the Creative Commons Attribution 4.0 License.
the Creative Commons Attribution 4.0 License.
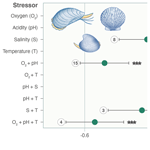
Reviews and syntheses: The clam before the storm – a meta-analysis showing the effect of combined climate change stressors on bivalves
Rachel A. Kruft Welton
Daniela N. Schmidt
James D. Witts
Benjamin C. Moon
The impacts of climate change on marine organisms have been increasingly documented in laboratory and experimental studies. However, the use of different taxonomic groupings and the assessment of a range of processes make identifying overall trends challenging. Meta-analysis has been used to determine general trends, but coarse taxonomic granularity may mask phylogenetically specific responses. Bivalve molluscs are a data-rich clade of ecologically and economically important calcifying marine taxa that allow for the assessment of species-specific vulnerability across developmental stages. Drawing on the large body of available literature, we conduct a meta-analysis of 203 unique experimental set-ups in order to examine how bivalve growth responds to increased water temperature, acidity, deoxygenation, and changes in salinity in 10 climate change stressor combinations. This is the most complete examination of bivalve responses to date and shows that anthropogenic climate change will disproportionally affect particular families, suggesting taxonomic differentiation in climate change response. Specifically, Mytilidae, Ostreidae, and Pectinidae (67 % of experiments) respond with negative effect sizes for all individual stressors, whereas responses in Pinnidae, Tellinidae, and Veneridae are more complex. Our analysis shows that earlier studies reporting negative impacts on bivalves are driven by only three or four well-studied, commercially important families. Despite the taxonomic differentiation, almost all drivers and their combinations have significant negative effects on growth. The synergistic impacts of deoxygenation, acidification, and temperature result in the largest negative effect size. Infaunal taxa, including Tellinidae and Veneridae, appear more resistant to warming and oxygen reduction than epifaunal or motile taxa, but this difference between the two taxa is also based on a small number of data points. The current focus of experimental set-ups on commercially important taxa and families within a small geographic range creates gaps in the understanding of global impacts on these economically important foundation organisms.
- Article
(2767 KB) - Full-text XML
- BibTeX
- EndNote
Rising levels of atmospheric carbon dioxide will alter the marine environment over the coming decades. Sea surface temperatures are projected to rise 2–4 ∘C globally by the end of the century, depending on the region and emission scenario (IPCC, 2021), with some areas, such as the Gulf of Mexico, already suffering frequent, severe deoxygenation events (Breitburg et al., 2018). Higher latitudes will be exposed to more severe warming than the tropics (Meredith et al., 2019), resulting in sea ice and glacial melting, raising global sea levels, and increasing runoff and freshwater influx into marine settings (Lu et al., 2022). Ocean pH will decline by between 0.3 and 1 units by the end of the 21st century, with coastal regions expected to experience greater pH decreases than the open ocean (IPCC, 2021). Oxygen levels in the ocean are projected to decrease by up to 7 %, leading to an expansion of “dead zones” (Breitburg et al., 2018; Messié and Chavez, 2017; Schmidtko et al., 2017). The heterogeneous nature of change in each environmental driver will result in location-specific stressor combinations and extents. This complexity presents a significant challenge for decision-makers in fisheries and marine conservation. Therefore, it is increasingly important to identify how stressors arising from climate change work, both individually and in different combinations, on communities and organisms in different settings.
At the intersection of the marine and terrestrial realms, shallow marine and coastal settings typically exhibit large spatiotemporal variations in physicochemical conditions such as pH, oxygen, and temperature (e.g. Hoffmann et al., 2011). This variability is exacerbated by anthropogenic climate change, leading to more frequent extreme climate events as well as the redistribution of upwelling zones, circulation and currents, and alterations in both the quantity and quality of terrestrial runoff (Sydeman et al., 2014). Therefore, environmental changes are projected to especially impact shallow marine and coastal habitats, which harbour socially and economically important ecosystems: up to 40 % of the world's population lives within 200 km of the coastline (Neumann et al., 2015), and an estimated 775 million people globally have a high dependence on these systems and their services (Selig et al., 2019). Costal ecosystems are estimated to contribute more than 60 % of the total economic value of the biosphere (Martínez et al., 2007), but organisms adapted to live in these systems are predicted to suffer large alterations in their population fitness in response to future climate change (Kroeker et al., 2013; Sampaio et al., 2021; Hoppit and Schmidt, 2022).
Bivalve molluscs (class Bivalvia) are the cornerstone of coastal and shallow marine ecosystems, with representatives in all marine biomes where they provide provisioning and regulatory services (Olivier et al., 2020; Carss et al., 2020; Vaughn and Hoellein, 2018). Bivalves are a key global food source, with production for human consumption growing from 5.1×104 t in 1950 to more than 12.4×106 t today (Smaal et al., 2019). As a result, bivalve aquaculture has an estimated global market value of USD 17.1 billion (van der Schatte Olivier et al., 2018).
Additionally, there is a growing awareness of the wider ecosystem benefits of bivalves as habitat formers. Their biogenic three-dimensional reefs formed by sessile epifaunal taxa such as oysters increase the surrounding biodiversity (Fariñas-Franco and Roberts, 2014). Complex reef frameworks and individual bivalve shells in soft-substrate environments can act as microhabitats for other invertebrates via the creation of new hard substrates and by altering local flow regimes or even temperature (McAfee et al., 2017). Filter-feeding bivalves can reduce pollution and clear large particulates out of the water column (Smyth et al., 2018). They enhance or change local marine productivity (Donnarumma et al., 2018; Strain et al., 2021) via the selective removal of specific species of phytoplankton (Ward and Shumway, 2004). Deposition of bivalve faeces and pseudofaeces, along with the burrowing activity of motile infaunal deposit or suspension feeders, can lead to changes in the local flux or enrichment of organic matter into the sediment and biogeochemical cycling (Smyth et al., 2013) and, ultimately, habitat suitability for other benthos.
Anthropogenic pressures, including coastal development, overfishing, and pollution, have resulted in demonstrable decreases in global bivalve populations (e.g. De Groot, 1984; Baeta et al., 2014). Akin to other calcifying invertebrate organisms, it has been hypothesized that the physiochemical changes resulting from climate change will reduce bivalve growth, impair maintenance of shells (Maynou et al., 2020; Knights et al., 2020), and disrupt larval settlement patterns and spawning (Bascur et al., 2020; Figueirodo et al., 2022). In recognition of the environmental, social, and economic benefits bivalves produce as well as the current and future pressures that they face, the group is a focus for conservation efforts (zu Ermgassen et al., 2020; Buelow and Waltham, 2020; Gagnon et al., 2020). However, despite extensive study, there remain important gaps in our understanding of their response to climate change across different bivalve families.
Currently, our understanding of how bivalves will respond to various climate change stressors is based on field studies and lab-derived experimental data focused largely on ocean acidification and response to warming, generally observing negative responses (e.g. Beukema et al., 2009; Van Colen et al., 2012; Addino et al., 2019; Eymann et al., 2020). Synthesis work through meta-analysis supports the notion that bivalves will generally respond negatively to climate change (Kroeker et al., 2013; Harvey et al., 2013; Sampaio et al., 2021; Hoppit and Schmidt, 2022; Leung et al., 2022). These studies have shown that the synergistic effects of ocean acidification, ocean warming, and an increase in hypoxic events decrease the growth rates of calcifying marine organisms (Kroeker et al., 2010; Maynou et al., 2020; Knights et al., 2020; Sampaio et al., 2021). However, these analyses have been conducted at high taxonomic levels, e.g. examining changes at phylum level, thereby risking averaging differential outcomes at a finer taxonomic resolution. Organisms experience disparate responses to environmental drivers based on local phenotypic expression (Dong et al., 2017) and environmental influences (Genner et al., 2010), resulting, for example, in a species-specific mortality risk to extreme heat based on local microclimates and adaptation (Montalto et al., 2016). “Clumping” these diverse responses make high-level analyses and their generalized trends difficult to interpret (Helmuth et al., 2005). Therefore, our current understanding of how bivalves respond to climate change based on broad-scale synthesis work might not capture the granularity (the level of detail) and diversity of the responses that this group exhibits.
We aimed to fill this gap by employing a meta-analysis methodology to explore the effects of marine climate stressors, and combinations thereof, on bivalve growth at both the whole-group and family levels. Our analysis explores 10 stressor combinations (found in Table 2), greatly expanding earlier work examining only four stressors (Sampaio et al., 2021). For the first time in a meta-analysis of impacts of marine climate change, we explore taxonomic sensitivities within a family and identify large gaps in the taxonomic understanding of how response to climate change in this very well-studied group. Our aim is to determine whether a negative response to climate change is intrinsic to the group or driven by specific taxa. We focused on studies that emphasize bivalve growth rates – a commonly studied trait that offers insight into organism vulnerability to answer how these growth rates are impacted by climate stressors – and whether different families or developmental stages are more sensitive to climate stressors than others. Additionally, we examine the taxonomic diversity of experiments available in the published literature for meta-analysis. We hypothesize that a focus on commercially important bivalve taxa may be creating a bias in current observations. Our findings encourage a new approach to meta-analysis by moving towards more differentiated taxonomic understandings of a group's responses to future conditions while still providing a summative response above the individual species level needed for conservation decision-making.
2.1 Study selection criteria
Primary literature focusing on bivalve growth was identified using the Web of Science Core Collection. The keywords used were as follows: “bivalve”, “Bivalvia”, “meta-analysis”, “acidification”, “pH”, “hypercapnia”, “ocean change” “temperature”, “salinity”, “oxygen”, “deoxygenation”, “hypoxia”, “anoxia”, and combinations thereof. The publication date of articles collected ranged from 1997 to 2020. Articles were initially screened through title relevance, then abstract content, and, finally, full-text content (Fig. 1), from which individual experimental set-ups were extracted. Article lists from previous meta-analyses with a similar scope (Kroeker et al., 2013; Harvey et al., 2013; Sampaio et al., 2021) were additionally consulted to identify material missed from initial search strings. For a list of included articles used for analysis, the reader is referred to Hoppit (2023).
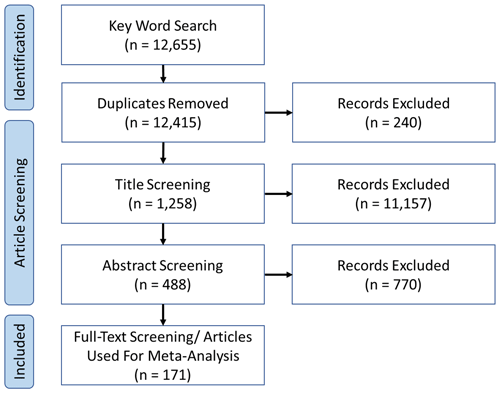
Figure 1Preferred Reporting Items for Systematic Reviews and Meta-Analyses (PRISMA) flow diagram of the screening process for the present study, following recommended guidelines (Page et al., 2021). Relevant articles on Bivalvia growth experiments were identified from the Web of Science Core Collection using a series of keywords (see main text). Screening resulted in the identification of 79 relevant articles with 203 experimental set-ups that were included in our meta-analysis.
When extracting data from papers, we selected experiments that represented plausible end-of-century climate conditions or their location-specific expression (according to what study authors stated were plausible conditions based on the study location/bivalve examined), whereas we excluded physiological stress experiments, which often subject animals to artificially unnatural conditions. Data were extracted from studies that maintained constant experimental conditions, thereby also not exploring the natural diurnal variability, which is large in coastal settings (Dong et al., 2017; Hofmann et al., 2011), especially for species that are exposed to air at low tide. The data overview for individual species is available in our accompanying data.
We used growth as a broad measure of organism physiological response to climate stressors (and not more specific measures like shell thickness or soft tissue mass) for two main reasons. First, disentangling specific growth measurements would weaken our analysis due to the wide disparity in the approaches measuring growth responses to climate stressors. Second, using growth in this manner keeps our study in line with previous meta-analyses (Krocker et al., 2013; Harvey et al., 2013; Sampaio et al., 2021), allowing direct comparisons with past work with different foci. We opted against using survivorship because death is often recorded just as a percentage which is not sufficient for this type of analysis, as meta-analysis requires means, the standard deviation, and sample size being reported to calculate effect size.
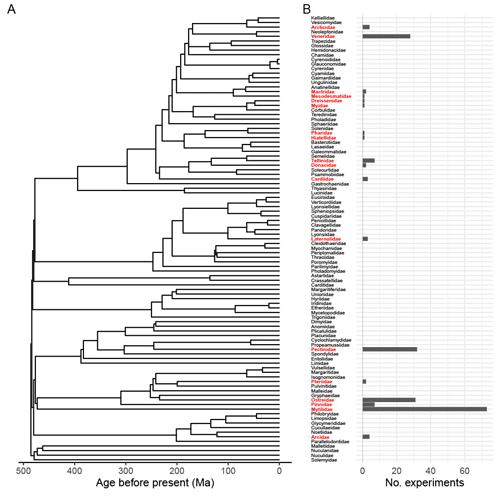
Figure 2Experimental representation of 18 Bivalvia families in 203 unique experimental set-ups from 79 relevant articles found in the Web of Science Core Collection. Panel (a) presents the time-scaled “budding II” phylogeny of extant Bivalvia from Crouch et al. (2021); the root age is 485.4 Ma. Panel (b) provides the number of experiments representing each extant family.
We included articles with lab-based studies that focused on direct measurements of Bivalvia growth, including length, mass, condition index, or shell thickness. Proxies for growth were excluded, such as scope for growth or RNA production, as these introduce additional uncertainties and variability into the growth signal and were not directly comparable to absolute measures of growth. Only studies where the bivalves were fed ad libitum and studies on larvae that developed without feeding were included, as nutrient intake has a strong impact on growth (Norkko et al., 2005; Thomsen et al., 2013; Ballesta-Artero et al., 2018). Study sample size, mean growth value of both control and treatment groups, and indication of the variation in growth values (confidence intervals, standard error, and standard deviation) were extracted from articles. Absolute values were used, as percentage data could not be combined with absolute measurements within the metafor package. Data were extracted directly from result text, tables, or supplementary data when possible. Data from figures were collected using WebPlotDigitizer (version 4.4; Rohatgi, 2022). Control values for climate stressors for each article were based on the article author's determination of control conditions for their respective bivalve. Climate stressor values were based on realistic end-of-century projections based on the article author's determination for that experimental set-up or study location. The phylogeny and column chart (Fig. 2) were plotted using R version 4.1.0 (R Core Team, 2021) and the following packages: ggplot2 (version 3.3.5; Wickham, 2016), ggtree (version 3.2.1; Yu et al., 2017), ape (version 5.6.1; Paradis and Schliep, 2019), and patchwork (version 1.1.1; Pederson, 2020). The topology was taken from the time-scaled “budding II” family-level phylogeny of Crouch et al. (2021).
2.2 Statistical analysis
We preformed meta-analysis on the impacts of climate stressors on the growth of Bivalvia at the whole-class and family levels following the methods described in Hoppit and Schmidt (2022). Stressors identified from the included experiments were water oxygen depletion (O2), increased acidity (decreased pH), salinity change (S), and temperature increase (T) as well as combinations of these stressors (indicated as e.g. O2 + pH) (Figs. 3, 4, 5; Table 2). Stressor effects could be synergistic (additive) or antagonistic (reductive) (sensu Harvey et al., 2013) or dominated by one stressor (unaffected by changes in another stressor). Additionally, we separated out the effect sizes of these stressors on different growth stages (egg/larva, juvenile, and adult) for the entire Bivalvia class.
Analyses used R version 4.0.3 (R Core Team, 2020) and the metafor package (version 3.0-2; Viechtbauer, 2010). The escalc metafor function was used to calculate the effect size and sampling variance. We chose the log-response ratio (LnRR; the natural log of the response ratio) as the measure of the effect size to measure the proportion of change between the mean of the treatment and control responses to experimental intervention. An effect size of zero corresponds to a statistically insignificant effect. Linear multivariate meta-analytical models (function rma.mv) were used to calculate the mean effect sizes of climate stressor impacts on bivalve growth rates for three subsets of data: all bivalves pooled, different developmental stages, and families with sufficient sample sizes (n≥7). Significant results were identified when the model 95 % confidence intervals did not overlap with the zero effect size. Models used random intercepts for articles and species intercepts for each treatment to compensate for similarities introduced by studies, as data originating from the same experimental set-up or from the same species are assumed more likely to be similar than data from different articles or species. Residual heterogeneity (QE), calculated as part of the meta-analytical models, was used to determine whether additional study moderators not considered might be influencing study results (Hedges and Olkin, 1985).
Publication bias was tested using Egger's regression test. Following Habeck and Schultz (2015), the rma.mv function was extended using the square root of the effect size variance in the model moderator variables to conduct a regression test. Egger's regression test looks at the symmetry of the data published and determines whether there are statistically “missing” studies within the spread of the papers published (Egger et al., 1997). We used meta-regression to determine whether published results had changed over the 25 years from which studies had been collated, using the study year as a moderator variable. This would indicate whether increasingly detailed knowledge has altered the overall picture with regards to the effect of each climate change stressor.
Our literature search produced the most detailed examination of bivalve growth rates under multiple climate stressors to date. We identified 79 studies with 203 unique experiments meeting the criteria, comprising 18 families and 37 species (Figs. 1, 2; Table 1). The sampling of families was highly uneven. Mytilidae made up 36 % of the experiments, and 81 % of the experiments include just four families: Mytilidae, Ostreidae, Pectinidae, and Veneridae. Including Pinnidae and Tellinidae increases the total to 88 % (Table 1).
We find consistent and significant negative effects of all single stressors and most combinations acting on the entire Bivalvia class, in agreement with previous meta-analyses (Fig. 3, Table 2). At the class level, many combinations of stressors increase the negative effect on growth in a synergistic way (Fig. 3, Table 2). For example, the pH and O2 treatments are greater in combination than either alone, as were salinity + temperature and pH + temperature. The effect of pH + salinity is intermediate between that of the two single stressors, while O2 + temperature causes a smaller effect than either single stressor. The combination of three stressors, O2 + pH + temperature, causes the strongest negative effect size compared with both individual stressors and any combinations. While low heterogeneity is preferable in terms of data validity, it is rarely achievable in environmental meta-analyses. Therefore, the significant heterogeneity in the data is expected given that it is drawn from so many disparate studies: QE = 300509.7155, degrees of freedom (df) = 148, P<0.0001.
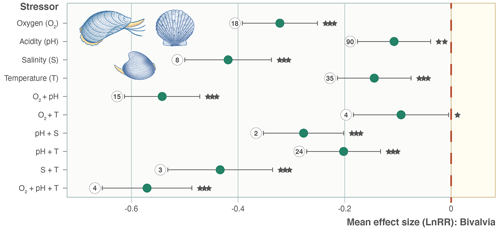
Figure 3Effect size (log-response ratio, LnRR) for the individual and combined effects of temperature (T), acidity (pH), oxygenation (O2), and salinity (S) as stressors on bivalve growth rates for all Bivalvia. Points represent the mean effect size, with error bars indicating the 95 % confidence intervals. Numbers indicate the number of included experiments. Significance is indicated with asterisks: , , .
Table 1Representation of bivalve families across 203 experimental studies included in this meta-analysis.
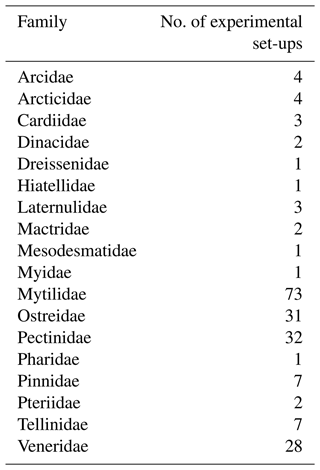
Table 2Single and combined stressor effect sizes from a meta-analysis of 203 experimental set-ups for bivalves (log-response ratio, LnRR).
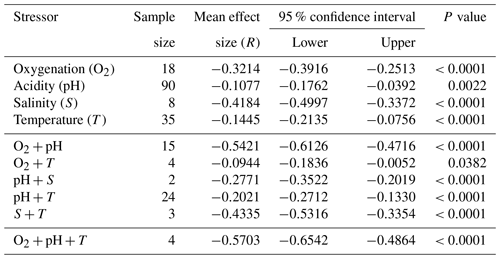
A total of 31 of the 203 experimental set-ups involve adult Bivalvia, 14 focused on unspecified ages/stages, 45 focused on eggs/larvae, and the remaining 113 focused on juvenile stages. Separation by growth stage shows that the combination of pH and O2 stressors causes a significantly negative effect size at all points in the life cycle (Fig. 4). Salinity is not an important stressor for larval or juvenile bivalves but causes a reduction in growth in adults. Juveniles show responses to most stressors, whereas eggs/larvae and adult bivalves have much smaller sample sizes and do not show significant effect size responses across the stressors.
Families do not all respond in the same way as the whole Bivalvia class, and stressors affect different families in unexpected ways (Fig. 5). Mytilidae, Ostreidae, and Pectinidae (67 % of experiments) respond with negative effect sizes for all individual stressors (Fig. 5a, b, c). Pinnidae show positive responses for the single T and pH stressors but negative responses when combined (Fig. 5d). Tellinidae show positive responses for O2 and O2 + pH (Fig. 5e). Veneridae (14 % of experiments) show mixed results, with significant negative effect sizes for S, pH + S, O2 + pH, and O2 + pH + T but strong positive responses to T and O2 + T (Fig. 5f).
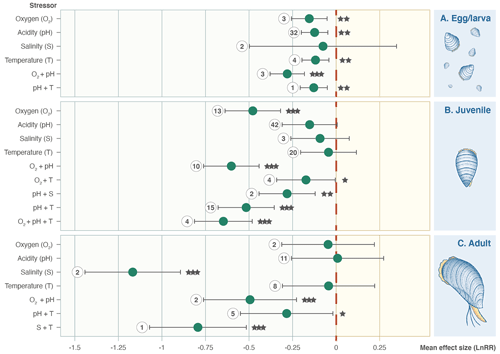
Figure 4Effect size (log-response ratio, LnRR) for the individual and combined effects of oxygenation (O2), acidity (pH), salinity (S), and temperature (T) as stressors on Bivalvia growth rates at different life stages (egg/larval, juvenile, and adult). Points represent the mean effect size, with error bars indicating the 95 % confidence intervals. Numbers indicate the number of included experiments. Significance is indicated with asterisks: , , .
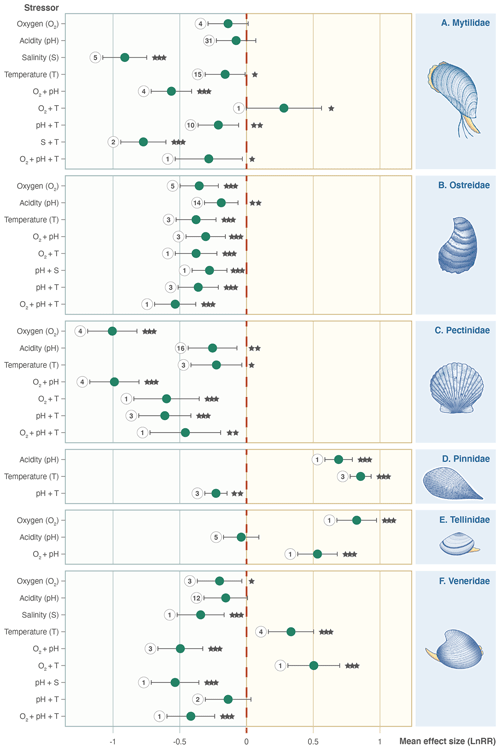
Figure 5Effect size (log-response ratio, LnRR) for the individual and combined effects of oxygenation (O2), acidity (pH), salinity (S), and temperature (T) as stressors on Bivalvia growth rates separated by family: (a) Mytilidae, (b) Ostreidae, (c) Pectinidae, (d) Pinnidae, (e) Tellinidae, and (f) Veneridae. Points represent the mean effect size, with error bars indicating the 95 % confidence intervals. Numbers indicate the number of included experiments. Significance is indicated with asterisks: , , .
Publications with significant results are published more often than would be expected by chance, suggesting that negative observations are less frequently reported (see Table A1 in Appendix A), as shown by the highly significant Egger's regression test (P<0.001) results for every stressor. Meta-regression analysis of publication by year and stressor showed that no individual stressor's effect size signal changes through time, supporting consistency in findings over the years (see Fig. B1 and Table B1 in Appendix B).
The impact of individual and combined climate stressors on growth rates of all bivalve molluscs in our study concurs with previous meta-analyses on marine calcifying invertebrates. Our analysis demonstrates that increased incidences of deoxygenation, pH decrease, and changes in temperature and salinity in nearshore marine environments in the future will inhibit the growth of bivalves. However, by separating out both the bivalve family-level response and different life stages, we build upon previous synthesis work by revealing a previously unappreciated complexity in these responses. The effects of climate change on bivalves will, in addition to the physicochemical environment, depend on the varied ecological and taxonomic makeup of specific habitats and will also vary across growth stages, which exploit the marine habitat differently. We also highlight numerous biases that exist in currently available studies (e.g. taxonomic, ecological, and geographic) which hinder the upscaling of the individual bivalve responses to a true global picture. Furthermore, our analysis hopes to move synthesis literature studies towards a higher taxonomic understanding of an individual group's responses to future ocean conditions by showing the level of variability that can be present once taxonomic distinctions within a group are teased out.
4.1 Climate change stressors will negatively impact bivalve growth
Our findings clearly show that growth rates in Bivalvia are negatively affected by climate stressors (Fig. 3). By exploring responses at the family level, we confirm our hypothesis that negative growth responses to climate change are likely inherent to this taxonomic group as a whole, despite the experimental bias in the published literature towards key families. Previous meta-analyses that incorporated bivalves did not focus on the group specifically but rather included them alongside numerous other taxa (i.e. within the phylum Mollusca) (Harvey et al., 2013; Kroeker et al., 2013; Sampaio et al., 2021). These analyses found little evidence of significant effect sizes except for a few single stressors (pH and temperature – Harvey et al., 2013; hypoxia – Sampaio et al., 2021). Unsurprisingly, the effect of temperature on bivalve growth is the most studied stressor in the experiments included in our meta-analysis (35 experiments; Fig. 3) – a feature seen in other studies of the effects of marine climate change (Borges et al., 2022). This bias is likely because temperature-altering experiments require less-complex equipment and sensors than pH or oxygen manipulation, have been performed over decades, and target the most obvious effect of climatic change (i.e. global warming). Inclusion of a substantially greater number of previous experiments within our meta-analysis (over 200 specific bivalve growth observations vs. 45, 46, and 34 Mollusca for Kroker et al., 2013; Harvey et al., 2013; and Sampaio et al., 2021, respectively) confirms that all single climate stressors show significant negative effect sizes in bivalves (Fig. 3). Our analysis also shows that, in many cases, this effect prevails when individual growth stages (Fig. 4) and the families containing the largest number of experiments or observational data (Fig. 5, Table 1) are examined separately.
An important result is the identification of synergistic, additive, and antagonistic effects between different stressors which, in all cases in our analysis, increase the negative response (Table 2; Gobler et al., 2014; Stevens and Gobler, 2018). For example, we identify significant negative effect sizes for O2 + pH, and T + S when analysing overall bivalve responses (Fig. 3). The combination O2 + pH has a stronger negative synergistic effect size compared with either O2 or pH individually in all analyses (Figs. 3, 4, 5). Decreases in pH restrict growth via restricting the availability of CO and increasing HCO3− ions, making shell building more metabolically expensive and increasing shell dissolution (Ivanina et al., 2013; Byrne and Fitzer, 2019). Internal tissues also require buffering against pH changes, incurring a further metabolic cost (Byrne and Fitzer, 2019). Marine deoxygenation impacts metabolism, reducing an organism's ability to respond to these increased metabolic requirements of shell generation and tissue buffering in a more acidic environment. Therefore, the increased impact of combining these two stressors confirms our physiological understanding of the organism (Pörtner and Farrell, 2008).
Mean effect sizes for each climate stressor differ between families within Bivalvia. Consequently, the effects of climate change on this group will be habitat dependent and alter the taxonomic composition of coastal ecosystems. The four most investigated families in our dataset (Mytilidae, Ostreidae, Pectinidae, and Veneridae) exhibit consistent negative growth responses to climate stressors (Fig. 5). Exceptions exist for oxygen and temperature changes for Mytilidae and Veneridae and temperature increase for Veneridae where we find mixed responses that are discussed in more detail below (Sect. 4.3). However, pH causes antagonistic decreases in the growth rate across these main families (Fig. 5), suggesting that any temperature-driven growth increases are unlikely to occur under projected future conditions.
Importantly, our examination of publication bias in the experimental literature shows that studies finding statistically significant results are more likely to be published than those reporting insignificant results. The likely explanation for this is there may be a bias in the experimental literature: authors may prioritize the publication of papers with significant results, leading to fewer papers or the delayed publication of papers with negative results (Nakagawa et al., 2022). Such a publication bias is well documented across many fields but hard to explicitly prove (Van Aert et al., 2019). While this bias likely skews our results towards collective negative growth rates, we can still be confident in our conclusions given the consistent decreases in bivalve growth rates across all stressor permutations. Research finding bivalves with neutral responses to climate change should be more readily published, as this will produce a better understanding of future marine ecosystems and allow scientists and conservationists to identify species or areas of resilience.
4.2 Different bivalve life stages and ecologies show distinct responses to climate stressors
Climate change will be acting on each part of the development of an organism. In bivalves, these different life stages have different habitats and mobility, from motile larvae to sessile adults. Our results on how different bivalve life stages are affected by a range of climate stressors generally confirm previous meta-analyses across calcifying organisms. Our results suggest that early life stages are the most vulnerable to a specific set of stressors and that the threat diminishes as organisms mature, supporting analyses by Sampaio et al. (2021) and Kroeker et al. (2013), who focused on the impacts of ocean acidification. However, it is important to note that the earlier developmental stages are more mobile and, hence, able to relocate their niche to track their environmental needs.
Combined climate stressors (e.g. pH + T, O2 + pH, and S + T) showed negative responses across all growth stages. Our findings oppose those of Harvey et al. (2013), who suggested limited variation in organism growth responses between life stages exposed to individual and synergistic ocean acidification and warming. In contrast to our analysis, their data were pooled from multiple phyla, reiterating the need to avoid too much pooling and averaging in meta-analysis.
Most studies in our dataset, especially those on the families that dominate our meta-analysis (Fig. 4), are focused on larval or juvenile stages. This likely explains some of the negative impacts on growth, as, for example, shell mineralogy influences the impact of pH changes. Amorphous calcium carbonate secreted by larval bivalves is 50 times more susceptible to dissolution than either calcite or aragonite (Bressan et al., 2014). Changes in body size or decreased shell thickness could increase vulnerability to predation (Sadler et al., 2018). Non-significant effects of lowered pH alone in adult bivalves likely result from more diverse shell mineralogy (Weiss et al., 2002), the effects of a more robust adult shell (Beadman et al., 2003, or individuals found in microhabitats with naturally low water pH where generational acclimation to low pH may have occurred (Thomsen et al., 2010; Hiebenthal et al., 2013)). The adult's lifestyle, which, for some taxa, includes exposure to air and/or closed valves while respiring naturally, results in high variability in pH in the calcifying fluid; therefore, the pH changes in the experiments possibly resulted in relatively less stress compared with earlier developmental stages. Most of the adult experiments included in our meta-analysis were on aragonitic individuals or on mixed aragonitic–calcitic Mytilidae and Pectinidae. Only one study (Lemasson et al., 2018) included two genera of adult oysters (Ostreidae family) which construct their shells primarily from calcite (Stenzel, 1963), a more stable carbonate polymorph.
Our results indicate that adults have an increased susceptibility to salinity changes compared with juvenile and egg/larval stages, suggesting that habitats projected to experience decreased salinity due to increased seasonal runoff or enhanced evaporation in a restricted setting (Robins et al., 2016) may become challenging for adult bivalves. It is important to note, however, that fewer experiments were conducted exploring salinity (13 experiments tested salinity out of 203 unique experimental set-ups), resulting in a low number of experimental studies and a greater need to determine the interaction with other drivers such as pH, temperature, and oxygen. Increased sensitivity to climate stressors at different life stages has implications for bivalve aquaculture and conservation efforts (Smaal et al., 2019), with the potential to be disruptive to lifecycles in some taxa. Decreased growth rates in larval and juvenile stages might impact population recruitment by limiting the number of individuals surviving to adulthood. Settlement efficacy will affect repopulation success following disturbance (Gagnon et al., 2021). Aquaculture and fisheries will need to account for these increased vulnerabilities and adapt culturing strategies to compensate for the negative growth impacts of climate change.
Efforts to restore historical bivalve populations, such as oysters in Chesapeake Bay (Bersoza Hernández et al., 2018) and Europe (Sas et al., 2020), will need to consider how climate stressors will impact population dynamics. Restoration projects often transplant adult or juvenile species into new environments (Johnson et al., 2019); our findings suggest that transplanting adults might be a preferable strategy given the lower impact of climate stressors at this developmental stage.
4.3 Consideration of habitat and ecology in the context of climate change
Many species belonging to the families Mytilidae, Ostreidae, and Veneridae occur in intertidal habitats which experience frequent fluctuations in oxygen, acidity, and temperature, and this has been hypothesized to provide some species with an innate ability to resist or mitigate the effects of future environmental change (Gazeau et al., 2013; Zhang et al., 2020). Furthermore, this high variability may include environments overlapping with those replicated in some of the experimental set-ups. In natural environments, species can evade some stressors via behavioural thermoregulation, for example, mobile infaunal bivalves have been shown to migrate to more offshore habitats or to burrow deeper into the sediment (Domínguez et al., 2020, 2021).
However, negative growth responses generally repeat across the taxa in our dataset irrespective of habitat. An intertidal habitat or preference for marine or brackish water does not appear to alter observed growth responses in the experimental setting to accumulated climate stressors. We find consistent decreases in growth rates across taxa, with commonly subtidal, epifaunal bivalves (such as many Pectinidae) also exhibiting negative responses (Aguirre-Velarde et al., 2019; Maynou et al., 2020). Interpreting the effects of ecology on our results is complicated by the previously mentioned dominance of studies focused on juvenile and early growth stages; many bivalves feature a veliger or early larval stage that lives in and can tolerate quite different environmental conditions to those of the later stages of life history (i.e. pelagic, motile larvae vs. infaunal or benthic attached lifestyles for juveniles and adults) (Waldbusser et al., 2013).
Examining the effect of other aspects of the bivalve mode of life on our results also reveals complexities in their response. Negative growth responses generally repeat across taxa irrespective of habitat. Most experiments in our dataset are conducted on suspension-feeding taxa with an epifaunal habitat. The investigated bivalves are motile (Pectinidae), cemented to substrates or form biogenic reefs (Ostreidae), or use byssal threads to anchor in sediments or attach to hard substrates (Pinnidae, Mytilidae, and some Pectinidae). In our dataset, there is much lower representation of infaunal or burrowing taxa which may also include deposit feeders (e.g. Tellinidae and Veneridae families). Our data suggest overwhelmingly negative impacts on growth of all stressors for epifaunal or motile suspension-feeding taxa (Mytilidae, Ostreidae, and Pectinidae families in Fig. 5).
Tellinidae and Veneridae show more varied responses to temperature, pH, and O2 depletion. These taxa are active infaunal burrowers in soft sediment, which can often be undersaturated with respect to calcium carbonate and limited with respect to oxygen (Green et al., 2013; Stevens and Gobler, 2018), suggesting some resilience to these conditions. Both semi-infaunal Pinnidae and infaunal Veneridae also show significant positive effect sizes in response to warming (Fig. 5). It has been hypothesized that an infaunal habitat may reduce the immediate susceptibility of bivalves to warming, as the substrate may act as a thermal refugia (Zhou et al., 2022). Taken together, these results suggests that lifestyle and habitat will control the ability of bivalves to evade climate stressors. However, interpreting the general role of ecology in providing resilience is complicated by the currently small number of experiments or observations on infaunal taxa, further highlighting the need for additional data on the effects of environmental stressors on the growth of burrowing bivalves and those from a wider range of specific shallow marine habitats.
4.4 Experimental studies of bivalve response are biased by commercially important taxa and have disparate protocols
Our meta-analysis clearly reveals that available data on bivalve growth responses to climate stressors contain a number of biases, likely due to the commercial importance of certain families (e.g. Mytilidae, Ostreidae, Pectinidae, and Veneridae) and individual species within them for aquaculture and common ecosystem services (e.g. van der Schatte Olivier et al., 2020) as well as the ease of access to specimens. Many experiments document that bivalve specimens were sourced from commercial aquaculture facilities. A number of families included in our meta-analysis are represented only by individual experiments – for example, Dreissenidae, Hitellidae, Mesodesmatidae, Myidae, and Pharidae. Comparison of the number of experiments vs. bivalve phylogeny shows that entire families have no documented experimental or observational work investigating climate stressor impacts on growth (Fig. 2). This confirms our hypothesis that, even though bivalves are considered a well-studied group, the signals detected in the literature are often driven by a subset of bivalve taxa.
While our results are based on studies with varying experimental protocols, our approach is consistent with other meta-analyses (e.g. Hoppit and Schmidt, 2022; Harvey et al., 2013; Kroeker et al., 2013; Sampaio et al., 2021). As we are using studies with disparate protocols and experimental measurements, the meta-regression analyses that we conducted (Fig. B1 in Appendix B) show that effect sizes across studies and experimental set-ups have not changed across time. This result suggests that variability in research practices does not impact our results. Some stressor combinations have low sample sizes, as multi-factor experiments are notoriously labour-intensive and difficult to perform. These lower numbers decrease confidence in those specific observations and conclusions and, thus, highlight the importance of considering a wider range of drivers than the most frequently assessed combination of warming and acidification alone.
Our findings are also geographically biased towards the “Global North”. Most studies clearly gathered organisms and data from the coasts of the USA, Europe, or China, resulting in important portions of the global ocean, like the Caribbean or African coasts, being unrepresented in these data. While our meta-analysis focused specifically on bivalve growth, this result emphasizes the unevenness of experimental research into this group. If this disparity of understanding is not rectified, implementing effective climate change adaptation and mitigation strategies and upscaling these results to ecosystem-scale changes will be challenging.
While our experimental sample is larger than previous meta-analyses, these biases also leave much uncertainty about how responses will scale up from commercially important species to other, rarely studied groups of bivalves, which, although of lesser importance for aquaculture or commercial exploitation, may act as keystone species within fragile marine ecosystems. This further limits the quality and quantity of available information that conservationists and stakeholders need to develop strategies to safeguard marine social-ecological systems. Given that our findings overwhelmingly suggest that bivalves as a group (Figs. 3, 4) and common, well-studied families (Fig. 5) will likely experience decreased growth rates under projected end-of-century conditions, one must also question how likely it is that families or species with no current experimental observations will also follow this trend. Therefore, additional experimental and observational work on specific bivalve species and families is urgently required, as such research would greatly assist with the development of conservation strategies for this important group of marine calcifiers.
The following points outline the main conclusions of this work:
-
Our results show that the growth rates of bivalve molluscs decrease when exposed to climate stressors with synergistic factors (e.g. the effects of combined temperature + O2 + pH change), causing greater reductions in bivalve growth then individual stressors. This result is true for bivalves overall as well as when separating them out by growth stage in the most commonly studied bivalve families (Ostreidae, Mytilidae, Pectinidae, and Veneridae). The reduced growth rates predicted by our meta-analysis have important implications for population stability in these commercially important keystone marine taxa as well as for guiding future conservation and mitigation efforts.
-
Epifaunal filter feeders, such as Ostreidae and Mytilidae, had mostly negative growth responses to environmental stressors. In contrast, infaunal and semi-infaunal suspension- or deposit-feeding bivalves, Veneridae, Tellinidae, and Pinnidae showed more mixed or even positive growth response under higher temperatures, suggesting that burrowing or buried taxa may be buffered from or resilient to some predicted changes. However, these data are based on a small number of studies, providing less confidence in the negative growth effects with other stressors and combinations of stressors.
-
By focusing on a few commercially relevant taxa, these commonly studied families are creating a bias in the literature. A large proportion of bivalve families lack any rigorous experimental or observational data, resulting in large knowledge gaps that hamper conservation efforts. Available data on the bivalve response to climate stressors also contain large biases towards early or juvenile growth stages and commercially important species from the Global North. Our results should be replicated for other commonly studied marine organisms like seaweeds or echinoderms to assess if taxonomic bias drives the commonly assumed physiological responses to climate change.
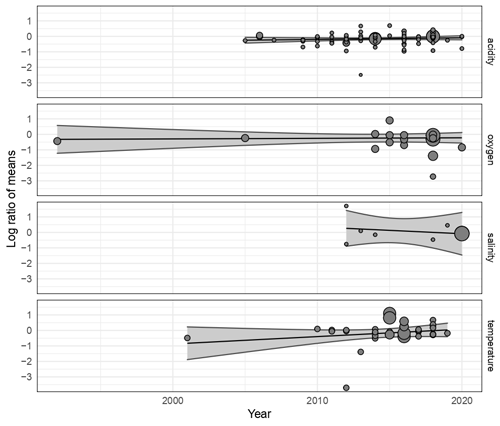
Figure B1Meta-regression change in effect sizes of 203 experimental set-ups on Bivalvia growth through time from 1997 to 2020 for acidity (pH), oxygen, salinity, and temperature. Each point shows the effect size against the dataset publication year. The point size indicates the experiment contribution weight to the linear model. Each plot shows the regression of the effect size against the publication year, with the 95 % confidence interval shaded. All regression analyses show no significant change during this period.
Article data can be found at https://doi.org/10.5281/zenodo.10118176 (Hoppit, 2023).
GH and BCM: conceptualization; RKW and GH: data curation, formal analysis, investigation, and methodology; RKW, GH, BCM, and JDW: resources, software, and validation; GH, JDW, and BCM: supervision; RKW, GH, and BCM: visualization; RKW: writing – original draft; RKW, GH, DNS, BCM, and JDW: writing – review and editing (https://credit.niso.org, last access: January 2023).
The contact author has declared that none of the authors has any competing interests.
Publisher’s note: Copernicus Publications remains neutral with regard to jurisdictional claims made in the text, published maps, institutional affiliations, or any other geographical representation in this paper. While Copernicus Publications makes every effort to include appropriate place names, the final responsibility lies with the authors.
The authors would like to thank Lisa Levine, Niels de Winter, and an anonymous reviewer for their support in improving the manuscript. Benjamin C. Moon is supported by the European Research Council (ERC) under the European Union's Horizon 2020 Research and Innovation programme (grant no. 788203), George Hoppit is supported by a NERC scholarship (grant no. NE/L002434/1), and Daniela N. Schmidt is supported by the Leverhulme Trust (grant no. RF-2021-489\4).
This paper was edited by Steven Bouillon and reviewed by Lisa Levin, Niels de Winter, and one anonymous referee.
Addino, M. S., Alvarez, M, F., Brey, T., Iribarne, O., and Lomovasky, B. J.: Growth changes of the stout razor clam Tagelus plebeius (Lightfoot, 1786) under different salinities in SW Atlantic estuaries, J. Sea. Res., 146, 14–23, https://doi.org/10.1016/j.seares.2019.01.005, 2019.
Aguirre-Velarde, A., Thouzeau, G., Jean, F., Mendo, J., Cueto-Vega, R., Kawazo-Delgado, M., Vasquez-Spencer, J., Herrera-Sanchez, D., Vega-Espinoza, A., and Flye-Sainte-Marie, J.: Chronic and severe hypoxic conditions in Paracas Bay, Pisco, Peru: Consequences on scallop growth, reproduction, and survival, Aquaculture, 512, 734259, https://doi.org/10.1016/j.aquaculture.2019.734259, 2019.
Baeta, M., Ramón, M., and Galimany, E.: Decline of a Callista chione (Bivalvia: Veneridae) bed in the Maresme coast (northwestern Mediterranean Sea), Ocean Coast Manage., 93, 15–25, https://doi.org/10.1016/j.ocecoaman.2014.03.001, 2014.
Ballesta-Artero, I., Janssen, R., van der Meer, J., and Witbaard, R.: Interactive effects of temperature and food availability on the growth of Arctica islandica (Bivalvia) juvenile, Mar. Environ. Res., 133, 67–77, https://doi.org/10.1016/j.marenvres.2017.12.004, 2018.
Bascur, M., Muñoz-Ramírez, C., Román-González, A., Sheen, K., Barnes, D. K., Sands, C. J., Brante, A., and Urzúa, Á.: The influence of glacial melt and retreat on the nutritional condition of the bivalve Nuculana inaequisculpta (Protobranchia: Nuculanidae) in the West Antarctic Peninsula, Plos One, 15, e0233513, https://doi.org/10.1371/journal.pone.0233513, 2020.
Benson, J. A., Stewart, B. A., Close, P. G., and Lymbery, A. J.: Freshwater mussels in Mediterranean-climate regions: Species richness, conservation status, threats, and Conservation Actions Needed, Aquat. Conserv., 31, 708–728, https://doi.org/10.1002/aqc.3511, 2021.
Bersoza Hernández, A., Brumbaugh, R. D., Frederick, P., Grizzle, R., Luckenbach, M. W., Peterson, C. H., and Angelini, C. L.: Restoring the eastern oyster: how much progress has been made in 53 years?, Front. Ecol. Environ., 16, 463–471, https://doi.org/10.1002/fee.1935, 2018.
Beadman, H., Caldow, R., Kaiser, M., and Willows, R.: How to toughen up your mussels: using mussel shell morphological plasticity to reduce predation losses, Mar. Biol., 142, 487–494, https://doi.org/10.1007/s00227-002-0977-4, 2003.
Beukema, J. J., Dekker, R., and Jansen, J. M.: Some like it cold: populations of the tellinid bivalve Macoma balthica (L.) suffer in various ways from a warming climate, Mar. Ecol. Prog. Ser., 384, 135–145, https://doi.org/10.3354/meps07952, 2009.
Bishop, M. J., Powers, S. P., Porter, H. J., and Peterson, C. H.: Benthic biological effects of seasonal hypoxia in a eutrophic estuary predate rapid coastal development, Estuar. Coast Shelf S., 70, 415–422, https://doi.org/10.1016/j.ecss.2006.06.031, 2006.
Borges, F. O., Sampaio, E., Santos, C. P., and Rosa, R.: Impacts of low oxygen on marine life: neglected, but a crucial priority for research, Biol. Bull., 243, 104–119, https://doi.org/10.1086/721468, 2022.
Breitburg, D., Levin, L. A., Oschlies, A., Grégoire, M., Chavez, F. P., Conley, D. J., Garçon, V., Gilbert, D., Gutiérrez, D., Isensee, K., and Jacinto, G. S.: Declining oxygen in the global ocean and coastal water, Science, 359, eaam7240, https://doi.org/10.1126/science.aam7240, 2018.
Bressan, M., Chinellato, A., Munari, M., Matozzo, V., Manci, A., Marčeta, T., Finos, L., Moro, I., Pastore, P., Badocco, D., and Marin, M. G.: Does seawater acidification affect survival, growth and shell integrity in bivalve juveniles?, Mar. Environ. Res., 99, 136–148, https://doi.org/10.1016/j.marenvres.2014.04.009, 2014.
Buelow, C. A. and Waltham, N. J.: Restoring tropical coastal wetland water quality: ecosystem service provisioning by a native freshwater bivalve, Aquat. Sci., 82, 1–16, https://doi.org/10.1007/s00027-020-00747-7, 2020.
Byrne, M. and Fitzer, S.: The impact of environmental acidification on the microstructure and mechanical integrity of marine invertebrate skeletons, Conserv. Physiol., 7, coz062, https://doi.org/10.1093/conphys/coz062, 2019.
Carss, D. N., Brito, A. C., Chainho, P., Ciutat, A., de Montaudouin, X., Otero, R. M. F., Filgueira, M. I., Garbutt, A., Goedknegt, M. A., Lynch, S. A., and Mahony, K. E.: Ecosystem services provided by a non-cultured shellfish species: The common cockle Cerastoderma edule, Mar. Environ. Res., 158, 104931, https://doi.org/10.1016/j.marenvres.2020.104931, 2020.
Clare, D. S., Robinson, L. A., and Frid, C. L. J.: Community variability and ecological functioning: 40 years of change in the North Sea benthos, Mar. Environ. Res., 107, 24–34, https://doi.org/10.1016/j.marenvres.2015.03.012, 2015.
Crouch, N. M., Edie, S. M., Collins, K. S., Bieler, R., and Jablonski, D.: Calibrating phylogenies assuming bifurcation or budding alters inferred macroevolutionary dynamics in a densely sampled phylogeny of bivalve families, P. Roy. Soc. B-Biol Sci., 288, 1964, https://doi.org/10.1098/rspb.2021.2178, 2021.
De Groot, S. J.: The impact of bottom trawling on benthic fauna of the North Sea, Ocean Manage., 9, 177–190, https://doi.org/10.1016/0302-184X(84)90002-7, 1984.
Domínguez, R., Vázquez, E., Woodin, S. A., Wethey, D. S., Peteiro, L. G., Macho, G., and Olabarria, C.: Sublethal responses of four commercially important bivalves to low salinity, Ecol. Indic., 111, 106031, https://doi.org/10.1016/j.ecolind.2019.106031, 2020.
Domínguez, R., Olabarria, C., Woodin, S. A., Wethey, D. S., Peteiro, L. G., Macho, G., and Vázquez, E.: Contrasting responsiveness of four ecologically and economically important bivalves to simulated heat waves, Mar. Environ. Res., 164, 105229, https://doi.org/10.1016/j.marenvres.2020.105229, 2021.
Dong, Y. W., Li, X. X., Choi, F. M., Williams, G. A., Somero, G. N., and Helmuth, B.: Untangling the roles of microclimate, behaviour and physiological polymorphism in governing vulnerability of intertidal snails to heat stress, P. Roy. Soc. B-Biol Sci., 284, 20162367, https://doi.org/10.1098/rspb.2016.2367, 2017.
Donnarumma, L., Sandulli, R., Appolloni, L., Sánchez-Lizaso, J. L., and Russo, G. F.: Assessment of Structural and Functional Diversity of Mollusc Assemblages within Vermetid Bioconstructions, Diversity, 10, 96, https://doi.org/10.3390/d10030096, 2018.
Egger, M., Smith, G. D., Schneider, M., and Minder, C.: Bias in meta-analysis detected by a simple, graphical test, Bmj, 7109, 629–634, https://doi.org/10.1136/bmj.315.7109.629, 1997.
Eymann, C., Götze, S., Bock, C., Guderley, H., Knoll, A. H., Lannig, G., Sokolova, I. M., Aberhan, M., and Pörtner, H. O.: Thermal performance of the European flat oyster, Ostrea edulis (Linnaeus, 1758) – explaining ecological findings under climate change, Mar. Biol., 167, 1–15, https://doi.org/10.1007/s00227-019-3620-3, 2020.
Fariñas-Franco, J. M. and Roberts, D.: Early faunal successional patterns in artificial reefs used for restoration of impacted biogenic habitats, Hydrobiologia, 727, 75–94, https://doi.org/10.1007/s10750-013-1788-y, 2014.
Gagnon, K., Rinde, E., Bengil, E. G., Carugati, L., Christianen, M. J., Danovaro, R., Gambi, C., Govers, L. L., Kipson, S., Meysick, L., and Pajusalu, L.: The potential for plant-bivalve interactions to improve habitat restoration success, J. Appl. Ecol., 57, 1161–1179, https://doi.org/10.1111/1365-2664.13605, 2020.
Gagnon, K., Christie, H., Didderen, K., Fagerli, C. W., Govers, L. L., Gräfnings, M. L., Heusinkveld, J. H., Kaljurand, K., Lengkeek, W., Martin, G., and Meysick, L.: Incorporating facilitative interactions into small-scale eelgrass restoration – challenges and opportunities, Restor. Ecol., 29, e13398, https://doi.org/10.1111/rec.13398, 2021.
Gazeau, F., Parker, L. M., Comeau, S., Gattuso, J. P., O'Connor, W. A., Martin, S., Pörtner, H. O., and Ross, P. M.: Impacts of ocean acidification on marine shelled molluscs, Mar. Biol., 160, 2207–2245, https://doi.org/10.1007/s00227-013-2219-3, 2013.
Genner, M. J., Halliday, N. C., Simpson, S. D., Southward, A. J., Hawkins, S. J., and Sims, D. W.: Temperature-driven phenological changes within a marine larval fish assemblage, J. Plankton. Res., 32, 699–708, https://doi.org/10.1093/plankt/fbp082, 2010.
Gobler, C. J., DePasquale, E. L., Griffith, A. W., and Baumann, H.: Hypoxia and acidification have additive and synergistic negative effects on the growth, survival, and metamorphosis of early life stage bivalves, PloS one, 9, e83648, https://doi.org/10.1371/journal.pone.0083648, 2014.
Green, M. A., Waldbusser, G. G., Hubazc, L., Cathcart, E., and Hall, J.: Carbonate mineral saturation state as the recruitment cue for settling bivalves in marine muds, Estuar. Coast., 36, 18–27, https://doi.org/10.1007/s12237-012-9549-0, 2013.
Habeck, C. W. and Schultz, A. K.: Community-level impacts of white-tailed deer on understorey plants in North American forests: a meta-analysis, AoB Plants, 7, plv119, https://doi.org/10.1093/aobpla/plv119, 2015.
Harvey, B. P., Gwynn-Jones, D., and Moore, P. J.: Meta-analysis reveals complex marine biological responses to the interactive effects of ocean acidification and warming, Ecol. Evol., 3, 1016–1030, https://doi.org/10.1002/ece3.516, 2013.
Hedges, L. V. and Olkin, I.: Statistical methods for meta-analysis, Academic Press, ISBN 0123363802, 1985.
Helmuth, B., Kingsolver, J. G., and Carrington, E.: Biophysics, physiological ecology, and climate change: does mechanism matter?, Annu. Rev. Physiol., 67, 177–201, https://doi.org/10.1146/annurev.physiol.67.040403.105027, 2005.
Hiebenthal, C., Philipp, E. E., Eisenhauer, A., and Wahl, M.: Effects of seawater pCO2 and temperature on shell growth, shell stability, condition and cellular stress of Western Baltic Sea Mytilus edulis (L.) and Arctica islandica (L.), Mar. Biol., 160, 2073–2087, https://doi.org/10.1007/s00227-012-2080-9, 2013.
Hofmann, G. E., Smith, J. E., Johnson, K. S., Send, U., Levin, L. A., Micheli, F., Paytan, A., Price, N. N., Peterson, B., Takeshita, Y., and Matson, P. G.: High-frequency dynamics of ocean pH: a multi-ecosystem comparison, Plos one, 6, e28983, https://doi.org/10.1371/journal.pone.0028983, 2011.
Hoppit, G. and Schmidt, D. N.: A Regional View of the Response to Climate Change: A Meta-Analysis of European Benthic Organisms' Responses, Front. Mar., 9, 896157, https://doi.org/10.3389/fmars.2022.896157, 2022.
Hoppit, G.: Data for: The Clam Before the Storm: A Meta Analysis Showing the Effect of Combined Climate Change Stressors on Bivalves, Zenodo [data set], https://doi.org/10.5281/zenodo.10118176, 2023.
IPCC, 2021: Climate Change 2021: The Physical Science Basis. Contribution of Working Group I to the Sixth Assessment Report of the Intergovernmental Panel on Climate Change, edited by: Masson-Delmotte, V., Zhai, P., Pirani, A., Connors, S. L., Péan, C., Berger, S., Caud, N., Chen, Y., Goldfarb, L., Gomis, M. I., Huang, M., Leitzell, K., Lonnoy, E., Matthews, J. B. R., Maycock, T. K., Waterfield, T., Yelekçi, O., Yu, R., and Zhou, B., Cambridge University Press, Cambridge, United Kingdom and New York, NY, USA, 2391 pp., https://doi.org/10.1017/9781009157896.2, 2021.
Ivanina, A. V., Dickinson, G. H., Matoo, O. B., Bagwe, R., Dickinson, A., Beniash, E., and Sokolova, I. M.: Interactive effects of elevated temperature and CO2 levels on energy metabolism and biomineralization of marine bivalves Crassostrea virginica and Mercenaria mercenaria, Comp. Biochem. Phys. A., 166, 101–111, https://doi.org/10.1016/j.cbpa.2013.05.016, 2013.
Johnson, E. E., Medina, M. D., Hernandez, A. C. B., Kusel, G. A., Batzer, A. N., and Angelini, C.: Success of concrete and crab traps in facilitating Eastern oyster recruitment and reef development, Peerj, 7, e6488, https://doi.org/10.7717/peerj.6488, 2019.
Kamermans, P. and Saurel, C.: Interacting climate change effects on mussels (Mytilus edulis and M. galloprovincialis) and oysters (Crassostrea gigas and Ostrea edulis): experiments for bivalve individual growth models, Aquat. Living Resour., 35, 1, https://doi.org/10.1051/alr/2022001, 2022.
Knights, A. M., Norton, M. J., Lemasson, A. J., and Stephen, N.: Ocean acidification mitigates the negative effects of increased sea temperatures on the biomineralization and crystalline ultrastructure of Mytilus, Front. Mar., 7, 773, https://doi.org/10.3389/fmars.2020.567228, 2020.
Kroeker, K. J., Kordas, R. L., Crim, R. N., and Singh, G. G.: Meta-analysis reveals negative yet variable effects of ocean acidification on marine organisms, Ecol. Lett. 13, 1419–1434, https://doi.org/10.1111/j.1461-0248.2010.01518.x, 2010.
Kroeker, K. J., Kordas, R. L., Crim, R., Hendriks, I. E., Ramajo, L., Singh, G. S., Duarte, C. M., and Gattuso, J. P.: Impacts of ocean acidification on marine organisms: quantifying sensitivities and interaction with warming, Glob. Change Biol., 19, 1884–1896, https://doi.org/10.1111/gcb.12179, 2013.
Lemasson, A. J., Hall-Spencer, J. M., Fletcher, S., Provstgaard-Morys, S., and Knights, A. M.: Indications of future performance of native and non-native adult oysters under acidification and warming, Mar. Environ. Res., 142, 178–189, https://doi.org/10.1016/j.marenvres.2018.10.003, 2018.
Leung, J. Y., Zhang, S., and Connell, S. D.: Is Ocean Acidification Really a Threat to Marine Calcifiers?, A Systematic Review and Meta-Analysis of 980+ Studies Spanning Two Decades, Small, 18, 2107407, https://doi.org/10.1002/smll.202107407, 2022.
Lu, Y., Li, Y., Duan, J., Lin, P., and Wang, F.: Multidecadal Sea Level Rise in the Southeast Indian Ocean: The Role of Ocean Salinity Change, J. Climate, 35, 1479–1496, https://doi.org/10.1175/JCLI-D-21-0288.1, 2022.
Martínez, M. L., Intralawan, A., Vázquez, G., Pérez-Maqueo, O., Sutton, P., and Landgrave, R.: The coasts of our world: ecological, economic and social importance, Ecol. Econ., 63, 254–272, https://doi.org/10.1016/j.ecolecon.2006.10.022, 2007.
Maynou, F., Galimany, E., Ramón, M., and Solé, M.: Impact of temperature increase and acidification on growth and the reproductive potential of the clam Ruditapes philippinarum using DEB, Estuar. Coast. Shelf. S., 247, 107099, https://doi.org/10.1016/j.ecss.2020.107099, 2020.
McAfee, D., O'Connor, W. A., and Bishop, M. J.: Fast-growing oysters show reduced capacity to provide a thermal refuge to intertidal biodiversity at high temperatures, J. Anim. Ecol., 86, 1352–1362, https://doi.org/10.1111/1365-2656.12757, 2017.
Meredith, M., Sommerkorn, M., Cassotta, S., Derksen, C., Ekaykin, A., Hollowed, A., Kofinas, G., Mackintosh, A., Melbourne-Thomas, J., Muelbert, M. M. C., and Ottersen, G.: Polar Regions. Chapter 3, IPCC Special Report on the Ocean and Cryosphere in a Changing Climate, IPCC, Cambridge, UK and New York, NY, USA, 203–320, https://doi.org/10.1017/9781009157964.005, 2019.
Messié, M. and Chavez, F. P.: Nutrient supply, surface currents, and plankton dynamics predict zooplankton hotspots in coastal upwelling systems, Geophys. Res. Lett., 44, 8979–8986, https://doi.org/10.1002/2017GL074322, 2017.
Montalto, V., Helmuth, B., Ruti, P. M., Dell'Aquila, A., Rinaldi, A., and Sarà, G.: A mechanistic approach reveals non-linear effects of climate warming on mussels throughout the Mediterranean Sea, Clim. Change, 139, 293–306, https://doi.org/10.1007/s10584-016-1780-4, 2016.
Nakagawa, S., Lagisz, M., Jennions, M. D., Koricheva, J., Noble, D. W., Parker, T. H., Sánchez-Tójar, A., Yang, Y., and O'Dea, R. E.: Methods for testing publication bias in ecological and evolutionary meta-analyses, Methods Ecol. Evol., 13, 4–21, https://doi.org/10.1111/2041-210X.13724, 2022.
Neumann, B., Vafeidis, A. T., Zimmermann, J., and Nicholls, R. J.: Future coastal population growth and exposure to sea-level rise and coastal flooding – a global assessment, Plos one, 10, e0118571, https://doi.org/10.1371/journal.pone.0131375, 2015.
Norkko, J., Pilditch, C. A., Thrush, S. F., and Wells, R. M. G.: Effects of food availability and hypoxia on bivalves: the value of using multiple parameters to measure bivalve condition in environmental studies, Mar. Ecol. Prog. Ser., 298, 205–218, https://doi.org/10.3354/meps298205, 2005.
Olivier, F., Gaillard, B., Thébault, J., Meziane, T., Tremblay, R., Dumont, D., Bélanger, S., Gosselin, M., Jolivet, A., Chauvaud, L., and Martel, A. L.: Shells of the bivalve Astarte moerchi give new evidence of a strong pelagic-benthic coupling shift occurring since the late 1970s in the North Water polynya, Philos. T. Roy. Soc. A, 378, 20190353, https://doi.org/10.1098/rsta.2019.0353, 2020.
Page, M. J., McKenzie, J. E., Bossuyt, P. M., Boutron, I., Hoffmann, T. C., Mulrow, C. D., Shamseer, L., Tetzlaff, J. M., Akl, E. A., Brennan, S. E., and Chou, R.: The PRISMA 2020 statement: an updated guideline for reporting systematic reviews, Int. J. Surg., 88, 105906, https://doi.org/10.1016/j.ijsu.2021.105906, 2021.
Paradis, E. and Schliep, K.: ape 5.0: an environment for modern phylogenetics and evolutionary analyses in R, Bioinformatics, 35, 526–528, https://doi.org/10.1093/bioinformatics/bty633, 2019.
Pendersen, T. L.: Patchwork: The composer of plots, https://github.com/thomasp85/patchwork (last access: December 2022), 2020.
Pörtner, H. O. and Farrell, A. P.: Physiology and climate change, Science, 322, 690–692, https://doi.org/10.1126/science.11631, 2008.
R Core Team, R: A language and environment for statistical computing, R Found. for Stat. Comput., Vienna, https://www.R-project.org/ (last access: January 2023), 2021.
Robins, P. E., Skov, M. W., Lewis, M. J., Giménez, L., Davies, A. G., Malham, S. K., Neill, S. P., McDonald, J. E., Whitton, T. A., Jackson, S. E., and Jago, C. F.: Impact of climate change on UK estuaries: A review of past trends and potential projections, Estuar. Coast. Shelf. S., 169, 119–135, https://doi.org/10.1016/j.ecss.2015.12.016, 2016.
Rohatgi, A.: Web Plot Digitizer User Manual Version 4.3, https://automeris.io/WebPlotDigitizer/userManual.pdf (last access: November 2022), 2022.
Sadler, D. E., Lemasson, A. J., and Knights, A. M.: The effects of elevated CO2 on shell properties and susceptibility to predation in mussels Mytilus edulis, Mar. Environ. Res., 139, 162–168, https://doi.org/10.1016/j.marenvres.2018.05.017, 2018.
Sampaio, E., Santos, C., Rosa, I. C., Ferreira, V., Pörtner, H. O., Duarte, C. M., Levin, L. A., and Rosa, R.: Impacts of hypoxic events surpass those of future ocean warming and acidification, Nat. Ecol. Evol., 5, 311–321, https://doi.org/10.1038/s41559-020-01370-3, 2021.
Sas, H., Kamermans, P., zu Ermgassen, P. S., Pogoda, B., Preston, J., Helmer, L., Holbrook, Z., Arzul, I., van der Have, T., Villalba, A., and Colsoul, B.: Bonamia infection in native oysters (Ostrea edulis) in relation to European restoration projects, Aquat. Conserv., 30, 2150–2162, https://doi.org/10.1002/aqc.3430, 2020.
Schmidtko, S., Stramma, L., and Visbeck, M.: Decline in global oceanic oxygen content during the past five decades, Nature, 542, 335–339, https://doi.org/10.1038/nature21399, 2017.
Selig, E. R., Hole, D. G., Allison, E. H., Arkema, K. K., McKinnon, M. C., Chu, J., de Sherbinin, A., Fisher, B., Glew, L., Holland, M. B., and Ingram, J. C.: Mapping global human dependence on marine ecosystems, Conserv. Lett., 12, e12617, https://doi.org/10.1111/conl.12617, 2019.
Smaal, A. C., Ferreira, J. G., Grant, J., Petersen, J. K., and Strand, Ø.: Goods and Services of Marine Bivalves, Springer Nature, https://doi.org/10.1007/978-3-319-96776-9, 2019.
Smyth, A. R., Murphy, A. E., Anderson, I. C., and Song, B.: Differential Effects of Bivalves on Sediment Nitrogen Cycling in a Shallow Coastal Bay, Estuar. Coast., 41, 1147–1163, https://doi.org/10.1007/s12237-017-0344-9, 2018.
Smyth, A. R., Geraldi, N. R., and Piehler, M. F.: Oyster-mediated benthic-pelagic coupling modifies nitrogen pools and processes, Mar. Ecol. Prog. Ser., 493, 23–30, https://doi.org/10.3354/meps10516, 2013.
Spooner, D. E. and Vaughn, C. C.: A trait-based approach to species' roles in stream ecosystems: Climate change, community structure, and material recycling, Oecologia, 158, 307–317, https://doi.org/10.1007/s00442-008-1132-9, 2008.
Stenzel, H. B.: Aragonite and calcite as constituents of adult oyster shells, Science, 142, 232–233, https://doi.org/10.1126/science.142.3589.23, 1963.
Stevens, A. M. and Gobler, C. J.: Interactive effects of acidification, hypoxia, and thermal stress on growth, respiration, and survival of four North Atlantic bivalves, Mar. Ecol. Prog. Ser., 604, 143–161, https://doi.org/10.3354/meps12725, 2018.
Strain, E. M., Steinberg, P. D., Vozzo, M., Johnston, E. L., Abbiati, M., Aguilera, M. A., Airoldi, L., Aguirre, J. D., Ashton, G., Bernardi, M., and Brooks, P.: A global analysis of complexity–biodiversity relationships on marine artificial structures, Global Ecol. Biogeogr., 30, 140–153, https://doi.org/10.1111/geb.13202, 2021.
Sydeman, W. J., García-Reyes, M., Schoeman, D. S., Rykaczewski, R. R., Thompson, S. A., Black, B. A., and Bograd, S. J.: Climate change and wind intensification in coastal upwelling ecosystems, Science, 345, 77–80, https://doi.org/10.1126/science.1251635, 2014.
Tallqvist, M.: Burrowing behaviour of the Baltic clam Macoma balthica: effects of sediment type, hypoxia and predator presence, Mar. Ecol. Prog. Ser., 212, 183–191, https://doi.org/10.3354/meps212183, 2001.
Thomsen, J., Casties, I., Pansch, C., Körtzinger, A., and Melzner, F.: Food availability outweighs ocean acidification effects in juvenile M ytilus edulis: laboratory and field experiments, Glob. Change Biol., 19, 1017–1027, https://doi.org/10.1111/gcb.12109, 2013.
Thomsen, J., Gutowska, M. A., Saphörster, J., Heinemann, A., Trübenbach, K., Fietzke, J., Hiebenthal, C., Eisenhauer, A., Körtzinger, A., Wahl, M., and Melzner, F.: Calcifying invertebrates succeed in a naturally CO2-rich coastal habitat but are threatened by high levels of future acidification, Biogeosciences, 7, 3879–3891, https://doi.org/10.5194/bg-7-3879-2010, 2010.
Van Aert, R. C., Wicherts, J. M., and Van Assen, M. A.: Publication bias examined in meta-analyses from psychology and medicine: A meta-meta-analysis, Plos one, 14, e0215052, https://doi.org/10.1371/journal.pone.0215052, 2019.
Van Colen, C., Debusschere, E., Braeckman, U., Van Gansbeke, D., and Vincx, M.: The Early Life History of the Clam Macoma balthica in a High CO2 World, Plos one, 7, e44655, https://doi.org/10.1371/journal.pone.0044655, 2012.
van der Schatte Olivier, A., Jones, L., Vay, L. L., Christie, M., Wilson, J., and Malham, S. K.: A global review of the ecosystem services provided by bivalve aquaculture, Rev. Aquacult., 12, 3–25, https://doi.org/10.1111/raq.12301, 2020.
Vaughn, C. C.: Biodiversity losses and ecosystem function in freshwaters: emerging conclusions and research directions, Bioscience, 60, 25–35, https://doi.org/10.1525/bio.2010.60.1.7, 2010.
Vaughn, C. C. and Hoellein, T. J.: Bivalve impacts in freshwater and marine ecosystems, Annu. Rev. Ecol. Evol. S., 49, 183–208, https://doi.org/10.1146/annurev-ecolsys-110617-062703, 2018.
Viechtbauer, W.: Conducting meta-analyses in R with the metafor package, J. Stat. Softw. 36, 1–48, https://doi.org/10.18637/jss.v036.i03, 2010.
Waldbusser, G. G., Brunner, E. L., Haley, B. A., Hales, B., Langdon, C. J., and Prahl, F. G.: A developmental and energetic basis linking larval oyster shell formation to acidification sensitivity, Geophys. Res. Lett., 40, 2171–2176, https://doi.org/10.1002/grl.50449, 2013.
Ward, J. E. and Shumway, S. E.: Separating the grain from the chaff: particle selection in suspension-and deposit-feeding bivalves, J. Exp. Mar. Biol. Ecol., 300, 83–130, https://doi.org/10.1016/j.jembe.2004.03.002, 2004.
Weiss, I. M., Tuross, N., Addadi, L. I. A., and Weiner, S.: Mollusc larval shell formation: amorphous calcium carbonate is a precursor phase for aragonite, J. Exp. Zool., 293, 478–491, https://doi.org/10.1002/jez.90004, 2002.
Wickham, H.: Programming with ggplot2, Springer, Cham, https://doi.org/10.1007/978-3-319-24277-4_ 12, 2016.
Yu, G., Smith, D. K., Zhu, H., Guan, Y., and Lam, T. T. Y:. ggtree: an R package for visualization and annotation of phylogenetic trees with their covariates and other associated data, Meth. Ecol. Evol., 8, 28–36, https://doi.org/10.1111/2041-210X.12628, 2017.
Zhang, W. Y., Storey, K. B., and Dong, Y. W.: Adaptations to the mudflat: Insights from physiological and transcriptional responses to thermal stress in a burrowing bivalve Sinonovacula constricta, Sci. Total Environ., 710, 136280, https://doi.org/10.1016/j.scitotenv.2019.136280, 2020.
Zhou, Z., Bouma, T. J., Fivash, G. S., Ysebaert, T., van IJzerloo, L., van Dalen, J., van Dam, B., and Walles, B.: Thermal stress affects bioturbators' burrowing behavior: A mesocosm experiment on common cockles (Cerastoderma edule), Sci. Total Environ., 824, 153621, https://doi.org/10.1016/j.scitotenv.2022.153621, 2022.
Zu Ermgassen, P. S., Thurstan, R. H., Corrales, J., Alleway, H., Carranza, A., Dankers, N., DeAngelis, B., Hancock, B., Kent, F., McLeod, I., and Pogoda, B.: The benefits of bivalve reef restoration: A global synthesis of underrepresented species, Aquat. Conserv., 30, 2050–2065, https://doi.org/10.1002/aqc.3410, 2020.