the Creative Commons Attribution 4.0 License.
the Creative Commons Attribution 4.0 License.
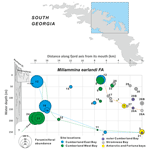
Unique benthic foraminiferal communities (stained) in diverse environments of sub-Antarctic fjords, South Georgia
Witold Szczuciński
Andrew J. Gooday
Sub-Antarctic fjords are among the environments most affected by the recent climate change. In our dynamically changing world, it is essential to monitor changes in these vulnerable settings. Here, we present a baseline study of “living” (rose-bengal-stained) benthic foraminifera from fjords of South Georgia, including fjords with and without tidewater glaciers. Their distribution is analyzed in the light of new fjord water and sediment property data, including grain size and sorting, total organic carbon, total sulfur, and δ13C of bulk organic matter. Four well-defined foraminiferal assemblages are recognized. Miliammina earlandi dominates in the most restricted, near-shore and glacier-proximal habitats, Cassidulinoides aff. parkerianus in mid-fjord areas, and Globocassidulina aff. rossensis and an assemblage dominated by Ammobaculites rostratus, Reophax subfusiformis, and Astrononion echolsi are in the outer parts of the fjords. Miliammina earlandi can tolerate strong glacial influence, including high sedimentation rates in fjord heads and sediment anoxia, as inferred from sediment color and total organic carbon sulfur ratios. This versatile species thrives both in the food-poor inner reaches of fjords that receive mainly refractory petrogenic organic matter from glacial meltwater and in shallow-water coves, where it benefits from an abundant supply of fresh, terrestrial, and marine organic matter. A smooth-walled variant of C. aff. parkerianus, apparently endemic to South Georgia, is the calcareous rotaliid best adapted to inner-fjord conditions characterized by moderate glacial influence and sedimentation rates and showing no preference for particular sedimentary redox conditions. The outer parts of fjords with clear, well-oxygenated bottom water are inhabited by G. aff. rossensis. Ammobaculites rostratus, R. subfusiformis, and A. echolsi dominate in the deepest-water settings, with water salinities ≥ 33.9 PSU and temperatures 0.2–1.4 ∘C, characteristic of winter water and Upper Circumpolar Deep Water. The inner- and mid-fjord foraminiferal assemblages seem specific to South Georgia, although with continued warming and deglaciation, they may become more widespread in the Southern Ocean.
- Article
(5174 KB) - Full-text XML
-
Supplement
(3889 KB) - BibTeX
- EndNote
South Georgia (SG) is uniquely positioned between the northern and southern streams of the Antarctic Circumpolar Current (ACC; Orsi et al., 1995). Since 1925, it has experienced significant warming of the surrounding shallow oceanic waters (Whitehouse et al., 2008) and widespread glacier retreat (Gordon et al., 2008; Cook et al., 2010), changes that coincide with the climatic reorganization of the Southern Ocean caused by the southward shift in the ACC (Gille, 2014) and intensification and southward migration of the southern westerly wind belt (Perren et al., 2020). These major environmental changes will likely continue into the future, strongly affecting marine and terrestrial ecosystems (Constable et al., 2014). They will be particularly evident in fjords (Bianchi et al., 2020) and especially in the highly sensitive sub-Antarctic island of SG, where biological invasions due to increasing vessel traffic are also likely to occur (Frenot et al., 2005). Now is therefore a timely moment to widen our understanding of fjord biota and their links with the local environmental conditions.
This study focuses on foraminifera, a major component of benthic assemblages in marine ecosystems. They are abundant, highly diverse, and many species are preserved in the fossil record. The short life cycle of foraminifera means that they are highly responsive to ecological changes (Jorissen et al., 2009) and therefore particularly valuable for reconstructing paleoenvironments and for monitoring the current state of marine environments (Murray, 1991). Moreover, elemental and isotopic composition of tests of calcareous foraminiferal may be calibrated to reflect composition of ambient seawater, which makes them useful for reconstructing past environmental conditions, including water temperatures and salinities (de Nooijer et al., 2014). All these factors make foraminifera an important subject of study.
The primary goal of this research is to document the distribution of different foraminiferal communities inhabiting fjords of SG. Based on new hydrological (water salinity and temperature) and sedimentological data, including grain size and sorting, total organic carbon (TOC), total sulfur (TS), and δ13C of bulk organic matter, we aim to link these communities with their typical habitats. This will provide a present-day baseline for interpreting fossil records and monitoring future faunal change. Finally, we compare the composition of foraminiferal assemblages inhabiting the fjords of SG with those in similar habitats north and south of the ACC. These results may provide insights into likely faunal and ecosystem changes in the Southern Ocean linked to anticipated climate warming.
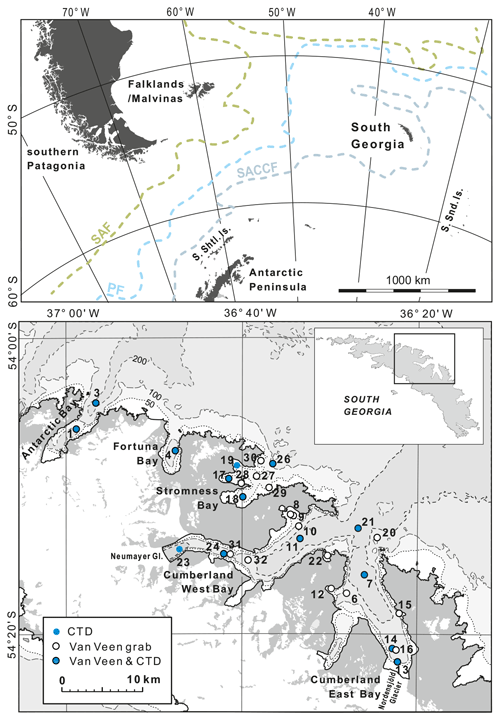
Figure 1Study area. The upper map (after https://freevectormaps.com, last access: 8 October 2021) shows the position of South Georgia in the southwestern Atlantic and the southern Antarctic Circumpolar Current front (SACCF), the polar front (PF), and the subantarctic front (SAF), after Orsi et al. (1995). The lower map (after the “South Georgia and The Shackleton Crossing” map 1 : 200 000, published by the British Antarctic Survey in 2017, and “Admiralty Chart 3588: Approaches to Stromness and Cumberland Bays”, 1 : 50 000, second edition, 23 January 2003) shows locations of sampling stations and those conductivity, temperature, and depth (CTD) stations, for which the data profiles are shown in Fig. 2.
1.1 Study area
South Georgia is approximately 170 km long and up to 40 km wide, making it one of the largest sub-Antarctic islands, in addition to being one of the most remote. It is located within the influence of the ACC (Fig. 1). The southern ACC front wraps anticyclonically around SG before continuing westwards to the north of the island (Thorpe et al., 2002). The island is surrounded by a wide shelf composed of continental crust, with a long geological history (Curtis et al., 2010), and water depths rarely exceeding ∼ 300 m (Graham et al., 2008). The closest large land areas are the Falklands (Malvinas) and southern Patagonia, with ∼ 1400 and ∼ 1700 km, respectively, to the west, and the Antarctic Peninsula, ∼ 1500 km, to the southwest. The smaller archipelagos of the South Sandwich Islands and the South Orkney Islands, lie about 700 km southeast and about 850 km southwest of SG, respectively (Fig. 1).
SG is under the influence of a maritime climate, with mean annual temperatures of ∼ 2 ∘C at Grytviken and annual precipitation of ∼ 1400 mm (Smith, 1960). Weather conditions can be quite variable from year to year, depending on the behavior of the ACC (Cook et al., 2010). SG is strongly affected by westerly winds that make its SW shores quite exposed and NE shores more sheltered. Due to structural upwelling on the leeward side of the archipelago, the close proximity of oceanic fronts, and local lithogenic sources, waters are rich in nutrients and productive (Davenport, 1995). Coastal water is very cold throughout the year, and in winter and spring it approaches freezing point. In Cumberland Bay, late summer fjord water temperatures decrease from ∼ 2.5 to ∼ 1.5 ∘C, and salinity increases from 33–34 to > 34.2 PSU near the sea floor at ∼ 250 m water depth (Römer et al., 2014; Geprägs et al., 2016). The water column is stratified, with lower salinity local water at the surface (< 33.6 PSU), underlain by a water mass resembling Antarctic Surface Water, and usually with the deepest water mass approaching the density of winter water and Upper Circumpolar Deep Water (Meredith et al., 2003).
SG is a heavily glaciated island, with ice caps and snowfields covering over 50 % of the surface. Many fjords are terminated by tidewater glaciers. Out of 103 coastal glaciers, 97 % have retreated since the 1950s (Cook et al., 2010). The areas of this study include most of the Cumberland East and West bays. These are fed at their heads by the Nordenskjöld and Neumayer glaciers, which rank among the largest on the island but show strikingly different retreat rates. While the Nordenskjöld Glacier is almost stable (Gordon et al., 2008), the retreat rate of the Neumayer Glacier accelerated to nearly 400 m yr−1 during 2005–2008 (Cook et al., 2010). The other main sampling area was Stromness Bay. Its catchment is mainly ice free, and only small cirque glaciers are present. All these bays are located in the center of the NE coast of SG. Additional material was collected in Fortuna Bay and Antarctic Bay, located to the northwest (Fig. 1).
Detailed multibeam swath bathymetry of the studied fjords indicated diverse geomorphology, with underwater glacial moraine ridges and distinct basins overprinting large-scale geomorphological features, including troughs that continue out to the shelf edge (Hodgson et al., 2014). The age of these submarine features, in both the larger and smaller scale, remains controversial because marine geological data are sparse (Barnes et al., 2016). The extent of the Last Glacial Maximum (LGM) glaciation is also debated, although an extensive LGM glaciation overriding at least much of the continental shelf (Graham et al., 2008, 2017; Barlow et al., 2016; White et al., 2018) seems more likely than the restriction of LGM glaciers to the fjords (Bentley et al., 2007; Hodgson et al., 2014). The millennial-scale sediment accumulation rates are known only from a few sediment cores recovered from the outer parts of Cumberland Bay and vary from 0.1 to 0.2 cm yr−1 (Berg et al., 2019, 2020; Graham et al., 2017).
1.2 Previous research
Terrestrial ecosystems of sub-Antarctic islands, including SG, have attracted considerable scientific attention, focusing on the impacts of climate change and the dispersal of invasive species (Bergstrom and Chown, 1999). However, because of its remoteness, marine ecosystems of SG remain largely understudied (Barnes, 2005), despite their important contribution to global and regional biodiversity (Hogg et al., 2011).
Table 1List of stations investigated for this study. Sedimentary indices include grain size, total carbon, total organic carbon, total sulfur, and δ13C of bulk organic matter (indicated in Table S1 in the Supplement). Note that CTD is for conductivity, temperature, and depth.
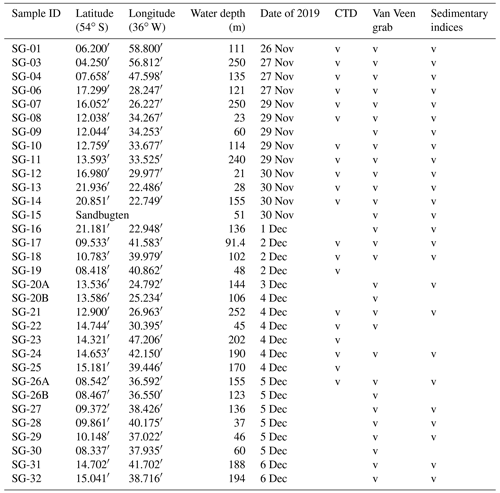
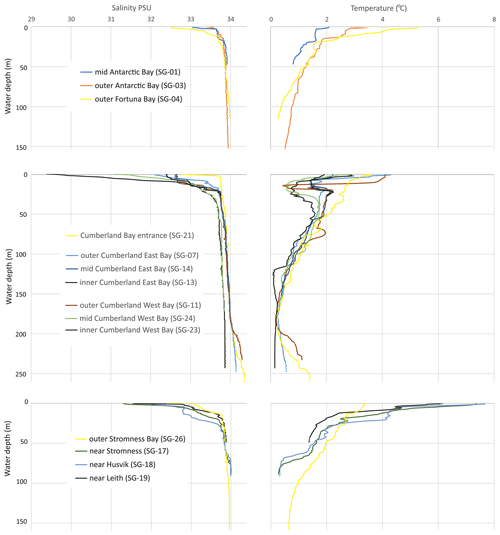
Figure 2Selected salinity and temperature profiles from Antarctic, Fortuna, Cumberland, and Stromness bays in South Georgia. The locations of the CTD profiles are indicated in Fig. 1 and in Table 1.
Nevertheless, thanks to financing from the whaling industry, SG was the location of one of the classic foraminiferal studies in the Southern Ocean (Earland, 1933, with preliminary papers by Heron-Allen and Earland, 1930, 1932). This work was based mainly on surface sediments collected during expeditions of the Royal Research Ship (RRS) Discovery (1925) and RRS William Scoresby (1926–1930), mainly at shelf sites but including also some shallower stations in Stromness Bay and Cumberland East and West bays. These publications established 49 new taxa, making SG one of the taxonomically best-known areas for foraminifera in the Southern Ocean. Apart from a recent paper based on samples from the outer shelf and upper slope (238–354 m water depth) north of SG (Dejardin et al., 2018), and an earlier study that included several sites at similar upper bathyal depths to the south of SG (Echols, 1971), nothing further has been published on the foraminiferal distribution and taxonomy in this region. However, a number of foraminiferal studies have focused on surrounding shelf areas, including the Falkland Islands and their adjacent shelf (Heron-Allen and Earland, 1932), fjords and channels of Patagonia (Violanti et al., 2000; Hromic et al., 2006), the South Shetland Islands (Finger and Lipps, 1981; Gray et al., 2003; Majewski, 2005, 2010; Majewski et al., 2007; Rodrigues et al., 2010), and the Antarctic Peninsula (Ishman and Domack, 1994; Ishman and Szymcek, 2003; Murray and Pudsey, 2004; Majewski et al., 2016). Research was also conducted in deepwater regions, highlighting foraminiferal gradients across the Drake Passage (Herb, 1971) and the frontal system of the ACC (Mackensen et al., 1993). Together, these works provide a firm regional background for this study.
2.1 Fieldwork
The fieldwork was conducted in fjords located in the central part of the northern coast of SG (Fig. 1 and Table 1) from the sailing research vessel (SRV) Saoirse in November and December of 2019. Hydrologic parameters, namely water density, temperature, and salinity (conductivity), were measured using a CTD48M memory probe (Sea & Sun Technology GmbH) at 20 stations (Table 1). Bottom-water measurements for particular stations were based either on direct conductivity, temperature, and depth (CTD) measurements or extrapolated from the nearest CTD profile at a similar water depth, since the water properties indicated a uniform water column stratification throughout each particular fjord (Fig. 2). The salinity is expressed in PSUs (practical salinity units). The classification of local water masses mainly followed a scheme proposed by Orsi et al. (1995), Meredith et al. (2003), Carter et al. (2008), and Geprägs et al. (2016).
2.2 Sampling
All sediment samples were collected using a Van Veen grab sampler with a sample area of 1000 cm2, manufactured by KC Denmark (model 12.211, matching Norwegian ISO standards). The grab was equipped with inspection windows and rubber plates that opened during descent. After the grab was recovered and secured on board, the inspection windows were opened and excess seawater carefully removed. In the case of over penetration, or if the sediment surface was disturbed (e.g., due to failure in sealing the grab during the ascent), the operation was repeated until a good sample was recovered.
For quantitative foraminiferal analyses, the upper 2 cm of sediment was sampled from an area of 63.5 cm2, defined by a ring 9 cm in diameter pressed to the sediment surface through one of the inspection windows. Samples for grain size and geochemical analyses were taken from the remaining surface sediment if available. Additional material was also taken for studies of delicate gromids (Gooday et al., 2022) and monothalamous foraminifera (Holzmann et al., 2022). At most stations, two replicate samples were obtained from two separate deployments of the grab. At stations SG-20 and SG-26, the boat's position could not be maintained due to difficult conditions, resulting in the recovery of replicates from significantly different water depths. In these two cases, both samples were analyzed. Replicates from the remaining stations were archived. In total, 29 samples were fully processed for stained foraminifera, with seven from Cumberland East Bay, eight from Cumberland West Bay, three from outer Cumberland Bay, eight from Stromness Bay, two from Antarctic Bay, and one from Fortuna Bay (Fig. 1 and Table 1). They were obtained at water depths down to 250 m, i.e., including the deepest parts of the fjords. To study the depth distribution of “live” (stained) foraminifera in the sediment profile, grab samples taken at stations SG-21 and SG-27 were subsampled with tubes of 7 cm diameter, providing cores 6 cm long. These were sliced into 1 cm thick intervals directly after recovery.
2.3 Sediment types and grain size analysis
All surface sediment samples were described on board in terms of sediment type, color, presence of ice-rafted clasts, and macro-biota. Subsamples were taken from 25 grab samples for volumetric grain size analysis using laser beam diffraction. Prior to the analysis, grains > 2 mm in size and organic matter were removed by passing the sample through a sieve, and the remaining sediment was treated with sodium hexametaphosphate and ultrasound to avoid grain aggregation. The analyses were performed in a Mastersizer 2000 particle size analyzer, and the resulting grain size statistics were calculated with GRADISTAT (Blott and Pye, 2001), using the logarithmic method of moments.
2.4 Geochemistry
Total carbon, TOC, and TS analyses were performed using an Eltra CS-500 IR analyzer at the Faculty of Natural Sciences, Institute of Earth Sciences, University of Silesia, following the standard procedure (Racka et al., 2010). The ratio was used as an approximate indicator of sediment redox, where ratios < 1.5 indicate anoxic, 1.5–5 periodically anoxic, and > 5 oxic conditions (Berner, 1983). The stable carbon isotopes of bulk organic matter in the sediments were analyzed using a Thermo Electron DeltaV Advantage isotope ratio mass spectrometer (IRMS) with ConFlo II and a Carlo Erba NA-1500 elemental analyzer at the University of Florida. The data were related to the USGS40 (United States Geological Survey) standard (n = 28; standard deviation, SD, = 0.081). All carbon isotopic results are expressed in standard delta notation relative to Vienna Peedee Belemnite (VPDB).
2.5 Micropaleontology
Directly after collection, samples were gently washed on a 63 µm mesh sieve with cold seawater and stained with rose bengal (2 g L−1) and 70 % ethanol diluted in seawater. Samples were left to stain for at least 2 d, washed, and left to dry for easier transport. The 63–125 and > 125 µm grain size fractions were dry-picked. Wherever possible, at least 300 stained individuals were picked from each fraction. If samples yielded < 300 stained individuals, then specimens from replicates were also picked in the same way as the regular samples. Consequently, for stations SG-12, SG-13, SG-14, SG-16, and SG-28, specimens from both replicates were further analyzed. Samples rich in foraminifera were divided using a dry microsplitter, and all stained tests were picked from the splits. Diversity indices, i.e., Shannon diversity index and dominance were calculated using PAST 4.03 software (Hammer et al., 2001).
Transparent calcareous foraminifera were classified as “living” if at least the final chamber was occupied by bright red- or violet-stained cytoplasm (Silva et al., 1996), and opaque agglutinated tests were conducted if the cement was intensely stained, especially when wet, or if stained material filled chambers that had been broken open. Porcellaneous foraminifera (miliolids) were regarded as living if material exposed in the aperture was stained or if the test acquired a distinct coloration after re-wetting (Schönfeld et al., 2012).
All foraminiferal specimens were arranged by taxon on micropaleontological slides and counted. Photographic documentation of specimens typical of each species (Figs. S1–S6 in the Supplement) was performed with a Phillips XL20 scanning electron microscope. The classification scheme used here is that of Loeblich and Tappan (1988) and the WoRMS database (World Register of Marine Species; Hayward et al., 2021). For species of Cassidulinidae, we adopted the latest species-level taxonomy of Majewski et al. (2021), which is based on molecular data. Specimens are housed at the Institute of Paleobiology, Polish Academy of Sciences (Warsaw), under the catalogue number ZPAL F.65.
2.6 Statistics
As a first step, we selected species that were most characteristic of different environmental settings. The frequencies of foraminiferal specimens collected from the > 125 µm fraction and the entire assemblage (> 63 µm; i.e., the > 125 plus 63–125 µm fractions) were analyzed individually with Q-mode orthogonal rotated (Varimax) principal component (PC) analysis, following Malmgren and Haq (1982) and Mackensen et al. (1990) and using a commercially distributed statistics package (SYSTAT 12). Fragile monothalamous foraminifera, such as Cribrothalammina, Pelosina, and Vanhoefenella, which are not preserved in subfossil samples, in addition to species never surpassing 1 % of the entire fauna and those present only at a single station, were excluded from the statistical analyses, as was sample SG-28 for the > 125 µm dataset, which yielded only 28 specimens in that size fraction. This procedure left 36 and 43 taxa in the > 125 and > 63 µm datasets, respectively.
In the second step, a P-mode PC analysis, with the number of factors set at two, was conducted, and PC loadings were plotted to identify species with similar distributions. Species within distinct groupings were assumed to have similar environmental requirements, thus belonging to distinct foraminiferal assemblages (Caulle et al., 2014). Combined abundance, expressed as a percentage of the entire assemblage, was then used for further analysis. Canonical correspondence analysis (CCA) was conducted using PAST 4.03 software (Hammer et al., 2001) in order to investigate the relationship between cumulative percentages of foraminiferal assemblages (FAs) and environmental parameters and sediment properties, including water depth, distance to major sediment source, distance to fjord mouth, bottom-water salinity and temperature, TOC, TS, δ13C, mean grain size, and sorting.
3.1 Fjord hydrology and sediments
3.1.1 Water temperature and salinity
The hydrology of the fjords was surveyed during the spring–summer onset of 2019 and revealed a similar pattern of water mass distribution in all the studied fjords (Fig. 2). The surface local water layer was characterized by relatively low salinity (28.4 to 33.6 PSU) and variable temperatures (0.5 to 7.6 ∘C). This water mass ranged in thickness from ∼ 30 m in the inner part of Cumberland Bay (SG-24) and 26 m in the inner Stromness Bay (SG-18) to 2.4–3.2 m at the outer-fjord/shelf stations (e.g., SG-01, SG-03, and SG-21). The lowest salinity (< 32 PSU) was found in the surface water of the inner Cumberland West Bay (SG-23, SG-24, and SG-25), which was influenced by meltwater from the tidewater Neumayer Glacier, and in bays supplied with freshwater snowmelt by streams and small rivers (e.g., stations SG-17 and SG-19 in Stromness and shallow coves in Cumberland Bay at SG-08, SG-12 and SG-22). The lowest temperatures for local water (< 1 ∘C) were noted in the inner parts of Cumberland Bay affected by tidewater glaciers and the highest (> 5 ∘C) in the inner part of Stromness Bay.
The major fjord water mass was found below local water, down to ca. 190–200 m of water depth (m w.d.) (Fig. 2). It was characterized by temperatures that decreased downwards from approximately 2.5 to 0.2 ∘C and salinities of between 33.6 and 34 PSU. This water mass was generally similar to Antarctic Surface Water and to winter water in the deepest parts. The deepest water mass (> 200 ) was encountered in two parts of Cumberland Bay (Fig. 1), near the fjord mouth and in the innermost western arm of the fjord, which is bounded by a shallow sill, < 30 , according to “Admiralty Chart 3588” (Fig. 1), and supplied with meltwater by the rapidly retreating Neumayer Glacier. In the outer part of Cumberland Bay (SG-11 and SG-21), the salinity of this deepest water mass increased with depth, reaching almost 34.4 PSU, while its temperature slightly increased downward from 0.2 to 1.3 ∘C, thus corresponding to Upper Circumpolar Deep Water. However, in the innermost part of the fjord (SG-22), the water mass below 200 m was colder (< 0.2 ∘C) and with maximum salinities reaching only 33.86 PSU. The temperature and salinity of the near-bottom water, which directly influences the benthic biota, ranged from 0.1 to 2.3 ∘C and 33.5 to 34.35 PSU and varied with water depth (Fig. 2 and Table S1). The highest temperatures (> 1.5 ∘C) were not only found at stations shallower than 60 m, located mainly in small bays far away from glacier fronts in Cumberland Bay (SG-22) and Stromness Bay (SG-28, SG-29, and SG-30), but also at SG-13 located close to the front of the Nordenskjöld Glacier. Moderate near-bottom temperatures of 0.75 to 1.5 ∘C were also found at shallow stations (< 60 ) and at the deepest stations of outer Cumberland Bay (SG-11 and SG-21; both > 240 m deep). The lowest near-bottom water temperatures (0.1–0.6 ∘C) were encountered at water depths of 90–250 m. Near-bottom waters with a salinity of < 33.8 PSU were found at water depths < 45 m and those with values between 33.8 and 34 PSU at depths between 37 and 210 m. In the deepest stations (SG-07, SG-11, and SG-21), water salinity was slightly > 34 PSU.
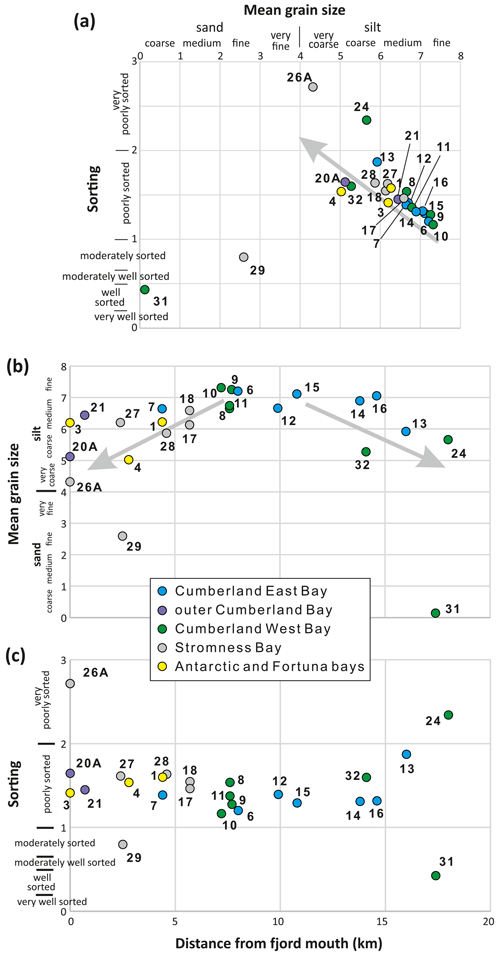
Figure 3Sediment mean grain size vs. sorting expressed in phi scale (a) and the same parameters in relation to distance from fjord mouth (b, c). Sediment categories are after Blott and Pye (2001). Note the trend line to coarser and less sorted sediments in panel (a), two coarsening trends towards the fjord mouth and glacier front in panel (b), and the presence of generally better sorted sediments in samples collected between 7 and 15 km away from fjord mouth in panel (c).
3.1.2 Sedimentology and grain size
Sediments in Antarctic Bay (SG-01 and SG-03) were composed of light gray, poorly sorted glacimarine mud (medium to coarse silt). Sediments in Fortuna Bay (SG-04) were also composed of gray, poorly sorted, sandy mud inhabited by Ophiuroidea. In Stromness Bay, sediments mainly comprised poorly sorted olive brown to light brown sandy mud. The mean grain size displayed a coarsening offshore trend, from medium silt in inner fjords to very coarse silt/fine sand at the fjord mouth (Fig. 3). Sediment in the central part of Stromness Bay (SG-27) was covered with an algal mat. There was some kelp and abundant plant detritus at station SG-28, located next to Grass Island at the entrance to the Stromness Harbor, the middle branch of Stromness Bay. Here, the sediments below the thin oxidized layer were dark (blackish). At station SG-29 (46 ), a near-shore location located at the fjord mouth and exposed to ocean swell, sediment was composed of dark, moderately sorted, fine sand.
Samples retrieved from the small coves of Cumberland Bay, i.e., Jason Harbor, King Edward Cove, Sandbugten, and Maiviken, contained olive gray, poorly sorted muds. In Jason Harbor (stations SG-08 to SG-10), they displayed a subtle, offshore fining trend. Sediments from the restricted, shallow-water settings of Jason Harbor (SG-08) and King Edward Cove (SG-12) were characterized by a ca. 1 cm thick light layer with dark (blackish) sediment below. At other stations, the color difference between surface and underlying sediment was much less striking. Fragmented kelp, algal mats, and biogenic sediment granules covered the surfaces of sediments retrieved at stations SG-08, SG-09, and SG-12. Sediment collected from deeper water (SG-10) contained more ice-rafted debris (IRD), with gravels up to 6 cm in diameter, and tubes of Polychaeta.
Sediments in the inner part of Cumberland West Bay (SG-23 and SG-24) were light gray, very loose, and very poorly sorted sandy muds with IRD gravel. In the outer part (SG-32, SG-11, SG-20, and SG-21), they were composed of IRD-rich, poorly sorted sandy muds and muds colonized by macroscopic benthic organisms (e.g., sponges, Polychaeta). At station SG-31, located west of the shallow sill in the middle part of the fjord, gray and well-sorted coarse sand predominated.
Cumberland East Bay was characterized by gray (SG-13 and SG-16 in the inner part) to olive gray (SG-06 and SG-07 in the outer part of the bay), poorly sorted, mud to sandy mud (SG-13 next to the glacier). These sediments displayed an offshore grain size fining trend (Fig. 3). In front of the tidewater glacier (SG-13), the sediment was loose, rich in IRD, and inhabited by Ophiuroidea, while in the central part of the fjord, Polychaeta tubes were present more frequently than at other locations.
The majority of the analyzed sediment samples were classified as poorly sorted fine to coarse silt. The sorting was generally correlated with mean grain size, with the finer sediments being generally better sorted (Fig. 3). The two outliers were moderately to well-sorted sand samples from the swell-affected, shallow-water station SG-29 in Stromness Bay and from SG-31 located on the slope of a sill/moraine in the central part of Cumberland West Bay, a station apparently swept by currents. When related to the distance along the fjord, samples collected between 7 and 15 km from the fjord mouth (all from Cumberland Bay) included the finest sediments and were slightly better sorted than those from other stations, except for the two outliers (Fig. 3b and c). Subtle trends of coarsening towards both the mouth and head of the fjord (Fig. 3b) may reflect a reduced influence of meltwater discharge with distance from the tidewater glaciers, combined with an increasing winnowing effect of the oceanic currents and swell, and/or increasing relative contribution of IRD in bulk sediments towards the fjord mouth.
3.1.3 TOC and TS
The total carbon concentrations in the analyzed sediment samples were almost the same as TOC, reflecting the very low total inorganic carbon values (< 0.04 %). The TOC values varied between 0.23 % and 1.1 %, except for sample SG-28 located next to Grass Island in Stromness Bay, which had a TOC content of 2.92 % (Fig. 4). The lowest value (< 0.5 %) was recorded at all stations located in the inner part of Cumberland Bay, > 13 km away from the fjord mouth (Fig. 4a). TOC was elevated (∼ 1 %) in the shallow-water settings of King Edward Cove (SG-12), Jason Harbor (SG-08), and stations inside Stromness Bay (SG-17, SG-18, and SG-27). Sediments from stations near the fjord mouth and in the middle of Cumberland Bay yielded intermediate TOC contents.
TS varied between 0.07 % and 0.38 % (Fig. 4). The highest values (> 0.2 %) were limited to the four shallowest stations (SG-08, SG-12, SG-13, and SG-28), all < 40 Intermediate values between 0.15 % and 0.2 % were only recorded in the innermost part of Cumberland West Bay (SG-24, SG-31, and SG-32), while the remaining samples yielded the lowest values (< 0.15 %; Fig. 4b).
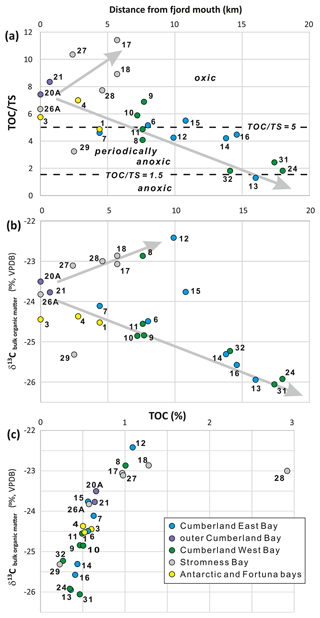
Figure 5Total organic carbon to total sulfur ratio () (a), carbon stable isotopes of bulk organic matter in the sediments (b) in relation to distance from the fjord mouth, and the relation between TOC and the δ13C values (c). Note the different trends/mixing lines in panel (a), towards increasing ratios in Stromness Bay and towards lower ratios in Cumberland Bay, and in panel (b), towards less negative δ13C values for Stromness Bay and coves and towards more negative values for the main basins of Cumberland Bay affected by tidewater glaciers. Dashed lines in panel (a) are after Berner (1983). They demarcate ratios suggested for anoxic (< 1.5), periodically anoxic (1.5–5), and oxic conditions (ratios > 5). Trends in panel (b) may represent the progressive mixing of different types of bulk organic matter, namely (1) material typical of open-marine conditions (δ13C ∼ −24 ‰), (2) likely petrogenic organic carbon supplied by glaciers (δ13C ∼ −26 ‰), and (3) organic matter derived from fresh terrestrial and marine sources (δ13C ∼ −23 ‰).
The variations in TOC and TS were not consistent, and their ratio was used as a rough indicator of redox conditions (Fig. 5). The highest ratios, typical of mainly oxic sediments, were found in Stromness Bay sediments (all except of SG-29) and in the outer parts of the Cumberland and Antarctic bays. The lowest values, typical of anoxic sediments, were found in the inner parts of the two main branches of Cumberland Bay, next to terminating tidewater glaciers (Fig. 5a). Two general trends in were observed. Ratios increasing from the fjord mouth towards the fjord head in Stromness Bay and decreased from the fjord mouth towards tidewater glaciers occupying fjord heads in Cumberland Bay (Fig. 5a).
3.1.4 δ13C of bulk organic matter
The δ13C in bulk organic matter ranged from −26.06 ‰ to −22.42 ‰ (Fig. 5) and correlated with TOC. The most negative δ13C values were in samples poorest in TOC, close to the tidewater glacier fronts in the innermost parts of Cumberland Bay. The least negative values were found in samples richest in TOC in the restricted, shallow-water settings of Jason Harbor (SG-08) and King Edward Cove (SG-12) and in Stromness Bay (SG-17, SG-18, SG-27, and SG-28). Two major trends were observed in relation to the distance from the fjord mouth (Fig. 5). In fjords with tidewater glaciers, δ13C became more negative towards the glaciers at the fjord heads. However, in the case of the coves and Stromness Bay, which are not directly affected by glaciers, values increased in the opposite direction. Station SG-15, located in a semi-restricted cove of Sandbugten at a modest distance of ∼ 12 km from the Nordenskjöld Glacier, showed δ13C values between these two trends.
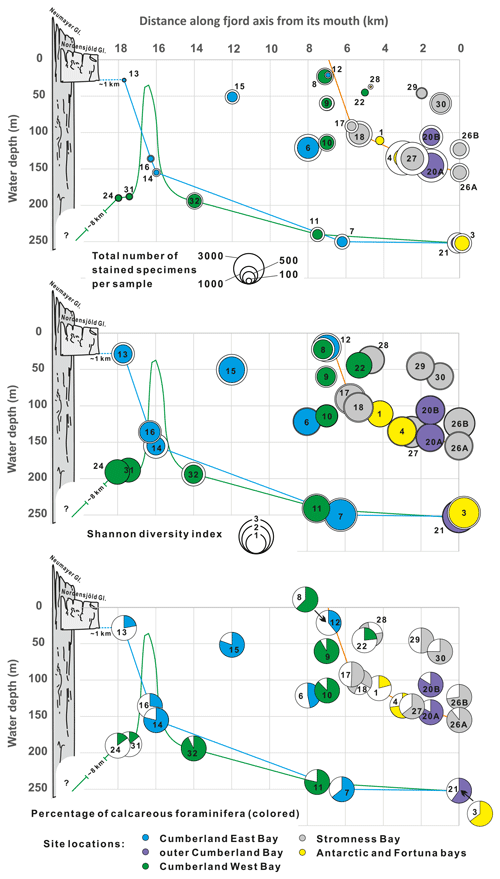
Figure 6General indices of benthic foraminiferal assemblages plotted against bathymetry and distance to open sea along fjord axes. Different colors represent locations in various fjords. Color lines show simplified bathymetric profiles for Stromness Bay, Cumberland East Bay, and Cumberland West Bay. Colored circles are for the > 125 µm fraction, and white circles in two upper graphs are for the > 63 µm fraction. Schematic profiles of the Nordenskjöld and Neumayer glaciers marked in bright and dark gray, respectively.
3.2 Foraminiferal data
3.2.1 General indices
In the 29 surface samples, a total of 15 191 living (i.e., stained) benthic foraminiferal specimens were isolated, including 8136 specimens in the > 125 µm fraction (Tables S2–S4 in the Supplement). They represented > 55 species (Figs. S1–S6). The assemblages show significant faunal variability, with numbers of taxa in a single sample (> 125 µm fraction) ranging from 5 to 32 (average 16.6) and the Shannon diversity index from 0.84 to 2.61 (average 1.70; n = 29), with standard deviations (SDs) of 6.92 and 0.50, respectively. The Shannon diversity index is the highest in outer fjords at depths of ∼ 100 m or more, and the lowest near the heads of fjords with terminating tidewater glaciers (Fig. 6). Dominance reaches 0.12–0.64 (average 0.30). The numbers of stained specimens per sample (63.5 cm2 in area) range from 14 to 1979 (average 551; SD = 501). The highest abundances are found between 150 and 100 in outer parts of fjords and in the deepest parts of Stromness Bay. The lowest abundances are in the shallow parts of Stromness Bay and at locations near fjord heads (Fig. 6). For the > 63 µm fraction, the values of all indices, except dominance, are higher (Fig. 6). The Shannon diversity index ranged from 1.18 to 2.85 (average 1.99).
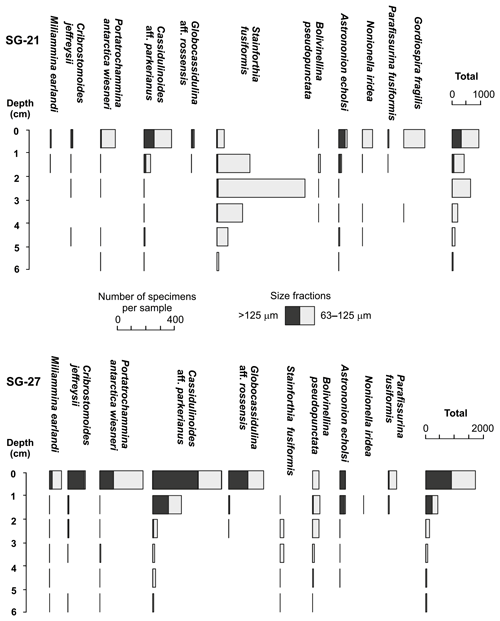
Figure 7Abundances in numbers per sample of stained foraminifera in two short cores taken at stations SG-21 and SG-27. Note the different scales for the specific and total abundances.
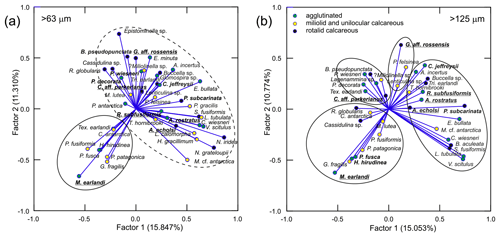
Figure 8Plots of the factor loadings for the P-mode PC analysis, showing the distribution of different foraminifera species for the > 63 µm (a) and > 125 µm (b) datasets. Important species for each foraminiferal assemblage (FA), encircled, defined by the Q-mode PC analysis (Table S5) are in bold, while the dominant species are underlined. The PC model was based on relative abundances of the more important species, i.e., > 1 % of total assemblage in at least one sample. Important species for each FA are in bold, while dominant species are underlined. Note the much better resolution of the four FAs for the coarser fraction.
The two short cores taken at stations SG-21 and SG-27 yielded a total of 2810 stained specimens, including 1062 from the > 125 µm fraction, representing 47 species. The richest assemblages (> 63 µm), in terms of abundances and number of species per sample, were in the upper layers (0–1 cm), totaling 952 and 1724 individuals in cores SG-21 and SG-27, respectively (Fig. 7). The lowest numbers of specimens (36 and 34, respectively) were in the bottom layers (5–6 cm). In the SG-21 sample, the decline in abundances downcore was not uniform, due to a sharp increase in Stainforthia fusiformis between 2 and 3 cm below sediment surface.
3.2.2 Identification of foraminiferal assemblages
Based on the Q-mode PC analyses, four PC models were selected as best reflecting the actual assemblages for both the > 125 and > 63 µm datasets. They explain 83.1 % and 82.0 % of the total variance in the two datasets, respectively. The calculated PCs are defined by foraminiferal species with large score values (Tables S5 and S6 in the Supplement; Fig. 8). They are hereafter referred to as foraminiferal assemblages (FAs) using the names of the dominant taxa, namely the calcareous rotaliids Globocassidulina aff. rossensis (Fig. S6; see 12–13) and Cassidulinoides aff. parkerianus (Fig. S6: 6–11), and the agglutinated Ammobaculites rostratus (Fig. S2: 1–2) and Miliammina earlandii (Fig. S1: 17). The species with the highest PC scores are the same in both datasets and are therefore not affected by the size–fraction bias.
The P-mode PC analysis was also run on the two datasets separately. The four faunal groups identified in the Q-mode PC analysis could be better distinguished in the PC loadings plot for the > 125 µm fraction (Fig. 8b). They seem to include different numbers of species, ranging from 3 in the case of the G. aff. rossensis FA to 16 in the case of the A. rostratus FA. Importantly, all FAs combine agglutinated and calcareous species. The plot for the > 63 µm fraction is less resolved, showing only two groups of taxa (Fig. 8a), indicating that assemblages may be better resolved in the coarser fraction (Jennings and Helgadottir, 1994; Schönfeld et al., 2012; Caulle et al., 2014). Consequently, only the > 125 µm results are discussed further.
3.2.3 Spatial distribution patterns of the FAs and their nominative species
Trends in the relative combined abundances of the four FAs and in the nominative species alone along the fjord axes are shown in Fig. 9. The M. earlandi FA dominated near the fronts of tidewater glaciers and at the most restricted shallow-water stations. The nominative species, M. earlandi, exceeded 35 % of the entire assemblage in samples from King Edward Cove near Grytviken and from Jason Harbor (Fig. 6), both small coves with water depths of ∼ 20 m. Miliammina earlandi accounts also for > 30 % of the entire assemblage at four stations located closest to the fjord heads and tidewater glaciers of Cumberland West and East bays, with water depths of 190 and 28 m, respectively (Fig. 6). In Cumberland West Bay, this species is commonly accompanied by the agglutinated species Psammosphaera fusca and Hippocrepinella hirudinea, and in Cumberland East Bay this species is commonly accompanied by the calcareous species Gordiospira fragilis and Pyrgo patagonica, all of which contribute to the M. earlandi FA (Fig. 8b).
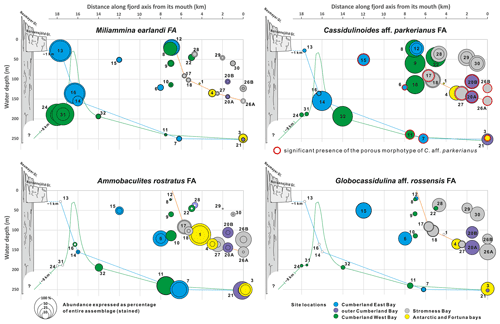
Figure 9Cumulative percentages of the four species groups (FAs) defined by the PC analysis in relation to the entire > 125 µm assemblages plotted against bathymetry and distance along fjord axis to its mouth. Smaller circles indicate percentages of the nominative species and accumulative percentage of A. rostratus, R. subfusiformis, and A. echolsi for the A. rostratus FA. All graphs are at the same scale. Different colors represent locations in various fjords. Color lines show simplified bathymetric profiles for Stromness Bay, Cumberland East Bay, and Cumberland West Bay. Schematic profiles of the Nordenskjöld and Neumayer glaciers marked in bright and dark gray, respectively.
The C. aff. parkerianus FA dominates in the largest number of samples (Fig. 9). It is common in transitional locations between stations dominated by the three remaining FAs. The nominative C. aff. parkerianus is the most widespread and abundant species in our dataset. It is represented by two forms (Majewski et al., 2021), namely a dominant, smooth-walled conical morphotype, assigned by Heron-Allen and Earland (1929) and Earland (1933) to Ehrenbergina crassa, and a subordinate porous form resembling C. parkerianus, sensu Brady (1881), the presence of which is marked in Fig. 9 by red circles. The dominant morphotype reaches the highest percentages, usually well over 50 %, far inside Cumberland East and West bays, at water depths > 150 m, and at stations SG-08 to SG-10 between 114 and 23 in the middle Cumberland West Bay (Fig. 9). At stations in the outer Stromness (SG-26A, SG-26B and SG-27) and outer Cumberland bays (SG-20A and SG-20B), between 100 and ∼ 150 , the subordinate porous morphotype of C. aff. parkerianus is also present.
The G. aff. rossensis FA dominates near fjord mouths, where foraminifera are relatively abundant and rather rich in calcareous forms (Figs. 6 and 9). Globocassidulina aff. rossensis strongly dominates its FA, reaching up to 51 % in the sample from station SG-26A in the outer Stromness Bay and exceeding 40 % SG-20A in the outer Cumberland Bay. It contributes not only to the highest proportion between ∼ 50 and ∼ 150 in the outer reaches of fjords but also at two stations, SG-15 and SG-06 (51 and 121 , respectively), in central Cumberland East Bay (Fig. 5). Globocassidulina aff. rossensis is also present at water depths > 150 m, well inside Cumberland West Bay, at stations dominated by M. earlandi and C. aff. parkerianus, albeit in low and definitely subordinate numbers.
The A. rostratus FA shows a strong presence in the middle and outer Cumberland Bay and throughout Stromness Bay, although not at stations < 100 It is especially important in the deepest fjord settings but not proximal to glacier fronts (Fig. 9). This FA dominates at stations with the highest species diversity (Fig. 6) and comprises the largest number of species, including agglutinated and calcareous forms (Fig. 5a). Its nominative species, the agglutinated A. rostratus, is less dominant than those of other FAs, and according to the Q-mode PC analysis (Table S5), Reophax subfusiformis and Astrononion echolsi, and to a lesser degree Cribrostomoides jeffreysii and Pullenia subcarinata, are also important for defining this FA.
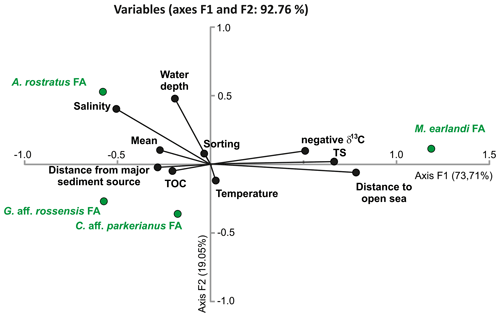
Figure 10CCA plot of the faunal assemblages (FAs) defined by PC analysis (in green) with various parameters (in black), including location (water depth, distance from major sediment source, and distance to open sea), environmental parameters (salinity and temperature), and sediment properties (total organic carbon (TOC) and its isotopic composition, total sulfur (TS), mean grain size, and sorting).
3.2.4 Relation between FAs and environmental and sediment properties
CCA was performed in order to explore the relationship between location (water depth, distance to major sediment source, and distance to fjord mouth), environmental parameters (water salinity and temperature), and sediment properties (mean grain size and sorting, TOC, TS, and δ13C of bulk organic matter), all listed in Table S1, and the cumulative percentages of the four FAs (Table S1 and Fig. 8b). CCA axis 1 explains 73.71 % of the variance, and axis 2 explains 19.05 % (together 92.76 %). Most of the variables plot along axis 1, with the M. earlandi FA, positive distance to open sea, TS, and negative δ13C strongly on the positive side and most of the remaining parameters and the three remaining FAs on the negative side (Fig. 10). The A. rostratus and C. aff. parkerianus FAs, in addition to water depth, bottom-water salinity, and temperature, showed significant variability along axis 2. The A. rostratus FA appears to be correlated with elevated salinity, and the G. aff. rossensis FA appears to be correlated with elevated TOC, distance from major sediment source, and less negative δ13C. No clear correlation can be noted for the C. aff. parkerianus FA, which also shows the weakest relation with axis 1 of the CCA plot.
4.1 Data quality
Members of the FOraminiferal BIo-MOnitoring initiative (FOBIMO) have proposed several recommendations for monitoring soft-bottom environments using benthic foraminifera (Schönfeld et al., 2012). We aimed to follow their recommendations, such as collecting replicates, rose bengal staining, and analyzing the > 125 µm, as well as the 63–125 µm, fractions. In some cases, however, we had to adapt the protocol to our field conditions.
First, because sampling time was limited, staining had to be reduced from the recommended 14 d to only a few days. In order to ease the penetration of the stain, we gently washed the samples on a 63 µm sieve with cold seawater, as is routinely done for studying fragile monothalamid foraminifera, before adding rose bengal in ethanol. We believe it was a reasonable precaution, especially since 24–48 h of staining can already provide satisfactory results (Bernhard et al., 2006). Second, we sampled the upper 0–2 cm instead of the 0–1 cm interval as recommended by FOBIMO. Due to a frequent presence of a semi-liquid, flocculent top layer, rich in suspended organic fragments, it was difficult to recognize the sediment–water interface precisely (Fossile et al., 2020). Our motivation was that, for studying robust calcareous and agglutinated species, it is better to sample a thicker rather than a thinner upper sediment layer, especially considering the sharp decline in living individuals with depth in similar fjord settings (Majewski, 2013; Fossile et al., 2020). The final and potentially the most critical modification was our use of a Van Veen grab sampler, a device not recommended by Schönfeld et al. (2012). However, our model, equipped with rubber plates that opened during descent and reduced the bow wave, proved to be highly efficient and reliable during former sampling for soft-testate foraminifera (Majewski et al., 2007; Gschwend et al., 2016). A Kajak sampler was also available during our sampling campaign, but it was inefficient.
To provide additional confidence in our procedures, we collected and analyzed two short cores from two of the Van Veen grab samples (SG-21 and SG-27; Fig. 7). In both sediment profiles, we observed abundant and diverse stained assemblages (> 125 µm fraction) that were concentrated mainly in the surface layer (Fig. 7). Although the lower distribution limits appeared significantly shallower than on the shelf north of SG (Dejardin et al., 2018), they were comparable to those from similar fjord settings in the South Shetland Islands (Majewski, 2010) and Spitsbergen (Fossile et al., 2020). Moreover, the 63–125 µm fraction of the SG-21 subcore revealed a strong subsurface peak in the abundance of Stainforthia fusiformis (Fig. 7). The clear distribution pattern of this minute, predominantly infaunal species agrees with its known occupancy of infaunal microhabitats, as reported, for example, by Alve (1994), and confirms that the Van Veen grab samples were relatively undisturbed. The Shannon diversity index calculated for > 63 µm data was in the range from 1.18 to 2.85, encompassing the values of 2.29 and 2.36 recorded more offshore by Dejardin et al. (2018). Nevertheless, it is important to note that our surface sampling targeted mainly epibenthic species and that the following discussion is based on the > 125 µm data, thereby underestimating the presence of minute, infaunal species such as S. fusiformis.
A final point is that two of the four nominative species for the FAs, G. aff. rossensis and C. aff. parkerianus, are phylogenetically closely related (Majewski et al., 2021) and their immature individuals can be difficult to distinguish (Nomura, 1983). However, the color of the cytoplasm when stained was clearly different, with the color being more violet in the case of G. aff. rossensis and more pinkish in C. aff. parkerianus case, making it easy to discriminate between living individuals.
4.2 Environmental zones characterized by FAs
We identified four FAs by PC analysis (Fig. 8b). They are very distinctive in terms of species composition and distribution. Figure 9 emphasizes the gradation between FAs dominating proximal to fjord head stations and those showing a stronger presence near the open sea or at greater water depths. According to the CCA (Fig. 10), these four FAs are related to different environmental conditions and sediment properties. In the following sections, we discuss the environmental setting of each.
4.2.1 Inner parts of Cumberland Bay and shallow-water coves: strong glacial influence and sediment anoxia
Restricted areas near tidewater glacier fronts at fjord heads (SG-13, SG-16, SG-24, and SG-31) and shallow-water coves (SG-08 and SG-12) are dominated by the M. earlandi FA (Fig. 9). In these locations, foraminiferal assemblages are impoverished, showing generally low numbers of stained specimens, low to moderate species diversities, and, except at SG-08, a clear dominance of agglutinated forms (Fig. 6). The CCA (Fig. 10) indicates that this FA correlates with (1) a large distance from the open sea and, although less clearly, proximity to major sediment sources, (2) strongly negative δ13C values, and (3) a high TS.
The first two correlations are somehow ambiguous as the two types of settings characteristic of the M. earlandi FA, i.e., near the fjord heads and in the coves (Figs. 1 and 6), although both quite restricted, have rather different characteristics. The environments near tidewater glacier fronts are characterized by variable water depth, relatively coarse glacially derived sediments that display a fining trend with distance from the sediment source (Fig. 3), and a thick layer of brackish, turbid surface water (Fig. 2). In these glacial fjords, sediment TOC values are low (< 0.5 %, Fig. 4a) and organic matter δ13C values strongly negative (Fig. 5b), likely due to the mainly petrogenic source of organic carbon (Berg et al., 2021). Given the climate (high precipitation), mountainous relief, and the susceptibility of rock types to erosion (mudstones and sandstones), it can be expected that sedimentation rates in front of tidewater glaciers are high and of the order of several centimeters per year, as in the case of similar subpolar fjord systems (e.g., Majewski et al., 2012; Boldt et al., 2013; Streuff et al., 2015). Indeed, preliminary 210Pb dating of a sediment core from station SG-16 (ca. 2.5 km away from the front of the Nordenskjöld Glacier) revealed a sediment accumulation rate of ∼ 1.4 cm yr−1 (Witold Szczuciński, unpublished data). In similar depositional environments, the sediment accumulation rate usually exhibits a rapid exponential decrease within the first several kilometers from the glacier front (e.g., Syvitski, 1989; Jaeger and Nittrouer, 1999; Szczuciński and Zajączkowski, 2012). Close to the tidewater glacier fronts, the rates may be of the order of several tens of centimeters per year. The sediments in glacial fjords are also loose and not compacted, which is also typical of high sedimentation rate conditions. In contrast, sediments in the shallow-water settings of King Edward Cove and Jason Harbor are among the finest in terms of mean grain size (Fig. 3) and have a thin surficial oxidized lighter mud layer underlain by dark (blackish), probably anoxic, sediments. Their sediment accumulation rates were found to be still relatively high, of the order of 0.4–0.7 cm yr−1, as revealed by 210Pb dating (Witold Szczuciński, unpublished data). These sediments also yielded high TOC concentrations (> 1 %; Fig. 4a) and δ13C ratios that were the least negative in this study (Fig. 5b). These differences seem to reflect different organic matter sources (Caulle et al., 2014; Jernas et al., 2018), with petrogenic organic carbon dominating near the tidewater glacier fronts and fresher organic matter of mixed origin (both terrigenous and marine) dominating in the coves (Berg et al., 2021).
These fairly substantial differences raise the question of whether M. earlandi might comprise several cryptic species, as suggested by molecular data for a related species, Miliammina fusca (Jan Pawłowski and Maria Holzmann, unpublished data). All stations dominated by the M. earlandi FA, however, do have one factor in common, namely elevated TS at roughly ∼ 0.2 % (Fig. 4b). The environmental implications of TS alone are difficult to interpret unequivocally, but the proportion has been used to evaluate the redox conditions in sediments (Berner, 1983). Thus, although we do not have direct measurements of bottom-water oxygenation or oxygen penetration depths (Caulle et al., 2014), the low ratios suggest oxygen deficiency at all stations dominated by M. earlandi FA (Fig. 5a). It is also in accordance with macroscopic sediment observations in shallow cove sediment samples. This interpretation is consistent with the occurrence of other representatives of Miliammina in dysoxic settings (Hayward and Hollis, 1994; Tyszka, 1997; Habura et al., 2006).
Earlier studies have shown that Miliammina earlandi is not confined to near-shore habitats in the Southern Ocean. This species has a rather complicated taxonomic history, with the name having been established by Loeblich and Tappan (1955) to replace the earlier name M. oblonga, which was applied incorrectly by Heron-Allen and Earland (1930) and Earland (1933, 1934; see also p. 53 in Loeblich and Tappan, 1988). The lectotype designated by Loeblich and Tappan (1955) is from 200 m depth, near the shelf edge well to the south of SG. According to Earland (1933), M. oblonga (= M. earlandi) is “universally distributed” and “probably the commonest and most widely distributed rhizopod of the South Georgia area”. The sites in which it is common range from 100 to 200 m, consistent with our new data, but it is also found down to 1752 m. In his subsequent Discovery report on the foraminifera of the Falklands area, Earland (1934) concludes that M. oblonga (= M. earlandi) is “generally distributed in all areas within the Antarctic convergence and sometimes very common”. The stations he mentioned are at depths of between 244 and 1838 m. Miliammina earlandi also occurs in the Patagonian fjords, although it is not common (Hromic et al., 2006). Assuming that these records refer to a single species, M. earlandi is clearly widely distributed both geographically and bathymetrically in the Southern Ocean and adjacent areas, suggesting that it can tolerate a wide range of conditions.
Adaptability to different habitats seems to be a characteristic of the Miliammina species more generally. Together with other members of the Rzehakinidae, they inhabit a wide spectrum of both open- and marginal-marine environments (Habura et al., 2006). They are common in salt marshes, mangrove swamps, and oligohaline estuaries (Sen Gupta, 1999), persist longer than other foraminifera in marine basins isolated from the sea (Lloyd and Evans, 2002), and dominate in a volcanic caldera less than a decade after significant eruptions (Finger and Lipps, 1981). Miliammina species have been also reported from mesohaline habitats (Hayward and Hollis, 1994; Lloyd and Evans, 2002; Habura et al., 2006). In Antarctic waters, M. arenacea is associated with corrosive high-salinity shelf water (Kennett, 1968; Milam and Anderson, 1981; Ishman and Sperling, 2002) and thrives in areas affected by Antarctic polynya (Capotondi et al., 2018). In SG, highly saline bottom waters were not detected during the present study (Fig. 2) or previous SG surveys (Römer et al., 2014; Geprägs et al., 2016). Instead, the M. earlandi FA tends to be associated with rather low salinity (Fig. 10), and its distribution is not restricted to deeper stations (Fig. 9).
This adaptability could confer advantages for M. earlandi over other foraminifera at our stations in SG fjords. This may be the last species with a robust test to withstand elevated sediment accumulation rates in fjord heads. It seems to survive anoxia, be able to exploit different food sources, and at the shallow-water stations (SG-08, SG-12, and SG-13), which are at the lower limit of local water influence (Fig. 2), it might experience changes in water salinity and temperature.
It is also worth noting that the M. earlandi FA includes different auxiliary species in different areas. In Cumberland West Bay, these are mainly the agglutinated Psammosphaera fusca and Hippocrepinella hirudinea, and in Cumberland East these are the calcareous Gordiospira fragilis and Pyrgo patagonica (Fig. 8b). This difference may be related to different glacial regimes in these two branches of Cumberland Bay (Gordon et al., 2008).
4.2.2 Mid-fjord settings: transitional conditions
Stations bordering those dominated by the M. earlandi FA in the inner reaches of Cumberland Bay (SG-14 and SG-32) show low to intermediate foraminiferal abundance and diversity (Fig. 6) and a dominance of the C. aff. parkerianus FA. This is the most widespread FA in our dataset (Fig. 9) and also the most enigmatic. It shows the weakest relation with the main axis 1 on the CCA plot and does not reveal a straightforward correlation with any of the environmental parameters measured in our study (Fig. 10). The C. aff. parkerianus FA possibly shows some association with limited water depths and water properties corresponding with Antarctic Surface Water. However, it is less important near fjord mouths, where this water mass predominates (Figs. 1 and 2); therefore an explicit correlation of this FA with Antarctic Surface Water is unlikely. The subordinate, porous morphotype of C. aff. parkerianus (Majewski et al., 2021) is rare or absent far inside Cumberland Bay and in the central part of its western branch (Fig. 9), suggesting a preference towards more open marine conditions.
Cassidulinoides aff. parkerianus is exceptionally well represented throughout Cumberland Bay but not in the innermost part, where the M. earlandii FA dominates, or at the deepest stations dominated by the A. rostratus FA or around fjord mouths, where the G. aff. rossensis FA predominates. Its strongest presence is in transitional locations between those characterized by these three FAs, so it seems to flourish where conditions are not optimal for other taxa. The abundance of the smoothly walled conical morphotype (Majewski et al., 2021) at stations SG-14 and SG-32, adjacent to the most glacier-proximal stations dominated by the M. earlandi FA, suggests that C. aff. parkerianus FA and its associated species (Fig. 8b) are well adapted to inner-/middle-fjord conditions characterized by moderate sedimentation rates. This would be unique among Cassidulinoides species, which are generally rare or absent in similar settings in the Arctic (Alve et al., 2016), Antarctic (Majewski, 2005), and Patagonia (Hromic et al., 2006). The strong presence of this FA in Jason Harbor, within Cumberland West Bay (SG-08, SG-09, and SG-10), suggests an association with the finest-grained sediments (Fig. 3b). The ratios at stations dominated by the C. aff. parkerianus FA cover a wide range (1.8–6.9), consistent with hypoxic to fully oxic conditions (Berner, 1983) and no strong preference for any particular redox conditions.
4.2.3 Outer-fjord settings: weak glacial influence, low accumulation rate, relation to bathymetry and water masses
The outer-fjord areas are usually characterized by the lowest water turbidity and sedimentation rates (Syvitski, 1989), which, in the outer Cumberland Bay, are of the order of 0.1 to 0.2 cm yr−1 (Berg et al., 2019, 2020; Graham et al., 2017). Our data show variable foraminiferal abundances, with the highest between 100 and 150 and some of the lowest around 250 , with high Shannon diversity at the deepest stations (Fig. 6). The G. aff. rossensis and A. rostratus FAs dominating in outer fjords are located on the side of axis 1 opposite to the M. earlandi FA in the CCA plot (Fig. 10). They correlate with (1) decreased distance to the open sea and increased distance from a major sediment source, (2) less negative δ13C, and (3) high TOC and low TS; thus, high ratios indicate oxic conditions (Berner, 1983). The A. rostratus FA seems to be associated with greater water depths and particularly with elevated salinity (Fig. 10).
The A. rostratus FA is the most species-rich of the four FAs (Fig. 8b). It is abundant throughout Stromness Bay at > 100 and at the deepest stations in the middle and outer Cumberland Bay (Fig. 9). Its nominative species was described in SG from water depth considerably > 200 m (Heron-Allen and Earland, 1929) and seems endemic to this area (Dejardin et al., 2018). It is less dominant than the nominative species of other FAs, and R. subfusiformis and A. echolsi are almost equally important for defining this FA (Table S5). According to our CCA, this FA is strongly correlated with elevated salinity (Fig. 10), pointing to a possible association with winter water and Upper Circumpolar Deep Water. This would be consistent with observations from the Northern Hemisphere, where R. subfusiformis was recorded from locations with high and stable salinities (Höglund, 1947; Thiede et al., 1981; Jernas et al., 2018) and seemed to be more tolerant of a lower quality or quantity of food (Jernas et al., 2018). Moreover, the calcareous species A. echolsi and Bulimina aculeata, also important for this FA (Fig. 8b), are typical of open-marine assemblages in western Antarctica and show an affinity with highly saline Circumpolar Deep Water (Ishman and Domack, 1994; Majewski et al., 2016) that are the least cold (Mackensen, 2001) and one of the oldest (Catalá et al., 2015) water masses in that area.
In contrast with the apparent association of A. rostratus FA with winter water and Upper Circumpolar Deep Water, the distribution of the G. aff. rossensis FA (Fig. 9) may coincide with the upper and warmer Antarctic Surface Water, which dominates down to 100–150 in Cumberland Bay and throughout the outer Stromness Bay (Fig. 2). Correlation with high ratios indicates oxic conditions (Berner, 1983). However, it is important to note that the G. aff. rossensis FA is abundant only in the central part of Cumberland East Bay and not in Cumberland West Bay (Fig. 9). The front of the Nordenskjöld Glacier is grounded at a relatively shallow water depth of 20–30 m (Hodgson et al., 2014), and its position is relatively stable (Gordon et al., 2008). It is therefore likely delivering less turbid meltwater to Cumberland East Bay than the rapidly retreating Neumayer Glacier and several land-terminating glaciers in the Cumberland West Bay. As a result, the central part of the eastern branch of Cumberland Bay is less glacially affected, promoting a FA dominated by G. aff. rossensis rather than by C. aff. parkerianus. Thus, the G. aff. rossensis FA appears to flourish in the presence of well-oxygenated, clearer water and possibly lower sediment accumulation rates.
The presence of G. aff. rossensis near fjord mouths at locations distant to glacial and fluvial sediment sources (Fig. 9) is consistent with the distribution of this species in the Chilean fjords, where it is an important component of the oceanic and intermediate biofacies but is insignificant in the inner-fjord biofacies (Hromic et al., 2006). Its Antarctic sister species Globocassidulina biora (Finger and Lipps, 1981; Majda et al., 2018), on the other hand, seems to be well adopted to habitats proximal to glacier fronts (Majewski, 2005) and beneath ice shelves (Majewski et al., 2019). However, western Antarctic glaciers deliver significantly less turbid water than rapidly retreating glaciers in SG (Gordon et al., 2008; Cook et al., 2010), which may be one reason for the different distribution patterns near glacier fronts of these two phylogenetically related species.
4.3 Regional comparisons
Foraminifera have been widely studied in fjords, especially in the Arctic (Alve et al., 2016), where there is evidence for strong faunal differences between outer- and inner-fjord locations (e.g., Hald and Korsun, 1997; Korsun and Hald, 1998; Zajączkowski et al., 2010; Fossile et al., 2020) and links with different water masses (Jennings and Helgadottir, 1994; Jennings et al., 2004). The taxonomic composition of foraminiferal assemblages in Arctic fjords, however, is very different from that in the Southern Hemisphere. For example, in Svalbard and Novaya Zemlya, Cribroelphidium/Elphidium excavatum f. clavata thrives close to glacier fronts (Hald and Korsun, 1997; Korsun and Hald, 1998), while in SG we encountered only a few empty tests assigned to this genus, and it is also very rare in Antarctica (Majewski and Tatur, 2009). Cassidulinoides, so widespread in SG, seems absent in Arctic fjords (Alve et al., 2016), as are species important for other FAs, with the exception of the cosmopolitan R. subfusiformis and Trifarina earlandi/angulosa (Alve et al., 2016). These strong taxonomic differences between the Northern and Southern hemispheres make direct faunal comparisons impossible.
Previous studies of foraminiferal distributions in fjords located in the same sector of the Southern Hemisphere as SG are limited to Patagonia (Hromic et al., 2006; Sergei Korsun et al., unpublished data) and Admiralty Bay in South Shetland Islands (Majewski, 2005, 2010; Majewski et al., 2007). These have revealed clear taxonomic disparities between foraminifera inhabiting different sides of the Drake Passage (Majda et al., 2018; Majewski et al., 2021).
The taxonomic succession observed in the fjords of SG corresponds only in part with observations from Patagonia. Hromic et al. (2006) distinguished three benthic foraminiferal biofacies in the fjords and channels of southern Chile. The oceanic sandy biofacies, characterized by elevated organic matter concentrations and strongly influenced by Pacific water, showed high species richness and high abundances of G. rossensis and Trifarina angulosa. The intermediate fine-grained biofacies with low organic matter content and influenced by mixed Pacific and freshwater was not only dominated by two calcareous species, Cassidulina laevigata and G. rossensis, but also included an increased number of agglutinated taxa. The inner-fjord silty biofacies, influenced by cold, low-salinity water, was characterized by low species diversity and dominated by Bulimina notovata and C. laevigata (calcareous), together with Alveophragmium orbiculatum, Labrospira kosterensis, Recurvoides scitulum, and Labrospira jeffreysii (agglutinated), with the last two corresponding to Veleroninoides scitulus and Cribrostomoides jeffreysii, which are members of the A. rostratus FA in SG.
It appears, therefore, that the dataset of Hromic et al. (2006) only includes representatives of the two outer-fjord associations from SG, the G. aff. rossensis and A. rostratus FAs. Although M. earlandi, the nominative taxon of the inner-fjord FA, and C. parkerianus were encountered in Patagonia, they were not significant species in defining foraminiferal biofacies. The dataset of Hromic et al. (2006), however, did not include the most southerly glaciated fjords in Chilean Patagonia. These were analyzed by Sergei Korsun et al. (unpublished data), who sampled seafloor sediments in the Beagle Channel and its tributary fjords, including glaciomarine muds deposited in direct proximity to glacial fronts (Gschwend et al., 2016). They noted C. parkerianus as an important species, but it seems morphologically and genetically different from C. aff. parkerianus in SG (Majewski et al., 2021). The innermost fjord samples in the Beagle Channel region contained only a few foraminifera (∼ cm3), which alternated randomly between stations. There was no distinct glacier-proximal assemblage, and Miliammina, which is so prominent in the most restricted settings in SG, was absent (Sergei Korsun et al., unpublished data).
In conclusion, it appears that the faunal change is more pronounced along the axes of sub-Antarctic SG fjords, where the M. earlandi and C. aff. parkerianus FAs are well established, than elsewhere around the Scotia Sea. Moreover, there is a disparity between FAs dominant in inner-fjord settings, which are found only in SG, and the more biogeographically widespread assemblages inhabiting outer fjords and shelf sites (Earland, 1933). This is consistent with the contrast between shallow-water SG macrofaunal communities, which show clear Antarctic characteristics, and the more geographically widespread macrofauna in surrounding deep waters which do not (Barnes et al., 2006), further emphasizing the exceptional character of the SG biota (Hogg et al., 2011).
As already indicated, benthic foraminifera can serve as valuable proxies for marine environmental conditions recorded in the geological record. It is therefore somewhat surprisingly that no attempts have been made to use them in order to reconstruct coastal environments around sub-Antarctic islands during past climatic oscillations, such as those associated with Quaternary glacial/interglacial cycles. Our results demonstrate that these microfossils have considerable potential in this regard. They seem especially suitable for studying paleoenvironmental changes in the most restricted settings proximal to tidewater glacial fronts and in shallow-water settings that are strongly affected by processes taking place on land. These may be rich in organic matter, which is believed to exert a strong control on foraminiferal assemblage composition and diversity.
The sensitivity of foraminifera to environmental changes linked to current and likely future climatic changes is of more immediate interest. Since the 1920s, there has been a 0.9–2.3 ∘C warming in the top 100 m of the water column around SG (Whitehouse et al., 2008), in addition to air temperature increases since the 1950s that have coincided with dynamic glacial retreats (Gordon et al., 2008; Cook et al., 2010). The warming around SG and in the Southern Ocean (Meredith and King, 2005) is also associated with the southward migration of the ACC (Gille, 2014) and shifts in the position and intensity of the southern westerly wind belt (Lamy et al., 2010; Perren et al., 2020). These changes, and their environmental consequences, raise questions regarding the stability of present FA distributions in SG fjords and the possible wider-scale reorganization of biogeographic patterns in the Atlantic sector of the Southern Ocean. There is already a broad overlap between foraminiferal species occurring in the fjords of SG and those located to the north and south of the ACC. Among the 60 species recognized in our study, 27 are shared with Chilean fjords (Hromic et al., 2006; Sergei Korsun et al., unpublished data) and 31 with Admiralty Bay in the South Shetland Islands (Majewski, 2005, 2010; Majewski et al., 2007). However, as discussed above, differences currently exist both at species and assemblage levels between foraminiferal faunas in these regions.
Although the data of Earland (1933) cannot be quantitatively compared with the present study due to different methodologies, they do show that all major taxa defining the FAs were present in SG during the 1920s (see also Dejardin et al., 2018). Hence, there seems to be no evidence of recent species invasions. Elsewhere, however, the biogeographic barrier of the Drake Passage and the ACC (Orsi et al., 1995) has not prevented pulses of faunal migration in and out of Antarctica, especially during past warm periods (Clarke et al., 1992; Majewski et al., 2021). The current warming will likely have major ecological consequences south of the ACC, especially around the Antarctic Peninsula, exposing this region to alien species (Fraser et al., 2018; Convey and Peck, 2019; Avila et al., 2020). This process has already begun (Fillinger et al., 2013; Griffiths et al., 2013) and may be exacerbated by human activity (Frenot et al., 2005; Hughes et al., 2020). With a warming climate and accelerating glacial retreats, conditions in South Shetland Islands and the Antarctic Peninsula will become increasingly temperate, making them more suitable for the development of foraminiferal assemblages currently typical of SG. Warming is also likely to promote natural faunal dispersal between these areas and SG, with intensified ship traffic (McCarthy et al., 2022) possibly providing an additional vector for the rapid introduction of species. Given the potential for major ecological and biogeographic readjustments in this crucial region of the globe, it is clearly important to continue monitoring for evidence of increasing faunal connectivity across the ACC and between both sides of the Drake Passage and the unique fjord environments of SG.
Data used for this study can be found in the Supplement, and are also available via https://doi.org/10.1594/PANGAEA.954898 (Majewski et al., 2023).
Tables S1 to S6 can be found in the Supplement. Scanning electron micrographs (plates) of benthic foraminiferal species are shown in Figs. S1 to S6 in the Supplement. The supplement related to this article is available online at: https://doi.org/10.5194/bg-20-523-2023-supplement.
WM and WS designed the research and conducted most of laboratory work. WM, WS, and AJG did fieldwork. WM drafted the paper and prepared the figures. AJG and WS substantially revised this work.
The contact author has declared that none of the authors has any competing interests.
Publisher's note: Copernicus Publications remains neutral with regard to jurisdictional claims in published maps and institutional affiliations.
We kindly thank Keri Pashuk, Greg Landreth, the crew of RSV Saoirse crew, and Maria Holzmann, Jan Pawlowski, and Piotr Rozwalak, for their help during the cruise to South Georgia in November–December 2019. Paul Brickle (SAERI), Alistair Crame (BAS), and Robert Bialik (IBB PAS) are thanked for help in organizing the fieldwork. Karolina Leszczyńska kindly helped with grain size analyses. We also thank the reviewers for their constructive comments that helped to improve this paper.
The study has been funded by Polish National Science Centre (grant no. 2018/31/B/ST10/02886).
This paper was edited by Tina Treude and reviewed by Katrine Husum and two anonymous referees.
Alve, E.: Opportunistic features of the foraminifer Stainforthia fusiformis (Williamson): evidence from Frierfjord, Norway, J. Micropalaeontol., 13, 24–24, https://doi.org/10.1144/jm.13.1.24, 1994.
Alve, E., Korsun, S., Schönfeld, J., Dijkstra, N., Golikova, E., Hess, S., Husum, K., and Panieri, G.: Foram-AMBI: A sensitivity index based on benthic foraminiferal faunas from North-East Atlantic and Arctic fjords, continental shelves and slopes, Mar. Micropaleontol., 122, 1–12, https://doi.org/10.1016/j.marmicro.2015.11.001, 2016.
Avila, C., Angulo-Preckler, C., Martín-Martín, R. P., Figuerola, B., Griffiths, H. J., and Waller, C. L.: Invasive marine species discovered on non–native kelp rafts in the warmest Antarctic island, Sci. Rep.-UK, 10, 1639, https://doi.org/10.1038/s41598-020-58561-y, 2020.
Barlow, N. L. M., Bentley, M. J., Spada, G., Evans, D. J. A., Hansom, J. D., Brader, M. D., White, D. A., Zander, A., and Berg, S.: Testing models of ice cap extent, South Georgia, sub-Antarctic, Quaternary Sci. Rev., 154, 157–168, https://doi.org/10.1016/j.quascirev.2016.11.007, 2016.
Barnes, D. K.: Changing chain: past, present and future of the Scotia Arc's and Antarctica's shallow benthic communities, Sci. Mar., 69, 65–89, https://doi.org/10.3989/scimar.2005.69s265, 2005.
Barnes, D. K. A., Linse, K., Waller, C., Morely, S., Enderlein, P., Fraser, K. P. P., and Brown, M.: Shallow benthic faunal communities of South Georgia Island, Polar Biol., 29, 223–228, https://doi.org/10.1007/s00300-005-0042-0, 2006.
Barnes, D. K. A., Ireland, L., Hogg, O. T., Morley, S., Enderlein P., and Sands C. J.: Why is the South Orkney Island shelf (the world's first high seas marine protected area) a carbon immobilization hotspot?, Glob. Change Biol., 22, 1110–1120, https://doi.org/10.1111/gcb.13157, 2016.
Bentley, M. J., Evans, D. J. A., Fogwill, C. J., Hansom, J. D., Sugden, D. E., and Kubik, P. W.: Glacial geomorphology and chronology of deglaciation, South Georgia, sub-Antarctic, Quaternary Sci. Rev., 26, 644–677, https://doi.org/10.1016/j.quascirev.2006.11.019, 2007.
Berg, S., White, D., Jivcov, S., Melles, M., Leng, M. J., Rethemeyer, J., Allen, C., Perren, B., Bennike, O., and Viehberg, F.: Holocene glacier fluctuations and environmental changes in Sub-Antarctic South Georgia, Quaternary Res., 91, 132–148, https://doi.org/10.1017/qua.2018.85, 2019.
Berg, S., Jivcov, S., Kusch, S., Kuhn, G., Wacker, L., and Rethemeyer, J.: Compound-specific radiocarbon analysis of (Sub-)Antarctic coastal marine sediments – potential and challenges for chronologies, Paleoceanogr. Paleoclimatol., 35, e2020PA003890, https://doi.org/10.1029/2020PA003890, 2020.
Berg, S., Jivcov, S., Kusch, S., Kuhn, G., White, D., Bohrmann, G., Melles, M., and Rethemeyer, J.: Increased petrogenic and biospheric organic carbon burial in sub-Antarctic fjord sediments in response to recent glacier retreat, Limnol. Oceanogr., 66, 4347–4362, https://doi.org/10.1002/lno.11965, 2021.
Bergstrom, D. M. and Chown, S. L.: Life at the front: history, ecology and change on southern ocean islands, Trends Ecol. Evol., 14, 472–476, https://doi.org/10.1016/S0169-5347(99)01688-2, 1999.
Berner, R. A.: Sedimentary pyrite formation: An update, Geochim. Cosmochim. Ac., 48, 605–615, https://doi.org/10.1016/0016-7037(84)90089-9, 1983.
Bernhard, J. M., Ostermann, D. R. Williams, D. S., and Blanks J. K.: Comparison of two methods to identify live benthic foraminifera: A test between Rose Bengal and CellTracker Green with implications for stable isotope paleoreconstructions, Paleoceanography and Paleoclimatology, 21, PA4210, https://doi.org/10.1029/2006PA001290, 2006.
Bianchi, T., Arndt, S., Austin, W., Benn, D. I., Bertrand., S., Cui., X., Faust., J., Koziorowska-Makuch, K., Moy, C., Savage, C., Smeaton, C., Smith, R., and Syvitski, J.: Fjords as Aquatic Critical Zones (ACZs), Earth-Sci. Rev, 203, 103–145, https://doi.org/10.1016/j.earscirev.2020.103145, 2020.
Blott, S. J. and Pye, K.: Gradistat: A grain size distribution and statistics package for the analysis of unconsolidated sediments, Earth Surf. Proc. Land., 26, 1237–1248, https://doi.org/10.1002/esp.261, 2001.
Boldt, K. V., Nittrouer, C. A., Hallet, B., Koppes, M. N., Forrest, B. K., Wellner, J. S., and Anderson, J. B.: Modern rates of glacial sediment accumulation along a 15∘ S–N transect in fjords from the Antarctic Peninsula to southern Chile, J. Geophys. Res.-Earth, 118, 2072–2088, https://doi.org/10.1002/jgrf.20145, 2013.
Brady, H. B.: Notes on some of the Reticularian Rhizopoda of the “Challenger” Expedition. Part III, J. Cell Sci., 21, 31–71, https://doi.org/10.1242/jcs.s2-21.81.31, 1881.
Capotondi, L., Bergami, C., Giglio, F., Langone, L., and Ravaioli, M.: Benthic foraminifera distribution in the Ross Sea (Antarctica) and its relationship to oceanography, Boll. Soc. Paleontol. I., 57, 187–202, https://doi.org/10.4435/BSPI.2018.12, 2018.
Carter, L., McCave, I. N., and Williams, M. J. M.: Circulation and water masses of the Southern Ocean: a review, in: Antarctic Climate Evolution, edited by: Florindo, F. and Siegert, M., Elsevier, Amsterdam, the Netherlands, 85–114, ISBN 9780444528476, 2008.
Catalá, T. S., Reche, I., Álvarez, M., Khatiwala, S., Guallart, E. F., Benítez-Barrios, V. M., Fuentes-Lema, A., Romera-Castillo, C., Nieto-Cid, M., Pelejero, C., Fraile-Nuez, E., Ortega-Retuerta, E., Marrasé, C., and Álvarez-Salgado X. A.: Water massage and aging driving chromophoric dissolved organic matter in the darkglobal ocean, Global Biogeochem. Cy., 29, 917–934, https://doi.org/10.1002/2014GB005048, 2015.
Caulle, C., Koho, K. A., Mojtahid, M., Reichart, G. J., and Jorissen, F. J.: Live (Rose Bengal stained) foraminiferal faunas from the northern Arabian Sea: faunal succession within and below the OMZ, Biogeosciences, 11, 1155–1175, https://doi.org/10.5194/bg-11-1155-2014, 2014.
Clarke, A., Crame, J. A., Stromberg, J.-O., and Barker, P. F.: The Southern Ocean benthic fauna and climate change: a historical perspective [and discussion], Philos. T. R. Soc. B, 338, 299–309, https://doi.org/10.1098/rstb.1992.0150, 1992.
Constable, A. J., Melbourne-Thomas, J., Conrey, S. P., Arrigo, K. R., Barbraud, C., Barnes, D. K., Bindoff, N. L., Boyd, P. W., Brandt, A., Costa, D. P., Davidson, A. T., Ducklow, H. W., Emmerson, L., Fukuchi, M., Gutt, J., Hindell, M. A., Hofmann, E. E., Hosie, G. W., Iida, T., Jacob, S., Johnston, N. M., Kawaguchi, S., Kokubun, N., Koubbi, P., Lea, M. A., Makhado, A., Massom, R. A., Meiners, K., Meredith, M. P., Murphy, E. J., Nicol, S., Reid, K., Richerson, K., Riddle, M. J., Rintoul, S. R., Smith Jr., W. O., Southwell, C., Stark, J. S., Sumner, M., Swadling, K. M., Takahashi, K. T., Trathan, P. N., Welsford, D. C., Weimerskirch, H., Westwood, K. J., Wienecke, B. C., Wolf-Gladrow, D., Wright, S. W., Xavier, J. C., and Ziegler, P.: Climate change and Southern Ocean ecosystems I: how changes in physical habitats directly affect marine biota, Glob. Change Biol., 20, 3004–3015, https://doi.org/10.1111/gcb.12623, 2014.
Convey, P. and Peck, L. S.: Antarctic environmental change and biological responses, Sci. Adv., 11, eaaz0888, https://doi.org/10.1126/sciadv.aaz0888, 2019.
Cook, A. J., Poncet, S., Cooper, A. P. R., Herbert, D. J., and Christie, D.: Glacier retreat on South Georgia and implications for the spread of rats, Antarct. Sci., 22, 255–263, https://doi.org/10.1017/S0954102010000064, 2010.
Curtis, M. L., Flowerdew, M. J., Riley, T. R., Whitehouse, M. J., and Daly, J. S.: Andean sinistral transpression and kinematic partitioning in South Georgia, J. Struct. Geol., 32, 464–477, https://doi.org/10.1016/j.jsg.2010.02.002, 2010.
Davenport, J.: Upwelling-generated plankton strandlines: Important predictable food sources for seabirds at Husvik, South Georgia, Mar. Biol., 123, 207–217, https://doi.org/10.1007/BF00353612, 1995.
De Nooijer, L. J., Spero, H. J., Erez, J. Bijma, J., and Reichart, G. J.: Biomineralization in perforate foraminifera, Earth-Sci. Rev., 135, 48–58, https://doi.org/10.1016/j.earscirev.2014.03.013, 2014.
Dejardin, R., Kender, S., Allen, C. S., Leng, M. J., Swann, G. E. A., and Peck, V. L.: “Live” (stained) benthic foraminiferal living depths, stable isotopes, and taxonomy offshore South Georgia, Southern Ocean: implications for calcification depths, J. Micropalaeontol., 37, 25–71, https://doi.org/10.5194/jm-37-25-2018, 2018.
Earland, A.: Foraminifera, Part II, South Georgia, Discov. Rep., 7, 27–138, 1933.
Earland A.: Foraminifera. Part III. The Falklands sector of the Antarctic (excluding South Georgia), Discov. Rep., 10, 1–208, 1934.
Echols, R. J.: Distribution of Foraminifera in sediments of the Scotia Sea area, Antarctic waters, Antarct. Res. Ser., 15, 93–168, 1971.
Fillinger, L., Janussen, D., Lundälv, T., and Richter, C.: Rapid glass sponge expansion after climate-induced Antarctic ice shelf collapse, Curr. Biol., 23, 1330–1334, https://doi.org/10.1016/j.cub.2013.05.051, 2013.
Finger, L. F. and Lipps, J. H.: Foraminiferal decimation and repopulation in an active volcanic caldera, Deception Island, Antarctica, Micropaleontology, 27, 11–139, https://doi.org/10.2307/1485283, 1981.
Fossile, E., Nardelli, M. P., Jouini, A., Lansard, B., Pusceddu, A., Moccia, D., Michel, E., Péron, O., Howa, H., and Mojtahid, M.: Benthic foraminifera as tracers of brine production in the Storfjorden “sea ice factory”, Biogeosciences, 17, 1933–1953, https://doi.org/10.5194/bg-17-1933-2020, 2020.
Fraser, C. I., Morrison, A. K., Hogg, A. M., Macaya, E. C., van Sebille, E., Ryan, P. G., Padovan, A., Jack, C., Valdivia, N., and Waters, J. M.: Antarctica's ecological isolation will be broken by storm-driven dispersal and warming, Nat. Clim. Change, 8, 704–708, https://doi.org/10.1038/s41558-018-0209-7, 2018.
Frenot, Y., Convey, P., Lebouvier, M., Chown, S. L., Whinam, J., Selkirk, P. M., Convey, P., Skotnicki, M., and Bergstrom, D. M.: Biological invasions in the Antarctic: extent, impacts and implications, Biol. Rev., 80, 45–72, https://doi.org/10.1017/s1464793104006542, 2005.
Geprägs, P., Torres, M. E., Mau, S., Kasten, S., Römer, M., and Bohrmann, G.: Carbon cycling fed by methane seepage at the shallow Cumberland Bay, South Georgia, sub-Antarctic, Geochem. Geophy. Geosy., 17, 1401–1418, https://doi.org/10.1002/2016GC006276, 2016.
Gille, S. T.: Meridional displacement of the Antarctic Circumpolar Current, Philos. T. R. Soc. A, 372, 20130273, https://doi.org/10.1098/rsta.2013.0273, 2014.
Gooday, A. J., Holzmann, M., Majewski, W., and Pawlowski, J.: New species of Gromia (Protista, Rhizaria) from South Georgia and the Falkland Islands, Polar Biol., 45, 647–666, https://doi.org/10.1007/s00300-022-03017-4, 2022.
Gordon, J. E., Haynes, V. M., and Hubbard, A.: Recent glacier changes and climate trends on South Georgia, Global Planet. Change, 60, 72–84, https://doi.org/10.1016/j.gloplacha.2006.07.037, 2008.
Graham, A. G., Fretwell, P. T., Larter, R. D., Hodgson, D. A., Tate, A. J., Morris, P., and Wilson, C. K.: A new bathymetric compilation highlighting extensive paleo-ice sheet drainage on the continental shelf, South Georgia, sub-Antarctica, Geochem. Geophy. Geosy., 9, Q07011, https://doi.org/10.1029/2008GC001993, 2008.
Graham, A. G. C., Kuhn, G., Meisel, O., Hillenbrand, C.-D., Hodgson, D. A., Ehrmann, W., Wacker, L., Wintersteller, P., Dos Santos Ferreira, C., Römer, M., White, D., and Bohrmann, G.: Major advance of South Georgia glaciers during the Antarctic Cold Reversal following extensive sub-Antarctic glaciation, Nat. Commun., 8, 14798, https://doi.org/10.1038/ncomms14798, 2017.
Gray, S. C., Sturz, A., Bruns, M. D., Marzan, R. L., Dougherty, D., Law, H. B., Brackett, J. E., and Marcou, M.: Composition and distribution of sediments and benthic foraminifera in a submerged caldera after 30 years of volcanic quiescence, Deep-Sea Res. Pt. II, 50, 1727–1751, https://doi.org/10.1016/S0967-0645(03)00090-0, 2003.
Griffiths, H. J., Whittle, R. J., Roberts, S. J., Belchier, M., and Linse, K.: Antarctic Crabs: Invasion or Endurance?, PLOS ONE, 8, e66981, https://doi.org/10.1371/journal.pone.0066981, 2013.
Gschwend, F., Majda, A., Majewski, W., and Pawlowski, J.: Psammophaga fuegia sp. nov., a new monothalamid foraminifer from the Beagle Channel, South America, Acta Protozool., 55, 101–110, https://doi.org/10.4467/16890027AP.16.009.4944, 2016.
Habura, A., Goldstein, S. T., Parfrey, L. W., and Bowser, S. S.: Phylogeny and ultrastructure of Miliammina fusca: Evidence for secondary loss of calcification in a miliolid foraminifer, J. Eukaryot. Microbiol., 53, 204–210, https://doi.org/10.1111/j.1550-7408.2006.00096.x, 2006.
Hald, M. and Korsun, S.: Distribution of modern benthic foraminifera from fjords of Svalbard, European Arctic, J. Foramin. Res., 27, 101–122, https://doi.org/10.2113/gsjfr.27.2.101, 1997.
Hammer, Ø., Harper, D. A. T., and Ryan, P. D.: Past: Paleontological statistics software package for education and data analysis, Palaeontol. Electron., 4, 1–9, 2001.
Hayward, B. W. and Hollis, C.: Brackish Foraminifera in New Zealand: a taxonomic and ecologic review, Micropaleontology, 40, 185–222, https://doi.org/10.2307/1485816, 1994.
Hayward, B. W., Cedhagen, T,. Kaminski, M., and Gross, O.: WoRMS Foraminifera: World Foraminifera Database (version 2016-03-01), in: Species 2000 & ITIS Catalogue of Life, 2016 Annual Checklist, edited by: Roskov, Y., Abucay, L., Orrell, T., Nicolson, D., Flann, C., Bailly, N., Kirk, P., Bourgoin, T., DeWalt, R. E., Decock, W., and De Wever, A., https://www.catalogueoflife.org/annual-checklist/2016 (last access: 22 May 2022), Naturalis, Leiden, the Netherlands, 2021.
Herb, R.: Distribution of recent benthonic foraminifer in the Drake Passage, Antarct. Res. Ser., 17, 251–300, https://doi.org/10.1029/AR017p0251, 1971.
Heron-Allen, E. and Earland, A.: Some new foraminifera from the South Atlantic. Part 2, J. Roy. Micr. Soc., 49, 324–334, https://doi.org/10.1111/j.1365-2818.1929.tb00787.x, 1929.
Heron-Allen, E. and Earland, A.: Some new foraminifera from the South Atlantic. Part 3, Miliammina, a new siliceous genus, J. Roy. Micr. Soc. 30, 38–45, https://doi.org/10.1111/j.1365-2818.1930.tb01474.x, 1930.
Heron-Allen, E. and Earland, A.: Foraminifera. Part I. The ice-free area of the Falkland Islands and adjacent seas, Discov. Rep., 4, 291–460, 1932.
Hodgson, D. A., Graham, A. G., Griffiths, H. J., Roberts, S. J., Cofaigh, C. O., Bentley, M. J., and Evans, D. J.: Glacial history of sub-Antarctic South Georgia based on the submarine geomorphology of its fjords, Quaternary Sci. Rev., 89, 129–147, https://doi.org/10.1016/j.quascirev.2013.12.005, 2014.
Hogg, O. T., Barnes, D. K. A., and Griffiths, H. J.: Highly diverse, poorly studied and uniquely threatened by climate change: an assessment of marine biodiversity on South Georgia's continental shelf, PLoS ONE, 6, e19795, https://doi.org/10.1371/journal.pone.0019795, 2011.
Höglund, H.: Foraminifera in the Gullmar Fjord and the Skagerak, Zool. Bidr. Upps., 26, 1–328, 1947.
Holzmann, M., Gooday, A. J., Majewski, W., and Pawlowski, J.: Molecular and morphological diversity of monothalamous foraminifera from South Georgia and the Falkland Islands: description of four new species, Eur. J. Protistol., 85, 125909, https://doi.org/10.1016/j.ejop.2022.125909, 2022.
Hromic, T., Ishman, S., and Silva, N.: Benthic foraminiferal distributions in Chilean fjords: 47∘ S to 54∘ S, Mar. Micropaleontol., 59, 115–134, https://doi.org/10.1016/j.marmicro.2006.02.001, 2006.
Hughes, K. A., Pescott, O. L., Peyton, J., Adriaens, T., Cottier-Cook, E. J., Key, G., Rabitsch, W., Tricario, E., Barnes, D. K. A., Baxter, N., Belchier, M., Blake, D., Convey, P., Dawson, W., Frohlich, D., Gardiner, L. M., Gonzalez-Moreno, P., James, R., Malumphy, C., Martin, S., Martinou, A. F., Minchin, D., Monaco, A., Moore, N., Morley S. A., Ross, K., Shanklin, J., Turvey, K., Vaughan, D., Vaux, A. G. C., Werenkraut, V., Winfield, I. J., and Roy, H. E.: Invasive non-native species likely to threaten biodiversity and ecosystems in the Antarctic Peninsula region, Glob. Change Biol., 26, 2702–2716, https://doi.org/10.1111/gcb.14938, 2020.
Ishman, S. E. and Domack, E. W.: Oceanographic controls on benthic foraminifers from the Bellingshausen margin of the Antarctic Peninsula, Mar. Micropaleontol., 24, 119–155, https://doi.org/10.1016/0377-8398(94)90019-1, 1994.
Ishman, S. E. and Sperling, M. R.: Benthic foraminiferal record of Holocene deep-water evolution in the Palmer Deep, western Antarctic Peninsula, Geology 30, 435–438, https://doi.org/10.1130/0091-7613(2002)030<0435:BFROHD>2.0.CO;2, 2002.
Ishman, S. E. and Szymcek, P.: Foraminiferal distributions in the former Larsen-A Ice Shelf and Prince Gustav Channel region, eastern Antarctic Peninsula margin: a baseline for Holocene paleoenvironmental interpretation, Antarct. Res. Ser., 79, 239–260, https://doi.org/10.1029/AR079p0239, 2003.
Jaeger, J. M. and Nittrouer, C. A.: Sediment deposition in an Alaskan fjord: Controls on the formation and preservation of sedimentary structures in Icy Bay, J. Sediment. Res., 69, 1011–1026, https://doi.org/10.2110/jsr.69.1011, 1999.
Jennings, A. E. and Helgadottir, G.: Foraminiferal assemblages from the fjords and shelf of eastern Greenland, J. Foramin. Res., 24, 123–144, https://doi.org/10.2113/gsjfr.24.2.123, 1994.
Jennings, A. E., Weiner, N. J., Helgadottir, G., and Andrews, J. T.: Modern foraminiferal faunas of the southwestern to northern Iceland shelf: oceanographic and environmental controls, J. Foramin. Res. 34, 180–207, https://doi.org/10.2113/34.3.180, 2004.
Jernas, P., Klitgaard-Kristensen, D., Husum, K., Koç, N., Tverberg, V., Loubere, P., Prins, M., Dijkstra, N., and Gluchowska, M.: Annual changes in Arctic fjord environment and modern benthic foraminiferal fauna: Evidence from Kongsfjorden, Svalbard, Global Planet. Change, 163, 119–140, https://doi.org/10.1016/j.gloplacha.2017.11.013, 2018.
Jorissen, F. J., Bicchi, E., Duchemin, G., Durrieu, J., Galgani, F., Cazes, L., Gaultier, M., and Camps, R.: Impact of oil-based drill mud disposal on benthic foraminiferal assemblages on the continental margin off Angola, Deep-Sea Res. Pt. II, 56, 2270–2291, https://doi.org/10.1016/j.dsr2.2009.04.009, 2009.
Kennett, J. P.: The fauna of the Ross Sea. Part 6. Ecology and distribution of the Foraminifera, New Zealand DSIR Bull., 186, 1–46, 1968.
Korsun, S. and Hald, M.: Modern benthic foraminifera off Novaya Zemlya tidewater glaciers, Russian Arctic, Arct. Antarct. Alp. Res., 30, 61–77, https://doi.org/10.2307/1551746, 1998.
Lamy, F., Kilian, R., Arz, H. W., Francois, J. P., Kaiser, J., Prange, M., and Steinke, T.: Holocene changes in the position and intensity of the southern Westerly wind belt, Nature Geosci., 3, 695–699, https://doi.org/10.1038/NGEO959, 2010,.
Lloyd, J. M. and Evans, J. R.: Contemporary and fossil foraminifera from isolation basins in northwest Scotland, J. Quaternary Sci., 17, 431–443, https://doi.org/10.1002/jqs.719, 2002.
Loeblich, A. R. and Tappan, H.: Revision of some recent foraminiferal genera, Smithsonian miscellaneous collections, 128, 1–37, 1955.
Loeblich, A. R. and Tappan, H. (Eds.): Foraminiferal Genera and their Classification, Van Nostrand Reinhold Company, New York, USA, ISBN 9780442259372, 1988.
Mackensen, A.: Oxygen and carbon stable isotope tracers of Weddell Sea water masses: new data and some paleoceanographic implications, Deep-Sea Res. Pt. I, 48, 1401–1422, https://doi.org/10.1016/S0967-0637(00)00093-5, 2001.
Mackensen, A., Grobe, H., Kuhn, G., and Fütterer, D. K.: Benthic foraminiferal assemblages from the eastern Weddell Sea between 68 and 73∘ S: distribution, ecology and fossilization potential, Mar. Micropaleontol., 16, 241–283, https://doi.org/10.1016/0377-8398(90)90006-8, 1990.
Mackensen, A., Fütterer, D. K., Grobe, H., and Schmiedl, G.: Benthic foraminiferal assemblages from the eastern South Atlantic Polar Front region between 35∘ and 57∘ S: Distribution, ecology and fossilization potential, Mar. Micropaleontol., 22, 33–69, https://doi.org/10.1016/0377-8398(93)90003-G, 1993.
Majda, A., Majewski, W., Mamos, T., Grabowski, M., Godoi, M. A., and Pawlowski, J.: Variable dispersal histories across the Drake Passage: The case of coastal benthic Foraminifera, Mar. Micropaleontol., 140, 81–94, https://doi.org/10.1016/j.marmicro.2018.02.004, 2018.
Majewski, W.: Benthic foraminiferal communities: distribution and ecology in Admiralty Bay, King George Island, West Antarctica, Pol. Polar Res., 26, 159–214, 2005.
Majewski, W.: Benthic foraminifera from West Antarctic fiord environments: An overview, Pol. Polar Res., 31, 61–82, https://doi.org/10.4202/ppres.2010.05, 2010.
Majewski, W.: Benthic foraminifera from Pine Island and Ferrero bays, Amundsen Sea, Pol. Polar Res., 34, 169–200, 2013.
Majewski, W. and Tatur, A.: A new Antarctic foraminiferal species for detecting climate change in sub-Recent glacier-proximal sediments, Antarct. Sci., 5, 439–448, https://doi.org/10.1017/S0954102009990150, 2009.
Majewski, W., Lecroq, B., Sinniger, F., and Pawlowski, J.: Monothalamous foraminifera from Admiralty Bay, King George Island, West Antarctica, Pol. Polar Res., 28, 187–210, 2007.
Majewski, W., Wellner, J. S., Szczuciński, W., and Anderson, J. B.: Holocene oceanographic and glacial changes recorded in Maxwell Bay, West Antarctica, Mar. Geol., 326–328, 67–79, https://doi.org/10.1016/j.margeo.2012.08.009, 2012.
Majewski, W., Wellner, J. S., and Anderson, J. B.: Environmental connotations of benthic foraminiferal assemblages from coastal West Antarctica, Mar. Micropaleontol., 124, 1–15, https://doi.org/10.1016/j.marmicro.2016.01.002, 2016.
Majewski, W., Stolarski, J., and Bart, P. J.: Two rare pustulose/sponose morphotypes of benthic foraminifera from eastern Ross Sea, J. Foramin. Res. 49, 405–422, https://doi.org/10.2113/gsjfr.49.4.405, 2019.
Majewski, W., Holzmann, M., Gooday, A. J., Majda, A., Mamos T., and Pawlowski J.: Cenozoic climatic changes drive evolution and dispersal of coastal benthic foraminifera in the Southern Ocean, Sci. Rep.-UK, 11, 19869, https://doi.org/10.1038/s41598-021-99155-6, 2021.
Majewski, W., Szzczuciński, W., and Gooday, A. J.: Benthic foraminifera (stained) in sub-Antarctic fjords of South Georgia, PANGAEA [data set], https://doi.org/10.1594/PANGAEA.954898, 2023.
Malmgren, B. A. and Haq, B. U.: Assessment of quantitative techniques in paleobiogeography, Mar. Micropaleontol., 7, 213–236, https://doi.org/10.1016/0377-8398(82)90003-2, 1982.
McCarthy, A. H., Peck, L. S., and Aldridge, D. C.: Ship traffic connects Antarctica's fragile coasts to worldwide ecosystems, P. Natl. Acad. Sci. USA, 119, e2110303118, https://doi.org/10.1073/pnas.2110303118, 2022.
Meredith, M. P. and King, J. C.: Rapid climate change in the ocean west of the. Antarctic Peninsula during the second half of the 20th century, Geophys. Res. Lett., 32, L19604, https://doi.org/10.1029/2005GL024042, 2005.
Meredith, M. P., Watkins, J. L., Murphy, E. J., Ward, P., Bone, D. G., Thorpe, S. E., Grant, S. A., and Ladkin, R. S.: Southern ACC front to the northeast of South Georgia: Pathways, characteristics, and fluxes, J. Geophys. Res., 108, 3162, https://doi.org/10.1029/2001JC001227, 2003.
Milam, R. W and Anderson, J. B.: Distribution and ecology of recent benthonic foraminifera of the Adelie–George V continental shelf and slope, Antarctica. Mar. Micropaleontol., 6, 297–325, https://doi.org/10.1016/0377-8398(81)90009-2, 1981.
Murray, J. W. (Ed.): Ecology and palaeoecology of benthic foraminifera, Logman Scientific & Technical, London, UK, https://doi.org/10.4324/9781315846101, 1991.
Murray, J. W. and Pudsey, C. J.: Living (stained) and dead foraminifera from the newly ice-free Larsen Ice Shelf, Weddell Sea, Antarctica: Ecology and taphonomy, Mar. Micropaleontol., 53, 67–81, https://doi.org/10.1016/j.marmicro.2004.04.001, 2004.
Nomura, R.: Foraminifera from the raised beach deposits on the east coast of Lützow–Holm Bay, Antarctica, Mem. Natl. Inst. Polar Res., 28, 219–230, 1983.
Orsi, A. H., Whitworth, T., and Nowlin, W. D.: On the meridional extent and fronts of the Antarctic Circumpolar Current, Deep-Sea Res. Pt. I, 42, 641–673, https://doi.org/10.1016/0967-0637(95)00021-W, 1995.
Perren, B. B., Hodgson, D. A., Roberts, S. J., Sime, L., Van Nieuwenhuyze, W., Verleyen, E., and Vyverman, W.: Southward migration of the southern Hemisphere westerly winds corresponds with warming climate over centennial timescales, Commun. Earth Environ., 1, 58, https://doi.org/10.1038/s43247-020-00059-6, 2020.
Racka, M., Marynowski, L., Filipiak, P., Sobstel, M., Pisarzowska, A., and Bond, D. P. G.: Anoxic Annulata Events in the Late Famennian of the Holy Cross Mountains (Southern Poland): Geochemical and palaeontological record, Palaeogeogr. Palaeocl., 297, 549–575, https://doi.org/10.1016/j.palaeo.2010.08.028, 2010.
Rodrigues, A. R., Maluf, J. C. C., De Santis Braga, E., and Eichler, B. B.: Recent benthic foraminiferal distribution and related environmental factors in Ezcurra Inlet, King George Island, Antarctica, Antarct. Sci., 22, 343–360, https://doi.org/10.1017/S0954102010000179, 2010.
Römer, M., Torres, M., Kasten, S., Kuhn, G., Graham, A. G. C., Mau, S., Little, C. T., Linse, K., Pape, T., Geprägs, P., Fischer, D., Wintersteller, P., Marcon, Y., Rethemeyer, J., Bohrmann, G., and shipboard scientific party ANT-XXIX/4: First evidence of widespread active methane seepage in the Southern Ocean, off the sub-Antarctic island of South Georgia, Earth Planet. Sc. Lett., 403, 166–177, https://doi.org/10.1016/j.epsl.2014.06.036, 2014.
Schönfeld, J., Alve, E., Geslin, E., Jorissen, F., Korsun, S., and Spezzaferri, S.: The FOBIMO (Foraminiferal BIo-MOnitoring) initiative – towards a standardised protocol for soft bottom benthic foraminiferal monitoring studies, Mar. Micropaleontol., 94–95, 1–13, https://doi.org/10.1016/j.marmicro.2012.06.001, 2012.
Sen Gupta, B. K. (Ed.): Foraminifera in marginal marine environments, in: Modern Foraminifera, Kluwer Academic Publishers, Dordrecht, the Netherlands, 141–160, https://doi.org/10.1007/0-306-48104-9_9, 1999.
Silva K. A., Corliss B. H., Rathburn A. E., and Thunell R. C.: Seasonality of living benthic foraminifera from the San Pedro Basin, California Borderland, J. Foramin. Res., 26, 71–93, https://doi.org/10.2113/gsjfr.26.1.71, 1996.
Smith, J.: Glacier problems in South Georgia, J. Glaciol., 3, 705–714, https://doi.org/10.3189/S0022143000018001, 1960.
Streuff, K., Forwick, M., Szczuciński, W., Andreassen, K., and Ó Cofaigh, C.: Submarine landform assemblages and sedimentary processes related to glacier surging in Kongsfjorden, Svalbard, Arktos, 1, 14, https://doi.org/10.1007/s41063-015-0003-y, 2015.
Syvitski, J. P. M.: On the deposition of sediment within glacier-influenced fjords: oceanographic controls, Mar. Geol., 85, 301–329, https://doi.org/10.1016/0025-3227(89)90158-8, 1989.
Szczuciński, W. and Zajączkowski, M.: Factors controlling downward fluxes of particulate matter in glacier-contact and non-glacier contact settings in a subpolar fjord (Billefjorden, Svalbard), IAS Spec. Publ., 44, 369–385, https://doi.org/10.1002/9781118311172.ch18, 2012.
Thiede, J., Ovale, G., Skarbø, O., and Strand, J. E.: Benthonic foraminiferal distributions in a southern Norwegian fjord system: A re-evaluation of Oslo Fjord data, IAS Spec. Publ., 5, 469–495, https://doi.org/10.1002/9781444303759.ch33, 1981.
Thorpe, S. E., Heywood, K. J., Brandon, M. A., and Stevens, D. P.: Variability of the southern Antarctic Circumpolar Current front north of South Georgia, J. Mar. Syst., 37, 87–105, https://doi.org/10.1016/S0924-7963(02)00197-5, 2002.
Tyszka, J.: Miliammina gerochi n. sp. – a middle Jurassic rzehakinid (Foraminiferida) from quasi-anaerobic biofacies, Ann. Soc. Geol. Pol., 67, 355–364, 1997.
Violanti, D., Loi, B., and Melis, R.: Recent foraminifera from the Strait of Magellan: first quantitative data, Boll. Mus. Regionale. Sci. Nat. Torino, 17, 511–539, 2000.
White, D. A., Bennike, O., Melles, M., Berg, S., and Binnie, S. A.: Was South Georgia covered by an ice cap during the Last Glacial Maximum?, Geol. Soc. Spec. Publ., 461, 49, https://doi.org/10.1144/SP461.4, 2018.
Whitehouse, M. J., Meredith, M. P., Rothery, P., Atkinson, A., Ward, P., and Korb, R. E.: Rapid warming of the ocean around South Georgia, Southern Ocean, during the 20th century: Forcings, characteristics and implications for lower trophic levels, Deep-Sea Res. Pt. I, 55, 1218–1228, https://doi.org/10.1016/j.dsr.2008.06.002, 2008.
Zajączkowski, M., Szczuciński, W., Plessen, B., and Jernas, P.: Benthic foraminifera in Hornsund, Svalbard – Implications for paleoenvironmental reconstructions, Pol. Polar Res., 31, 349–375, 2010.