the Creative Commons Attribution 4.0 License.
the Creative Commons Attribution 4.0 License.
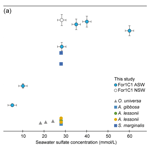
Impact of seawater sulfate concentration on sulfur concentration and isotopic composition in calcite of two cultured benthic foraminifera
Caroline Thaler
Guillaume Paris
Marc Dellinger
Delphine Dissard
Sophie Berland
Arul Marie
Amandine Labat
Annachiara Bartolini
Marine sediments can be used to reconstruct the evolution of seawater [SO] and δ34S over time, two key parameters that contribute to refine our understanding of the sulfur cycle and thus of Earth's redox state. δ34S evolution can be measured from carbonates, barites and sulfate evaporites. [SO] variations can be reconstructed using fluid inclusions in halites, a method that only allows a low-resolution record. Reconstruction of the past sulfur cycle could be improved if carbonates allowed the tracking of both seawater δ34S and [SO] variations in a sole, continuous sedimentary repository. However, most primary carbonates formed in the ocean are biogenic, and organisms tend to overprint the geochemical signatures of their carbonates through a combination of processes often collectively referred to as vital effects. Hence, calibrations are needed to allow seawater δ34S and [SO] reconstructions based on biogenic carbonates. Because foraminifera are important marine calcifiers, we opted to focus on calcite synthesized by individuals of rosalinid benthic foraminifera cultured in the laboratory under controlled conditions, with varying seawater [SO] (ranging from 0 to 180 mM). Our experimental design allowed us to obtain foraminiferal asexual reproduction over several generations. We measured bulk carbonate-associated sulfate (CAS) content and sulfur isotopic composition (δ34SCAS) on samples of tens to hundreds of specimens from a selection of culture media, where [SO] varied from 5 to 60 mM. Increasing or decreasing [SO] with respect to modern-day seawater concentration (28 mM) impacted foraminiferal population size dynamics and the total amount of bioprecipitated carbonate. Foraminiferal CAS concentration increased proportionally with [SO] concentration from 5 mM up to 28 mM and then showed a plateau from 28 to 60 mM. The existence of a threshold at 28 mM is interpreted as the result of a control on the precipitation fluid chemistry that foraminifera exert on the carbonate precipitation loci. However, at high seawater sulfate concentrations (> 40 mM) the formation of sulfate complexes with other cations may partially contribute to the non-linearity of the CAS concentration in foraminiferal tests at high increases in [SO]. Yet, despite the significant effect of seawater [SO] on foraminiferal reproduction and on CAS incorporation, the isotopic fractionation between CAS and seawater remains stable through varying seawater [SO]. Altogether, these results illustrate that CAS in biogenic calcite could constitute a good proxy for both seawater [SO] and δ34S and suggests that sulfate likely plays a role in foraminiferal biomineralization and biological activity.
- Article
(4332 KB) - Full-text XML
-
Supplement
(303 KB) - BibTeX
- EndNote
In the modern ocean, marine organisms control the precipitation of most calcium carbonates through the biomineralization of calcite or aragonite, the two main CaCO3 polymorphs. Biogenic calcium carbonates from the sedimentary record have been used for decades to reconstruct past environmental conditions. At modern sulfate and magnesium concentrations in seawater (about 28 and 50 mM respectively), aragonite precipitates preferentially over calcite in abiotic conditions at room temperature (Bots et al., 2011; Barkan et al., 2020; Goetschl et al., 2019). Seawater sulfate and magnesium concentrations varied over the last 550 Myr, ranging from ∼ 5 to ∼ 28 mM (Horita et al., 2002) and from ∼ 44 to ∼ 55 mM, respectively (Lowenstein et al., 2001; Brennan et al., 2004). Lower and higher seawater sulfate and magnesium concentrations have been shown to match calcitic and aragonitic oceans, where calcite or aragonite forming organisms were favored respectively (Lowenstein et al., 2003; Algeo et al., 2015; Lin et al., 2018, Goestchl et al., 2019). In the modern aragonitic ocean (Sandberg et al., 1983) as well as through parts of the geological past of Earth's history, the occurrence of calcitic organisms (e.g., foraminifera, coccolithophorids, some mollusks, bryozoans and coralline algae) could thus appear as a paradox. These calcitic organisms growing in aragonite oceans with high sulfate content would then have developed adaptive strategies and exerted a high degree of biological control in calcite bioprecipitation and sulfate incorporation, which need to be better understood.
Among the main calcite synthesizers, foraminifera are unicellular eukaryotes that build mainly calcitic (rare aragonitic species exist) shells named “tests” that accumulate on the ocean seafloor (Schiebel, 2002; Langer, 2008). As foraminifera build their tests, elements present in seawater get incorporated as traces in the biomineral structure. Sulfur is assumed to be incorporated in the calcium carbonate lattice structure as SO by replacing a CO group (Kontrec et al., 2004; Fernández-Diaz et al., 2010) and is referred to as CAS, for carbonate-associated sulfate. This has been illustrated by an increase in in benthic foraminiferal calcite as a function of seawater [CO] decrease (van Dijk et al., 2017). Paris et al. (2014) evidenced that the planktic species Orbulina universa faithfully records the [SO] [Ca2+] ratio of the seawater in which it grew for [SO] values from 18 to 28 mM. These encouraging results, however, needed to be tested on benthic species and on a wider range of [SO], to cover deep time oceanic values, which varied from less than 5 mM up to 28 mM nowadays (Algeo et al., 2015) and potentially beyond, during important volcanic events in the past, or in the vicinity of sulfate-rich volcanic hydrothermal fluids on the seabed (Gamo et al., 1997; Laakso et al., 2020). Furthermore, the possibility that foraminiferal calcite could serve both as a [SO] and δ34S record needs to be further validated. While so far measurements in biogenic carbonates have shown that sulfur isotopes are systematically fractionated by ± 1 ‰ from seawater (Kampschulte et al., 2001; Paris et al., 2014; Present et al., 2015; Rennie et al., 2018), recent experiments of abiotic CaCO3 precipitation have shown that a 2 ‰–5 ‰ fractionation of sulfur isotopes between aqueous sulfate and CAS in calcite covaries with [SO] and, to a lesser extent, with precipitation rates (Barkan et al., 2020). There is thus a contrasting abiotic–biotic behavior that needs to be elucidated in order to determine whether calcitic foraminiferal tests could be used as a paleoenvironmental archive for the sulfur cycle and suggests the possibility that seawater [SO] variations impact foraminiferal biocalcification and carbonate production.
To answer these questions, we grew two strains of Rosalinidae (Fig. 1), which are asymbiotic benthic foraminifera, at constant temperature, pH and salinity over a range from 0 to 180 mM of seawater [SO]. Compared to planktic foraminifera, benthic foraminifera have two advantages: (i) they cover deeper geological times, and (ii) they can reproduce more easily in experimental conditions.
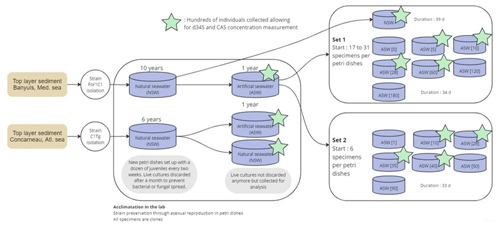
Figure 1Experimental design workflow diagram illustrating the sampling, acclimation, and experiments set 1 and set 2. Stars highlight samples where δ34S and CAS analysis could be performed.
In general, in both planktic and benthic foraminiferal culture experiments performed to calibrate geochemical proxies, populations of individuals captured in the wild do not have the time to adapt to the experimental conditions because maintaining foraminiferal reproductions over several generations is a complicated task. Therefore, measurements of geochemical proxies are usually performed either on the few test chambers that precipitated in the experimental medium (e.g., Dissard et al., 2010a, b; van Dijk et al., 2017; Schmidt et al., 2022) or on whole tests that include the initial chambers grown in the natural marine environment prior to collection (e.g., Paris et al., 2014; Le Houedec et al., 2021) and account for this in some way, e.g., using size–mass relationships or labels. Our experiment was carefully designed to obtain several generations grown over several weeks in each experimental medium, ensuring both acclimatization and full precipitation of the test in the medium. Only live individuals that had fully grown under the experimental conditions were collected for analysis, as empty shells of dead individuals were discarded at each previous water change. We analyzed population size dynamics, as well as shell δ34S and [SO], in bulk samples of tens to hundreds of specimens in each medium to shed light on the mechanisms of sulfate incorporation in benthic foraminiferal calcite.
2.1 Culture conditions
2.1.1 Long-term culture with asexual reproduction
Culture experiments were conducted at the French National Museum of Natural History (Muséum national d'Histoire naturelle, MNHN) in the free-living protist collection facilities (collection group: Biological Resources of Living and Cryopreserved Cells; Collection of Unicellular Eukaryotes) on two previously cloned foraminiferal strains adapted to in vitro cell culture in 90 mm diameter Petri dishes with natural sea water (NSW) and fed with Chlorogonium sp. (specimen MNHN-CEU-2016-0001), a freshwater microalga. Two strains, namely, For1C1 (specimen MNHN-CEU-2016-0075) and C1Tg (specimen MNHN-CEU-2016-0075) (Figs. 1 and 2), were isolated from the top layer of sediments collected from Banyuls sea shore (Mediterranean French coast) in 2006 and Concarneau (Atlantic French coast) in 2011, respectively.
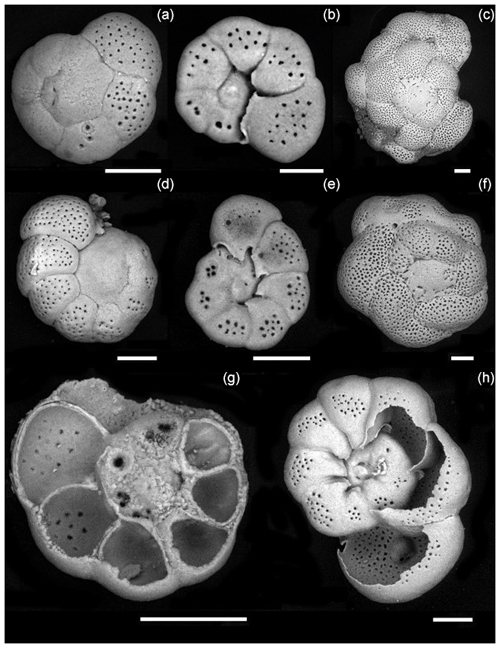
Figure 2Foraminiferal strains cultured in this study. For1C1: (a) Rosalina like morphotype (11–12 chambers) reproducing asexually, dorsal view, (b) same as (a), ventral view, (c) morphotype with more than 12 chambers, starting as Rosalina morphotype and then developing annular disposition of the last chambers, dorsal view. C1Tg: (d) Rosalina like morphotype, (e) same as (d), ventral view, (f) morphotype with annular arrangement of the last chambers, dorsal view. (g, h) Ventral view of C1Tg with a broken test permitting to see the layered structure of the test's wall (g) and the foramen position inside of the test (h). Scale bar: 50 µm, SEM picture in back scattered electron (BSE) mode operated at 10 to 22 mPa and 20 000 kV.
Both strains were maintained through asexual reproduction (Fig. 3), using the following method: foraminifera were cultured in 90 mm diameter Petri dishes filled with 0.22 µm filtered NSW from Banyuls, France, for the For1C1 strain or from Concarneau, France, for the C1Tg strain (Fig. 1).
The NSW was kept in a cold room for at least a month, and its pH (NBS scale) was adjusted to 8.2 through addition of NaOH and/or HCl, before use. The Petri dishes were kept at 22 ∘C in an incubator (Memmert IPP 110 plus) equipped with cold white-light modules (5500 K) with a 12 h day–12 h night cycle. Water in the Petri dishes was changed once a week and foraminifera fed with living freshwater algae (Chlorogonium sp.). The algae were cultured in Basal Bold medium at 25 ∘C under medium light intensity and suspended in sterile pH 8.2 NSW after three steps of rinsing with NSW. Live algae can have a major impact on the seawater carbonate chemistry system by reproducing and consuming CO2 through photosynthesis. As freshwater algae, the Chlorogonium cells died immediately in seawater, without undergoing lysis. This prevents those not eaten by foraminifera from spreading and/or being metabolically active, and thus they do not influence the seawater chemistry conditions within the Petri dishes. The use of live freshwater instead of seawater algae to feed foraminifera is therefore an innovative approach that is particularly suited to long-term culture experiments for the calibration of foraminiferal geochemical proxies, where seawater chemical conditions must be kept under control. Every other week a new Petri dish was set up with a dozen of new juveniles (pre-adults below the age of asexual reproduction, characterized by test with ∼ 10 chambers). Live cultures were discarded after a month to prevent bacterial or fungal spread.
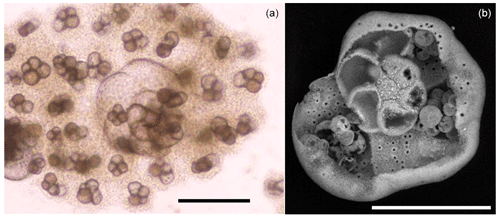
Figure 3Asexual reproduction of an individual of the For1C1 strain. (a) Light microscope image of a megalospheric schizont adult that has ∼ 12 visible chambers and whose cell has divided asexually into viable juveniles (for further details, see Appendix A). The darker appearance of the juveniles compared to the adult is due to the presence of cellular material. After division, the adult is empty and its test partially dissolved, as shown in the SEM micrograph (b). Scale bar: 100 µm.
2.1.2 Culture in artificial seawater with varying [SO]
In 2016, the two foraminiferal strains (For1C1 and C1Tg) were transferred to 0.22 µm filtered artificial seawater (ASW) mimicking NSW (Fig. 1). The ASW was prepared following Kester et al. (1967). The total salinity was 35.0 g L−1, and the main ionic concentrations were as follows (in mM): Cl− 543.9, Na+ 467.3, SO 28.2, Mg2+ 53.1, Ca2+ 9.9, K+ 10.0, HCO 2.3, Br− 0.8, H3BO3 0.4, Sr2+ 0.1 and F− 0.1. After equilibration with the atmosphere for 2 to 3 h, the pH of ASW was adjusted to pH 8.2 by the addition of NaOH and HCl. ASW and NSW were sterilized by filtration on a 0.22 µm filter. The acclimation to ASW lasted approximately for a year (with foraminifera being transferred to new 90 mm diameter Petri dishes monthly) without any noticeable effect on the foraminiferal life cycle and morphology. Over this period of time, batches of several hundreds of foraminifera of each species (strains For1C1 and C1Tg), cultured either in ASW or NSW, were sampled for [SO] and δ34S composition measurements. The C1Tg strain was only used for [SO] and δ34S composition measurements of specimens from media in ASW and NSW at the current seawater average [SO] of 28 mM, whereas the For1C1 strain was also used for [SO] and δ34S composition measurements of specimens from media with different [SO].
To produce media with different [SO], we created an ASW without SO (hereafter ASW[0]) and another with [SO] = 180 mM (hereafter ASW[180]). The amount of NaCl in those two media was adjusted to keep the total salinity constant (35.06 g L−1). Na+ concentrations for ASW[0] and ASW[180] were 479 and 402 mM, respectively, representing a maximum 24 % change, while the Cl− concentrations were 612 and 175 mM, respectively, representing a maximum 71 % change, for a maximum 180 % change in sulfate concentration.
ASW[0] and ASW[180] were mixed in various proportions in order to obtain nine other ASWs with the following [SO]: 1, 5, 10, 35, 40, 50, 60, 90 and 120 mM. Each of these media had the same salinity as ASW (35.06 g L−1), pH (8.2), DIC (dissolved inorganic carbon: [CO2] + [H2CO3] + [HCO] + [CO]) and ALK (alkalinity). For1C1 was the sole strain grown under different SO concentrations (Fig. 1).
In a first set of experiments (set 1), 22 to 31 For1C1 individuals (Fig. 1, Table 1) with ∼ 10 chambers each were transferred from ASW to new 60 mm diameter Petri dishes filled with the following media: ASW (hereafter ASW[28]), ASW[0], ASW[5], ASW[10], ASW[60], ASW[120] and ASW[180] and then cultured for 34 d. In parallel, 17 individuals so far cultured in natural seawater were moved to a new 60 mm diameter Petri dish containing NSW from Banyuls and were cultured for 39 d.
Table 1Weekly number of accumulated live individuals incremented by reproduction for each medium at different [SO].
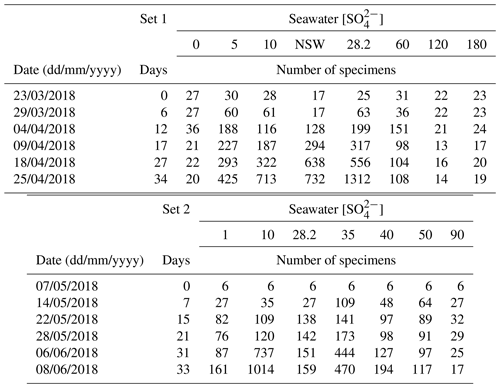
In a second set of experiments designed to refine the concentration step between 0 and 90 mM (set 2), six individuals of For1C1, also presenting ∼ 10 chambers each, were transferred from ASW to new 60 mm diameter Petri dishes and were cultured for 33 d in the following media: ASW[28], ASW[1], ASW[10], ASW[35], ASW[40], ASW[50] and ASW[90] (Fig. 1, Table 1).
For populations of more than approximately 300 individuals, as obtained in media with concentration ranging from 5 to 35 mM sulfate, the specimens were distributed over several 60 mm diameter Petri dishes (up to 3) to avoid problems associated with overpopulation. In both sets of experiment, Chlorogonium fed to foraminifera were rinsed and suspended in the media corresponding to each Petri dish prior to their addition. Each week, live individuals were counted in each environment. As the species under study remains attached to the substrate when alive, individuals that no longer adhered to the Petri dishes, and therefore floated, were considered dead and not counted. However, it should be noted that rare dead individuals, which can sometimes be identified as unequivocally empty tests or as individuals without reticulopodial activity and/or change in color for several days (Bernhard, 2000), may remain attached and might have been counted as alive. At the same time, a few living adults may also have become detached from the substrate and were therefore not counted as alive. As these cases are rare, the error generated by these two phenomena is largely covered by the error bar on the count in growing samples, while in samples not growing from the inoculum, living cells remain estimated. After counting, we sampled 6 mL of water through a 0.2 µm filtered for DIC and [SO] measurements in gastight Exetainer© tubes full to the brim and stored at 5 ∘C. Consecutively, pH was measured using a Hach PHC281101 probe calibrated following the three-point procedure (Hach singlet solutions calibrated against NIST standards, precision of ± 0.01 pH unit). Finally, the old water was completely replaced by fresh sterile water.
2.1.3 DIC analyses
DIC analyses were performed using 3 mL samples of seawater that were slowly withdrawn from each assay through the Exetainer© rubber septa using needle syringes. Ultra-pure helium gas was injected in each vial during sampling to ease solution withdrawal and to prevent atmospheric CO2 contamination. Each 3 mL sample was injected into a new Exetainer© vial, previously flushed with ultra-pure helium gas (2.5 bar) and loaded with 0.3 mL of 100 % H3PO4. Acidification with pure H3PO4 converts the total DIC of the sample into gaseous CO2, which was allowed to degas and mix with the helium gas overnight under shaking. The CO2 and the He mix was then sampled with an autosampler and sent to a dual-inlet FinniganTM DELTAplus XP isotope ratio mass spectrometer (Thermo Fisher Scientific) (reproducibility = ± 0.05 ‰) at IPGP, Paris. [DIC] was quantified using the linear relationship between DIC concentration and intensity of the 44 (12C16O16O) peak provided by the mass spectrometer (Assayag et al., 2006). This linear relationship was established based on repeated analyses of internal laboratory carbonate standards calibrated against international standards (100 % calcite), run in different aliquots. The reproducibility for [DIC] measurements was ± 5 % of the measured values (1σ).
2.2 Collection and rinsing procedure of the tests for geochemical analyses
At the end of the culture experiments (which varied between 34 and 39 d), all live individuals of the strain For1C1, as specified above, still attached to the substrate from each Petri dish were recovered for geochemical analyses. Individuals from set 1 and set 2, grown under the same conditions (same medium [SO]), were not combined for analysis. They were measured separately. Each sample typically weighed few milligrams. The collected tests were rinsed three times in Milli-Q water (basified to pH 9.5 with NH4OH) to remove all traces of salts without dissolving the carbonate phase. In order to remove fresh organic matter, foraminifera were cleaned following Paris et al. (2014): foraminifera were bathed in a NaOH (0.5 M) + H2O2 (15 %) solution at 60 ∘C for 30 min. They were then rinsed three times in basified Milli-Q water and dried overnight at 50 ∘C in a drying oven. All samples were then dissolved at Centre de Recherches Pétrographiques et Géochimiques (CRPG; Nancy) in 0.5 mL of 1 % to 2 % HCl. In addition, in order to determine how the remaining traces of organic matter could affect δ34S measurements, some individuals from both For1C1 and C1Tg strains were dissolved in aqua regia (a mix of concentrated HNO3 and HCl), without prior cleaning in NaOH and H2O2. They were left overnight at 120 ∘C and dried out. All acids were distilled at CRPG and the 18.2 MΩ water purified through a Helga device (Veolia).
2.3 Geochemical analyses
2.3.1 CAS concentration analysis
In order to determine the SO Ca2+ ratio of the tests, two dissolved foraminiferal calcite aliquots of 50 µL were used to independently measure the sulfate and calcium concentrations of the samples. To measure [SO], one of the 50 µL aliquots was diluted in 200 µL of 18.2 MΩ water and ran on a Metrohm ion chromatography system (ICS). The calcium content of the samples was measured using an X-series II ICP-MS using the second aliquot that was dried out and taken up in 3 mL of 2 % HNO3. For the latter, data were measured in groups of five bracketed by a 5.3 ppm Ca standard solution and bracketed assuming linear drift between two standards. In both cases, a calibration line was established to convert the signal to concentrations using homemade concentration standards. The typical reproducibility for sulfate and calcium concentrations is better than 2 % based on multiple measurements of a diluted seawater solution for sulfate and of the standard solution for calcium.
2.3.2 δ34S analysis
Sulfate isolation from the carbonate matrix was performed by ionic chromatography using the anionic resin Biorad AG1X8 (Paris et al., 2014) using precleaned disposable Biorad columns. Each column was prepared by loading 0.6 mL of resin and rinsed with 10 % HNO3 (2×10 CV–1 CV = 1 column volume = 0.6 mL), 33 % HCL (2×10 CV) and 0.5 N HCl (1 × 10CV). After introducing the dissolved carbonates sample on the resin, the column was rinsed with ultrapure water (5 × 5CV) to remove cations. SO was then eluted with 0.45 M HNO3 (3 × 2CV). Each batch of columns included a sample of 50 µl of seawater as a reference and total procedure blanks. After elution, samples were dried out on hotplates with open lids (105 ∘C). The total procedure blank was measured at 0.12 nmol S ± 47 % RSD (relative standard deviation) with a δ34S value of 6.1 ± 3.5 ‰ 1σ, while the analyzed samples contained between at least 23 nmol S (For1C1 grown in ASW[60]). Overall, a blank correction modifies values only within error bars and is thus not applied here.
Purified samples were analyzed on the Thermo Scientific Neptune Plus MC-ICP-MS at the CRPG using a standard-sample bracketing method (Paris et al., 2013). Samples were run at high resolution using an Aridus II desolvating membrane to decrease oxide and hydride interferences. Isotopic ratios were collected at 32 and 34 as 1 block of 50 cycles of 4.2 s each. Data were corrected offline for instrumental fractionation, drift and background following Paris et al. (2013). Each sample was measured twice on the Neptune, and the value provided is an average of both measured ratios. The bracketing Na2SO4 solution had been previously calibrated against international standard IAEA S1 and checked against IAEA S2 and S3. Seawater samples ran during each Neptune sessions ensure that the data are not biased. Seawater external replicates were measured in association with those samples. They yield an average δ34S value of 21.1 ± 0.2 ‰ (n=4), in full agreement with published values (e.g., Paris et al., 2013; Present et al., 2015; Rennie et al., 2018). Because carbonate samples were too small to measure full external replicates, we assume the reproducibility for all δ34S measurements to be the same as seawater (± 0.2 ‰; 2σ), with the exception of For1C1 grown in ASW [40] and [60]. In these two cases, the reproducibility is calculated based on the weighted mean of the internal errors multiplied by the standard deviation of the external normalized deviates (Paris et al., 2013), yielding a 2σ smaller than 0.2 ‰ except for these two samples (0.25 and 0.35 ‰ respectively).
3.1 Population growth in each medium
Individuals For1C1 are morphologically similar to Rosalina (Fig. 2). They reproduced asexually when their tests reached a development of 11–12 chambers (Figs. 3 and 4). Under standard culture conditions of low cell density (i.e., where cells do not compete for food), which in our case was less than about 300 individuals per Petri dish, the reproductive cycle lasted ∼ 12 to 15 d and individuals “died” after asexual reproduction by dividing themselves, usually into 20 to 40 viable juveniles, leaving an empty test (Fig. 3). Adult specimens were smaller than the traditional foraminiferal fraction obtained from sieving (through > 125 µm mesh) in geochemical studies; they thus may well be common and rarely collected because of their size. A morphological and taxonomic description of the cultured strains is available in the Appendix A. The weekly number of accumulated live individuals incremented by reproduction is given in Fig. 5 and Table 1.
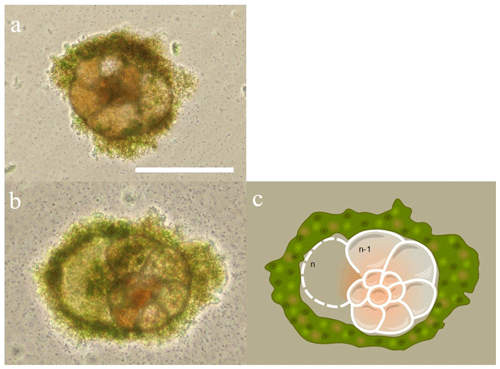
Figure 4Chamber formation during day 2 of the set 1 series of experiment. Two For1C1 individuals (from Banyuls) in (a) ASW[5] (containing 5 mM SO) and (b) ASW[10] (containing 10 mM SO) and its schematic representation illustrating the new chamber (n) formation and the surrounding gangue (algal cyst) constituted by the foraminifer by the accumulation of foreign detritus and other materials, confining the new chamber in formation in a microenvironment. In the case of rotaliid foraminifera, the formation of a new chamber begins with the isolation of the chamber volume from the surrounding environment by a structure which probably forms the organic scaffolding that shapes the morphology of the chamber and serves as a template for the calcification of the wall (Bé et al., 1979; De Nooijer et al., 2014; Nagai et al., 2018). Precipitation of calcium carbonate takes place on both sides of an organic layer, called primary organic sheet (POS, Erez, 2003), sandwiched between the outer and inner organic layers. Scale bar: 100 µm.
The number of accumulated individuals can trace the population size dynamics for each medium and depends on reproduction rate, number of juveniles produced by the individual and mortality. However, while the increase in the number of individuals clearly shows that living cells are being produced, no certainty about their viability can be drawn when the number of individuals stagnates or decreases, as no vital staining has been performed. It was therefore not always easy to distinguish between inactive and dead cells. We inferred mortality of foraminifera still adhering to the Petri dishes from the cessation of reticulopodial activity and cytoplasmic streaming, as well as from the change in cell color (Bernhard, 2000). In the media with no sulfate or sulfate concentrations above 60 mM, we observed little to no reproduction, cell inactivity and probably mortality. As a result, the number of attached foraminifera remained constant and/or decreased over time (Fig. 5, Table 1). The most dramatic reactions were observed within a few hours in the media with highest [SO] (ASW[120] and ASW[180]), where individuals neither reproduced nor even showed any reticulopodial activity. In ASW[90] and ASW[1], only one reproduction cycle was observed and after few days all the cells were inactive (Fig. 5, Table 1). Overall, the highest numbers of individuals at the end of the experiment were obtained in the ASW[28], NSW (Banyuls), ASW[5], ASW[10] and ASW[35] media (Fig. 5). Two media configurations, ASW[10] and ASW[28], from set 1 experiments were replicated in set 2 experiments. If the abundances for condition ASW[10] are of the same order of magnitude in both sets, the abundances for condition ASW[28] are much lower in set 2 compared to set 1. This was related to the reproduction rate in set 2, which slowed down drastically after 15 d. This decrease can be explained by a microbial bloom in the media that was observed in no other media (Appendix C, Fig. C1). The microbial spread could not be reduced by the weekly water change, and any transfer and rinsing of foraminifera or antibiotic treatment would have constituted an additional experimental modification. We thus kept counting foraminifera and sampling seawater but took into account neither pH and DIC value nor foraminifera counts measured in that media after day 15.
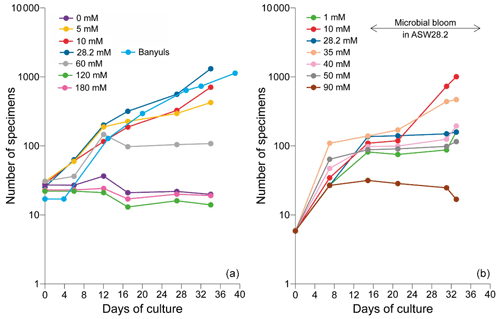
Figure 5Evolution of the number of the attached specimens of the For1C1strain in each culture medium at different [SO] for (a) set 1 and (b) set 2. The number of foraminifera counted (with a precision of ± 3 individual) corresponds to the living ones adhering to the bottom of the Petri dish before the change of medium. The increase in the number of individuals is due to asexual multiplication (see Appendix A). In set 1, the largest population in terms of size occurs for 28.2 mM (ASW[28] and NSW Banyuls). In set 2, a microbial bloom occurred after 12 d in medium ASW[28], likely affecting the reproduction rate (Appendix C, Fig. C1). The y axes are on log scales.
3.2 pH and DIC evolution
pH variations remained within ± 0.3 pH units during each experiment (Table 2).
pH drifted from the starting point between 8.1 and 8.2 towards more acidic values (7.83 minimum) and was reset close to 8.2 at each medium change for the first 15 d and then remained rather stable with values varying between 8.19 and 8.07. DIC ranged from 3.2± 0.2 mM (2σ) to 4.2± 0.3 mM (2σ) (Table 3). These concentrations are higher than the theoretical initial concentration of 2.8 mM using the recipe of Kester et al. (1967). While in Kester et al.'s recipe the targeted 8.2 pH is achieved after 2 h equilibration with the CO2 in the atmosphere, we had to proceed to NaOH addition despite a similar equilibration time. It is possible that higher CO2 dissolution at the atmospheric pressure of the year we performed the experiments (407 ppm in the atmosphere and probably more in the lab against 322 ppm in 1967) led to an increase in DIC. In addition, DIC probably built up in the Petri dishes each week as the foraminifera respire. pH and DIC variations for cultures in ASW[28] and ASW[10] are shown in Fig. 6.
Table 2pH values measured in media all along set 1 and set 2 experiments.
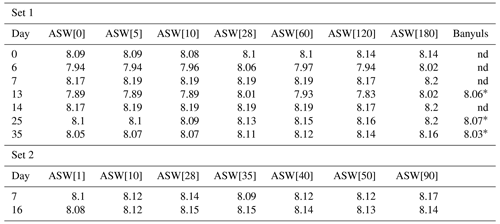
* Foraminiferal culture in seawater from Banyuls started with a delay, making the pH measurement on days 12, 22 and 26. nd: no data.
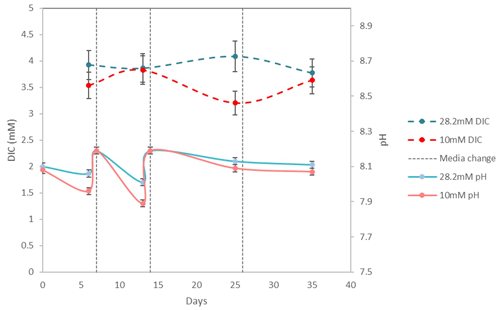
Figure 6Evolution of pH and DIC over 35 d of For1C1 culture at 10 and 28.2 mM during set 2, with a change of medium on days 7, 14 and 26. Measured DIC in all experiments (Table 3) varies between 3.2 and 4.1 mM; it decreases by calcite formation and increases by specimen respiration. The variation of pH in all experiments when measured (Table 2) is between 7.89 and 8.19. The decrease in pH is caused by both respiration (CO2 production) and calcite precipitation.
3.3 CAS concentration
CAS concentration in foraminiferal calcite was performed for the media ASW[5], ASW[10], ASW[28], ASW[35], ASW[40] and ASW[60], as the other samples were lost during the manipulations or were below the detection limits. The obtained values are presented in Fig. 7 and Table 4. Each data point was obtained using hundred to several hundreds of foraminifera for each medium. CAS concentration (sulfate to calcite ratio) increased from 3320 ppm to ∼ 14 000 ppm SO CaCO3 (± 5 %, 2σ) in proportion to total SO concentrations in artificial seawater, which increased from 5 to 28 mM. Above the modern seawater concentration of 28 mM, the foraminiferal CAS concentration no longer covaries with seawater and remains on a plateau between seawater [SO] 28 and 60 mM (Fig. 7). This suggests that a threshold (∼ 14 000 ppm) is probably reached at about 28 mM [SO] in seawater. Because we only have a natural seawater replicate for the sulfate concentration of modern seawater (28 mM), we observe a scatter at 28 mM that makes it difficult to determine precisely when the plateau starts. The slight decrease in foraminiferal CAS to 9740 ppm at ASW[60] is actually part of the variability of CAS values at 28 mM [SO] in seawater (Fig. 7 and Table 4). The foraminiferal CAS values from the ASW60 configuration can therefore be considered as part of a plateau (Fig. 7).
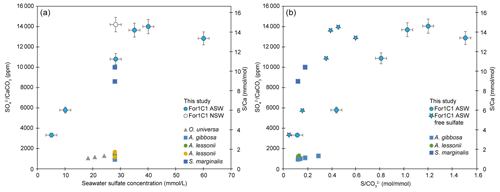
Figure 7Left panel: SO CaCO3 and ratios on tests of the For1C1 strain at the end of set 1 and set 2 experiments, as a function of seawater [SO] (5, 10, 28.2, 35, 40 and 60 mM). Right panel: SO CaCO3 and ratios on tests of the For1C1 strain at the end of set 1 and set 2 experiments, as a function of seawater S CO . For our experimental results, we report the values using both S as the sum of free and complexed sulfate based on our model results (circles), and as only free sulfate (stars). Each measurement has been performed on a pool of a hundred to several hundreds of specimens. Values are compared to other culture experiments of foraminifera targeting specifically the CAS content of the tests (Paris et al., 2014; van Dijk et al., 2017, 2019) and, when available, S CO as well. See Appendix B, Table B2 for details.
3.4 Sulfur isotopic composition
The δ34S values of the foraminiferal CAS from the different media are plotted in Fig. 8 and listed in Table 4. Measurements were performed both on foraminiferal samples from the C1Tg and For1C1 strains cultured in NSW or ASW[28] during the acclimation period and in those coming from the [SO] variation experiment (For1C1strain only), from a selection of culture media in which [SO] varied from 5 to 60 mM (Table 4). NSW δ34S composition was measured before (21.1 ± 0.2 ‰) and 7 d after adding the algae (19.9 ± 0.2 ‰). There was a difference beyond error bars between the two values. Considering that algae δ34S composition is 7.0 ± 0.2 ‰, the difference may be explained by the isotopically depleted sulfate added, resulting in algae decomposition, lowering the average δ34S of the media. This effect was not detectable in ASW possibly because the δ34S values of medium (9.1 ± 0.2 ‰ for ASW[28] and 0.1 ± 0.2 ‰ for ASW[5], ASW[10], ASW[35], ASW[40] and ASW[60]) were closer to that of the algae (Appendix B, Table B1). Considering that algae were added at each water change and degraded within 1 or 2 d, and that foraminifera entered into a chamber formation sequence after feeding (Fig. 4), we consider that the seawater δ34S that prevailed during chamber formation is the value measured after several days of culture with algae, 19.9 ± 0.2 ‰ in NSW and 9.1 ± 0.2 ‰ or 0.1± 0.2 ‰ in ASW[28] (Appendix B Table B1). A δ34SCAS−δ34Ssw fractionation value of 1.6 ± 0.3 ‰ was observed for For1C1 pool (1 SD, 8 samples in total coming from all [SO] concentrations), while it was 1.4 ± 0.2 ‰ for C1Tg specimens (1 SD, 5 samples in total coming from NSW or ASW[28]), which is indistinguishable within the error range (Fig. 8).
Table 4Sulfate concentration and isotopic composition measured in the foraminiferal calcite.
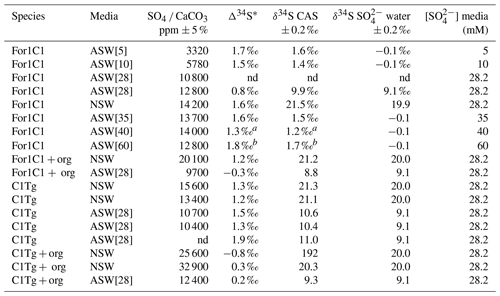
* Δ34S = δ34S CAS − δ34S SO water. a The 2 SD value of this sample is estimated to be 0.25 ‰. b The 2 SD value of this sample is estimated to be 0.35 ‰. nd: no data.
Samples for which organic matter was preserved yielded δ34S values of 1.1 ± 0.2 ‰ (For1C1 in ASW[28]), 0.4 ± 0.2 ‰ (For 1 C1 in NSW), 1.4 ± 0.2 ‰ (C1Tg in NSW) and 0.5 ± 0.2 ‰ (C1Tg in ASW[28]) lower than the value that was obtained for the For1C1 and C1Tg tests from which organic matter had been oxidatively removed (Table 4).
4.1 [SO] changes in seawater can affect foraminiferal biology
Our results highlight that a change in seawater [SO] concentration can affect foraminiferal cellular activity, reproduction and population size.
Reticulopodial activity stopped few hours after the transfer of individuals of the For1C1 strain from 28 mM of sulfate (ASW[28]) to concentrations above 120 mM (ASW[120] and ASW[180]) or without sulfate (ASW[0]). Dissolved sulfate and food were the only sources of sulfur in this experiment, which is essential for life. Since ASW[0] prevented any reproduction and induced cellular inactivity, we infer that sulfur from food appears insufficient and that dissolved sulfate in seawater is necessary for cellular activity in foraminifera. At the other extreme, toxic impact of the highest [SO] (ASW[120] and ASW[180]) can explain the non-reproduction and the cellular inactivity of individuals after a few hours. For1C1 individuals survived and even reproduced once in the ASW[1] and ASW[90] media (Fig. 5). Thus, our results suggest that foraminifera can reproduce and show pseudopodial activity only within a certain range of [SO], from 1 to 90 mM, extreme values at which the cellular activity is already very low. Individuals appear to tolerate these extreme conditions for only the first week and then cease all reproductive activity. They appear to be adapted, beyond the modern oceanic [SO] (28.2 mM), to a range of seawater [SO] from 5 to 35 mM, as shown by the high number of accumulated live individuals incremented by reproduction at the end of set 1 and set 2 experiments (Fig. 5). As already mentioned, the low number of individuals at the end of the second set of experiments is due to a bacterial proliferation after 3 weeks. Population sizes are growing less fast above [SO] of 35 mM and below 5 mM, suggesting a foraminiferal reproduction sensitivity to [SO] variations.
The effect of changes in seawater [SO] on foraminiferal reproduction highlights a possible mechanism by which changes in the composition of seawater can affect the carbonate record. It has previously been hypothesized that seawater ratio and SO Ca ratio control the switch between calcite and aragonite dominance in the sedimentary record, as a high SO and Mg concentrations in seawater inhibit calcite precipitation and promote aragonite precipitation, as shown by inorganic precipitation experiments (Bots et al., 2011; Barkan et al., 2020). Here, we show that a change in seawater [SO] may also affect foraminiferal reproduction, population size and hence their calcitic test accumulation in the sediment. However, this appears to be for seawater [SO] variations far below and above the range thought to be involved in long-term secular variations in the Phanerozoic (∼ 5–28 mM), suggesting an adaptation of foraminifera in this range of variations. Indeed, under conditions that mimic the Phanerozoic range of [SO] variations, reproduction and population growth appear to be unaffected.
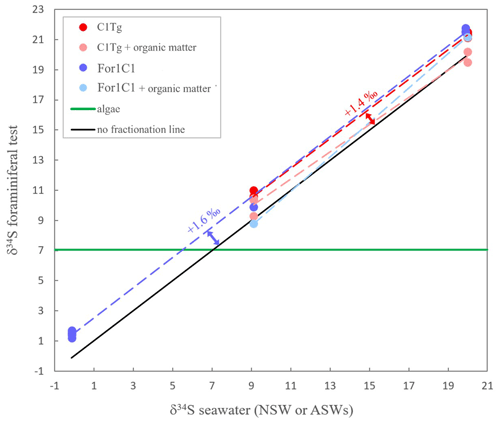
Figure 8δ34S in foraminiferal test (with or without organic matter) for the two strains For1C1 and C1Tg. The two strains were cultured in several media (For1C1 in ASW[5], ASW[10] ASW[28], ASW[35], ASW[ 40], ASW[60] and NSW (Banyuls), C1Tg in NSW (Concarneau) and ASW[28]) whose δ34S has been also measured. The δ34S values of the media depend on the salts used to make the solution. The green line corresponds to the δ34S composition of the algae that were fed to the foraminifera and whose isotopic composition remains stable; 2σ error bars (± 0.2 ‰ to ± 0.3 ‰) are smaller than symbols.
4.2 Foraminifer CAS concentration versus seawater [SO] or S CO
Our cultured foraminifera contain high levels of sulfates, similar to high-Mg calcite foraminifera previously grown during culture experiments and significantly higher than low-Mg calcite foraminifera (Paris et al., 2014; van Dijk et al., 2017, 2019) (Fig. 7, Appendix B, Table B2). Similar to previous results of foraminiferal culture experiments comparing CAS content with seawater sulfate concentration, we note an increase in foraminiferal CAS content with seawater sulfate concentration increase. More specifically, our results show that CAS concentration in foraminiferal calcite grown in experimental seawater increases with seawater [SO] concentration from 5 to 28 mM (Fig. 7), similarly to what is observed in inorganic carbonates (Busenberg and Plummer, 1985; Fernández-Diaz et al., 2010; Barkan et al., 2020) or previous foraminiferal investigation (Paris et al., 2014). At [SO] higher than 28 mM in seawater, the incorporation of sulfate in the foraminiferal calcite seems to reach a saturation point (Fig. 7). It is remarkable to note that foraminifera can reproduce and thus calcify at [SO] as high as 90 mM (Fig. 5), concentrations at which no inorganic calcite precipitation occurs (Bots et al., 2011; Barkan et al., 2020). However as discussed before, their reproduction is limited to the first week, which strongly suggests that they could only tolerate brief exposure to such a high level of sulfates in their environment.
A geochemical modeling of experiments in which CAS, pH and DIC were measured is available in Appendix D and permitted us to extract CO concentrations. Overall, we observe an increase and a plateau, whether we compare our CAS content to seawater sulfate concentration or S CO ratios. When we replace total sulfate (the sum of free SO and its major complexed forms (NaSO4, CaSO4 and MgSO4) by only free sulfate), the linearity of the 5 to 28 mM CAS accumulation trend is maintained. However, the plateau from 28 to 60 mM is less visible, potentially evidencing the role of complexes formation in the lower SO incorporation in the tests. As shown in the Appendix, the sole formation of complexes cannot explain the plateau observed in Fig. 7.
To understand this evolution of sulfate content, we must first describe where sulfur is located in the test. Two options are possible:
- i.
CAS. Sulfate is incorporated into both inorganic and biogenic CaCO3 minerals as CAS within the growing mineral structure, the larger tetrahedral sulfate substituting for the smaller trigonal–planar carbonate ion (Busenberg and Plummer, 1985; Kontrec et al. 2004; Balan et al., 2014; Tamenori et al., 2014; Perrin et al., 2017; Tamenori and Yoshimura, 2018).
- ii.
Sorg. Sulfur is present in the organic matrix used by biomineralizing organisms to initiate calcification and orient the growing crystals (e.g., Cuif et al., 2004; Richardson et al., 2019; de Nooijer et al., 2014). The organic matrix of the test of a wide variety of foraminiferal taxa contains over-sulfated glycosaminoglycans and proteins (Weiner and Erez, 1984; Langer, 1992). The benthic foraminifera Rosalinidae belong to the order Rotaliida and likely share the same mechanisms of biomineralization and bilayer test construction as other rotaliid families. In the case of the rotaliid test, the calcareous wall growth of each new chamber results from the bioprecipitation of two calcite layers, on either side of an organic matrix (Bé et al., 1979; de Nooijer et al., 2014; Nagai et al., 2018) referred to as the primary organic sheet (POS, Erez, 2003). However, since we applied an oxidative cleaning to the foraminiferal tests to destroy the organic matter, we assume that most of the measured [SO] in the tests are linked to the CAS concentration, although a small contribution might be still associated with Sorg within the biomineralized calcite (Burdett et al., 1989; Cuif et al., 2003; Paris et al., 2014). In the following discussion, we will thus assume that our measured sulfate content reflects structurally bound CAS.
Several hypotheses can be formulated to explain this SO CaCO3 incorporation pattern:
- i.
Foraminifera may be able to regulate [SO] at the site of calcification (SOC) during calcite precipitation through active transmembrane transport, removing excess sulfate and lowering it in the precipitating fluid, enabling calcite nucleation and precipitation, as sulfate in high concentration inhibits calcite precipitation and makes it more soluble (Busenberg and Plummer, 1985; Bots et al., 2011; Barkan et al., 2020). In fact, under our experimental conditions the amount of CAS incorporated in foraminiferal calcite correlates with seawater SO concentration, from 5 up to a plateau that starts at 28 mM. The mere fact that calcite precipitates therefore suggests that sulfate is at least partially removed from the precipitating fluid, altering the local SO concentration. The correlation suggests that this removal is partial and, to some extent, proportional to the concentration of SO in seawater.
- ii.
An increase in the carbonate ion concentration may help maintain a constant SO CO. Previous investigations demonstrated that it is more appropriate to reason in terms of SO CO ratio of the calcifying fluid rather than [SO] as sulfate substitutes for CO in the forming mineral (van Dijk et al., 2019; Barkan et al., 2020). Another way to maintain SO CO constant while [SO] increases would be to proportionally increase CO. Like other calcifying organisms, benthic foraminifera modify the pH of the precipitating fluid to promote calcite formation (Erez, 2003; de Nooijer et al., 2009; Rollion-Bard and Erez, 2010; Toyofuku et al., 2017). Foraminifera most probably actively pump protons out of the SOC (Sabbatini et al., 2014; Toyofuku et al., 2017). Intensifying this process in the case of elevated [SO] would induce an increase in carbonate ion concentration (and the saturation state) and could therefore help to maintain a constant SO CO when [SO] reaches values between 28 and 90 mM, allowing calcite bio-precipitation. This mechanism, like that of active sulfate transmembrane transport mentioned above, would cease to function at seawater [SO] levels neighboring 120 mM, when the foraminiferal cells become inactive.
- iii.
A preferential sequestering of sulfate in some organic-rich layers at the incipient phase of biocalcification might allow the decrease of the [SO] in the remaining liquid and thereby prevent further sulfate incorporation into foraminiferal calcite above 28 mM [SO] in seawater. High-resolution sulfur nano-mapping on transversal section of perforate foraminiferal tests (such as Rosalinidae or Orbulina) showed a banded heterogeneity in sulfur distribution across the multi-layer structure (Paris et al., 2014; van Dijk et al., 2019). XRF intra-test mapping revealed a preferential incorporation of metals and sulfur in the POS zone, the organic incipient stage of the buildup of the wall of a new chamber of test (Lemelle et al., 2020). In our case, organic matter has been oxidized, and most of the “stored” SO was likely removed.
- iv.
A kinetic effect could also explain the non-linearity of the CAS concentration in foraminiferal tests with corresponding increases in [SO] above 28 mM, as inorganic calcite precipitation experiments suggest a reduction in crystal growth rates at higher [SO] (Busenberg and Plummer, 1985). However, it is worth noting that a decrease in precipitation rate can also be associated with a lower CAS content in inorganic calcite (Barkan et al., 2020). As a result, one could imagine that the change in sulfate concentration reflects a change in precipitation rate induced by different sulfate concentration in seawater and/or in the biomineralizing fluid. However, as calcite is more soluble and precipitates less easily at high sulfate concentration, we would expect an opposite effect to what we observe in the 5–40 mM part of our results. There could nonetheless be a contribution of the precipitation rate effect to the plateau we observe.
- v.
Finally, another possibility to explain why the CAS in foraminiferal tests does not increase linearly with a corresponding increase in the [SO] beyond 28 Mm could be that, at such concentrations in solution, sulfate might complex more easily with other cations such as Ca2+, K+, Mg2+, Na+, Sr2+, etc. (Garrels and Thompson, 1962). Such complexes cannot be effectively incorporated into the mineral lattice structure. This might influence the amount of SO substituted in carbonates and thus the CAS in foraminiferal tests. A geochemical model, available in Appendix D, taking into account our media configurations where we had CAS, pH and DIC data, shows that the model CAS concentration follows the seawater SO CO and CaSO4 CaHCO3 concentrations, which in turn depend mainly on the [SO] in the solution, with a slowed increase between 40 and 60 mM, likely related to the formation of complexes. However, the sole formation of complexes cannot explain the plateau observed beyond 28 mM.
The putative mechanisms for sulfate regulation could have been adopted by foraminifera as evolutionary strategies to maintain carbonate precipitation despite potential variation in [SO]. Indeed, at [SO] greater than 8 mM, abiotic calcite nucleation and precipitation is inhibited, and aragonite precipitates from saturated solutions (Kitano and Hood, 1962; Kitano et al., 1975; Bots et al., 2011). This inhibition is also true in the lack in magnesium (Barkan et al., 2020), and thus sulfate alone can affect calcite precipitation. Mechanisms such as increasing calcium concentration, pH and/or saturation state (e.g., Zeebe and Sanyal, 2002; Nehrke et al., 2013; Evans et al., 2018), as well as the presence of organics, could help overcome such a high concentration of sulfate. However, when it comes to magnesium, active removal is also an option (Bentov and Erez, 2006). Calcitic foraminifera, which first appeared during the Devonian (Vachard et al., 2010) in a range of low-sulfate seawaters of ∼ 3–15 mM (Algeo et al., 2015), might have progressively adopted such strategies in order to precipitate calcite in high-sulfate (∼ 28 mM) seawaters such as those in the present-day ocean and preserve the capacity to precipitate calcite at concentrations reaching 90 mM as evidenced here. However, in addition to active biological control to remove sulfate from the calcification site, a reduction in sulfate uptake in the tests at high seawater sulfate concentrations (> 28 mM) is likely to be due to the formation of sulfate complexes with other cations, explaining also the non-linearity of the CAS concentration in foraminiferal tests at high increases in [SO].
4.3 Sulfur isotope fractionation
The isotopic composition of CAS remains constant through our experiments. Sulfur isotopic fractionation of CAS in benthic foraminifera (Rosalinidae) is not sensitive to the variation in [SO] in seawater (Fig. 8), thus confirming the earlier observation on planktic foraminifera by Paris et al. (2014). This result by itself is important and confirms that foraminiferal CAS constitutes a reliable proxy of seawater δ34S. This result, together with the correlation between SO4 CaCO3 and seawater [SO] (Fig. 7), supports that CAS in foraminiferal tests is of inorganic origin.
More importantly, the fractionation observed here is clearly different from the inorganic fractionation measured in the inorganic calcite (Barkan et al., 2020), highlighting the involvement of some biological isotopic fractionation. Considering that the algae's organic sulfur source had a fixed sulfur composition (7 ‰) while the seawater δ34S varied from one medium to the other (from −0.1 ‰ to 20.0 ‰), our isotopic measurements on Sorg+ CAS allow one to infer the origin of Sorg as well. Mass balance calculation permits one to determine that the isotopic composition of Sorg varies with seawater δ34S value, pointing towards mainly an inorganic source for Sorg (Fig. 8). This is consistent with our observation that no cellular activity of foraminifera was possible in medium with zero [SO], even in the presence of algae as food and a possible source of Sorg. The δ34S value of the combined S pool (Sorg+ CAS) is 0.4 ‰ to 1.4 ‰ more negative than the δ34S value of CAS alone, which points towards the involvement of some biological fractionation or vital effect associated with the incorporation of sulfur.
4.4 Implication for paleoenvironmental reconstructions
Sulfur isotopic composition in the sedimentary record, through sulfur species redox reactivity and multiple deposition form, records several paleoenvironmental processes occurring in the atmosphere and the ocean (Farquhar et al., 2000; Farquhar and Wing, 2003; Crockford et al., 2019). This makes sulfur one of the most studied elements for the surface processes. And yet, in order to investigate the sulfur cycle, it is necessary to interrogate different sedimentary archives: carbonates, barites, evaporites and pyrites (Paytan et al., 1998; Algeo et al., 2015; Halevy et al., 2012; Present et al., 2020) to reconstruct variations in both δ34S and [SO]. The work to match the sedimentary record of both δ34S and [SO] is laborious and requires calibrations. Our results show that benthic foraminifera (Rosalinidae) incorporate CAS in their test proportionally to the [SO] in seawater, at least in the 5–28 mmol L−1 range, confirming previous experiments on planktic foraminifers that foraminiferal CAS can serve as a proxy for variations of both δ34SCAS and [SO] in seawater (Paris et al., 2014). However, they also highlight that, above the seawater [SO] of 28 mM, it might not be possible to confidently determine the seawater [SO] using foraminiferal CAS, as the previous linear correlation no longer holds. This limitation means that foraminiferal CAS could be used to trace deep time secular changes in seawater [SO], which varies from about 5 to 28 mM today (Algeo et al., 2015), but probably not to trace past seawater [SO] enrichments above 28 mM, such as those that could be caused by important volcanic eruptions or sulfate-rich volcanic hydrothermal fluids on the seafloor. Future studies are therefore important to confirm whether or not the seawater [SO] threshold of 28 mM for CAS incorporation can be applied to other benthic and planktonic foraminifera or whether it is restricted to Rosalinidae.
The use of CAS concentration as a marine [SO] record is still promising, despite the limitation discussed above, but will require calibration on various types of carbonates and species that may each have their own fractionation factor. The preservation of that dual δ34S [SO] in foraminiferal calcite has to be evaluated in the carbonate record, as diagenesis has the capacity to affect [SO] in carbonates (e.g., Gill et al., 2008; Marenco et al., 2008; Rennie and Turchyn, 2014).
Additionally, it has been previously supported that can work as a proxy for CO concentration (van Dijk et al., 2017). Our results complement this finding under the condition that it is applied on timescales where seawater [SO] is constant.
The other major implication of our results for the interpretation of the geological record is that changes in seawater [SO] could affect the production of carbonate by affecting the reproduction/survival of at least some calcifying organisms, as the benthic foraminifera studied in this work. In theory, the increase in seawater [SO] is expected to have a purely “abiotic” effect on calcite production as sulfate thermodynamically inhibits calcite formation and makes calcite more soluble. As a result, higher sulfate content in the living medium would generate a decrease in calcification intensity for a given individual. In this experiment we showed that [SO] higher in the medium than those of the modern ocean can also decrease the amount of accumulated calcite by affecting foraminiferal population size, suggesting that their biological activity is harmed by such sulfate concentrations. As a result, the decrease the total amount of calcification would be explained partly by a decrease in biological activity. This work illustrated how variations in seawater composition can have a dual effect on biomineralizing organisms. Conditions that inhibit calcite formation, such as increases in marine concentrations of Mg2+ or SO, could have chemical “abiotic” effects on carbonate formation but could also affect biological processes involved in biomineralization.
We cultured rotaliid foraminifera in media with [SO] spanning from 0 to 180 mM, stable salinity and fixed seawater δ34S. [SO] changes in seawater affected foraminiferal reproduction, population size and hence test accumulation. Foraminifera kept precipitating calcite in media reaching [SO] = 90 mM but very temporarily. Sulfate from seawater is necessary for the cellular activity of foraminifera, but at concentrations equal and above 90 mM it becomes toxic to them, as evidenced by cellular inactivity and reproductive arrest. Sulfur concentration in CAS varied proportionally to seawater [SO] between 5 and 28 mM and then stabilizes. Our results highlight that isotope fractionation between CAS and seawater does not depend on seawater [SO]. Overall, similarly to planktic foraminifera the δ34SCAS value of a given species of benthic foraminifera is a reliable way to reconstruct seawater δ34S, despite variations of [SO] in seawater.
The two selected strains come from two distinct locations, from Banyuls (Mediterranean Sea) and Concarneau (Atlantic Ocean). Morphologically they may be related to the family Rosalinidae (Holzmann and Pawlowski, 2017). They are attached forms with a low trochospiral hyaline calcitic perforate test, with a peripherical low arch aperture on the umbilical side bordered by lips (Fig. 2). Chamber interior is simple (Fig. 2). Two morphotypes are noticeable in both the strains (Fig. 2). Individuals usually reproducing asexually after every 12–15 d when their test reaches a development of 11–12 chambers (Fig. 3) are morphologically very close to the genus Rosalina (Fig. 2). Individuals who lived for several weeks adding more than 12 chambers have the last chambers with an annular arrangement (Fig. 2).
Individuals with a Rosalina-like morphology (Fig. 2) probably belong to the schizont generation of their trimorphic life cycle (alternating gamont–agamont–schizont–gamont generations), documented for example in Planorbulina mediterraneensis and a few dozen other species (Le Calvez, 1938; Dettmering et al., 1998). More precisely, they are diploid megalospheric schizonts that have entered a cycle of successive asexual reproduction (apogamic cycle) (Fig. 3), during which the new generation of schizonts is produced by schizogony, i.e., by multiple fission of a multinucleate parental cytoplasm (Le Calvez, 1938; Dettmering et al., 1998). For this reason, it is not obvious to identify them morphologically at the species level because the morphology of the diploid agamont microspheric and/or of the haploid megalospheric gamont parent generation, on which the description of the species has often been made, is unknown to the best of our knowledge. For now, we leave these forms in open nomenclature and call them by the name of the strains For1C1 and C1Tg. Adult specimens of these strains are smaller than the traditional foraminifer fraction obtained after sieving (through > 125 µm mesh) in geochemical studies. They thus may be common but rarely collected because of their size.
Table B1Sulfur isotope composition of media and algae cells.
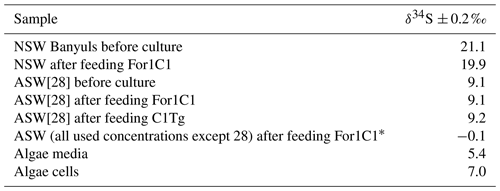
* Different salts were used to make all ASW and ASW28.
We designed set 2 to replicate the ASW[28] and ASW[10] results as well as to extend the range of concentrations and ran it right after set 1 (see methods for more details). Two differences can be observed. First the reproduction rate is significantly higher in the ASW[10] media of set 2 than it was in set 1 even though the media were identical (Fig. 5). However, it might be related to the starting number of foraminifera for each experiment. In set 2 we started each culture experiment with 6 foraminifera individuals (instead of 28 as in set 1); all 6 individuals were chosen more carefully, which could induce a bias and explain a more active behavior during experiment 2. If it were the case, the bias would nonetheless be systematic and similar for each medium (all Petri dishes in set 2 started with 6 individuals) and thus does not prevent comparison of results within set 2.
The second difference is observed in the ASW[28] medium in set 2. The reproduction rate, which was the highest observed, slowed down drastically after 15 d. This decrease can be explained by a microbial bloom in the media that was observed in no other media (Fig. C1). The microbial spread could not be reduced by the weekly water change, and any transfer and rinsing of foraminifera or antibiotic treatment would have constituted an additional experimental modification. We thus kept counting foraminifera and sampling seawater but did not take into account any results collected in that media after day 15.
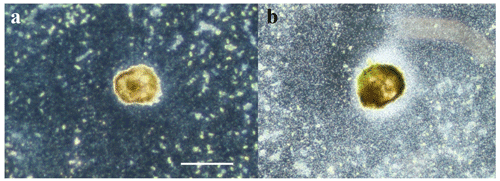
Figure C1Optical microscopy imaging in dark field. The foraminifera are observed from below. the background appears black, algae greenish, and bacterial contamination cloudy white. (a) Foraminifera in ASW[10] during experiment 2 where microbial spread stays limited. (b) Foraminifera in ASW[28] during experiment 2 where microbial bloom was uncontrolled after 15 d and could not be reduced. Scale bar: 100 µm.
D1 Geochemical modeling parameters
The relative abundances of Ca2+, SO, CaSO4(d), MgSO4(d), NaSO CaHCO, CO, HCO and CO2(d) in a solution were computed with the geochemical code JCHESS (Van der Lee, 1998). ASW composition from Kester (1967) and experimental temperature (20 ∘C) were chosen as input parameters assuming a closed system with no gas–solution exchange, and Cl, Na and SO were modified as they were in each media of the experiment (base input in the additional Excel Tables S1 to S6). All DIC is provided as HCO to the model.
Given that pH was adjusted by NaOH addition, this was reproduced in the model, by adding Na+ and OH− (in the same concentration) until reaching pH 8.2.
From this starting point, HCO was adjusted to the measured DIC value, and OH− was adjusted to match the measured pH value (adjusted input and measured values in the additional Excel table). No additional Na+ was added despite the slight electrical imbalance generated, as Na+ can form complexes with SO, and no Na+ was provided to the media after pH has been adjusted.
The output data considered are Ca2+, all free and complexed SO species, free DIC species and CaHCO. The sole DIC species present as a complex that was extracted is CaHCO, because it is the major complex. It is also a species that has been hypothesized to be potentially incorporated into calcite, as CaSO4 could be.
D2 Geochemical modeling results
The SO CO concentration increases linearly to a slight inflection point at 60 mM, linked to complex formation. Nevertheless, we do not observe a plateau from 28 mM onwards, which could have explained a constant incorporation of SO in calcite beyond 28 mM. Similarly, assuming that SO incorporation into calcite takes place from CaSO4, although an inflection of the CaSO4 CaHCO3 ratio is observable from 40 mM, no plateau is observed. These results show that the incorporation of sulfate into the calcite of the foraminifers in our experiments, which plateau above 28 mM, cannot be explained by the formation of complexes in seawater. Alternatively, CAS concentration is not a good recorder of either the SO CO ratio or the CaSO4 CaHCO3 ratio above a concentration of 28 mM.
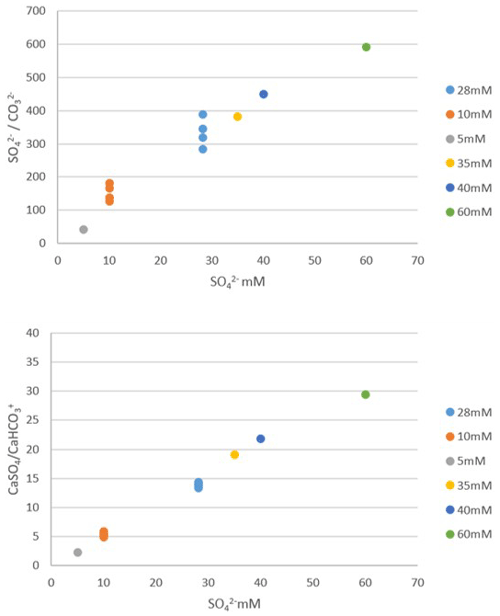
Figure D1Geochemical modeling results showing SO CO ratio and CaSO4 CaHCO3 ratio in a solution as a function of total sulfate concentration in a solution (dot colors indicate each sulfate concentration). Each point corresponds to a different computational run, for samples where DIC and pH were measured and were used as constraints to the model. Both ratios increase linearly to a slight inflection point at 60 mM, but no plateau is seen between 28 and 60 mM.
JChess code is available for download at the following website: https://chess.geosciences.mines-paristech.fr/presentation-1 (van der Lee, 1998).
All data are available in the article and its Supplement.
The supplement related to this article is available online at: https://doi.org/10.5194/bg-20-5177-2023-supplement.
All authors participated in designing and interpreting the experiments. CT carried out culture experiments under MD and AB supervision and technical support from AL. MD isolated the strains and developed the cultivation protocol. GP performed all isotopic and SO4 CaCO3 analyses. CT designed the figures and wrote the paper with contributions from all co-authors.
The contact author has declared that none of the authors has any competing interests.
Publisher’s note: Copernicus Publications remains neutral with regard to jurisdictional claims made in the text, published maps, institutional affiliations, or any other geographical representation in this paper. While Copernicus Publications makes every effort to include appropriate place names, the final responsibility lies with the authors.
The authors thank the reviewers – Julien Richirt and David Evans – for their very constructive criticism, which helped to improve the article.
This research was funded by the LabEx BCDiv project “SULFOR, Impact of SO2 on ocean acidification and foraminiferal biocalcification” (PI Annachiara Bartolini) and the CNRS INSU INTERRVIE project “Impact of sulfate variations on the biocalcification of foraminifera” (PI Annachiara Bartolini). Sylvain Pont (MNHN, IMPMC UMR 7590, France) produced the SEM imaging.
This paper was edited by Chiara Borrelli and reviewed by David Evans and Julien Richirt.
Algeo, T. J., Luo, G. M., Song, H. Y., Lyons, T. W., and Canfield, D. E.: Reconstruction of secular variation in seawater sulfate concentrations, Biogeosciences, 12, 2131–2151, https://doi.org/10.5194/bg-12-2131-2015, 2015.
Assayag, N., Rivé, K., Ader, M., Jézéquel, D., and Agrinier, P.: Improved method for isotopic and quantitative analysis of dissolved inorganic carbon in natural water samples, Rapid Commun. Mass Sp., 20, 2243–2251, 2006.
Balan, E., Blanchard, M., Pinilla, C., and Lazzeri, M.: First-principles modeling of sulfate incorporation and 34S 32S isotopic fractionation in different calcium carbonates, Chem. Geol., 374/375, 84–91, https://doi.org/10.1016/j.chemgeo.2014.03.004, 2014.
Barkan, Y., Paris, G., Webb, S. M., Adkins, J. F., and Halevy, I.: Sulfur isotope fractionation between aqueous and carbonate-associated sulfate in abiotic calcite and aragonite, Geochim. Cosmochim. Ac., 280, 317–339, https://doi.org/10.1016/j.gca.2020.03.022, 2020.
Bé, A. W., Hemleben, C., Anderson, O. R., and Spindler, M.: Chamber formation in planktonic foraminifera, Micropaleontology, 25, 294–307, https://doi.org/10.2307/1485304, 1979.
Bentov, S. and Erez, J.: Impact of biomineralization processes on the Mg content of foraminiferal shells: A biological perspective, Geochem. Geophy. Geosy., 7, Q01P08, https://doi.org/10.1029/2005gc001015, 2006.
Bernhard, J. M.: Distinguishing live from dead foraminifera: Methods review and proper applications, Micropaleontology , 46, 38–46, 2000.
Bots, P., Benning, L., Rickaby, R., and Shaw, S.: The role of SO4 in the switch from calcite to aragonite seas, Geology, 39, 331–334, https://doi.org/10.1130/G31619.1, 2011.
Brennan, S. T., Lowenstein, T. K., and Horita, J.: Seawater chemistry and the advent of biocalcification, Geology, 32, 473–476, https://doi.org/10.1130/G20251.1, 2004.
Burdett, J. W., Arthur, M. A., and Richardson, M.: A Neogene seawater sulfur isotope age curve from calcareous pelagic microfossils, Earth Planet. Sc. Lett., 94, 189–198, https://doi.org/10.1016/0012-821X(89)90138-6, 1989.
Busenberg, E. and Plummer, L. N : Kinetic and thermodynamic factors controlling the distribution of SO and Na+ in calcites and selected aragonites, Geochim. Cosmochim. Ac., 49, 713–725, https://doi.org/10.1016/0016-7037(85)90166-8, 1985.
Crockford, P. W., Kunzmann, M., Bekker, A., Hayles, J., Bao, H., Halverson, G. P, Peng, Y., Bui, T. H., Cox, G. M., Gibson, T. M., Wörndle, S., Rainbird, R., Lepland, A., Swanson-Hysell, N. L., Master, S., Sreenivas, B., Kuznetsov, A., Krupenik, V., and Wing, B. A.: Claypool continued: Extending the isotopic record of sedimentary sulfate, Chem. Geol., 513, 200–225, https://doi.org/10.1016/j.chemgeo.2019.02.030, 2019.
Cuif, J. P., Dauphin, Y., Doucet, J., Salome, M., and Susini, J.: XANES mapping of organic sulfate in three scleractinian coral skeletons, Geochim. Cosmochim. Ac., 67, 75–83, https://doi.org/10.1016/S0016-7037(02)01041-4, 2003.
Cuif, J. P., Dauphin, Y., Berthet, P., and Jegoudez, J.: Associated water and organic compounds in coral skeletons: quantitative thermogravimetry coupled to infrared absorption spectrometry, Geochem. Geophy. Geosy., 5, Q11011, https://doi.org/10.1029/2004GC000783, 2004.
de Nooijer, L. J., Toyofuku, T., and Kitazato, H: Foraminifera promote calcification by elevating their intracellular pH, P. Natl Acad. Sci. USA, 106, 15374–15378, 2009.
de Nooijer, L. J., Spero, H. J., Erez, J., Bijma, J., and Reichart, G. J: Biomineralization in perforate foraminifera, Earth-Sci. Rev., 135, 48–58, https://doi.org/10.1016/j.earscirev.2014.03.013, 2014.
Dettmering, C., Rottqer, R., Hoheneqqer, J., and Schrnaljohann, R.: The Trimorphic Life Cycle in Foraminifera: Observations from Cultures allow New Evaluation, Europ. J. Protistol., 34, 363–368, 1998.
Dissard, D., Nehrke, G., Reichart, G. J., and Bijma, J.: The impact of salinity on the and ratio in the benthic foraminifera Ammonia tepida: results from culture experiments, Geochim. Cosmochim. Ac., 74, 3–74, https://doi.org/10.1016/j.gca.2009.10.040, 2010a.
Dissard, D., Nehrke, G., Reichart, G. J., and Bijma, J.: Impact of seawater pCO2 on calcification and and ratios in benthic foraminifera calcite: results from culturing experiments with Ammonia tepida, Biogeosciences, 7, 81–93, https://doi.org/10.5194/bg-7-81-2010, 2010b.
Erez, J.: The source of ions for biomineralization in foraminifera and their implications for paleoceanographic proxies, in: Biomineralization, edited by: Dove, P. M., De Yoreo, J. J., and Weiner, S., De Gruyter, Berlin, Boston, Rev. Mineral. Geochem., 54, 115–149, https://doi.org/10.1515/9781501509346-010, 2003.
Evans, D., Müller, W., and Erez, J.: Assessing foraminifera biomineralisation models through trace element data of cultures under variable seawater chemistry, Geochim. Cosmochim. Ac., 236, 198–217, https://doi.org/10.1016/j.gca.2018.02.048, 2018.
Farquhar, J. and Wing, B. A.: Multiple sulfur isotopes and the evolution of the atmosphere, Earth Planet. Sc. Lett., 213, 1–13, https://doi.org/10.1016/S0012-821X(03)00296-6, 2003.
Farquhar, J., Bao, H. M., and Thiemens, M.: Atmospheric influence of Earth's earliest sulfur cycle, Science, 289, 756–759, https://doi.org/10.1126/science.289.5480.756, 2000.
Fernández-Díaz, L., Fernández-González, Á., and Prieto, M.: The role of sulfate groups in controlling CaCO3 polymorphism, Geochim. Cosmochim. Ac., 74, 6064–6076, https://doi.org/10.1016/j.gca.2010.08.010, 2010.
Gamo, T., Okamura, K., Charlou, J.-L., Urabe, T., Auzende, J.-M., Ishibashi, J., Shitashima, K., and Chiba, H.: Acidic and sulfate-rich hydrothermal fluids from the Manus back-arc basin, Papua New Guinea, Geology, 25, 139–142, 1997.
Garrels, R. M. and Thompson, M. E.: A chemical model for sea water at 25 ∘C and one atmosphere total pressure, Am. J. Science, 260, 57–66, https://doi.org/10.2475/ajs.260.1.57, 1962.
Gill, B. C., Lyons, T. W., and Frank, T. D.: Behavior of carbonate-associated sulfate during meteoric diagenesis and implications for the sulfur isotope paleoproxy, Geochim. Cosmochim. Ac., 72, 4699–4711, https://doi.org/10.1016/j.gca.2008.07.001, 2008.
Goetschl, K. E., Purgstaller, B., Dietzel, M., and Mavromatis, V.: Effect of sulfate on magnesium incorporation in low-magnesium calcite, Geochim. Cosmochim. Ac., 265, 505–519, https://doi.org/10.1016/j.gca.2019.07.024, 2019.
Halevy, I., Peters, S. E., and Fischer, W. W.: Sulfate burial constraints on the Phanerozoic sulfur cycle, Science, 337, 331–334, https://doi.org/10.1126/science.1220224, 2012.
Holzmann, M. and Pawlowski, J.: An updated classification of rotaliid foraminifera based on ribosomal DNA phylogeny, Mar. Micropaleontol., 132, 18–34, https://doi.org/10.1016/j.marmicro.2017.04.002, 2017.
Horita, J., Zimmermann, H., and Holland, H. D.: Chemical evolution of seawater during the Phanerozoic: Implications from the record of marine evaporites, Geochim. Cosmochim. Ac., 66, 3733–3756, 2002.
Kampschulte, A., Bruckschen, P., and Strauss, H.: The sulphur isotopic composition of trace sulphates in Carboniferous brachiopods: implications for coeval seawater, correlation with other geochemical cycles and isotope stratigraphy, Chem. Geol., 175, 149–173, https://doi.org/10.1016/S0009-2541(00)00367-3, 2001.
Kester, D. R., Duedall, I. W., Connors, D. N., and Pytkowicz, R. M.: Preparation of artificial seawater 1, Limnol. Oceanogr., 12, 176–179, https://doi.org/10.4319/lo.1967.12.1.0176, 1967.
Kitano, Y. and Hood, D. W.: Calcium carbonate crystal forms formed from sea water by inorganic processes, J. Oceanogr. Soc. Jpn., 18, 141–145, https://doi.org/10.5928/kaiyou1942.18.141, 1962.
Kitano, Y., Okumura, M., and Idogaki, M.: Incorporation of sodium, chloride and sulfate with calcium carbonate, Geochem. J., 9, 75–84, https://doi.org/10.2343/geochemj.9.75, 1975.
Kontrec, J., Kralj, D., Brečević, L., Falini, G., Fermani, S., Noethig-Laslo, V., and Mirosavljević, M.: Incorporation of Inorganic Anions in Calcite, EurJIC, 2004, 4579–4585, https://doi.org/10.1002/ejic.200400268, 2004.
Langer, M. R.: Biosynthesis of glycosaminoglycans in foraminifera: A review, Mar. Micropaleontol., 19, 245–255, 1992.
Langer, M. R.: Assessing the contribution of foraminiferan protists to global ocean carbonate production, J. Eukaryot. Microbiol., 55, 163–169, https://doi.org/10.1111/j.1550-7408.2008.00321.x, 2008.
Laakso, T. A., Waldeck, A., Macdonald, F. A., and Johnston, D.: Volcanic controls on seawater sulfate over the past 120 million years, P. Natl. Acad. Sci. USA, 117, 21118–21124, https://doi.org/10.1073/pnas.1921308117, 2020.
Le Calvcz, J.: Recherches sur les foraminifères, 1. Développernent et reproduction, Archives Zoologie Expérimentale et Génerale, 80, 163–333, 1938.
Le Houedec, S., Erez, J., and Rosenthal, Y.: Testing the influence of changing seawater Ca concentration on Elements Ca ratios in planktic foraminifera: A culture experiment, Geochem. Geophy. Geosy., 22, e2020GC009496, https://doi.org/10.1029/2020GC009496, 2021.
Lemelle, L., Bartolini, A., Simionovici, A., Tucoulou, R., De Nolf, W., Bassinot, F., and de Garidel-Thoron, T.: Nanoscale trace metal imprinting of biocalcification of planktic foraminifers by Toba's super-eruption, Sci. Rep., 10, 10974, https://doi.org/10.1038/s41598-020-67481-w, 2020.
Lin, C. Y., Turchyn, A. V., Steiner, Z., Bots, P., Lampronti, G. I., and Tosca, N. J.: The role of microbial sulfate reduction in calcium carbonate polymorph selection, Geochim. Cosmochim. Ac., 237, 184–204, https://doi.org/10.1016/j.gca.2018.06.019, 2018.
Lowenstein, T. K., Timofeeff, M. N., Brennan, S. T., Hardie, L. A., and Demicco, R.V.: Oscillations in Phanerozoic seawater chemistry: Evidence from fluid inclusions, Science, 294, 1086-8, https://doi.org/10.1126/science.1064280, 2001.
Lowenstein, T. K., Hardie, L. A., Timofeeff, M. N., and Demicco, R. V.: Secular variation in seawater chemistry and the origin of calcium chloride basinal brines, Geology, 31, 857–860, https://doi.org/10.1130/G19728R.1, 2003.
Marenco, P. J., Corsetti, F. A., Kaufman, A. J., and Bottjer, D. J.: Environmental and diagenetic variations in carbonate associated sulfate: an investigation of CAS in the Lower Triassic of the western USA, Geochim. Cosmochim. Ac., 72, 1570–1582, https://doi.org/10.1016/j.gca.2007.10.033, 2008.
Nagai, Y., Uematsu, K., Chen, C., Wani, R., Tyszka, J., and Toyofuku, T.: Weaving of biomineralization framework in rotaliid foraminifera: implications for paleoceanographic proxies, Biogeosciences, 15, 6773–6789, https://doi.org/10.5194/bg-15-6773-2018, 2018.
Nehrke, G., Keul, N., Langer, G., de Nooijer, L. J., Bijma, J., and Meibom, A.: A new model for biomineralization and trace-element signatures of Foraminifera tests, Biogeosciences, 10, 6759–6767, https://doi.org/10.5194/bg-10-6759-2013, 2013
Paris, G., Sessions, A. L., Subhas, A. V., and Adkins, J. F.: MC-ICP-MS measurement of δ34S and Δ33S in small amounts of dissolved sulfate, Chem. Geol., 345, 50–61, https://doi.org/10.1016/j.chemgeo.2013.02.022, 2013.
Paris, G., Fehrenbacher, J. S., Sessions, A. L., Spero, H. J., and Adkins, J. F.: Experimental determination of carbonate-associated sulfate δ34S in planktonic foraminifera shells, Geochem. Geophys. Geosyst., 15, 1452–1461, https://doi.org/10.1002/2014GC005295, 2014.
Paytan, A., Kastner M., Campbell, D., and Thiemens, M. H.: Sulfur Isotopic Composition of Cenozoic Seawater Sulfate, Science, 282, 1459–1462, 1998.
Perrin, J., Rivard, C., Vielzeuf, D., Laporte, D., Fonquernie, C., Ricolleau, A., and Floquet, N.: The coordination of sulfur in synthetic and biogenic Mg calcites: The red coral case, Geochim. Cosmochim. Ac., 197, 226–244, https://doi.org/10.1016/j.gca.2016.10.017, 2017.
Present, T. M., Paris, G., Burke, A., and Fischer, W. W.: Large carbonate associated sulfate isotopic variability between brachiopods, Earth Planet. Sci. Lett., 432, 187–198, https://doi.org/10.1016/j.epsl.2015.10.005, 2015.
Present, T. M., Adkins, J. F., and Fischer, W. W.: Variability in sulfur isotope records of Phanerozoic seawater sulfate, Geophys. Res. Lett., 47, e2020GL088766, https://doi.org/10.1029/2020GL088766, 2020.
Rennie, V. C. and Turchyn, A. V.: The preservation of δS and δO in carbonate-associated sulfate during marine diagenesis: A 25 Myr test case using marine sediments, Earth Planet. Sc. Lett., 395, 13–23, https://doi.org/10.1016/j.epsl.2014.03.025, 2014.
Rennie, C. F., Paris, G., Sessions, A. L., Abramovich, S., Turchyn, A. V., and Adkins, J. F.: Cenozoic record of δ34S in foraminiferal calcite implies an early Eocene shift to deep-ocean sulfide burial, Nat. Geosci., 11, 761–765, https://doi.org/10.1038/s41561-018-0200-y, 2018.
Richardson, J. A., Newville, M., Lanzirotti, A., Webb, S. M., Rose, C. V., Catalano, J. G., and Fike, D. A.: The source of sulfate in brachiopod calcite: Insights from μ-XRF imaging and XANES spectroscopy, Chem. Geol., 529, 119328, https://doi.org/10.1016/j.chemgeo.2019.119328, 2019.
Rollion-Bard, C. and Erez, J.: Intra-shell boron isotope ratios in the symbiont-bearing benthic foraminiferan Amphistegina lobifera: Implications for δ11B vital effects and paleo-pH reconstructions, Geochim. Cosmochim. Ac., 74, 1530–1536, https://doi.org/10.1016/j.gca.2009.11.017, 2010.
Sabbatini, A., Bédouet, L., Marie, A., Bartolini, A., Landemarre, L., Weber, M. X., Kade Mahardika, I. G. N., Berland, S., Zito, F., and Vénec-Peyré, M. T.: Biomineralization of Schlumbergerella floresiana, a significant carbonate-producing benthic foraminifer, Geobiology, 12, 289–307, https://doi.org/10.1111/gbi.12085, 2014.
Sandberg, P. A.: An oscillating trend in Phanerozoic non-skeletal carbonate mineralogy, Nature, 305, 19–22, 1983.
Schiebel, R.: Planktic foraminiferal sedimentation and the marine calcite budget, Global Biogeochem. Cy., 16, 1065, https://doi.org/10.1029/2001GB001459, 2002.
Schmidt, S., Hathorne, E. C., Schönfeld, J., and Garbe-Schönberg, D.: Heavy metal uptake of nearshore benthic foraminifera during multi-metal culturing experiments, Biogeosciences, 19, 629–664, https://doi.org/10.5194/bg-19-629-2022, 2022.
Tamenori, Y. and Yoshimura, T.: Sulfur speciation in growth layers of shell cross section of the long-lived bivalve Margaritifera laevis using synchrotron spectromicroscopy analysis, Geochim. Cosmochim. Ac., 237, 357–369, https://doi.org/10.1016/j.gca.2018.07.002, 2018.
Tamenori, Y., Yoshimura, T., Luan, N. T., Hasegawa, H., Suzuki, A., Kawahata, H., and Iwasaki, N.: Identification of the chemical form of sulfur compounds in the Japanese pink coral (Corallium elatius) skeleton using μ-XRF/XAS speciation mapping, J. Struct. Biol., 186, 214–223, https://doi.org/10.1016/j.jsb.2014.04.001, 2014.
Toyofuku, T., Matsuo, M. Y., de Nooijer, L. J., Nagai, Y., Kawada, S., Fujita, K., Reichart, G.-J., Nomaki, H., Tsuchiya, M., Sakaguchi, H., and Kitazato, H.: Proton pumping accompanies calcification in foraminifera, Nat. Commun., 8, 14145, https://doi.org/10.1038/ncomms14145, 2017.
Vachard, D., Pille, L., and Gaillot, J.: Palaeozoic Foraminifera: Systematics, palaeoecology and responses to global changes, Rev. Micropaleontol., 53, 209–254, https://doi.org/10.1016/j.revmic.2010.10.001, 2010.
van der Lee, J.: Thermodynamic and mathematical concepts of CHESS, Technical Report Nr. LHM/RD/98/39 CIG – Ecole des Mines de Paris, Fontainebleau, France, CHESS [code], https://chess.geosciences.mines-paristech.fr/presentation-1 (last access: 6 March 2003), 1998.
van Dijk, I., de Nooijer, L. J., and Reichart, G. J.: Trends in element incorporation in hyaline and porcelaneous foraminifera as a function of pCO2, Biogeosciences, 14, 497–510, https://doi.org/10.5194/bg-14-497-2017, 2017.
van Dijk, I., Barras, C., De Nooijer, L. J., Mouret, A., Geerken, E., Oron, S., and Reichart, G. J.: Coupled calcium and inorganic carbon uptake suggested by magnesium and sulfur incorporation in foraminiferal calcite, Biogeosciences, 16, 2115–2130, https://doi.org/10.5194/bg-16-2115-2019, 2019.
Weiner, S. and Erez, J.: Organic matrix of the shell of the foraminifer, Heterostegina depressa, J. Foramin. Res., 14, 206–212, https://doi.org/10.2113/gsjfr.14.3.206, 1984.
Zeebe, R. E. and Sanyal, A.: Comparison of two potential strategies of planktonic foraminifera for house building: Mg or H+ removal?, Geochim. Cosmochim. Ac., 66, 1159–1169. https://doi.org/10.1016/S0016-7037(01)00852-3, 2002.
- Abstract
- Introduction
- Materials and methods
- Results
- Discussion
- Conclusions
- Appendix A: Foraminifer taxonomy
- Appendix B: Appendix tables
- Appendix C: Potential experimental bias
- Appendix D:
- Code availability
- Data availability
- Author contributions
- Competing interests
- Disclaimer
- Acknowledgements
- Financial support
- Review statement
- References
- Supplement
- Abstract
- Introduction
- Materials and methods
- Results
- Discussion
- Conclusions
- Appendix A: Foraminifer taxonomy
- Appendix B: Appendix tables
- Appendix C: Potential experimental bias
- Appendix D:
- Code availability
- Data availability
- Author contributions
- Competing interests
- Disclaimer
- Acknowledgements
- Financial support
- Review statement
- References
- Supplement