The uncertainty value of 0.15 is recommended by Norris and Duvall (2014) as an estimate of the overall uncertainty in the data. It reasonably represents the uncertainty in this instrument as well, based on uncertainties in calibration slopes, and inherent uncertainty in integration of chromatographic peaks, which has been shown to be of the order of 10 %–15 % (Isaacman-VanWertz et al., 2017). Further information on the PMF output can be found in Norris and Duvall (2014).
2.3 Reactivity calculations
Reactivity of an individual BVOC with ozone (O3R) and OH (OHR) is calculated as the sum of the products of the concentration and oxidation reaction rate constant of each BVOCi, as follows:
All rate constants (cm3 molec.−1 s−1) used in this work are listed in Table S1 (Atkinson et al., 2006, 1990a; Pinto et al., 2007; Atkinson and Arey, 2003b; Shu and Atkinson, 1994; Pratt et al., 2012; Atkinson et al., 1990b). A temperature of 298 K is assumed for all rate constants, representing the approximate midpoint between day and night temperatures in the summer at this site, which vary by roughly 10 ∘C (McGlynn et al., 2021). Taking the temperature dependence of rate constants into account would increase daytime OH reactivity by 5 %–8 % and decrease nighttime OH reactivity by approximately the same amount. These differences suggest that the true difference between the light-dependent (daytime) and light-independent (nighttime) mixtures is ∼ 10 %–16 % higher than calculated, but this effect is not included quantitatively because temperature dependence is not known for many monoterpene reaction rates. Additionally, some rate constants such as thujene were calculated from structure activity relationships, and previous work has found that calculated rate constants add significant uncertainty to calculated ozone reactivity (Frazier et al., 2022). However, compounds that contribute the most to atmospheric reactivity, such as α-pinene, limonene, and sabinene have measured rate constants; therefore, we do not expect significant uncertainty in our calculations.
At the VFRL, concentrations of a wide range of species, including anthropogenic and other volatile organic compounds (VOCs), are measured hourly. The BVOCs measured include isoprene, methyl vinyl ketone, methacrolein, 11 monoterpenes, and 2 sesquiterpenes. This work focuses primarily on monoterpenes, which contribute the largest fraction of speciated ozone and OH reactivity from BVOCs at the research site throughout the year (McGlynn et al., 2021). While the measurement method captures two sesquiterpenes, they are not included in the analysis because these and related measurements have found they do not contribute significantly to most oxidant reactivity (Frazier et al., 2022; McGlynn et al., 2021).
3.1 Monoterpene seasonality
To understand the drivers of monoterpene variability, we first examine diurnal and seasonal patterns in two monoterpenes found at the site, α-pinene and limonene, that exhibit the features of two different concentration profiles. Seasonal averages are defined as December, January, and February (winter), March, April, and May (spring), June, July, and August (summer), and September, October, November (fall). Diurnal trends in these species demonstrate some clear differences in their concentration patterns (Fig. 1). α-pinene concentrations were lowest in the daytime winter hours at about 0.05 ppb (parts per billion) and highest in the nighttime summer hours, at 0.60 ppb. In all seasons, α-pinene concentrations were highest at night and decreased in the morning hours, following typical patterns of light-independent emitted monoterpene concentrations which are largely modulated by variability in the planetary boundary layer (Bouvier-Brown et al., 2009). Concentrations were lowest in the middle of the day, between 10:00 and 17:00 LT (local time; note that all times are LT unless otherwise indicated) and highest between 20:00 and 08:00 (Fig. 1a). Concentration transitions between these periods vary somewhat by season, which is in accordance with the changing temperature and daylight hours of a subtropical climate zone.
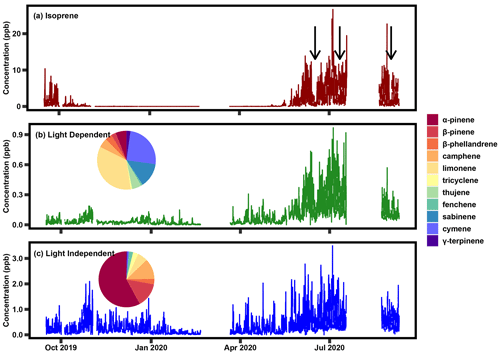
Figure 3Time series of isoprene concentration and the two positive matrix factorization factors between September 2019 and September 2020 and the breakdown of the monoterpene species that contribute to each factor. The black arrows in panel (a) denote the transient periods that are apparent in both the isoprene data (a) and the light-dependent factor (b).
In contrast, while limonene concentrations were similarly lowest in the daytime winter hours, at 0.01 ppb, they were highest during the daytime summer hours, at 0.2 ppb. In fall, winter, and spring, limonene exhibited the same seasonality as α-pinene, with daytime minima and nighttime maxima, though with weaker diurnal variability (Fig. 1b). In summer, however, diurnal trends in limonene concentrations are very different, with a peak in the mid- to late afternoon and evening concentrations higher than at other times of the year. To reach daytime peaks in concentration, daytime emissions of limonene must be high during the day, particularly given that the reaction rates of limonene with the OH radical and ozone are, respectively, 3 and 2.3 times faster than those of α-pinene.
The seasonal rise and fall in the observed daytime peak of limonene, in contrast to the relative stability of α-pinene, is apparent in a spring/summertime comparison of the daytime (07:00–19:00) and nighttime (19:00–7:00) average concentrations (Fig. 2). The full 2-year time series of this plot can be found in the Supplement (Fig. S1). As observed in the diurnal profiles, α-pinene nighttime concentrations are higher than daytime concentrations throughout the year; while concentrations increase in the summer, this increase is observed in both daytime and nighttime concentrations (Fig. 2a). In contrast, while concentrations of limonene are highest at night throughout the early spring, concentrations begin to peak in the daytime in late May (Fig. 2b). From late May through mid-September, concentrations are highest during the day, suggesting a strong daytime source of limonene specifically in the summer, which may be co-emitted with other monoterpenes but is not a strong feature for α-pinene. The daytime peak in limonene is unique to summer and occurs in both years (Figs. 1, 2, and S1). Interestingly, while the daytime peak in summer is relatively consistent across years, nighttime concentrations of limonene in the summer are substantially lower in 2021 compared to 2020 (Fig. S1), suggesting sources for daytime and nighttime limonene that differ in their interannual variation. However, additional years of data are likely necessary to better understand the drivers of this interannual variability. We demonstrate below that the timing of the rise and fall of the strong daytime source of limonene correlates with concentrations of isoprene, a known de novo emitted BVOC, and appears to be a component of a set of light-dependent monoterpene emissions.
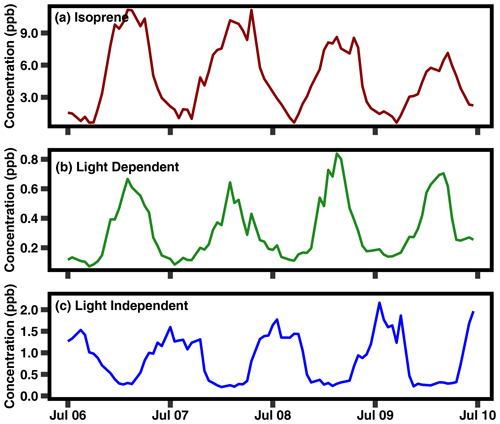
Figure 4A 4 d period in July 2020 of isoprene and the two PMF factors (light dependent and light independent).
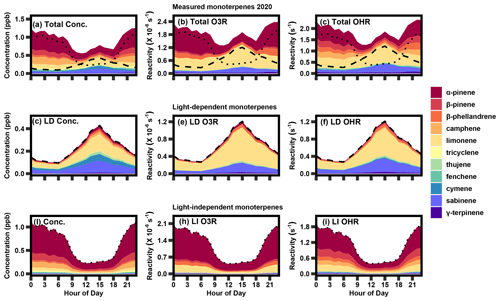
Figure 5The 2020 summer diurnal profile of (a) measured monoterpene concentration, (b) calculated monoterpene ozone reactivity, and (c) calculated OH reactivity, as well as light-dependent (LD) (d) concentrations, (e) ozone reactivity, and (f) OH reactivity and light-independent (LI) (g) concentration, (h) ozone reactivity, and (i) OH reactivity. The dashed lines in panels (a–c) represent the contribution from LD monoterpenes (d, e, f), while the dotted lines represent the contribution from LI monoterpenes (g, h, i).
3.2 Light-dependent and light-independent monoterpene concentration
To better characterize the observed light-dependent monoterpenes and quantify their impacts, the patterns in monoterpenes were deconvolved as two factors using PMF. The determined factors demonstrate a clear separation between a set of monoterpenes that exhibit only nighttime peaks in concentration and a set of compounds that exhibit a tendency toward daytime maxima. Quantitative assessment of the uncertainty in the two-factor solution is performed using bootstrapping, in which 100 runs are performed using arbitrary subsets of data; 95 % of the bootstrap runs reproduce both factors (Table S2) with no unmapped base factors. An unmapped base factor indicates that one or more of the bootstrap runs did not correlate with a determined factor from the base model run (Norris and Duvall, 2014). The Pearson correlation coefficient threshold used for this analysis was the EPA PMF default value of 0.6 (Norris and Duvall, 2014).
A light-dependent factor is present primarily during the summer, characterized by daytime peaks that roughly coincide with the seasonality and variability in isoprene (Fig. 3, with results from 2020 shown; Table S3 contains the percent contribution of each species in each factor in 2020, with results from 2021 shown in Fig. S1). This factor even mirrors the transient decreases in concentrations observed in isoprene, such as those observed in June 2020, July 2020, and September 2020, denoted with black arrows in Fig. 3a. The largest contributor to the light-dependent factor is limonene (roughly one-third), followed by cymene, sabinene, and a relatively small contribution from α-pinene, denoted by the pie charts above each factor time series. Table S2 in the Supplement indicates the percent contribution for the species in each factor. A more dominant factor contains most of the α- and β-pinene and exhibits a diurnal pattern and seasonality that is more in line with what is typical of temperature-driven monoterpenes; this factor is referred to as “light independent” to distinguish it and because the dominant biogenic emission model (Model of Emissions of Gases and Aerosols from Nature – MEGAN) distinguishes between emission pathways as being light dependent (i.e., de novo) vs. light independent (i.e., temperature-driven volatilization from storage pools; Guenther et al., 2012). Interpretation of factors is further supported by their diurnal trends, a representative sample of which is shown in Fig. 4, with a sampling from summer 2021 shown in Fig. S4. The light-dependent factor peaks at midday, following a similar temporal pattern as isoprene. We infer these monoterpenes to be emitted through similar processes to isoprene and attribute them to de novo emissions. Additionally, isoprene concentrations correlate reasonably well with light-dependent monoterpenes during summer (r2=0.57; Fig. S3a) and do not correlate with light-independent monoterpenes (r2=0.01; Fig. S3b). In contrast, the higher-concentration monoterpene factor peaks in the nighttime to early morning hours, following more typical monoterpene diurnal patterns. We attribute these monoterpene concentrations to temperature-driven, light-independent emissions of monoterpenes. It is important to note that most monoterpenes are split between the two factors and vary within the year, likely because of changing phenological patterns. While some compounds such as α- and β-pinene are almost wholly found in the light-independent factor, most of the compounds in the light-dependent factor, such as limonene, still exhibit a strong light-independent component.
Overall, the light-dependent factor accounts for ∼ 25 % of the summertime monoterpene concentration, but at times the light-dependent factor may contribute significantly or even dominate concentrations due to their differing diurnal variability in emissions. Interestingly, more than 85 % of the most dominant monoterpenes, including α-pinene, β-pinene, tricyclene, fenchene, and camphene are found almost entirely in the light-independent factor (Table 1). Conversely, more than 85 % of cymene, sabinene, and thujene are found in the light-dependent factor (Table 1). A small number of species are more evenly split, with larger percentages of their concentrations attributed to light-dependent emissions than light-independent emission in the summer months. These species include β-phellandrene, limonene, and γ-terpinene (Table 1).
3.3 Ozone and OH reactivity
Despite the low contribution of the light-dependent factor to total monoterpene concentration, this factor has a large impact on ozone and OH reactivity. Comparing the stacked diurnal concentration profile (Fig. 5a) to the stacked ozone and OH reactivity diurnal profile (Fig. 5b, c) in summer, limonene and α-pinene prevail as the major contributors to both ozone and OH reactivity. While the concentration profile shows that the majority of species peak at night, there is a slight increase in the middle of the day, owing to the contribution from light-dependent emissions. When this profile is multiplied by the respective reaction rate constant for each species and oxidant, there is a clear midday peak that is a significant contributor to ozone and OH reactivity in the summer. Furthermore, the largest contributor to total ozone and OH reactivity is limonene, despite its relatively low contribution to total concentration due to its high reaction rate with each atmospheric oxidant. PMF results from 2020–2021 are generally very similar to the results shown here in terms of diurnality and composition (Fig. S5).
A majority of the highly reactive isomer limonene is associated with light-dependent monoterpenes (57 %), while the more dominant α-pinene concentrations are almost entirely attributed to pool emissions (98 %; Table 1). Sabinene is also a notable contributor to the light-dependent mixture, contributing approximately 30 % to concentration, 25 % to ozone reactivity, and 33 % to OH reactivity; it is not found in the light-independent mixture. The major contribution of limonene and sabinene to the light-dependent monoterpene mixture makes light-driven emissions particularly reactive, with a reaction rate roughly 1.5 times that of the light-independent mixture for both ozone and OH reactivity. This daytime peak has an significant impact on daytime ozone and OH reactivity (Fig. 5e, f), such that calculated summertime ozone and OH reactivity consequently have a less pronounced diurnal pattern and are roughly uniform throughout the day (average of 1.4–2.4 × 10−6 s−1 for ozone reactivity and 1–2 s−1 for OH reactivity) during the summer months. Even in the summer, when concentrations of light-dependent monoterpenes are highest, the diurnal profile of the total monoterpene chemical class roughly follows that of α-pinene (Fig. 5a), with only moderate daytime concentrations. However, this average profile is a combination of a nighttime peak dominated by light-independent compounds (Fig. 5g) and a daytime peak dominated by light-dependent compounds (Fig. 5d) that has a greater contribution to daytime reactivity. Consequently, understanding light-dependent monoterpenes is critical, not only to better characterize the carbon cycle and predict long-term trends but also because it has immediate and substantial impacts on the atmospheric oxidant budget in the summer that would be overlooked when considering monoterpenes as a bulk compound class.
Using 2 years of hourly speciated BVOC concentrations collected at a meteorological tower in central Virginia, we identify and quantify diurnal and seasonal variability in chemically speciated monoterpenes. Though a majority of monoterpene concentrations exhibit temporal behavior expected from pool emissions whose flux rates are independent of light, we identify a minor (in mass terms) contribution from monoterpenes with seasonality and diurnal variability that show a strong light dependence and resemble de novo emissions. These light-dependent monoterpene emissions are strongest in the summer, where they contribute ∼ 25 % to total monoterpene concentrations, with smaller contributions in other seasons. However, the minor contribution to total monoterpene mass obscures their major impact on ozone and OH reactivity. Due to differences in the temporal variability in the two monoterpene classes and the significantly higher reaction rates of the light-dependent mixture, we observe high ozone and OH reactivity in the summer daytime that is not well captured by bulk monoterpene concentration. This reactivity is dominated by limonene, which contributes ∼ 80 % and ∼ 65 % to light-dependent sourced ozone and OH reactivity and roughly ∼ 20 % to light-independent sourced ozone and OH reactivity. In a changing climate, these BVOC emission sources may vary. For example, drought may decrease vegetative growth, which could increase the per unit leaf area in emissions for stored (i.e., light independent) monoterpenes, even as canopy leaf area declines (Lewinsohn et al., 1993; Funk et al., 2004). But increased precipitation can decrease photosynthesis, causing a decrease in de novo (i.e., light dependent) emissions (Lewinsohn et al., 1993; Funk et al., 2004). These findings highlight the need for speciated long-term monitoring studies with a focus on capturing low concentration, but highly reactive, species.
A significant implication of this work is that the unique drivers of each monoterpene isomer challenge our ability to view this class monolithically or simplify its variability. Measurement studies focused on total BVOC classes may be sufficient to gain an understanding of total BVOC concentrations but demonstrate a need for isomer-resolved understanding of oxidant reactivity. For example, while this work supports the general conclusion that light-dependent monoterpenes are a minor component (reflected in current emission models; Guenther et al., 2012) and are supported by measurement studies (Bouvier-Brown et al., 2009; Kesselmeier and Staudt, 1999; Niinemets et al., 2002; Tingey et al., 1979; Davison et al., 2009; Taipale et al., 2011; Rinne et al., 2002), the composition and temporal variability in light-dependent monoterpenes, in addition to their high per-molecule reactivity, drive strong atmospheric impacts. It is clear that drivers of limonene and sabinene emissions are particularly critical for understanding this ecosystem (see also McGlynn et al., 2021). Capturing the detail of this or any monoterpene in emissions models is difficult, as the light-dependent fraction depends on plant species and other ecological variables. However, it is clear there is some disconnect between the results here and dominant models that, for example, estimate α-pinene as being more strongly light-dependent than limonene (Guenther et al., 2012) and do not tend to vary light-dependent fraction by plant function type. These small gaps in our understanding of what drives monoterpene emissions may lead to significant uncertainty in models or outcomes with respect to oxidation and oxidant chemical loss. Furthermore, oxidation of these compounds ultimately leads to SOA formation, but the impacts on this process of the different long- and short-term temporal trends of each isomer is difficult to assess. It is clear from the existing literature that SOA yields vary significantly by isomer and are dependent on structure (Lee et al., 2006; Faiola et al., 2018; Friedman and Farmer, 2018; Lim and Ziemann, 2009). Consequently, we anticipate that light-dependent and light-independent monoterpenes vary in their average SOA yields, and the seasonal and interannual variability observed in this work has significant regional impacts on aerosol loadings. Unfortunately, these differences are difficult to quantify, with previous studies even disagreeing on whether α-pinene or limonene has a higher SOA yield (Faiola et al., 2018; Friedman and Farmer, 2018). Enhanced monitoring of BVOC concentrations and emissions needs to be supplemented by improved chemically resolved measurements of SOA concentrations and formation processes in order to enhance our understanding of the contribution of these emissions to SOA mass loadings.
The data set can be found at https://doi.org/10.17632/jx3vn5xxcn.3 (McGlynn and Isaacman-VanWertz, 2022).
The supplement related to this article is available online at: https://doi.org/10.5194/bg-20-45-2023-supplement.
DFM analyzed the data, ran the PMF algorithm, produced the figures, and wrote the paper. GF assisted in the deploying and maintenance of the instrument, and LERB assisted in keeping the instrument storage shed running. MTL and SEP contributed intellectual knowledge and provided feedback on the paper. GIV directed the instrument development, researched directions, and wrote the paper.
The contact author has declared that none of the authors has any competing interests.
Publisher’s note: Copernicus Publications remains neutral with regard to jurisdictional claims in published maps and institutional affiliations.
This research has been funded by the National Science Foundation (grant nos. AGS 1837882 and AGS 1837891). Tower maintenance and operation was supported in part by the Pace Endowment. Deborah F. McGlynn and Laura E. R. Barry were supported in part by Virginia Space Grant Consortium Graduate Research Fellowships. The authors gratefully acknowledge the assistance of Koong Yi and Bradley Sutliff, for their support in the upkeep and maintenance of the instrument at Pace Tower.
This research has been supported by the Division of Atmospheric and Geospace Sciences (grant nos. AGS 1837882 and AGS 1837891).
This paper was edited by Paul Stoy and reviewed by two anonymous referees.
Atkinson, R. and Arey, J.: Gas-phase tropospheric chemistry of biogenic volatile organic compounds: A review, Atmos. Environ., 37, 197–219, https://doi.org/10.1016/S1352-2310(03)00391-1, 2003a. a
Atkinson, R. and Arey, J.: Atmospheric Degradation of Volatile Organic Compounds, Chem. Rev., 103, 4605–4638, https://doi.org/10.1021/cr0206420, 2003b. a
Atkinson, R., Aschmann, S. M., and Arey, J.: Rate constants for the gas-phase reactions of OH and NO3 Radicals and O3 with sabinene and camphene, Atmos. Environ. Pt. A, 24, 2647–2654, https://doi.org/10.1016/0960-1686(90)90144-C, 1990a. a
Atkinson, R., Hasegawa, D., and Aschmann, S. M.: Rate constants for the gas-phase reactions of O3 with a series of monoterpenes and related compounds at 296 ± 2 K, Int. J. Chem. Kin., 22, 871–887, https://doi.org/10.1002/kin.550220807, 1990b. a
Atkinson, R., Baulch, D. L., Cox, R. A., Crowley, J. N., Hampson, R. F., Hynes, R. G., Jenkin, M. E., Rossi, M. J., and Troe, J.: Evaluated kinetic and photochemical data for atmospheric chemistry: Volume II – gas phase reactions of organic species, Atmos. Chem. Phys., 6, 3625–4055, https://doi.org/10.5194/acp-6-3625-2006, 2006. a
Bouvier-Brown, N. C., Goldstein, A. H., Gilman, J. B., Kuster, W. C., and de Gouw, J. A.: In-situ ambient quantification of monoterpenes, sesquiterpenes, and related oxygenated compounds during BEARPEX 2007: implications for gas- and particle-phase chemistry, Atmos. Chem. Phys., 9, 5505–5518, https://doi.org/10.5194/acp-9-5505-2009, 2009. a, b, c, d, e
Chan, W.: The Fate of Biogenic Hydrocarbons within a Forest Canopy: Field Observation and Model Results, Ph.D. thesis, University of Virginia, LibraETD, https://doi.org/10.18130/V3MV8J, 2011. a
Davison, B., Taipale, R., Langford, B., Misztal, P., Fares, S., Matteucci, G., Loreto, F., Cape, J. N., Rinne, J., and Hewitt, C. N.: Concentrations and fluxes of biogenic volatile organic compounds above a Mediterranean Macchia ecosystem in western Italy, Biogeosciences, 6, 1655–1670, https://doi.org/10.5194/bg-6-1655-2009, 2009. a
Delwiche, C. F. and Sharkey, T. D.: Rapid appearance of 13C in biogenic isoprene when 13CO2 is fed to intact leaves, Plant Cell Environ., 16, 587–591, https://doi.org/10.1111/j.1365-3040.1993.tb00907.x, 1993. a
Faiola, C. L., Buchholz, A., Kari, E., Yli-Pirilä, P., Holopainen, J. K., Kivimäenpää, M., Miettinen, P., Worsnop, D. R., Lehtinen, K. E., Guenther, A. B., and Virtanen, A.: Terpene Composition Complexity Controls Secondary Organic Aerosol Yields from Scots Pine Volatile Emissions, Sci. Rep., 8, 1–13, https://doi.org/10.1038/s41598-018-21045-1, 2018. a, b
Fischbach, R. J., Staudt, M., Zimmer, I., Rambal, S., and Schnitzler, J.-P.: Seasonal pattern of monoterpene synthase activities in leaves of the evergreen tree Quercus ilex, Physiol. Plantarum, 114, 354–360, https://doi.org/10.1034/j.1399-3054.2002.1140304.x, 2002. a
Frazier, G., McGlynn, D. F., Barry, L. E., Lerdau, M., Pusede, S. E., and Isaacman-VanWertz, G.: Composition, concentration, and oxidant reactivity of sesquiterpenes in the southeastern U.S., Environ. Sci.-Atmos., 2, 1208–1220, https://doi.org/10.1039/d2ea00059h, 2022. a, b
Friedman, B. and Farmer, D. K.: SOA and gas phase organic acid yields from the sequential photooxidation of seven monoterpenes, Atmos. Environ., 187, 335–345, https://doi.org/10.1016/j.atmosenv.2018.06.003, 2018. a, b
Funk, J. L., Mak, J. E., and Lerdau, M. T.: Stress-induced changes in carbon sources for isoprene production in Populus deltoides, Plant Cell Environ., 27, 747–755, https://doi.org/10.1111/j.1365-3040.2004.01177.x, 2004. a, b
Ghirardo, A., Koch, K., Taipale, R., Zimmer, I., Schnitzler, J.-P., and Rinne, J.: Determination of de novo and pool emissions of terpenes from four common boreal/alpine trees by 13CO2 labelling and PTR-MS analysis, Plant Cell Environ., 33, 781–792, https://doi.org/10.1111/j.1365-3040.2009.02104.x, 2010. a, b, c, d
Guenther, A.: Seasonal and spatial variations in natural volatile organic compound emissions, Ecol. Appl., 7, 34–45, 1997. a
Guenther, A., Nicholas, C., Fall, R., Klinger, L., Mckay, W. A., and Scholes, B.: A global model of natural volatile organic compound emissions, J. Geophys. Res., 100, 8873–8892, 1995. a
Guenther, A., Greenberg, J., Harley, P., Helmig, D., Klinger, L., Vierling, L., Zimmerman, P., and Geron, C.: Leaf, branch, stand and landscape scale measurements of volatile organic compound fluxes from U.S. woodlands, Tree Physiol., 16, 17–24, https://doi.org/10.1093/treephys/16.1-2.17, 1996. a
Guenther, A., Geron, C., Pierce, T., Lamb, B., Harley, P., and Fall, R.: Natural emissions of non-methane volatile organic compounds, carbon monoxide, and oxides of nitrogen from North America, Atmos. Environ., 34, 2205–2230, 2000. a, b
Guenther, A., Karl, T., Harley, P., Wiedinmyer, C., Palmer, P. I., and Geron, C.: Estimates of global terrestrial isoprene emissions using MEGAN (Model of Emissions of Gases and Aerosols from Nature), Atmos. Chem. Phys., 6, 3181–3210, https://doi.org/10.5194/acp-6-3181-2006, 2006. a
Guenther, A. B., Monson, R. K., and Fall, R.: Isoprene and monoterpene emission rate variability: Observations with eucalyptus and emission rate algorithm development, J. Geophys. Res., 96, 10799, https://doi.org/10.1029/91jd00960, 1991. a, b
Guenther, A. B., Jiang, X., Heald, C. L., Sakulyanontvittaya, T., Duhl, T., Emmons, L. K., and Wang, X.: The Model of Emissions of Gases and Aerosols from Nature version 2.1 (MEGAN2.1): an extended and updated framework for modeling biogenic emissions, Geosci. Model Dev., 5, 1471–1492, https://doi.org/10.5194/gmd-5-1471-2012, 2012. a, b, c, d
Haapanala, S., Rinne, J., Hakola, H., Hellén, H., Laakso, L., Lihavainen, H., Janson, R., O'Dowd, C., and Kulmala, M.: Boundary layer concentrations and landscape scale emissions of volatile organic compounds in early spring, Atmos. Chem. Phys., 7, 1869–1878, https://doi.org/10.5194/acp-7-1869-2007, 2007. a
Hakola, H., Hellén, H., Hemmilä, M., Rinne, J., and Kulmala, M.: In situ measurements of volatile organic compounds in a boreal forest, Atmos. Chem. Phys., 12, 11665–11678, https://doi.org/10.5194/acp-12-11665-2012, 2012. a
Harley, P., Eller, A., Guenther, A., and Monson, R. K.: Observations and models of emissions of volatile terpenoid compounds from needles of ponderosa pine trees growing in situ: Control by light, temperature and stomatal conductance, Oecologia, 176, 35–55, https://doi.org/10.1007/s00442-014-3008-5, 2014. a
Isaacman-VanWertz, G., Sueper, D. T., Aikin, K. C., Lerner, B. M., Gilman, J. B., de Gouw, J. A., Worsnop, D. R., and Goldstein, A. H.: Automated single-ion peak fitting as an efficient approach for analyzing complex chromatographic data, J. Chromatogr. A, 1529, 81–92, https://doi.org/10.1016/j.chroma.2017.11.005, 2017. a, b
Kesselmeier, J. and Staudt, M.: An Overview on Emission, Physiology and Ecology.pdf, J. Atmos. Chem., 33, 23–88, https://doi.org/10.1023/A:1006127516791, 1999. a
Kroll, J. H. and Seinfeld, J. H.: Chemistry of secondary organic aerosol: Formation and evolution of low-volatility organics in the atmosphere, Atmos. Environ., 42, 3593–3624, https://doi.org/10.1016/j.atmosenv.2008.01.003, 2008. a
Kuang, B. Y., Lin, P., Huang, X. H. H., and Yu, J. Z.: Sources of humic-like substances in the Pearl River Delta, China: positive matrix factorization analysis of PM2.5 major components and source markers, Atmos. Chem. Phys., 15, 1995–2008, https://doi.org/10.5194/acp-15-1995-2015, 2015. a
Lee, A., Goldstein, A. H., Keywood, M. D., Gao, S., Varutbangkul, V., Bahreini, R., Ng, N. L., Flagan, R. C., and Seinfeld, J. H.: Gas-phase products and secondary aerosol yields from the ozonolysis of ten different terpenes, J. Geophys. Res.-Atmos., 111, 1–18, https://doi.org/10.1029/2005JD006437, 2006. a
Lerdau, M. and Gray, D.: Ecology and evolution of light‐dependent and light‐independent phytogenic volatile organic carbon, New Phytol., 157, 199–211, https://doi.org/10.1046/j.1469-8137.2003.00673.x, 2003. a, b
Lerdau, M., Guenther, A., and Monson, R.: Plant Production and Emission of Volatile Organic Compounds, BioScience, 47, 373–383, https://doi.org/10.2307/1313152, 1997. a
Lewinsohn, E., Gijzen, M., Muzika, R. M., Barton, K., and Croteau, R.: Oleoresinosis in Grand Fir (Abies grandis) Saplings and Mature Trees (Modulation of this Wound Response by Light and Water Stresses), Plant Physiol., 101, 1021–1028, https://doi.org/10.1104/pp.101.3.1021, 1993. a, b
Lim, Y. B. and Ziemann, P. J.: Effects of Molecular Structure on Aerosol Yields from OH Radical-Initiated Reactions of Linear, Branched, and Cyclic Alkanes in the Presence of NOx, Environ. Sci. Technol., 43, 2328–2334, https://doi.org/10.1021/es803389s, 2009. a
Loreto, F., Förster, A., Dürr, M., Csiky, O., and Seufert, G.: On the monoterpene emission under heat stress and on the increased thermotolerance of leaves of Quercus ilex L. fumigated with selected monoterpenes, Plant Cell Environ., 21, 101–107, https://doi.org/10.1046/j.1365-3040.1998.00268.x, 1998. a
McGlynn, D. and Isaacman-VanWertz, G.: In-Canopy Biogenic Volatile Organic Compounds Mixing Ratios at the Virginia Forest Lab, Mendeley Data [data set], V3, https://doi.org/10.17632/jx3vn5xxcn.3, 2022. a
McGlynn, D. F., Barry, L. E. R., Lerdau, M. T., Pusede, S. E., and Isaacman-VanWertz, G.: Measurement report: Variability in the composition of biogenic volatile organic compounds in a Southeastern US forest and their role in atmospheric reactivity, Atmos. Chem. Phys., 21, 15755–15770, https://doi.org/10.5194/acp-21-15755-2021, 2021. a, b, c, d, e, f, g, h, i
Niinemets, Ü. and Monson, R. K. (Eds.): Biology, Controls and Models of Tree Volatile Organic Compound Emissions, vol. 5 of Tree Physiology, Springer Netherlands, Dordrecht, https://doi.org/10.1007/978-94-007-6606-8, 2013. a, b, c, d
Niinemets, Ü., Reichstein, M., Staudt, M., Seufert, G., and Tenhunen, J. D.: Stomatal constraints may affect emission of oxygenated monoterpenoids from the foliage of Pinus pinea, Plant Physiol., 130, 1371–1385, https://doi.org/10.1104/pp.009670, 2002. a
Norris, G. and Duvall, R.: EPA Positive Matrix Factorization (PMF) 5.0 Fundamentals and User Guide. United States Environmental Protection Agency (EPA), Office of Research and Development, Washington, U.S. (EPA/600/R-14/2018), 2014. a, b, c, d, e, f, g, h
Panopoulou, A., Liakakou, E., Sauvage, S., Gros, V., Locoge, N., Stavroulas, I., Bonsang, B., Gerasopoulos, E., and Mihalopoulos, N.: Yearlong measurements of monoterpenes and isoprene in a Mediterranean city (Athens): Natural vs anthropogenic origin, Atmos. Environ., 243, 117803, https://doi.org/10.1016/j.atmosenv.2020.117803, 2020. a, b
Pinto, D. M., Tiiva, P., Miettinen, P., Joutsensaari, J., Kokkola, H., Nerg, A.-M., Laaksonen, A., and Holopainen, J. K.: The effects of increasing atmospheric ozone on biogenic monoterpene profiles and the formation of secondary aerosols, Atmos. Environ., 41, 4877–4887, https://doi.org/10.1016/j.atmosenv.2007.02.006, 2007. a
Pollmann, J., Ortega, J., and Helmig, D.: Analysis of atmospheric sesquiterpenes: Sampling losses and mitigation of ozone interferences, Environ. Sci. Technol., 39, 9620–9629, https://doi.org/10.1021/es050440w, 2005. a
Porter, W. C., Safieddine, S. A., and Heald, C. L.: Impact of aromatics and monoterpenes on simulated tropospheric ozone and total OH reactivity, Atmos. Environ., 169, 250–257, https://doi.org/10.1016/j.atmosenv.2017.08.048, 2017. a
Pratt, K. A., Mielke, L. H., Shepson, P. B., Bryan, A. M., Steiner, A. L., Ortega, J., Daly, R., Helmig, D., Vogel, C. S., Griffith, S., Dusanter, S., Stevens, P. S., and Alaghmand, M.: Contributions of individual reactive biogenic volatile organic compounds to organic nitrates above a mixed forest, Atmos. Chem. Phys., 12, 10125–10143, https://doi.org/10.5194/acp-12-10125-2012, 2012. a
Rinne, H. J., Guenther, A. B., Greenberg, J. P., and Harley, P. C.: Isoprene and monoterpene fluxes measured above Amazonian rainforest and their dependence on light and temperature, Atmos. Environ., 36, 2421–2426, https://doi.org/10.1016/S1352-2310(01)00523-4, 2002. a, b, c
Shu, Y. and Atkinson, R.: Rate constants for the gas‐phase reactions of O3 with a series of Terpenes and OH radical formation from the O3 reactions with Sesquiterpenes at 296 ± 2 K, Int. J. Chem. Kinet., 26, 1193–1205, https://doi.org/10.1002/kin.550261207, 1994. a
Staudt, M. and Seufert, G.: Light-dependent Emission of Monoterpenes by Holm Oak (Quercus ilex L.), Naturwissenschaften, 82, 89–92, 1995. a, b, c
Staudt, M., Bertin, N., Frenzel, B., and Seufert, G.: Seasonal Variation in Amount and Composition of Monoterpenes Emitted by Young Pinus pinea Trees – Implications for Emission Modeling, Plant Cell Environ., 35, 77–99, https://doi.org/10.1023/A:1006233010748, 1999. a, b
Steinbrecher, R., Hauff, K., Hakola, H., and Rössler, J.: A revised parameterisation for emission modelling of isoprenoids for boreal plants, Air Pollut. Res. Report, 70, 29–44, 1999. a
Taipale, R., Kajos, M. K., Patokoski, J., Rantala, P., Ruuskanen, T. M., and Rinne, J.: Role of de novo biosynthesis in ecosystem scale monoterpene emissions from a boreal Scots pine forest, Biogeosciences, 8, 2247–2255, https://doi.org/10.5194/bg-8-2247-2011, 2011. a, b, c, d
Tingey, D., Manning, M., Grothaus, L. C., and Burns, W. F.: The Influence of Light and Temperature on Isoprene Emission Rates from Live Oak, Physiol. Plant., 47, 112–118, https://doi.org/10.1111/j.1399-3054.1979.tb03200.x, 1979. a, b
Ulbrich, I. M., Canagaratna, M. R., Zhang, Q., Worsnop, D. R., and Jimenez, J. L.: Interpretation of organic components from Positive Matrix Factorization of aerosol mass spectrometric data, Atmos. Chem. Phys., 9, 2891–2918, https://doi.org/10.5194/acp-9-2891-2009, 2009. a
Yee, L. D., Isaacman-VanWertz, G., Wernis, R. A., Meng, M., Rivera, V., Kreisberg, N. M., Hering, S. V., Bering, M. S., Glasius, M., Upshur, M. A., Gray Bé, A., Thomson, R. J., Geiger, F. M., Offenberg, J. H., Lewandowski, M., Kourtchev, I., Kalberer, M., De Sá, S., Martin, S. T., Alexander, M. L., Palm, B. B., Hu, W., Campuzano-Jost, P., Day, D. A., Jimenez, J. L., Liu, Y., McKinney, K. A., Artaxo, P., Viegas, J., Manzi, A., Oliveira, M. B., De Souza, R., Machado, L. A., Longo, K., and Goldstein, A. H.: Observations of sesquiterpenes and their oxidation products in central Amazonia during the wet and dry seasons, Atmos. Chem. Phys., 18, 10433–10457, https://doi.org/10.5194/acp-18-10433-2018, 2018. a
Yu, H., Guenther, A., Gu, D., Warneke, C., Geron, C., Goldstein, A., Graus, M., Karl, T., Kaser, L., Misztal, P., and Yuan, B.: Airborne measurements of isoprene and monoterpene emissions from southeastern U.S. forests, Sci. Total Environ., 595, 149–158, https://doi.org/10.1016/j.scitotenv.2017.03.262, 2017. a
Zimmerman, P. R.: Testing of hydrocarbones emissions from vegetation, leaf litter and aquatic surfaces, and development of a methodology for compiling biogenic emissions inventories, Tech. Rep., http://ci.nii.ac.jp/naid/10004472642/en/ (last access: 1 November 2022), 1979. a