the Creative Commons Attribution 4.0 License.
the Creative Commons Attribution 4.0 License.
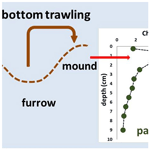
Bottom fishery impact generates tracer peaks easily confused with bioturbation traces in marine sediments
Stefan Forster
Claudia Runkel
Josephin Lemke
Laura Pülm
Martin Powilleit
In the process of reworking sediments and thus shaping biogeochemical processes, marine bottom-dwelling animals are thought to play a pivotal role in many benthic environments. Bioturbation (particle reworking) includes the downward transport of particles into the sediment as a major process and is sometimes detected as subsurface maxima (peaks) of specific particulate substances (tracers). Here, we document the fact that subsurface peaks, such as those typically attributed to biological particle transport in sediments, may equally be generated by otter boards in bottom-trawling fishery. Boards can generate tracer peaks whereby they scoop sediment from the surface, flip it over, and deposit it onto the adjacent seafloor. These peaks are indistinguishable from those generated by benthic fauna burying surface material at sediment depth. We demonstrate this for the particle tracer chlorophyll a in silty sand from the western Baltic Sea with fauna that generally do not burrow deep in a global comparison. Our inability to distinguish the driving processes generating the peaks indicates limits to our understanding of the magnitude and spatial extent of bioturbation traces in this environment. It also poses a problem for the assessment of fishery resource use and benthic processes. However, based on natural fauna abundance, behavioral information, and fishery intensity data, we identify macrofauna and not otter boards as the dominant cause of peaks at the sites investigated here.
- Article
(1721 KB) - Full-text XML
- BibTeX
- EndNote
Bottom trawling introduces anthropogenic disturbance to the seafloor. Research addressing different aspects of this activity is accumulating, for it causes partial destruction of benthic habitats and their biota (Sparks-McConkey and Watling, 2001; Watling and Norse, 1998), alters sediment structure both physically and in its granulometry (Oberle et al., 2016a; Bradshaw et al., 2012, 2021), suspends finer grain sizes from the bulk sediment, and may affect contaminant deposits (Oberle et al., 2016b). Trawling affects numerous ecosystem functions, such as carbon storage (Epstein et al., 2022) and sediment integrity (de Juan et al., 2015), and additionally interacts with other pressures on the benthic ecosystem, such as contaminant deposits and hypoxia (Oberle et al., 2016b; Bunke et al., 2019; van Denderen et al., 2022).
Investigations aiming to detect and quantify the effects of fishing gear at the seafloor face the difficulty that patterns may also stem from disturbances, natural or anthropogenic, other than trawling (Bunke et al., 2019). Deep-reaching storms, particle reworking by bioturbating fauna, construction, and dredging activity leave traces of disturbance at the seafloor as well.
Localization of the impact on the seafloor and sampling also pose a major problem. Both usually take place with limited spatial precision, which is why the majority of studies rely on statistically capturing average effects in areas of certain trawling intensities. Measures such as the swept-area ratio (SAR) of fishing intensity remain inaccurate in that they average bottom trawling over long periods (per year or per quarter) and relate the impact to comparatively large areas (several square kilometers). However, the damage to surface sediment by otter boards may be local. Studies investigating the change in the vertical distribution of sediment constituents demonstrate that an effect of trawling can be the removal of surface sediment and considerable alterations to matter concentrations and processes in this sediment surface layer (Mestdagh et al., 2018; van de Velde et al., 2018; Morys et al., 2021). In an experimental dredge-trawling activity, Morys et al. (2021) found that sediment excavated to 2.5–3 cm depth piled up irregularly on the sides of the track.
Particle reworking by bioturbating organisms is an important aspect of transport for substances at and just below the sediment–water interface. While specific motions associated with their way of life (burrow construction, feeding, defecation) move particles in all spatial directions at vastly different time intervals and over very different distances at any one time, the macroscopic pattern of the sum of these individual reworking events is mostly dealt with in simplified ways. A common differentiation describes numerous and small (“local”) transport steps as an erratic, non-directional mixing process (analogous to diffusion) and observes “non-local” transports when directional transport over longer distances takes place and creates peaks in vertical concentration profiles of particles in the sediment (e.g., when chlorophyll is used as a particle tracer).
The interpretation of peaks in stable-tracer distributions (glass beads, luminophores) or decaying-tracer distributions (radioisotopes, chlorophyll) as signs of non-local transport is widespread (Wheatcroft et al., 1994; Blair et al., 1996; Meysman et al., 2003; Morys et al., 2016, 2017; Oberle et al., 2016b). Peaks are observed regardless of the persistence of the tracers used, whether in stable-tracer distributions or distributions of tracers that decay with time. Natural decay of the tracer chlorophyll allows us to “look back in time” by 100–150 d to observe when its peak concentration declined to 25 % of its original value. Decay thus determines whether a peak will remain visible or if the event merges into the overall mixing, which is usually dealt with as a diffusion analog. Chlorophyll as a particle tracer can therefore show relatively recent events of particle mixing only. Compiling data on the frequency of use and geographic coverage of studies employing different tracers, Solan et al. (2019) showed that next to radioisotopes, the naturally occurring chlorophyll-a molecule (chl a) is commonly used.
Transport of particles in sediments potentially always implies changes in availability, concentration or distribution of food (organic particles), contaminants, and oxidizing agents such as iron oxides, with potential effects on carbon burial and inorganic nutrient release, including potential feedback of these rate changes on bioturbating macrofauna (Epstein et al., 2022; de Borger et al., 2021; van Denderen et al., 2022). It additionally affects dissolved-electron-acceptor distribution, as concomitant fluid movement is inevitable. This fosters the interest in bioturbation as an important regulator of bacterial activity and diagenesis (Aller, 2014).
In the framework of research on impacts of trawling in the Fehmarn Belt (FB) area, located in the western Baltic Sea (Fig. 1) (https://www.io-warnemuende.de/dam-mgf-baltic-sea-home.html, last access: 11 April 2024), we measured the depth distribution of chl a in order to study bioturbation. The western Baltic Sea also harbors seafloors in the most intensively trawled areas of the world (Amoroso et al., 2018). Trawl tracks in this area have been thoroughly analyzed (Schönke et al., 2022), and their biogeochemical signals have been interpreted by Rooze et al. (2024). Chlorophyll peaks detected here are usually attributed to Arctica islandica, the dominant reworking bivalve, or other biota in the community.
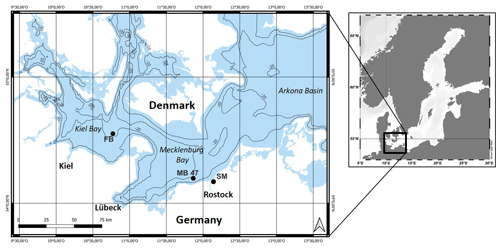
Figure 1Locations in the western Baltic Sea where (1) random sampling revealed subsurface tracer peaks in the field (FB), (2) an in situ trawling experiment was performed (MB 47), and (3) sediment was retrieved for an ex situ mesocosm experiment (SM).
The ocean quahog, Arctica islandica, lives just below the sediment surface, where it maintains contact with seawater via its short siphon (Winter, 1969). A. islandica is classified as a surface biodiffusor (also known as a surficial modifier/biodiffusor; Queirós et al., 2013) based on its surface-dwelling activity. Its activity causes constant and random local transport of particles over short distances in the uppermost centimeters of the sediment (Kristensen et al., 2012; Queirós et al., 2013). The species also shows a behavior known as “survival by metabolic suppression”, induced by hypoxia, when it burrows to deeper horizons. Therefore, the ocean quahog is also considered a “downward conveyor” that translocates particles to depth by non-local transport (Kristensen et al., 2012; Morys et al., 2017). In the Mecklenburg Bay, A. islandica accounts for up to 99 % of the biomass, representing the most important species below the halocline (Morys et al., 2017).
However, intense trawling raises the question of an alternative origin of the peaks. Previous studies (Oberle et al., 2016b), underwater (UW) video evidence, and a possible analogy with terrestrial soil turnover during plowing triggered the idea of surface sediment subduction by otter boards. To our knowledge, there is no evidence of this transport process in the literature.
Anticipating sediment displacement similar to Morys et al. (2021), we conducted an ex situ experiment to mimic otter board effects at the sediment–water interface. We subsequently performed an in situ experiment with trawling and immediate targeted sampling by scuba divers. Our aims were to (i) mimic the genesis of altered chl-a distributions experimentally, (ii) compare these distributions with peaks found in the field and potentially originating from commercial trawling, and finally (iii) discuss the likelihood and consequences of confusion between these peaks and those generated by bioturbation.
We performed experiments ex situ in a mesocosm and in situ by setting trawl marks to provide a proof of principle for the operation of the mechanisms. We investigated the changes in particle distribution brought about by the mechanical impact of otter trawling.
2.1 Ex situ experiment
In April 2021, we simulated the mechanical trawling impact on a small scale in a mesocosm using a shovel and a rake. The mesocosm consisted of a circular aquarium (0.8 m diameter, 1 m height) which had been filled 3 months prior with sandy sediment to reconstruct a horizontally homogeneous, vertically declining chl-a distribution. The lowest sediment layer consisted of 15 cm of sieved sand (0.5 mm) that had been stored in the dark for > 3 months. This was overlain by 8 cm of freshly sieved sediment from the field site “Schnatermann” (SM, Fig. 1) that had been removed from 2–10 cm depth, excluding the upper 2 cm of surface layer sediment with much microphytobenthos at this shallow location (SM; 0.5 m water depth). Finally, the uppermost layer in the mesocosm contained 2 cm of sieved surface material from that field site, harboring a rich microphytobenthos community. The upper 10 cm of sediment consisted of silty fine sand (median diameter: 190 µm) similar to the sediment at the in situ experimental site. The mesocosm stood outside the university buildings at ambient light and temperature levels (temperature range: 2 to 16 °C) and was covered by 15 cm of water (10 psu).
On 8 April 2021, we manipulated the surface sediment with a shovel (∼8 cm wide) and a rake according to the scheme in Fig. 2. We decided to excavate sediment while maintaining a defined geometry of depth and width when removing sediment rather than use a plow-like tool which we found difficult to implement. In fact, we are not sure about the exact mechanism and geometry of sediment removal and the deposition of sediment onto the adjacent sediment by an otter board; therefore, the method used in the mesocosm is a surrogate and not necessarily the same as the physical process that might be active in situ. We excavated sediment with a flat rectangular shovel, scoop by scoop, to about 4 cm depth from the surface (track leading along sediment core positions 6–10) and deposited it upside down to the left onto the adjacent sediment surface (core positions 11–15), as labeled in Fig. 2. The resulting “furrow” and “mound”, measuring about 40 cm in length, showed a surface topography with a wave-like cross section, as shown in Fig. 2 (dashed line). We also pulled a rake through the sediment along core positions 1–5, mixing the sediment to ∼ 2 cm depth while slowly moving up and down. This was to mimic the impact of the trawl net with its footrope (“net”). Immediately after the manipulation, sampling started with five 36 mm inner-diameter acrylic cores, which were taken in rows along each of the structures created (core positions 1–15), and controls were randomly placed across the unaffected area (core positions 16–20).
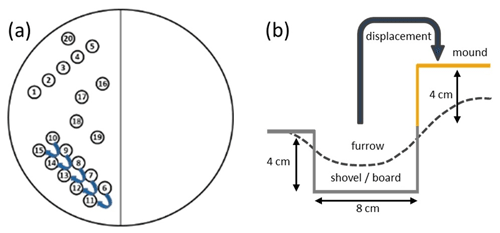
Figure 2(a) The sampling scheme is presented on the left side of the mesocosm; on the right side, a corresponding experiment including bioturbating organisms is not addressed here. Cores are presented as follows: 1–5 (net), 6–10 (furrow), 11–15 (mound), and 16–20 (control). Arrows indicate sediment removal and deposition. (b) The schematic of sediment excavation. The dashed line indicates the final surface topography.
2.2 In situ experiment
On 19 June 2021, a small otter-trawling activity typical for the area was performed at 20 m water depth at approximately 54°12′ N and 11°52′ E (MB 47) by RV Solea, while RV Limanda and further research vessels conducted a series of associated measurements and sampling (Fig. 1). The site at 20.3 m depth is inhabited by Arctica islandica (46 individuals m−2) in much the same way as the investigation area, the Fehmarn Belt (see below). Sediments consisted of silty fine sand (median diameter 180 µm). Scuba divers sampled the net area (which mimics the effect of footrope) in one trawl track, and control cores were taken in the recently untrawled vicinity that same day. Five 36 mm inner-diameter cores were taken randomly within 1 m2 in both areas. In a second trawl track, sampling by scuba diving occurred from furrow and mound areas. Here, cores were inserted along the axis of the shallow furrow carved by the otter board (Fig. 3). Material excavated by the board lay in irregular piles on the outer rim of the furrow. Cores were inserted along the highest ridge of these piles, parallel to the direction of the furrow.
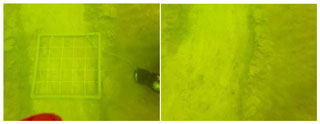
Figure 3UW photographs of an otter board track that is visible as light sediment with a depth of approximately 4 cm (counting square: 50 cm width). The material displaced from the furrow is visible as a mound with transversal cracks on the right-hand side of the lighter furrow (right photo). Photographs courtesy of Holger Pielenz.
2.3 Field data
In the Fehmarn Belt area, random sampling by a multiple corer (MUC) was performed in 2020 and subsequent years for chl-a depth distribution to describe the bioturbation activity. Cores (10 cm diameter) were sliced, slices were homogenized, and samples were treated as described below.
The site is characterized by muddy fine sand (median diameter: 50 µm; Corg: 5.5 % dw) (Gogina and Feldens, 2020) and is dominated by A. islandica (43 individuals m−2). Sampling of the benthic macrofauna from 30 grab hauls was performed using a Van Veen grab (75 kg, sieve lid) with a sampling area of 0.1 m2 and sieving (0.5 mm). Samples were preserved with a 4 % formaldehyde seawater solution.
2.4 Processing
Processing took place within 3 h after core retrieval. Sediment was carefully extruded from the tube and cut into intervals of 0.5 cm to 2 cm depth, followed by 1 cm slices to 8 cm depth and 2 cm slices to a final depth of 12 cm. Layers were homogenized with a spatula in Petri dishes. Then, 1 cm3 of sediment was subsampled using a cut syringe and placed in a centrifuge tube for storage at −18 °C. For pigment extraction, samples were thawed, mixed with 8 mL of > 96 % denatured ethanol (Walter CMP), and vortexed for 5 s. They were then extracted in the dark at ∼ 7 °C for 22–24 h with one additional mixing step several hours into the extraction. Prior to the measurement, samples were centrifuged at 2000 rpm for 4 min (∼ 690 g). Chlorophyll fluorescence was measured (Turner Designs Trilogy fluorometer, CHLA module), with additional acidification prior to the determination of phaeopigments (50 µL of 1 N HCl). Chl-a concentrations (µg cm−3) were calculated from raw fluorescence values following the Joint Global Ocean Flux Study (JGOFS) Report (1994). We calibrated the fluorimeter using a spectrophotometrically determined standard curve.
The rates of chl-a decay required for modeling particle reworking (Sun et al., 1991) were obtained by incubating (7 °C) surface sediment in the dark in resealable containers. During 35 d, three parallel samples were removed at different time intervals and treated as described above. From the temporal decline in chl-a concentration, a pseudo-first-order constant, kd, was calculated.
2.5 Modeling data
The distribution of chlorophyll concentrations with depth was used to infer rates of transport, separating local from non-local transport. Soetaert et al. (1996) derived a hierarchical model family consisting of six models of increasingly complex biological transport. The simplest model describes the tracer distribution without mixing by organisms and only depending on decay and sediment accumulation. In model 2, diffusive mixing is included (Db (cm2 yr−1)). Starting from model 3, all subsequent models include “non-local” transport of the tracer from the sediment surface to depth with increasing complexity (injection, J, and ingestion, r). There is no benefit in trying to differentiate between models of higher complexity (models 3, 4, 4a, and 5), since fitting results seemingly differ owing to some horizontal heterogeneity in our data. Also, we lack observations that would allow us to differentiate mechanisms like particle injection in physical sediment turnover and compare them to model results. Therefore, we grouped all fits of non-local models into the category “non-local” and have not interpreted them further. A level of p≤0.05 was used for statistical significance in modeling (see Soetaert et al., 1996, for details).
The two experiments provided similar displacement of surface sediment onto the adjacent seafloor. The effect of removal was visible by eye as surface topography, albeit only with a small vertical displacement of the sediment–water interface. Peaks found in our ex situ experiment were made by shoveling sediment and consciously depositing it upside down onto the adjacent sediment. This created peaks at 1.25 cm depth on average. After shoveling, the sediment visibly slid to both sides of the newly formed mound but particularly into the furrow. The relatively narrow furrow compared to the excavation depth (4 cm) might have promoted this sliding effect compared to the field experiment with a wider furrow. The chl-a profiles imply that an overall net deposition of 1–1.5 cm sediment thickness remained. The peak concentration (Fig. 4, upper row) declines upwards and downwards at similar rates and approaches the background of about 8 µg cm−3 at 3.5 cm depth. This is 2.25 cm below the original sediment–water interface and corresponds well to concentrations in control and net areas below 2 cm depth. Concentrations in the upper 1 cm of the furrow are surprisingly high on average. This may be a result of sediment sliding back from the mounds.
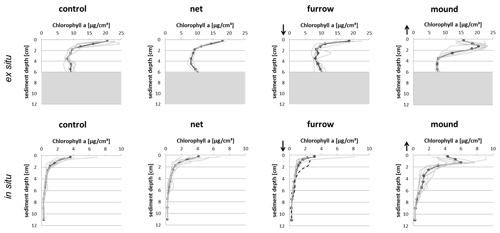
Figure 4Averaged (bold) and individual (grey) chl-a tracer profiles for both ex situ experiments (upper row) and in situ experiments (lower row). The dashed line in the lower furrow panel represents one core sampled with an individual of Arctica islandica found at 2 cm depth. Arrows indicate that the topography of the sediment–water interface was shifted relative to control or net areas by approximately 1–1.5 cm – down in the furrow area and up in the mound area. Shaded areas in the upper panels indicate pigment levels not relevant to the present discussion.
The pattern in the in situ experiment is the same as that in the ex situ experiment despite very different levels of concentrations. In the field experiment, the impact of the ground net remains undetectable, as net profiles resemble the controls closely. Surface mean concentrations of 4 µg cm−3 decline quickly with depth. Furrow surface concentrations are somewhat lower than those in the controls and indicate a net decapping effect of about 1 cm in the in situ experiment. The peak at 1.25 cm depth in the mound resembles that in the ex situ experiment. At 6 µg cm−3, its average concentration is higher than z=0 concentrations in the controls and may indicate some active sorting mechanism. Morys et al. (2021) described surface sediment removal by dredging as showing very similar patterns in the furrow zone.
Overall, the generating mechanism and the effect of this type of otter board on the particle tracer profile appear clear. The displaced sediment generated a peak in the vertical profile of particulate chlorophyll in both experiments. The profile shape indicates that the peaks result from the fact that the sediment material was flipped over. The maximum surface concentrations of the two interfaces meet at a depth of about 1.25 cm (Fig. 4). This generates a pronounced signal of sediment movement. A much higher concentration close to the sediment surface in the ex situ experiment than at the in situ site resulted from the sediments rich in microphytobenthos sampled from a shallow local site in preparation for our experiment.
Field data from the Fehmarn Belt showed peaks of different maximum concentration and depth in 7 out of 11 profiles randomly sampled. Figure 5 depicts three examples and one profile without a peak. Most peaks were positioned within the top 2 cm of the sediment with the exception of one single peak at 3–5 cm depth, as shown in Fig. 5. Such peaks are commonly interpreted as signs of non-local transport resulting from faunal activity.
We compiled the qualitative information on non-local versus local reworking retrieved using the Soetaert et al. (1996) mixing algorithm (“mixing.exe”) by giving the number of best fits in each category (Table 1). The overview includes three field sites with muddy sediment or silty fine sands. Peaks are a frequent feature in sediments from the Mecklenburg Bay and the Fehmarn Belt when sampled randomly from ships. Without explicitly targeting specific features produced by demersal fishery gear (net, mounds, or furrows), 32 % to 64 % of samples depicted peaks. In the two experiments where trawl features were specifically sampled from, peaks were most abundant in mound areas (70 %–80 %). Samples from furrows displayed comparatively many profiles without any mixing; however, they also showed local and non-local mixing in roughly equal numbers. Net areas and control areas were similar in that they were dominated by local mixing in the trawl experiment. The ex situ control and net values in Table 1 should be considered with caution because of inconsistencies in tracer distribution below 6 cm depth.
Table 1Compilation of results from targeted sampling in the two experiments, highlighting local versus non-local transport as interpreted using the software “mixing.exe” (Soetaert et al., 1996). Each column shows the numbers of depth profiles modeled, and the column “non-local” shows in parentheses the percentages of non-local modeling results. Our own results randomly obtained in the Fehmarn Belt area in 2020 and data from the Mecklenburg Bay reported by Morys et al. (2016) are provided for comparison.
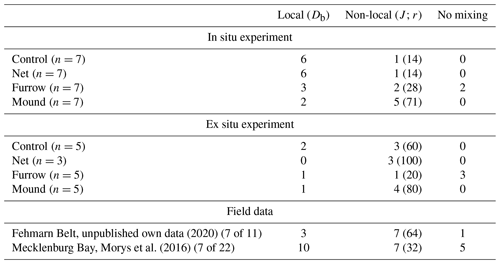
Two experimental approaches to sediment reworking by otter board trawling showed largely identical results. Both simulated ex situ sediment reworking using a shovel as a surrogate, and targeted sampling of in situ otter board marks revealed the same pattern of subsurface peaks. This leads us to conclude, at least qualitatively, that the mechanism at work is the same. It indicates, too, that the otter board reverses a sediment slab more than it pushes sediment aside. Deposition of part of the excavated surface sediment onto the adjacent sediment–water interface generates concentration peaks of the particle tracer chl a. These peaks resemble those of biogenic origin in non-local bioturbation.
This similarity poses a problem in areas like the Fehmarn Belt, where chl-a peaks in the vertical sediment profile may stem from bioturbating infauna or fishery gear. The uncertain origin of the observed peaks may affect the assessment of bioturbation intensity, since peaks may only indicate sediment perturbation in general and not necessarily that owed to macrofauna at the seafloor. Similarly, addressing quantitative aspects of otter board disruption of the seafloor may be difficult.
There are more or less pronounced additional peaks visible in some individual in situ profiles. Based on the decay of the chl-a tracer with time, we are certain that any previous trawling event that may have generated the peaks would be older than 3–4 months. It would therefore not interfere with our interpretation of the relocation of the fresh surface particle tracer. However, we found an A. islandica individual of 2 cm length at the peak depth in the sediment core, from which we obtained the profile highlighted in the in situ furrow (Fig. 4). In view of the core's inner diameter (36 mm) we attribute this peak directly to the activity of this animal. Patchiness in concentrations in both horizontal and vertical directions is obvious in both field and ex situ data. This is a reminder that there may always be peaks left without a known origin and that averaging of replicate profiles is advisable.
We regularly encountered chl-a peaks in the Fehmarn Belt, where MUC sampling was random. Still, 7 of 11 randomly cored locations displayed peaks at depths comparable to those of our experimentally generated peaks (Table 1; Figs. 4 and 5). These peaks cannot be assigned to either trawling or bioturbation with any certainty.
Below, we will pursue the questions of (1) whether this problem is unique to our area of investigation in the Fehmarn Belt, (2) what implications it may have for our understanding of the ecosystem, and (3) whether there are ways to resolve it.
4.1 Uniqueness to our area of investigation
It is not clear if the problem of indistinguishable peaks exists in other trawling areas of the oceans, too. In the areas investigated here, A. islandica is the benthic organism dominant in terms of biomass (Zettler et al., 2001) and is thought to be responsible for the reworking of sediments (Morys et al., 2016, 2017). The area is also among the most heavily trawled in the North Sea and Baltic Sea region (Amoroso et al., 2018). We are not aware of a similar comparison of trawling effects and bioturbation effects in the literature.
In general, both transport phenomena – biological non-local reworking and the physical impact of otter boards – move sediment particles in a similar way, at least on a relatively coarse scale of centimeters as employed here. Decapping by trawling is described by Morys et al. (2021) and Bradshaw et al. (2021), who reported the removal of the top sediment layer in an artificial furrow in the Baltic Sea off southern Sweden. While their report is based on direct observation and measured vertical distributions of sediment parameters, several indirect reports concerning other seas show that this decapping effect seems to be a feature observed in other trawling areas (Oberle et al., 2016b; Tiano et al., 2019; Depestele et al., 2019; Morys et al., 2021). However, the observation of a peak generated during the process of decapping, as shown in the present paper, has not been reported by any of the previous authors.
Oberle et al. (2016b) identify mixing of sediments by trawl boards on the Iberian shelf as deep as 35 cm into the sediment. They deciphered trawling effects on sediments off the Iberian west coast by combining lithological information, traces from recent oil pollution, and highly resolved spatial information on bottom trawling. These authors could not be certain when it came to exactly sampling the sediment site where an effect of a board would be visible. The authors suggested five scenarios of impact based on different lines of evidence. The second scenario is the one mirroring our turnover of surface sediment. The former sediment surface is buried, and the tracer appears at 18 cm rather than 6 cm depth, the usual mixing depth by animals and currents in the area. At the Iberian site, the large difference in depth makes differentiation between shallow biogenic peaks and deep peaks generated by fishing boards possible.
Contrary to the situation found by Oberle et al. (2016b), the peaks of chl a are undistinguishable when two co-occurring mechanisms of transport generate them at similar depths. Morys et al. (2016, 2017) used a pairwise comparison of the fauna present and the occurrence of a peak within the same sediment core to convincingly argue that the animals caused that peak. The authors found positive correlations in 40 %–70 % of the 22 investigated profiles, including locations in close proximity (in the Mecklenburg Bay) to both our field sites. This is compelling evidence for the biogenic origin of peaks. While there is little direct proof of a cause–effect relation of biological peak generation in the literature, there is no doubt that some benthos do generate peaks by non-local transport mechanisms (e.g., Blair et al., 1996). We suspect that the origin of peaks in a given area can only be inferred if the bioturbating organisms, gear used, and intensity of trawling are known.
4.2 Implications
The most notable consequence of indistinguishable peaks is likely that bioturbation studies may be suspected to overestimate the biogenic reworking effect. We will argue below (“Ways to resolve”) that this is not the case in the Fehmarn Belt; however, this issue might need observation in other areas where trawling and bioturbation are prominent. If some peaks were indicating trawling and not non-local reworking, our concept of how the seafloor is reworked might need reassessment.
Effects within the sediment may be visible when heavy gear is used. In the Fehmarn Belt area, fishing gear is comparatively light. We did not detect changes in chl-a distribution in the net area, as the simulated and real impact profiles in net areas were indistinguishable from those in respective controls in our study. Nets are reported to mix surface sediments and reduce chl-a content in the top centimeter (Oberle et al., 2016b; Tiano et al., 2019). Depestele et al. (2019), working at a southwestern Frisian Front site in the North Sea, found that trawling may affect particle size distribution down to 2–4 cm depth. They also suggested that this trawling caused injection of finer particles into the sediment at about 4 cm depth while winnowing the top surface sediment due to a combined mechanism of sediment removal (decapping) and mixing. While we cannot exclude similar winnowing or injection from our data, their role is likely small judging from the similarity to control depth profiles of chl a. Biological mixing of particles, reworking (Kristensen et al., 2012), and bioresuspension (Graf and Rosenberg, 1997) may generate the same profile of control sediment as seen in the net area. Observing our data, there is no visible decapping effect in the net area, possibly because of the smaller size of gear employed. Trawling gear referred to by Depestele et al. (2019) and used in the North Sea is in general much larger than the gear used in our study and in the Fehmarn Belt. We thus conclude that the net impact is not detectable in our chl-a depth distributions and that mixing appears to be quasi-random and similar to that in the controls.
While otter boards may generate peaks and tracer profiles that resemble those generated by bioturbating fauna, the mechanisms of particle transport differ considerably and imply different consequences. Removing and overthrowing sediment, such as that achieved by scouring otter boards, can bury animals, a process which does not usually happen during bioturbation. Particularly, smaller surface-dwelling benthos (Cumacea, Ophiuroidea) and meiofauna may not be able to escape from a slab of sediment deposited above them. Larger burrowers like A. islandica have the ability to escape, depending on the height of the deposits above the original sediment surface (Bromley, 1996; Powilleit et al., 2009). Some fauna may be excavated from the sediment or damaged during board passage and become available for predators and scavengers. Reports on the shift in functional groups towards smaller-sized and possibly opportunistic fauna caused by bottom trawling support this (Sparks-McConkey and Watling, 2001; Hiddink et al., 2019). As demonstrated by Mestdagh et al. (2018), particle reworking, possibly as a consequence of an escape reaction, and bioirrigation may mitigate the effects of trawling.
Reversal of the top sediment will also affect sediment biogeochemistry, since it changes chemical gradients close to the sediment–water interface completely. In the troughs, decapping locally exposes anoxic sediments to oxygenated overlying water. While oxygen consumption was not measured, it conceivably may increase when the board exposes high concentrations of reduced dissolved substances in the furrow or when reactive organic matter, such as chlorophyll, is buried in the mound. It may decrease, however, with less reactive sediment exposed in the furrow or with the reversed sediment slab forming the mound surface (Tiano et al., 2019, 2022). Van de Velde et al. (2018) simulated and measured complete homogenization of a 15 cm surface layer due to mixing by trawling nets as well as the dumping of a homogeneous 15 cm layer on top of an existing sediment. They found pronounced, enhanced mineralization dominated by anaerobic pathways and effects on manganese cycling as consequences of both scenarios. Based on samples from the experiment we report here, Röser et al. (2022) suggest that the coupled Fe–Mn–P cycle reacts very sensitively, as expressed by altered pore water gradients, indicating Mn enrichment in the mound area and Mn loss in the furrow. A disruption of the steady-state biogeochemical distribution is apparent in both furrow and mound areas, although our setting differs considerably from the more massive gear-trawling impacts discussed with regard to the North Sea (van de Velde et al., 2018; Tiano et al., 2019) in terms of both sediment height (∼ 5 versus 15 cm) and mechanism (decapping/turnover versus mixing).
The present investigation cannot further explore organic carbon fate after such trawling impacts (Epstein et al., 2022), since we did not generate corresponding data. Transient redox reactions in the sediment initiated by trawling (Bradshaw et al., 2021; Morys et al., 2021; Tiano et al., 2019), however, may differ considerably from redox oscillations known to occur during bioturbation (Forster, 1996; Aller, 2014; Gilbert et al., 2016). Redox oscillations, as they occur along burrows of infauna, are considered a driver for differences in chemical speciation, element cycling, and priming processes associated with bacterial carbon cycling. Their occurrence is intrinsically linked to a spatial diffusion geometry associated with burrows and biogenic structures (Aller, 1994). Since the physics and geometry of an otter board's impact on the seafloor are vastly different, we anticipate that their effects on diagenesis in fact differ substantially from bioturbation effects.
In conclusion, we argue that we need to know if peaks indicate trawling or bioturbation because their effects on biota and the way they affect geochemical processes differ substantially.
4.3 Ways to resolve
In our Fehmarn Belt data set, we cannot assign a single peak with any certainty to biological or physical reworking. Can knowledge of the environment help to resolve this issue, since differences in peak generation likely indicate different effects on the ecosystem? We may obtain some certainty about the origin of peaks by employing generalized information on faunal abundance and behavior as well as trawling intensity, as outlined below.
The Fehmarn Belt is among the areas with very high fishing intensity globally (Amoroso et al., 2018). The International Council for the Exploration of the Sea (ICES) assesses trawling effort using SARsubsurface, the swept-area ratio below 2 cm sediment depth (Christian von Dorrien, personal communication, 2023/2023 project report; ICES database: https://doi.org/10.17895/ices.data.20310255.v3; Eigaard et al., 2016), with 0.1 per quarter or 10 % of an area within 3 months. This number relates strictly to mobile bottom-contacting gear and includes specific information regarding gear and vessel size but lacks high spatial resolution. In order to relate this information to our data, we averaged four areas (area size: 5.6×3.2 km, i.e., 17.9 km2) from April–June 2020 overlapping with the research area in the Fehmarn Belt, in which we sampled and detected peaks in June 2020. Although it is difficult to relate this information to the very nature of physical scouring effects and their spatial distribution on the seafloor, it is the best information available. With SARsubsurface, we can imagine that otter boards affect below-surface sediment in 10 % of the area within this quarter (April–June 2020). We can equally imagine this as a probability: otter boards potentially generate peaks in 0.1 of every square meter (0.1 m−2) within 3 months or 0.4 if homogeneously spaced.
In contrast, A. islandica are likely to move daily. Their abundance in the Fehmarn Belt amounts to around 40 individuals m−2. The animals usually stay close to the sediment surface because of their short siphon. They move their shells when gaping and closing in response to threat from demersal predators and food supply from filter feeding. Ballesta-Artero et al. (2017) demonstrated that gaping activity in these bivalves changes seasonally with food supply but shows a minimum of one to two gaping events per month during the lowest activity in winter. The authors could not determine the frequency of gaping during high-activity phases, but it is certainly higher. We assume that shell movement during gaping is a mechanism allowing surface material, including chl-a pigments, to slide along the shell into deeper sediment layers (anywhere to 4 cm depth, the size of A. islandica individuals). A total of 40 individuals could produce at least 40 transport events per month or 480 . The numbers calculated suggest frequencies that are 3 orders of magnitude higher for biogenic non-local transport (480 versus 0.4 ).
The spatial aspects of the particle transport events discussed above are particularly difficult to assess, since patchy occurrence of A. islandica and clustering of trawl tracks (Schönke et al., 2022) are frequent. Mound width, and thus the area showing peaks generated by otter boards, likely depends on the sediment type and the steepness of the mounds and is additionally altered by the “bumpy” and discontinuous character of sediment deposition along the furrow (Morys et al., 2021). Despite this, with similar assumptions as above (and with more uncertainty), we estimate that A. islandica rework sediment on an area of 0.94 m2 yearly (5 cm diameter circle around one animal) – i.e., 19.6 cm2 reworked multiplied by 480 events per year = 0.94 m2. This is a larger area than the area disturbed by otter boards (0.1 m2).
Thus, we regard it as conservatively safe to assume that the majority of peaks detected in the Fehmarn Belt stem from A. islandica active on a daily scale. Bioturbation by A. islandica in the Fehmarn Belt should thus be the more frequent particle-reworking process when compared with otter board sediment reworking. Therefore, we may continue to interpret chlorophyll peaks as bioturbation traces in this area. Researchers observing other areas of the oceans impacted by bottom trawling may perform similar estimates of the likelihood of trawling and bioturbation traces to those shown here if information on trawling intensity and abundance of major bioturbating fauna is available.
While we feel confident to say that we generally look at bioturbation when we find peaks, this does not imply that the effects of bottom trawling in our area are not important or may be visible in other data. The present result does not account for impacts which we cannot measure using the particle tracer chl a, such as impacts on fauna mortality, sediment resuspension, or the remobilization of reduced sediment components. Furthermore, we cannot elucidate with the present data the associated biological and biogeochemical effects. Particularly, the spatial magnitude of both bioturbation and trawling needs better quantification for such a comparison. Future exclusion of fisheries in the area will provide a test field in which the persistence of peaks may be tested and their origin confirmed.
The data presented in this study are archived at https://doi.org/10.18453/rosdok_id00004543 (Forster et al., 2024).
All authors provided data and contributed to writing this paper. CR, JL, and LP conducted sampling and measurements for the experiments, while MP and SF did so for the field data. SF and MP provided the concept and most of the figures, and SF and MP wrote the paper.
The contact author has declared that none of the authors has any competing interests.
Publisher's note: Copernicus Publications remains neutral with regard to jurisdictional claims made in the text, published maps, institutional affiliations, or any other geographical representation in this paper. While Copernicus Publications makes every effort to include appropriate place names, the final responsibility lies with the authors.
We thank the crew of RV Limanda for their fieldwork assistance as well as Christian von Dorrien for the valuable discussion on the quantification of trawling effort.
This research has been supported by the Bundesministerium für Bildung und Forschung (German Federal Ministry of Education and Research) (grant no. 03F0848B).
This paper was edited by Jack Middelburg and reviewed by Justin Tiano and one anonymous referee.
Aller, R.: Bioturbation and remineralization of sedimentary organic matter: effects of redox oscillation, Chem. Geol., 114, 331–345, https://doi.org/10.1016/0009-2541(94)90062-0, 1994.
Aller, R. C.: Sedimentary Diagenesis, Depositional Environments, and Benthic Fluxes, in: Treatise on Geochemistry, vol. 8, 2nd edn., edited by: Holland, H. D. and Turekian, K. K., Elsevier, Oxford, 293–334, https://doi.org/10.1016/B978-0-08-095975-7.00611-2, 2014.
Amoroso, R. O., Pitcher, C. R., Rijnsdorp, A. D., and Jennings, S.: Bottom trawl fishing footprints on the world's continental shelves, P. Natl. Acad. Sci. USA, 115, E10275–E10282, https://doi.org/10.1073/pnas.1802379115, 2018.
Ballesta-Artero, I., Witbaard, R., Carroll, M. L. C., and van der Meer, J.: Environmental factors regulating gaping activity of the bivalve Arctica islandica in Northern Norway, Mar. Biol., 164, 116, https://doi.org/10.1007/s00227-017-3144-7, 2017.
Blair, N. E., Levin, L. A., DeMaster, D. J., and Plaia, G.: The short-term fate of fresh algal carbon in continental slope sediments, Limnol. Oceanogr., 41, 1208–1219, https://doi.org/10.4319/lo.1996.41.6.1208, 1996.
Bradshaw, C., Tjensvoll, I., Sköld, M., Allan, I. J., Molvaer, J., Magnusson, J., Naes, K., and Nilsson, H. C.: Bottom trawling resuspends sediment and releases bioavailable contaminants in a polluted fjord, Environ. Pollut., 170, 232–241, https://doi.org/10.1016/j.envpol.2012.06.019, 2012.
Bradshaw, C., Jakobsson, M., Brüchert, V., Bonaglia, S., Mörth, C.-M., Muchowski, J., Stranne, C., and Sköld, M.: Physical disturbance by bottom trawling suspends particulate matter and alters biogeochemical processes on and near the seafloor, Front. Mar. Sci., 8, 683331, https://doi.org/10.3389/fmars.2021.683331, 2021.
Bromley, R. G.: Trace Fossils: Biology, Taphonomy and Applications, 2nd edn., Chapmann and Hall, 361 pp., ISBN 9780412614804, 0412614804, 1996.
Bunke, D., Leipe, T., Moros, M., Morys, C., Tauber, F., Virtasalo, J. J., Forster, S., and Arz, H. W.: Natural and Anthropogenic Sediment Mixing Processes in the South-Western Baltic Sea, Front. Mar. Sci., 6, 677, https://doi.org/10.3389/fmars.2019.00677, 2019.
De Borger, E., Tiano, J., Braeckman, U., Rijnsdorp, A. D., and Soetaert, K.: Impact of bottom trawling on sediment biogeochemistry: a modelling approach, Biogeosciences, 18, 2539–2557, https://doi.org/10.5194/bg-18-2539-2021, 2021.
De Juan, S., Hewitt, J., Thrush, S., and Freeman, D.: Standardising the assessment of Functional Integrity in benthic ecosystems, J. Sea Res., 98, 33–41, https://doi.org/10.1016/j.seares.2014.06.001, 2015.
Depestele, J., Degrendele, K., Esmaeili, M., Ivanovi, A., Kro, S., Neill, F. G. O., Parker, R., Polet, H., Roche, M., Teal, L. R., Vanelslander, B., and Rijnsdorp, A. D.: Comparison of mechanical disturbance in soft sediments due to tickler-chain SumWing trawl vs. electro-fitted PulseWing trawl, ICES J. Mar. Sci., 76, 312–329, https://doi.org/10.1093/icesjms/fsy124, 2019.
Eigaard, O. R., Bastardie, F., Breen, M., Dinesen G. E., Hintzen, N. T., Laffargue, P., Mortensen, L. O., Nielsen, J. R., Nilsson, H. C., O'Neill, F. G., Polet, H., Reid, D. G., Sala, A., Sköld, M., Smith, C., Sørensen, T. K., Tully, O., Zengin, M., and Rijnsdorp, A. D.: Estimating seabed pressure from demersal trawls, seines, and dredges based on gear design and dimensions, ICES J. Mar. Sci., 73, i27–i43, https://doi.org/10.1093/icesjms/fsv099, 2016.
Epstein, G., Middelburg, J. J., Hawkins, J. P., Norris, C. R., and Roberts, C. M.: The impact of mobile demersal fishing on carbon storage in seabed sediments, Global Change Biol., 28, 2875–2894, https://doi.org/10.1111/gcb.16105. 2022.
Forster, S.: Spatial and temporal distribution of oxidation events occurring below the sediment water interface, Mar. Ecol., 17, 309–319, 1996.
Forster, S., Runkel, C., Lemke, J., Pülm, L., and Powilleit, M.: Chlorophyll-a concentrations in marine sediments from experiments and Fehmarn Belt (2020–2021), University of Rostock [data set], https://doi.org/10.18453/rosdok_id00004543, 2024.
Gilbert, F., Hulth, S., Grossi, V., and Aller, R. C.: Redox oscillation and benthic nitrogen mineralization within burrowed sediments: An experimental simulation at low frequency, J. Exp. Mar. Biol. Ecol., 482, 75–84, https://doi.org/10.1016/j.jembe.2016.05.003, 2016.
Gogina, M. and Feldens, P.: Elisabeth Mann Borgese-Berichte, MGF-Ostsee Project: Potential effects of closure for bottom fishing in the marine protected areas (MPAs) of the western Baltic Sea – baseline observations, Cruise No. EMB238 / Leg1+2, 26.05.2020–09.06.2020, Leibniz Institute for Baltic Sea Research Warnemünde, 43 pp., 2020.
Graf, G. and Rosenberg, R.: Bioresuspension and biodeposition: a review, J. Marine Syst., 11, 269–278, https://doi.org/10.1016/S0924-7963(96)00126-1, 1997.
Hiddink, J. G., Jennings, S., Sciberras, M., Bolam, S. G., Cambiè, G., McConnaughey, R. A., Mazor, T., Hilborn, R., Collie, J. S., Pitcher, C. R., Parma, A. M., Suuronen, P., Kaiser, M. J., and Rijnsdorp, A. D.: Assessing bottom trawling impacts based on the longevity of benthic invertebrates, J. Appl. Ecol., 56, 1075–1084, https://doi.org/10.1111/1365-2664.13278, 2019.
Kristensen, E., Penha-Lopes, G., Delefosse, M., Valdemarsen, T., Quintana, C. O., and Banta, G. T.: REVIEW – what is bioturbation? The need for a precise definition for fauna in aquatic sciences, Mar. Ecol. Prog. Ser., 446, 285–285, https://doi.org/10.3354/meps09506, 2012.
JGOFS Report: Report 19, Chap. 14, Protocols for the Joint Global Ocean Flux Study (JGOFS) Core Measurements, 97–100, 1994.
Mestdagh, S., Bagaço, L., Braeckman, U., Ysebaert, T., De Smet, B., Moens, T., and Van Colen, C.: Functional trait responses to sediment deposition reduce macrofauna-mediated ecosystem functioning in an estuarine mudflat, Biogeosciences, 15, 2587–2599, https://doi.org/10.5194/bg-15-2587-2018, 2018.
Meysman, F. J. R., Boudreau, B. P., and Middelburg, J. J.: Relations between local, nonlocal, discrete and continuous models of bioturbation, J. Mar. Res., 61, 391–410, 2003.
Morys, C., Forster, S., and Graf, G.: Variability of bioturbation in various sediment types and on different spatial scales in the southwestern Baltic Sea, Mar. Ecol. Prog. Ser., 557, 31–49, https://doi.org/10.3354/meps11837, 2016.
Morys, C., Powilleit, M., and Forster, S.: Bioturbation in relation to the depth distribution of macrozoobenthos in the southwestern Baltic Sea, Mar. Ecol. Prog. Ser., 579, 19–36, https://doi.org/10.3354/meps12236, 2017.
Morys, C., Brüchert, V., and Bradshaw, C.: Impacts of bottom trawling on benthic biogeochemistry in muddy sediments: Removal of surface sediment using an experimental field study, Mar. Environm. Res., 169, 105384, https://doi.org/10.1016/j.marenvres.2021.105384, 2021.
Oberle, F. K. J., Storlazzi, C. D., and Hanebuth, T. J. J.: What a drag: Quantifying the global impact of chronic bottom trawling on continental shelf sediment, J. Mar. Res., 159, 109–119, https://doi.org/10.1016/j.jmarsys.2015.12.007, 2016a.
Oberle, F. K. J., Swarzenski, P. W., Reddy, C. M., Nelson, R. K., Baasch, B., and Hanebuth, T. J. J.: Deciphering the lithological consequences of bottom trawling to sedimentary habitats on the shelf, J. Mar. Res., 159, 120–131, https://doi.org/10.1016/j.jmarsys.2015.12.008, 2016b.
Powilleit, M., Graf, G., Kleine, J., Riethmüller, R., Stockmann, K., Wetzel, M. A., and Koop, J. H. E.: The critical survival depth of common brackish macrofauna invertebrates from the Baltic Sea to dredged disposal induced sediment cover: A laboratory experiment and its implications for the field, J. Marine Syst., 75, 441–451, https://doi.org/10.1016/j.jmarsys.2007.06.011, 2009.
Queirós, A. M., Birchenough, S. N. R., Bremner, J., Godbold, J. A., Parker, R. E., Romero-Ramirez, A., Reiss, H., Solan, M., Somerfield, P. J., Van Colen, C., Van Hoey, G., and Widdicombe, S.: A bioturbation classification of European marine infaunal invertebrates, Ecol. Evol., 3, 3958–3985, https://doi.org/10.1002/ece3.769, 2013.
Roeser, P., Zeller, M. A., Feldens, P., Kallmeyer, J., Clemens, D., Rooze, J., Radtke, H., Schönke, M., Schmiedinger, I., Forster, S., Sommer, S., and Böttcher, M. E.: Short-term anthropogenic impact of mobile bottom-contact fishing on the biogeochemistry of coastal sediments and its long-term effects on mineral distribution, EGU General Assembly 2022, Vienna, Austria, 23–27 May 2022, EGU22-5051, https://doi.org/10.5194/egusphere-egu22-5051, 2022.
Rooze, J., Zeller, M. A., Gogina, M., Roeser, P., Kallmeyer, J., Schönke, M., Radtke, H., and Böttcher, M. E.: Bottom-trawling signals lost in sediment: A combined biogeochemical and modeling approach to early diagenesis in a perturbed coastal area of the southern Baltic Sea, Sci. Total Environ., 906, 167551, https://doi.org/10.1016/j.scitotenv.2023.167551, 2024.
Schönke, M., Clemens, D., and Feldens, P.: Quantifying the Physical Impact of Bottom Trawling Based on High-Resolution Bathymetric Data, Remote Sens.-Basel, 14, 2782, https://doi.org/10.3390/rs14122782, 2022.
Soetaert, K. H., Herman, P. M. J., Middelburg J. J., Heip, C., Destigter, H. S., Van Weering, T. C. E., Epping, E., and Helder, W.: Modeling 210Pb-derived mixing activity in ocean margin sediments: Diffusive versus nonlocal mixing, J. Mar. Res., 54, 1207–1227, 1996.
Solan, M., Ward, E. R., White, E. L., Cassidy, C., Schuster, J. M., Hale, R., and Godbold, J. A.: Worldwide measurements of bioturbation intensity, ventilation rate, and the mixing depth of marine sediments, Scientific Data, 6, 58, https://doi.org/10.1038/s41597-019-0069-7, 2019.
Sparks-McConkey, P. J. and Watling, L.: Effects on the ecological integrity of a soft-bottom habitat from a trawling disturbance, Hydrobiologia, 456, 73–85, https://doi.org/10.1023/A:1013071629591, 2001.
Sun, M., Aller, R. C., and Lee, C.: Early diagenesis of chlorophyll-a in Long Island Sound sediments: A measure of carbon flux and particle reworking, J. Mar. Res., 49, 379–401, 1991.
Tiano, J. C., Witbaard, R., Bergman, M. J. N., Van Rijswijk, P., Tramper, A., Van Oevelen, D., and Soetaert, K.: Acute impacts of bottom trawl gears on benthic metabolism and nutrient cycling, ICES J. Mar. Sci., 77, 2285–2294, https://doi.org/10.1093/icesjms/fsz027, 2019.
Tiano, J. C., Depestele, J., Van Hoey, G., Fernandes, J., van Rijswijk, P., and Soetaert, K.: Trawling effects on biogeochemical processes are mediated by fauna in high-energy biogenic-reef-inhabited coastal sediments, Biogeosciences, 19, 2583–2598, https://doi.org/10.5194/bg-19-2583-2022, 2022.
van de Velde, S., Van Lancker, V., Hidalgo-Martinez, S., and Berelson, W. M.: Anthropogenic disturbance keeps the coastal seafloor biogeochemistry in a transient state, Sci. Rep.-UK, 8, 5582, https://doi.org/10.1038/s41598-018-23925-y, 2018.
van Denderen, D., Törnroos, A., Sciberras, M., Hinz, H., Friedland, R., Lasota, R., Mangano, M., Robertson, C., Valanko, S., and Hiddink, J.: Effects of bottom trawling and hypoxia on benthic invertebrate communities, Mar. Ecol. Prog. Ser., 694, 13–27, https://doi.org/10.3354/meps14094, 2022.
Watling, L. and Norse, E. A.: Disturbance of the Seabed by Mobile Fishing Gear: A Comparison to Forest Clearcutting, Conserv. Biol., 12, 1180–1197, https://doi.org/10.1046/j.1523-1739.1998.0120061178.x, 1998.
Wheatcroft, R. A., Olmez, I., and Pink, F. X.: Particle bioturbation in Massachusetts Bay: Preliminary results using a new deliberate tracer technique, J. Mar. Res., 52, 1129–1150, 1994.
Winter, J. E.: Über den Einfluß der Nahrungskonzentration und anderer Faktoren auf Filtrierleistung und Nahrungsausnutzung der Muscheln Arctica islandica und Modiolus modiolus, Mar. Biol., 4, 87–135, https://doi.org/10.1007/BF00347037, 1969.
Zettler, M. L., Bönsch, R., and Gosselck, F.: Distribution, abundance and some population characteristics on the ocean quahog, Arctica islandica (Linnaeus, 1767) in the Mecklenburg Bight (Baltic Sea), J. Shellfish Res., 20, 161–169, 2001.