the Creative Commons Attribution 4.0 License.
the Creative Commons Attribution 4.0 License.
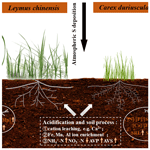
Plant functional traits modulate the effects of soil acidification on above- and belowground biomass
Xue Feng
Ruzhen Wang
Tianpeng Li
Jiangping Cai
Heyong Liu
Hui Li
Yong Jiang
Atmospheric sulfur (S) deposition has been increasingly recognized as a major driver of soil acidification. However, little is known about how soil acidification influences above- and belowground biomass by altering leaf and root traits. We conducted a 3-year S-addition experiment to simulate soil acidification in a meadow. Grass (Leymus chinensis (Trin.) Tzvelev) and sedge (Carex duriuscula C.A.Mey) species were chosen to evaluate the linkage between plant traits and biomass. Sulfur addition led to soil acidification and nutrient imbalances. Soil acidification decreased specific leaf area (SLA) but increased leaf dry-matter content (LDMC) in L. chinensis, showing a conservative strategy and thus suppressing aboveground instead of belowground biomass. However, in C. duriuscula, soil acidification increased plant height and root nutrients (N, P, S, and Mn), favoring competition for natural resources through enhanced above- and belowground biomass, i.e., adoption of an acquisitive strategy. Increased soil acidity resulted in an overall reduction in aboveground community biomass by 3 %–33 %, but it led to an increase in community root biomass by 11 %–22 % due to upregulation as a result of higher soil nutrient availability. Our results demonstrate that both above- and belowground plant biomass is affected by S-induced acidification. Understanding the linkage between plant biomass and functional traits contributes to a better understanding of plant–soil feedback in grassland ecosystems.
- Article
(2671 KB) - Full-text XML
-
Supplement
(471 KB) - BibTeX
- EndNote
Acid deposition, as a consequence of anthropogenic activities, has significant impacts on terrestrial biodiversity and ecosystem functions (Tian and Niu, 2015; Clark et al., 2019; Yang et al., 2021). Atmospheric sulfur (S) deposition is one of the main causes of soil acidification, with its effects equal to or surpassing those of nitrogen (N) deposition in Asia (Duan et al., 2016; Zhang et al., 2022). Despite a large decrease in average S deposition across China over the past decades, the problem is still very severe in northeast China and Inner Mongolia (Yu et al., 2017). The northern grasslands of China, an integral part of the Eurasian grasslands, experienced severe soil acidification with a significant decline in mean soil pH from 7.84 to 7.21 during the 1980s–2000s, and S deposition undoubtedly accelerates this process (Yang et al., 2012). Therefore, soil acidification has become a major global concern; it not only leads to soil nutrient imbalances but also decreases the productivity of terrestrial ecosystems (Chen et al., 2013a; Tibbett et al., 2019; Duddigan et al., 2021).
In natural ecosystems, sulfur is an essential nutrient element in forming plant proteins as it is a constituent of certain amino acids (Vitousek and Howarth, 1991; Garrison et al., 2000). Shifts in plant species and communities associated with S deposition are mainly a consequence of soil acidification rather than an effect of S fertilization (Clark et al., 2019). This is because soil pH is a primary regulator of nutrient availability, which plant growth and species coexistence rely on (Bolan et al., 2003; Tibbett et al., 2019). For instance, soil acidification inhibits nitrification (Kemmitt et al., 2005) but facilitates the release of soil-available phosphorus (P) and micronutrients and the leaching of soil base cations (Jaggi et al., 2005; Chen et al., 2015; Feng et al., 2019). Evidence from a previous manipulative S-addition experiment has shown that aboveground biomass (AGB) generally decreases with soil acidification, except for highly acid-tolerant species of sedges, which exhibit a reversed pattern of responses in subalpine grasslands (Leifeld et al., 2013). The acidification-mediated decreases in soil cation concentrations (such as Ca2+ and NO) could increase the relative abundance of acid-tolerant and oligotrophic species (van Dobben and de Vries, 2010; Clark et al., 2019) as a result of other species decreasing in abundance (Jung et al., 2018). Additionally, soil Mn toxicity caused by soil acidification in calcareous grasslands asymmetrically reduces the aboveground biomass of different species and functional groups through the suppression of photosynthesis (Tian et al., 2016).
Findings from a global meta-analysis predominantly focusing on forest ecosystems show negative acidification effects on root biomass affected by sulfuric acid addition (Meng et al., 2019). This is because forest soils with a low initial pH (pH <5) generally exhibit higher levels of Al3+ and Fe3+ but fewer base cations, thereby inhibiting of root growth (Li et al., 2018). In contrast to findings from forests, in typical and alpine grasslands, belowground biomass increases with soil acidification, which is mainly due to the compensatory growth that is concomitant with the dominance of graminoids over forbs (Chen et al., 2015; Wang et al., 2020). Plausibly, perennial rhizomatous grasses and sedges have a higher ionic tolerance (such as that regarding H+, Al3+, NH, and SO) than perennial bunchgrasses and forbs, which allows for the maintenance of high levels of community biomass under soil acidification (Chen et al., 2015; Cliquet and Lemauviel-Lavenant, 2019; Wang et al., 2020). Therefore, shifts in grassland communities are mainly regulated by soil nutrient fluctuations induced by soil acidification, which eventually affect both above- and belowground biomass (Mitchell et al., 2018; Wang et al., 2020).
Functional traits are known to substantially influence plant survival, growth, and reproduction through close association with a plant's capability for resource acquisition (Violle et al., 2007). To cope with environmental stresses in terms of persistence and reproduction, plants often rely on possessing a broad spectrum of functional traits, ranging from conservative to proactive strategies of resource acquisition (De Battisti et al., 2020). For example, some plant species can upregulate tissue nutrients as a fast resource acquisition strategy when the environmental conditions of soil become challenging (Mueller et al., 2012). In contrast, some plant species under environmental stresses tend to be more nutrient conservative in that they generally have long-lasting leaves with a low specific leaf area (SLA) but a high leaf dry-matter content (LDMC) (Kandlikar et al., 2022). Grass species may also increase their root length to avoid acid and Al3+ stresses (Göransson et al., 2011). In general, studies show that species with an acquisitive strategy accumulate biomass more rapidly, whereas those with a conservative strategy exhibit a slower pace of biomass growth to prolong their lifespan (Reich, 2014; Hao et al., 2020).
Due to difficulties in measuring grassland root traits in situ, we still have very limited knowledge of how root trait strategies are linked to the response of belowground processes to soil acidification. It is known that some plants can cope with nutrient deficiency in acidic soils through modifications of their root morphologies as well as through their nutrient uptake capability and metabolic adjustment (Hammond et al., 2004). Plants growing in resource-poor soils tend to have a lower specific root length (SRL) and lower root nutrient concentrations for the conservation of resources (Delpiano et al., 2020). Findings from a pot experiment show that the root length of perennial grasses decreases with soil acidification, demonstrating the constraint on root development under environmental stresses (Haling et al., 2010). However, in natural ecosystems, it has been found that grasses develop densely branched root systems with a higher nutrient use efficiency and are more stress tolerant with regard to nutrient deficiency in order to maintain nutrient balance and growth (Tian et al., 2022). Moreover, both aboveground and belowground biomass might be strongly influenced by specific functional traits (Clark et al., 2019; Wang et al., 2020), soil nutrient availability, and nutrient contents in leaves and roots under soil acidification (Geng et al., 2014; Rabêlo et al., 2018; Tian et al., 2021). To date, it still remains elusive how the functional traits of both above- and belowground components of different species respond to soil acidification.
To understand how soil acidification resulting from S deposition influences plant traits, biomass, and the linkage between the two in coping with S-induced stress, we conducted an S-addition experiment with eight loading rates (from 0 to 50 g S m−2 yr−1) to simulate different levels of soil acidification in a meadow grassland. We assessed the role of plant above- and belowground traits and abiotic soil variables in affecting the biomass of two dominant and contrasting plant species (Leymus chinensis (Trin.) Tzvelev and Carex duriuscula C.A.Mey) under soil acidification. Specifically, we aim to examine how the relationships between the functional traits of biomass and plant functional traits are modified by changes in soil conditions. As a perennial rhizomatous grass, L. chinensis is widely distributed in arid and semi-arid areas of northern China. This species is found in the top layers of local grassland communities, and as such, likely has an advantage in resource acquisition, especially in that regarding light. Additionally, grasses generally exhibit flexibility in absorbing various forms of soil N, thereby expanding their ecological niche (Grassein et al., 2015). The perennial rhizomatous sedge C. duriuscula is a shorter subordinate species and an indicator plant for soil degradation; it possesses cluster roots and tends to consume more photosynthetic products to increase its ability to acquire nutrients (Zhang et al., 2021). Both species exhibit distinct rhizosheaths that enable them to tightly adhere to the soil and show compensatory growth in response to environmental disturbance (Tian et al., 2022). Our study addresses the following questions. (i) How do soil properties (i.e., soil pH, Ca2+, Al3+, available N, and available P), above- and belowground plant traits (i.e., morphological and nutrient traits), and biomass respond to different doses of S addition in a meadow grassland? (ii) What are the key plant functional traits that regulate the biomass responses of two species to soil acidification? We hypothesized that soil acidification caused by S addition would lead to nutrient imbalances in the meadow grassland soil. The grass, L. chinensis, may respond to soil acidification by adjusting its aboveground light acquisition traits for biomass growth, whereas the sedge, C. duriuscula, may adopt a strategy of increased tissue nutrient concentrations to enhance its tolerance to soil acidification, consequently leading to compensatory root growth (Fig. S1 in the Supplement).
2.1 Experimental site and design
This study was conducted at the Erguna Forest-Steppe Ecotone Research Station (50°10′ N, 119°23′ E) of the Chinese Academy of Sciences in Inner Mongolia, China. The area lies within a transitional climate zone with a mid- to cold-temperate climate and a mean annual temperature and precipitation of −2.45 °C and 363 mm, respectively (Feng et al., 2019). Soil at the experimental site is classified as a Haplic Chernozem soil in accordance with the Food and Agriculture Organization of the United Nations classification (IUSS Working Group WRB, 2014) and is composed of 37±0.9 % sand, 40±1.0 % silt, and 24±0.8 % clay (Li et al., 2021). Vegetation in this area comprises a meadow steppe community predominantly consisting of L. chinensis, Stipa baicalensis Roshev., Cleistogenes squarrosa (Trin.) Keng, C. duriuscula, Pulsatilla turczaninovii Kryl. et Serg., and Cymbaria dahurica Linn.
The experimental field was used for hay harvesting until 2013 and has been fenced to prevent livestock from grazing since then. An elemental-S-addition field experiment was established in 2017 to simulate soil acidification caused by atmospheric S deposition in a homogeneous and flat field containing naturally assembled communities. The vegetation in the experimental plots is composed of the dominant species L. chinensis (relative abundance >40 %) and subordinate species (relative abundance between 1 % and 30 %), including S. baicalensis, C. duriuscula, C. squarrosa, C. dahurica, P. turczaninovii, Thermopsis lanceolata R.Br, and Achnatherum sibiricum (Linn.) Keng. The experimental setup adopted a randomized block design with eight levels of S-addition rates (0, 1, 2, 5, 10, 15, 20, and 50 g S m−2 yr−1) and five replicated 6 m×6 m plots (Fig. S2). The low-dose S applications in our study aimed to mimic the current atmospheric SO deposition level (2–4 g S m−2 yr−1) in northeast China (Yu et al., 2017). Adjacent plots were separated by a 2 m wide, mowed buffer strip. Purified sulfur fertilizer (elemental S >99 %), mixed with 200 g of soil collected from the untreated areas nearby, was applied by being spread by hand annually in late May, starting in 2017. Sulfur powder in soil can be oxidized by soil microorganisms to form H+ and SO, which simulate soil acidification well (Duddigan et al., 2021). In the present study, we collected plant and soil samples from all the plots from five out of eight levels of S-addition treatments (0, 5, 10, 20, and 50 g S m−2 yr−1).
2.2 Plant and soil sampling
In early August 2019, the annual aboveground biomass of the plant communities was harvested at peak growth. All living tissues were clipped within a randomly selected 1 m×1 m quadrat in each plot, sorted by species, and oven-dried at 65 °C for 48 h to obtain measurements of aboveground net primary productivity (ANPP) and leaf nutrient concentrations.
We measured three aboveground morphological traits for the two dominant species, L. chinensis and C. duriuscula. A total of 10 individual plants with complete shoots were randomly selected for each species within each plot. They were measured for their maximum height and then clipped at ground level. All the samples were immediately placed in a portable refrigerator and later detached to measure leaf area in the laboratory. To ensure the water saturation of the leaves, the samples were submerged in purified water and then rehydrated for a minimum period of 6 h. Then, the water-saturated leaves were carefully wiped with filter paper to remove the surface water and weighed. All leaf samples were scanned using an electronic scanner (Epson Perfection V39, Seiko Epson Corporation, Japan) to measure leaf area and then dried at 60 °C for 72 h to achieve a constant weight for measurements of dry mass. Specific leaf area (SLA; cm2 g−1) was calculated as the ratio of projected leaf area to dry mass. Leaf dry-matter content was calculated as the ratio of dry mass to saturated mass (LDMC; g g−1).
Plant roots were sampled using the soil block method in late August 2019. Specifically, a cm soil block was collected from each plot using a steel plate and a shovel, resulting in a total of 25 soil blocks. All soil blocks were immediately transported to the processing area upon collection and then gently loosened by hand to separate the roots from the soil. The separated plant roots were carefully washed to remove any adhering soil and placed in iceboxes for transportation to the laboratory. Before determining the root morphological and chemical traits, all root samples were frozen at −20 °C. Within each plot, at least 10 intact individual plants of both L. chinensis and C. duriuscula were used to determine the root nutrient traits (root [N], [P], [S], [Ca], [Fe], and [Mn]) and morphological traits. The total root length, surface area, and volume were determined using scanned images processed by WinRHIZO software (Regent Instruments Inc., Québec City, Quebec, Canada). Specific root length (SRL; m g−1) was calculated by dividing the total root length by its dry mass. Specific root surface area (SRA; cm2 g−1) was defined as the total surface area divided by its dry mass. Root tissue density (RTD, g cm−3) was obtained as the ratio of the dry root mass to its volume. All the above samples were dried at 65 °C to achieve a constant mass for measurements of root biomass at species and community levels. Root and leaf N concentrations were determined using an elemental analyzer, Vario EL III (Elementar, Hanau, Germany). Both the root and leaf samples were digested with 8 mL HNO3+4 mL HClO4 for measurements of P, S, Ca, Fe, and Mn concentrations using inductively coupled plasma optical emission spectrometry (5100 ICP-OES; PerkinElmer, Massachusetts, America).
Fresh soil sampling (0–10 cm depth) was performed using a soil auger (5 cm inner diameter). In each plot, three cores were collected and mixed into one composite sample. After removing the visible plant detritus and rocks, we sieved the fresh soils through a 2 mm screen and divided each soil sample into two portions. For each sample, 10 g of fresh soil was immediately extracted with 2 mol L−1 KCl solution. The extracted solution was analyzed for nitrate (NO) and ammonium (NH) concentrations using an AutoAnalyzer III continuous-flow analyzer (Bran+Luebbe, Norderstedt, Germany). The other subsamples were air-dried for measurements of physicochemical properties. Soil pH was determined in a 2.5 : 1 () water-to-soil ratio with a digital pH meter (Shanghai Precision Instrument Co., Ltd., Shanghai, China). Soil-available P concentration was measured using extractions in 0.5 mol L−1 NaHCO3 solution, and soil-available S concentration was measured using extractions in 0.1 mol L−1 Ca(H2PO4)2 solution with a UV–VIS spectrophotometer (UV-1700; Shimadzu, Japan) at 880 and 440 nm, respectively. Soil-exchangeable aluminum (Al3+) concentration was measured via titration with 0.25 M NaOH to a pH of 7.0 after extraction with 1 M KCl solution from air-dried soil samples. Soil-exchangeable calcium (Ca2+) was extracted using 1 M NH4OAc (pH =7.0) in a 1:10 ratio () for 30 min. Diethylenetriamine pentaacetic acid iron (DTPA-Fe) and DTPA manganese (DTPA-Mn) were extracted from 10 g of an air-dried soil sample with 20 mL 0.005 M diethylenetriamine pentaacetic acid (DTPA), 0.01 M CaCl2, and 0.1 M triethanolamine (TEA) at a pH of 7.3 and determined using an atomic absorption spectrophotometer (AAS; Shimadzu, Japan) (Feng et al., 2019; Li et al., 2021).
2.3 Statistical analyses
The effects of S addition on soil properties, plant traits, and biomass were analyzed using the one-way analysis of variance (ANOVA) with Duncan's test for comparing multiple means. Pearson's correlation analysis was performed to determine the relationships among plant traits, plant biomass, and abiotic soil variables across the S-addition levels. All the statistical analyses were performed using SPSS 16.0 (cc) with a significance level at p<0.05.
We used structural equation modeling (SEM) to determine the indirect effects of S addition in meditating aboveground and root biomass from the perspective of plant traits and soil factors. Prior to the SEM analysis, the number of variables was reduced by conducting principal component analysis (PCA) on all the variables for soil (pH, NH-N, NO-N, available P, available S, exchangeable cations (Ca2+ and Al3+), DTPA-Fe, DTPA-Mn), aboveground morphological traits (height, SLA, LDMC), leaf nutrient traits (Ca, Fe, Mn), root morphological traits (SRL, SRA, RTD), and root nutrient traits (N, P, S, Ca, Fe, Mn). We then used the first principal components (PC1s) for the subsequent SEM analysis to represent the soil acidification (PC1s explained 94.8 % of the variation), soil nutrients (PC1s explained 62.3 % of the variation), root nutrient traits of C. duriuscula (PC1s explained 45.7 % of the variation), and aboveground morphological traits of L. chinensis (PC1s explained 54.7 % of the variation) (Table S1 in the Supplement). A conceptual model of the hypothetical relationships was constructed (Fig. S1), assuming that S addition would directly impact soil physicochemical properties and indirectly influence aboveground and belowground biomass by altering soil pH, soil nutrient availability, and plant traits. The SEM analyses were performed using Amos 24.0 (Amos Development Co., Maine, USA), and the PCAs were performed using the “vegan” package in R 4.2.2. The Akaike information criteria (AIC) were used to evaluate the adequacy of the model.
Table 1Effects of S addition on abiotic soil variables. All numbers refer to the mean (the standard error). Lowercase letters indicate the significant difference among treatments (P<0.05).
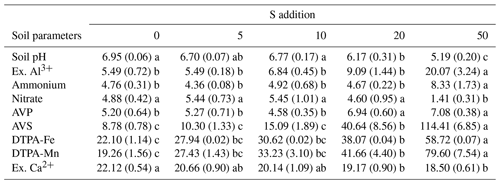
Please note the following. Ex. Al3+: exchangeable Al3+ (mg kg−1). Ammonium: soil NH-N concentration (mg kg−1). Nitrate: soil NO-N concentration (mg kg−1). AVP: soil-available phosphorus (mg kg−1). AVS: soil-available sulfur (mg kg−1). DTPA-Fe: soil DTPA-Fe concentration (mg kg−1). DTPA-Mn: soil DTPA-Mn concentration (mg kg−1). Ex. Ca: exchangeable Ca2+ (cmol kg−1).
3.1 Effects of S addition on soil properties
Sulfur addition significantly decreased soil pH from 6.95 to 5.19 but increased soil-exchangeable Al concentration only for the highest S-addition level of 50 g S m−2 yr−1 (Table 1). Similarly, S addition increased soil ammonium concentration but decreased soil nitrate concentration in the highest S-addition treatment (Table 1). Soil-available P, soil-available S, DTPA-Fe concentration, and DTPA-Mn concentrations increased as the S-addition rate increased, while soil-exchangeable Ca concentration decreased (Table 1).
3.2 Effects of S addition on above- and belowground biomass
In the third year of the treatment, S addition suppressed the aboveground biomass of the plant communities (Fig. 1). The aboveground biomass of the two dominant species showed contrasting responses to S addition; it increased for C. duriuscula but decreased for L. chinensis (Fig. 1). Moreover, S addition significantly increased the belowground biomass of the plant communities due to the increase in C. duriuscula but had no impact on the belowground biomass of L. chinensis (Fig. 1).
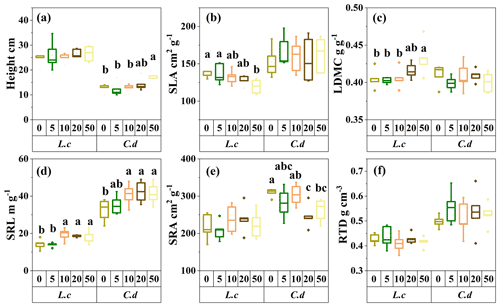
Figure 2The response of the morphological traits of the two dominant species to S addition in a meadow steppe. The abbreviations are defined as follows. SLA: specific leaf area. LDMC: leaf dry-matter content. SRL: specific root length. SRA: specific root area. RTD: root tissue density. L.c: L. chinensis. C.d: C. duriuscula. The different letters above the bars indicate the significant influence across the S-addition levels determined by the one-way ANOVA with P<0.05.
3.3 Effects of S addition on above- and belowground traits of L. chinensis and C. duriuscula
Sulfur addition resulted in increased plant height growth for C. duriuscula but had no impact on L. chinensis (Fig. 2a). The values of SLA decreased, and the values of LDMC increased in L. chinensis due to the S-addition treatment but were not affected with regard to C. duriuscula (Fig. 2b and c). For the belowground tissues, the S-addition treatment increased SRL in both species but decreased SRA only in C. duriuscula (Fig. 2d and e). However, RTD did not respond to the S-addition treatment in either of the two species (Fig. 2f).
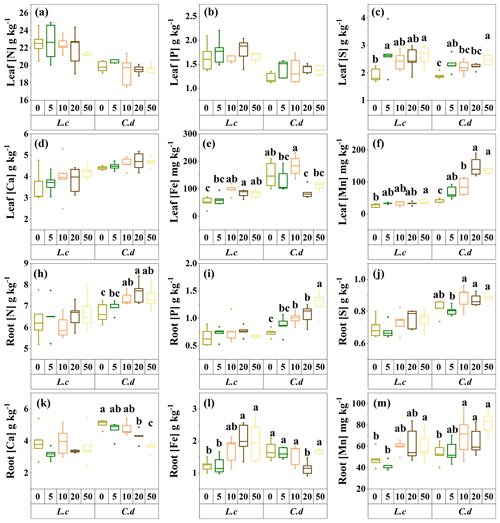
Figure 3The response of the chemical traits of the two dominant species to S addition in a meadow steppe. The abbreviations are defined as follows. Leaf [N]: leaf N concentration. Leaf [P]: leaf P concentration. Leaf [S]: leaf S concentration. Leaf [Ca]: leaf Ca concentration. Leaf [Fe]: leaf Fe concentration. Leaf [Mn]: leaf Mn concentration. Root [Ca]: root Ca concentration. Root [Fe]: root Fe concentration. Root [Mn]: root Mn concentration. Root [N]: root N concentration. Root [P]: root P concentration. Root [S]: root S concentration. L.c: L. chinensis. C.d: C. duriuscula. The different letters above the bars indicate the significant influence across the S-addition levels as determined by the one-way ANOVA with P<0.05.
In terms of the nutrient traits, S addition had no significant impact on leaf [N], [P], and [Ca], but it increased leaf [S] and [Mn] in both species. It also decreased leaf [Fe] in C. duriuscula and increased leaf [Fe] in L. chinensis (Fig. 3). Root [N], [P], and [S] increased in C. duriuscula in response to the S-addition treatment but were not affected in L. chinensis (Fig. 3h, i, and j). Sulfur addition decreased root [Ca] in C. duriuscula but did not affect root [Ca] in L. chinensis (Fig. 3k). Root [Fe] showed similar patterns of responses to S-addition treatments to those of leaf [Fe]: it decreased in C. duriuscula and increased in L. chinensis (Fig. 3l). Root [Mn] in both species was enhanced by S addition (Fig. 3m).
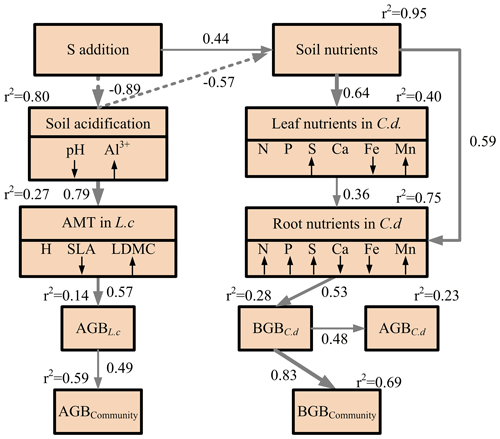
Figure 4Structural equation modeling of the impact of S addition on plant community biomass through the plausible pathways. Square boxes indicate the variables included in the analysis. These are as follows: S addition; soil nutrients, i.e., soil NH-N and NO-N concentrations, soil-available phosphorus, and soil-available sulfur; soil-exchangeable cations (Ca2+, Fe2+, and Mn2+); soil acidification, i.e., soil pH and exchangeable Al3+; aboveground morphological traits (AMTs), i.e., plant height (H), specific leaf area, and leaf dry-matter content, in L. chinensis; leaf nutrients, i.e., leaf N, P, S, Ca, Fe, and Mg concentrations, in C. duriuscula; root nutrients, i.e., root N, P, S, Ca, Fe, and Mg concentrations, in C. duriuscula; aboveground biomass of C. duriuscula; belowground biomass of C. duriuscula (BGBC.d); aboveground biomass of L. chinensis (AGBL.c); aboveground community biomass (AGB); and belowground community biomass (BGB). The symbols ↓ and ↑ indicate a significant decrease or increase, respectively, with increasing S addition. The final SEM adequately fits the data: χ2=51.83, df = 40, P=0.10, AIC = 103.83, and n=25. The r2 values next to the response variables indicate the proportion of variation explained by relationships with other variables. The solid and dashed arrows represent significant positive and negative pathways (P<0.05), respectively. Nonsignificant pathways (P<0.05) are not shown. The values by the arrows indicate the standardized path coefficient, which is equivalent to the correlation coefficient.
3.4 Correlations and pathways of S-induced soil acidification effects on plant traits and biomass
A correlation analysis revealed species-specific responses to S addition in the aboveground morphological traits and the root nutrient traits (Figs. S3 and S4). This was mainly due to an increase in soil acidity and the occurrence of Al3+ toxicity and nutrient imbalances (i.e., the deficient or excessive level of certain nutrients in the soil) induced by S addition, as demonstrated by the well-fitted SEM (χ2=51.83, P=0.10, degrees of freedom (df) =40, AIC = 103.83, n=25) (Fig. 4). The indirect positive effect of S addition on belowground community biomass was mainly imposed through decreased soil pH in combination with an imbalance of soil-available nutrients, changes in the leaf and root nutrient traits, and the increased belowground biomass of C. duriuscula, collectively accounting for 69 % of the variation in belowground community biomass (Fig. 4). The indirect negative effect of S addition on aboveground community biomass was mainly mediated through soil acidification, changes in the aboveground morphological traits, and the increased aboveground biomass of L. chinensis, collectively accounting for 59 % of the variation in aboveground community biomass (Fig. 4).
4.1 Species-specific trait responses to S addition
The patterns of trait responses to S addition differed between L. chinensis and C. duriuscula. Specifically, the nutrient traits of L. chinensis were less plastic, as evidenced by the unchanged concentrations of N, P, S, and Ca, compared to those of C. duriuscula. L. chinensis has been suggested to be a highly homoeostatic species that exhibits greater stability in elemental composition in a temperate steppe (Yu et al., 2010). Higher macroelement homeostasis helps plants maintain functional and growth stability to resist changes in soil environment (Yu et al., 2010; Feng et al., 2019).
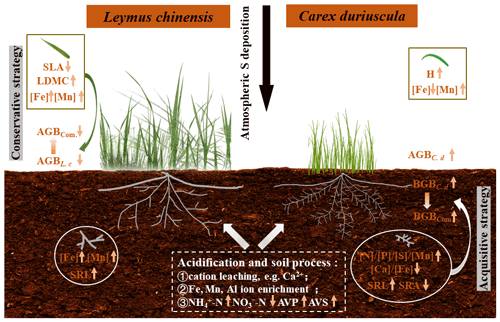
Figure 5A schematic diagram illustrating the ecological effects of S-induced soil acidification on above- and belowground biomass and the traits of the two dominant species in a meadow steppe. The symbol ↑ represents an increase in response to S addition; the symbol ↓ represents a decrease in response to S addition. Com. denotes community, AVP denotes soil-available phosphorus, and AVS denotes soil-available sulfur.
It is intriguing that increases in both leaf and root [Fe] in L. chinensis caused by S addition were not associated with soil-available [Fe] (Figs. 3 and S3). Iron uptake and assimilation have been shown to be dependent on sulfate availability (Zuchi et al., 2012). Previous research has demonstrated close relationships between Fe and S nutrition, suggesting common regulatory mechanisms for the homeostasis of the two elements (Forieri et al., 2013). For grasses, S addition could enhance the assimilation of plant S, which is subsequently incorporated into methionine in order to accelerate the secretion of phytosiderophore (Zuchi et al., 2012; Courbet et al., 2019). However, Fe absorption in C. duriuscula was inhibited by soil acidification, which is consistent with Fe(III)-reduction-based mechanisms (Tian et al., 2016). Namely, the acquisition of Fe by non-graminaceous monocotyledonous species is mediated by the reduction of Fe3+ to Fe2+, catalyzed by the ferric-chelate reductase in root cells; Fe2+ absorption can be further interfered with through competition with Mn2+ for the same metal transporter (Curie and Briat, 2003; Pittman, 2005). A higher acidification-induced DTPA-Mn concentration in the calcareous soil contributed to Mn accumulation in plant tissues of the two species (Figs. 3 and 5). Sulfur addition increased tissue [Mn] to a greater extent in C. duriuscula than in L. chinensis.
L. chinensis responded to soil acidification stress by decreasing SLA and increasing LDMC to reduce the loss of water and nutrients, adopting a conservative resource uptake strategy. The SLA and LDMC in L. chinensis were significantly correlated with soil-exchangeable Al, suggesting that conservative traits might also be linked to an Al-resistant strategy (Poozesh et al., 2007). We found that as soil pH decreased, soil nitrate reduced and was positively correlated with SLA but negatively with LDMC in L. chinensis (Table 1 and Fig. S3). Soil nitrification has been shown to be positively related to leaf traits, such as leaf [N] and SLA (Laughlin, 2011). This suggests that a decrease in soil nitrate under soil acidification could be an important driver of plant trait variations. With regards to L. chinensis, the belowground traits were unsusceptible to S addition compared to those of C. duriuscula. One plausible explanation for this insensitivity is that deep-rooted plants are more resilient to changing soil environments than shallow-rooted plants (such as the sedge C. duriuscula) (Zhang et al., 2019). We found that both species responded to S-induced soil acidification by enhancing SRL, in which is agreement with findings from Göransson et al. (2011) that grass species increase root length to avoid acid stress. These results indicate that variation in root morphological traits has the potential to mitigate the negative effects of soil acidity and should be considered part of stress-avoidance or tolerance strategies (Thomaes et al., 2013).
4.2 Species-specific and community biomass responses to S addition
To clarify the underlying mechanisms for the regulation of plant biomass through functional traits, we explored the role of morphological and nutrient traits in mediating aboveground and belowground biomass changes in response to S addition. We found that both the aboveground and root traits of L. chinensis and C. duriuscula exhibited contrasting adaptive strategies for acquiring aboveground and belowground resources (Figs. 4 and 5). SEM illustrated that the decrease in the aboveground biomass of L. chinensis was related to increased soil acidification and the conservative responses of aboveground morphological traits in response to S addition (Figs. 4 and 5). L. chinensis has been found to be a nitrophilic and resource-acquisitive species in N-rich environments (Feng et al., 2019; Yang et al., 2019) but is disadvantaged under S-induced soil acidification. For example, we found SLA and LDMC in L. chinensis were positively correlated with the aboveground biomass of both L. chinensis and the plant communities (Fig. S3). Soil acidification has been shown to enhance the toxic effects of proton and exchangeable Al (Roem and Berndse, 2000). In view of the environmental stress hypothesis, plant species could adopt different strategies in terms of trait responses to mitigate such environmental stress (Encinas-Valero et al., 2022). SLA and LDMC are often regarded as prominent indicators of a plant's strategy for maintaining productivity in response to environmental stress and disturbance regimes. Stress-tolerant species normally exhibit lower growth rates, photosynthetic rates, and SLA but have a higher LDMC (Pérez-Harguindeguy et al., 2013). Sulfur addition induces acidic stress in plants, leading to reduced SLA as well as lower photosynthetic capacity and decreased aboveground plant productivity. Damage to photosynthetic capacity associated with oxidative stress has been found to occur in tree species as a result of acid rain (Chen et al., 2013b). However, it is still less understood how plants in grassland ecosystems respond physiologically and biochemically to soil acidification. Future research needs to test the photosynthetic and antioxidant responses of plants to soil acidification.
We found that the aboveground biomass of the plant communities exhibited a declining trend from 22 % to 11 % under soil acidification, although the overall effect was rather weak between pH 6.7 and 5.19 (Fig. 1 and Table 1). In this study, L. chinensis was found to play a dominant role in contributing to aboveground productivity, which is consistent with the findings from Tibbett et al. (2019) that grasses make up about 60 % of plant coverage in acid grassland and heathland. Therefore, the decreased aboveground biomass of L. chinensis was one of the reasons for the decline in aboveground community productivity (Fig. 4). Another explanation for the decline in aboveground biomass may concern the competitive exclusion of bunchgrasses and forbs under soil acidification (Stevens et al., 2010; Chen et al., 2015). Together, findings from this study help advance our understanding of the importance of leaf morphological traits in regulating grassland productivity in response to soil acidification.
In contrast to the role of L. chinensis, C. duriuscula was found to dominate the influence on the belowground biomass of the plant communities under soil acidification; the belowground biomass of both C. duriuscula and the plant communities significantly increased from 19 % to 52 % by means of S addition (Fig. 1). The sedge species (i.e., C. duriuscula) appeared to be more tolerant to S-induced soil acidification than the perennial rhizome grass species (i.e., L. chinensis). This finding is partly supported by results for alpine grasslands and typical steppe ecosystems (Chen et al., 2015; Wang et al., 2020). Previous studies have suggested that sedges have a greater competitive advantage in nutrient-poor environments than other functional groups (Gusewell, 2004). An increase in root biomass under soil acidification suggested that sedges invest more resources in favor of nutrient acquisition. SEM provided further evidence that for C. duriuscula, a higher nutrient demand (such as that concerning root [N], [P], [S], and [Mn]) was matched by a greater root biomass in response to the S-addition treatment (Fig. 4). An increase in the root biomass of C. duriuscula contributed to an increase in the belowground biomass of the plant communities as a result of shifts in soil-available nutrients in response to S addition. Our study provides direct evidence that C. duriuscula is highly efficient in terms of nutrient acquisition and thereby responds to soil acidification stress with increased biomass production (Figs. 4 and 5). Our short-term findings suggest that sedges play an important role in preventing the decline in grassland productivity in acidified soils. Consistent with results from a long-term acidification experiment (Tibbett et al., 2019), compensatory growth of acid-tolerant species is probably key to maintaining grassland productivity over the longer term, particularly in ecosystems that experience acidification by means of chronic N and S deposition.
For grassland ecosystems, most of the carbon is allocated belowground (Bontti et al., 2009). The accumulation of roots may be beneficial in the competition for nutrient and water resources in the short term (Wang et al., 2020). In the longer term, however, the asymmetric competitive advantage of larger individuals (L. chinensis) in accessing light rather than the competition for soil resources (DeMalach and Kadmon, 2017) is likely to have a decisive effect on the productivity and diversity of grassland plant communities under soil acidification. Findings in this study contrast previous findings from Wang et al. (2020), who reported that the diameter of third-order roots contributed to increased belowground community biomass under soil acidification in an alpine grassland. Our study clearly demonstrates that leaf and root nutrients jointly mediate belowground community biomass in response to soil acidification induced by S addition.
Our results highlighted that aboveground and root traits play important roles in mediating competition between grassland plants for environmental resources in the presence of soil acidification. Sulfur addition resulted in soil acidification and led to nutrient imbalances (i.e., higher ammonium, available P, Fe, Mn, and exchangeable Al3+ but lower nitrate and exchangeable Ca2+). The dominant species, L. chinensis, showed a conservative trait response strategy, with decreased SLA and increased LDMC as a result of S addition. Moreover, the conservative traits were associated with a stable root biomass but a smaller aboveground biomass as a direct impact of soil acidification. In contrast, C. duriuscula displayed an efficient nutrient acquisition strategy, with increased plant height and more efficient root traits ([N], [P], [S], [Mn], SRL) favoring aboveground and root productivity in response to S addition, as mediated by altered soil acidity and nutrient availability. Such divergent and species-specific responses were strongly driven by the environmental conditions of the soil, which resulted in inconsistent changes in aboveground and belowground biomass in grassland communities in response to S addition. Our results highlight the important roles of both aboveground and root traits in regulating species and community biomass under soil acidification.
Data will be made available on request from the corresponding author.
The supplement related to this article is available online at: https://doi.org/10.5194/bg-21-2641-2024-supplement.
YJ conceived the study and designed the experiment. TL and HeL conducted the experimental work and the data analysis. XF wrote the paper with substantial editing by RW, JC, HuL, and YJ.
The contact author has declared that none of the authors has any competing interests.
Publisher's note: Copernicus Publications remains neutral with regard to jurisdictional claims made in the text, published maps, institutional affiliations, or any other geographical representation in this paper. While Copernicus Publications makes every effort to include appropriate place names, the final responsibility lies with the authors.
We would like to acknowledge the support from the Youth Innovation Promotion Association of the Chinese Academy of Sciences. We are grateful to Osbert J. Sun for helping to improve the English writing in an earlier draft of the paper.
This research has been supported by the National Natural Science Foundation of China (grant nos. 32271677, 32071563, 32222056, and 32271655), the Strategic Priority Research Program of the Chinese Academy of Sciences (grant no. XDA23080400), and the Doctoral Science Foundation of Liaoning Province (grant no. 2021-BS-015).
This paper was edited by Kees Jan van Groenigen and reviewed by two anonymous referees.
Bolan, N. S., Adriano, D. C., and Curtin, D.: Soil acidification and liming interactions with nutrient and heavy metal transformation and bioavailability, Adv. Agron., 78, 5–272, https://doi.org/10.1016/S0065-2113(02)78006-1, 2003.
Bontti, E. E., Decant, J. P., Munson, S. M., Gathany, M. A., Przeszlowska, A., Haddix, M. L., Owens, S., Burke, I. C., Parton, W. J., and Harmon, M. E.: Litter decomposition in grasslands of central North America (US Great Plains), Glob. Chang Biol., 15, 1356–1363, https://doi.org/10.1111/j.1365-2486.2008.01815.x, 2009.
Chen, D., Lan, Z., Bai, X., Grace, J. B., and Bai, Y.: Evidence that acidification-induced declines in plant diversity and productivity are mediated by changes in below-ground communities and soil properties in a semi-arid steppe, J. Ecol., 101, 1322–1334, https://doi.org/10.1111/1365-2745.12119, 2013a.
Chen, D., Wang, Y., Lan, Z., Li, J., Xing, W., Hu, S., and Bai, Y.: Biotic community shifts explain the contrasting responses of microbial and root respiration to experimental soil acidification, Soil Biol. Biochem., 90, 139–147, https://doi.org/10.1016/j.soilbio.2015.08.009, 2015.
Chen, J., Wang, W. H., Liu, T., Wu, F., and Zheng, H.: Photosynthetic and antioxidant responses of Liquidambar formosana and Schima superba seedlings to sulfuric-rich and nitric-rich simulated acid rain, Plant Physiol. Bioch., 64, 41–51, https://doi.org/10.1016/j.plaphy.2012.12.012, 2013b.
Clark, C. M., Simkin, S. M., Allen, E. B., Bowman, W. D., Belnap, J., Brooks, M. L., Collins, S. L., Geiser, L. H., Gilliam, F, S., Jovan, F. S., Pardo, L. H., Schulz, B. K., Stevens, C. J., Suding, K. N., Throop, H. L., and Waller, D. M.: Potential vulnerability of 348 herbaceous species to atmospheric deposition of nitrogen and sulfur in the United States, Nat. Plants, 5, 697–705, https://doi.org/10.1038/s41477-019-0442-8, 2019.
Cliquet, J. B. and Lemauviel-Lavenant, S.: Grassland species are more efficient in acquisition of S from the atmosphere when pedospheric S availability decreases, Plant Soil, 435, 69–80, https://doi.org/10.1007/s11104-018-3872-6, 2019.
Courbet, G., Gallardo, K., Vigani, G., Brunel-Muguet, S., Trouverie, J., Salon, C., and Ourry, A.: Disentangling the complexity and diversity of crosstalk between sulfur and other mineral nutrients in cultivated plants, J. Exp. Bot., 70, 4183–4196, https://doi.org/10.1093/jxb/erz214, 2019.
Curie, C. and Briat, J. F.: Iron transport and signaling in plants, Annu. Rev. Plant Biol., 54, 183–206, https://doi.org/10.1146/annurev.arplant.54.031902.135018, 2003.
De Battisti, D., Fowler, M. S., Jenkins, S. R., Skov, M. W., Bouma, T. J., Neyland, P. J., and Griffin, J. N.: Multiple trait dimensions mediate stress gradient effects on plant biomass allocation, with implications for coastal ecosystem services, J. Ecol., 108, 1227–1240, https://doi.org/10.1111/1365-2745.13393, 2020.
Delpiano, C. A., Prieto, I., Loayza, A. P., Carvajal, D. E., and Squeo, F. A.: Different responses of leaf and root traits to changes in soil nutrient availability do not converge into a community-level plant economics spectrum, Plant Soil, 450, 463–478, https://doi.org/10.1007/s11104-020-04515-2, 2020.
DeMalach, N. and Kadmon, R.: Light competition explains diversity decline better than niche dimensionality, Funct. Ecol., 31, 1834–1838, https://doi.org/10.1111/1365-2435.12841, 2017.
Duan, L., Yu, Q., Zhang, Q., Wang, Z., Pan, Y., Larssen, T., Tang, J., and Mulder, J.: Acid deposition in Asia: Emissions, deposition, and ecosystem effects, Atmos. Environ., 146, 55–69, https://doi.org/10.1016/j.atmosenv.2016.07.018, 2016.
Duddigan, S., Fraser, T., Green, I., Diaz, A., Sizmur, T., and Tibbett, M.: Plant, soil and faunal responses to a contrived pH gradient, Plant Soil, 462, 505–524, https://doi.org/10.1007/s11104-021-04879-z, 2021.
Encinas-Valero, M., Esteban, R., Hereş, A. M., Vivas, M., Fakhet, D., Aranjuelo, I., Solla, A., Moreno, G., and Curiel Yuste, J.: Holm oak decline is determined by shifts in fine root phenotypic plasticity in response to belowground stress, New Phytol., 235, 2237–2251, https://doi.org/10.1111/nph.18182, 2022.
Feng, X., Wang, R., Yu, Q., Cao, Y., Zhang, Y., Yang, L., Dijkstra, F. A., and Jiang, Y.: Decoupling of plant and soil metal nutrients as affected by nitrogen addition in a meadow steppe, Plant Soil, 443, 337–351, https://doi.org/10.1007/s11104-019-04217-4, 2019.
Forieri, I., Wirtz, M., and Hell, R.: Toward new perspectives on the interaction of iron and sulfur metabolism in plants, Front. Plant Sci., 4, 357, https://doi.org/10.3389/fpls.2013.00357, 2013.
Garrison, M. T., Moore, J. A., Shaw, T. M., and Mika, P. G.: Foliar nutrient and tree growth response of mixed-conifer stands to three fertilization treatments in northeast Oregon and north central Washington, Forest Ecol. Manag., 132, 183–198, https://doi.org/10.1016/S0378-1127(99)00228-5, 2000.
Geng, Y., Wang, L., Jin, D., Liu, H., and He, J.: Alpine climate alters the relationships between leaf and root morphological traits but not chemical traits, Oecologia, 175, 445–455, https://doi.org/10.1007/s00442-014-2919-5, 2014.
Göransson, P., Falkengren-Grerup, U., and Andersson, S.: Deschampsia cespitosa and soil acidification: general and trait-specific responses to acid and aluminium stress in a solution experiment, Nord. J. Bot., 29, 97–104, https://doi.org/10.1111 /j.1756-1051.2010.00793.x, 2011.
Grassein, F., Lemauviel- Lavenant, S., Lavorel, S., Bahn, M., Bardgett, R. D., Desclos-Theveniau, M., and Laîné, P.: Relationships between functional traits and inorganic nitrogen acquisition among eight contrasting European grass species, Ann. Bot., 115, 107–115, https://doi.org/10.1093/aob/mcu233, 2015.
Gusewell, S.: N: P ratios in terrestrial plants: Variation and functional significance, New Phytol., 164, 243–266, https://doi.org/10.1111/j.1469-8137.2004.01192.x, 2004.
Haling, R. E., Richardson, A. E., Culvenor, R. A., Lambers, H., and Simpson, R. J.: Root morphology, root-hair development and rhizosheath formation on perennial grass seedlings is influenced by soil acidity, Plant Soil, 335, 457–468, doi.org/10.1007/s11104-010-0433-z, 2010.
Hammond, J.P., Broadley, M. R., and White, P. J.: Genetic responses to phosphorus deficiency, Ann. Bot., 94, 323–332, https://doi.org/10.1093/aob/mch156, 2004.
Hao, M., Messier, C., Geng, Y., Zhang, C., Zhao, X., and von Gadow, K.: Functional traits influence biomass and productivity through multiple mechanisms in a temperate secondary forest, Eur. J. For. Res., 139, 959–968, https://doi.org/10.1007/s10342-020-01298-0, 2020.
IUSS Working Group WRB: World Reference Base for Soil Resources 2014, International Soil Classification System for Naming Soils and Creating Legends for Soil Maps, World Soil Resources Reports No. 106, FAO, Rome, https://www.fao.org/soils-portal/soil-survey/soil-classification/world-reference-base/en/ (last access: 28 May 2024), 2014.
Jaggi, R. C., Aulakh, M. S., and Sharma, R.: Impacts of elemental S applied under various temperature and moisture regimes on pH and available P in acidic, neutral and alkaline soils, Biol. Fert. Soils, 41, 52–58, https://doi.org/10.1007/s00374-004-0792-9, 2005.
Jung, K., Kwak, J. H, Gilliam, F. S., and Chang, S. X.: Simulated N and S deposition affected soil chemistry and understory plant communities in a boreal forest in western Canada, J. Plant Ecol., 11, 511–523, https://doi.org/10.1093/jpe/rtx030, 2018.
Kandlikar, G. S., Kleinhesselink, A. R., and Kraft, N. J.: Functional traits predict species responses to environmental variation in a California grassland annual plant community, J. Ecol., 110, 833–844, https://doi.org/10.1111/1365-2745.13845, 2022.
Kemmitt, S. J., Wright, D., and Jones, D. L.: Soil acidification used as a management strategy to reduce nitrate losses from agricultural land, Soil Biol. Biochem., 37, 867–875, https://doi.org/10.1016/j.soilbio.2004.10.001, 2005.
Laughlin, D. C.: Nitrification is linked to dominant leaf traits rather than functional diversity, J. Ecol., 99, 1091–1099, https://doi.org/10.1111/j.1365-2745.2011.01856.x, 2011.
Leifeld, J., Bassin, S., Conen, F., Hajdas, I., Egli, M., and Fuhrer, J.: Control of soil pH on turnover of belowground organic matter in subalpine grassland, Biogeochemistry, 112, 59–69, https://doi.org/10.1007/s10533-011-9689-5, 2013.
Li, T., Wang, R., Cai, J., Meng, Y., Wang, Z., Feng, X., Liu, H., Turco, R. F., and Jiang, Y.: Enhanced carbon acquisition and use efficiency alleviate microbial carbon relative to nitrogen limitation under soil acidification, Ecol. Process., 10, 1–13, https://doi.org/10.1186/s13717-021-00309-1, 2021.
Li, Y., Sun, J., Tian, D., Wang, J., Ha, D., Qu, Y., Jing, G., and Niu, S.: Soil acid cations induced reduction in soil respiration under nitrogen enrichment and soil acidification, Sci. Total Environ., 615, 1535–1546, https://doi.org/10.1016/j.scitotenv.2017.09.131, 2018.
Meng, C., Tian, D., Zeng, H., Li, Z., Yi, C., and Niu, S.: Global soil acidification impacts on belowground processes, Environ. Res. Lett., 14, 074003, doi.org/10.1088/1748-9326/ab239c, 2019.
Mitchell, R. J., Hewison, R. L., Fielding, D. A., Fisher, J. M., Gilbert, D. J., Hurskainen, S., Pakeman, R.J., Potts, J. M., and Riach, D.: Decline in atmospheric sulphur deposition and changes in climate are the major drivers of long-term change in grassland plant communities in Scotland, Environ. Pollut., 235, 956–964, doi.org/10.1016/j.envpol.2017.12.086, 2018.
Mueller, K. E., Eissenstat, D. M., Hobbie, S. E., Oleksyn, J., Jagodzinski, A. M., Reich, P. B., Chadwick, O. A., and Chorover, J.: Tree species effects on coupled cycles of carbon, nitrogen, and acidity in mineral soils at a common garden experiment, Biogeochemistry, 111, 601–614, https://doi.org/10.1007/s10533-011-9695-7, 2012.
Pérez-Harguindeguy, N., Diaz, S., Garnier, E., Lavorel, S., Poorter, H., Jaureguiberry, P., Bret-Harte, M. S, Cornwell, W. K., Craine, J. M., Gurvich, D. E., Urcelay, C., Veneklaas, E. J., Reich, P. B., Poorter, L., Wright, I. J., Ray, P., Enrico, L., Pausas, J. G., de Vos, A. C., Buchmann, N., Funes, G., Quetier, F., Hodgson, J. G., Thompson, K., Morgan, H. D., ter Steege, H., van der Heijden, M. G. A., Sack, L., Blonder, B., Poschlod, P., Vaieretti, M. V., Conti, G., Staver, A. C., Aquino, S., and Cornelissen, J. H. C.: New handbook for standardised measurement of plant functional traits worldwide, Aust. Bot., 61, 167–234, https://doi.org/10.1071/BT12225, 2013.
Pittman, J. K.: Managing the manganese: molecular mechanisms of manganese transport and homeostasis, New Phytol., 167, 733–742, https://doi.org/10.1111/j.1469-8137.2005.01453.x, 2005.
Poozesh, V., Cruz, P., Choler, P., and Bertoni, G., Relationship between the Al resistance of grasses and their adaptation to an infertile habitat, Ann. Bot., 99, 947–954, https://doi.org/10.1093/aob/mcm046, 2007.
Rabêlo, F. H. S., Lux, A., Rossi, M. L., Martinelli, A. P., Cuypers, A., and Lavres, J.: Adequate S supply reduces the damage of high Cd exposure in roots and increases N, S and Mn uptake by Massai grass grown in hydroponics, Environ. Exp. Bot., 148, 35–46, https://doi.org/10.1016/j.envexpbot.2018.01.005, 2018.
Reich, P. B.: The world-wide “fast-slow” plant economics spectrum: a traits manifesto, J. Ecol., 102, 275–301, https://doi.org/10.1111/1365-2745.12211, 2014.
Roem, W. J. and Berendse, F.: Soil acidity and nutrient supply ratio as possible factors determining changes in plant species diversity in grassland and heathland communities, Biol. Conserv., 92, 151–161, https://doi.org/10.1016/S0006-3207(99)00049-X, 2000.
Stevens, C. J., Thompson, K., Grime, J. P., Long, C. J., and Gowing, D. J.: Contribution of acidification and eutrophication to declines in species richness of calcifuge grasslands along a gradient of atmospheric nitrogen deposition, Funct. Ecol., 24, 478–484, https://doi.org/10.1111/j.1365-2435.2009.01663.x, 2010.
Thomaes, A., De Keersmaeker, L., De Schrijver, A., Baeten, L., Vandekerkhove, K., Verstraeten, G., and Verheye, K.: Can soil acidity and light help to explain tree species effects on forest herb layer performance in post-agricultural forests?, Plant Soil, 373, 183–199, https://doi.org/10.1007/s11104-013-1786-x, 2013.
Tian, D. and Niu, S.: A global analysis of soil acidification caused by nitrogen addition, Environ. Res. Lett., 10, 024019, https://doi.org/10.1088/1748-9326/10/2/024019, 2015.
Tian, Q., Liu, N., Bai, W., Li, L., Chen. J., Reich, P. B., Yu Q., Guo, D., Smith, M. D., Knapp, A. K., Cheng, W., Lu, P., Gao, Y., Yang, A., Wang, T., Li, X, Wang, Z., Ma, Y., and Zhang, W.: A novel soil manganese mechanism drives plant species loss with increased nitrogen deposition in a temperate steppe, Ecology, 97, 65–74, https://doi.org/10.1890/15-0917.1, 2016.
Tian, Q., Lu, P., Ma, P., Zhou, H., Yang, M., Zhai, X. Chen M., Wang, H., Li W., Bai, W., Lambers, H., and Zhang, W.: Processes at the soil-root interface determine the different responses of nutrient limitation and metal toxicity in forbs and grasses to nitrogen enrichment, J. Ecol., 109, 927–938, https://doi.org/10.1111/1365-2745.13519, 2021.
Tian, Q., Lu, P., Zhai, X., Zhang, R., Zheng, Y., Wang, H., Nie, B., Bai, W., Niu, S., Shi, P., Yang, Y., Yang, D., Stevens, C., Lambers, H., and Zhang, W.: An integrated belowground trait-based understanding of nitrogen-driven plant diversity loss, Glob. Change Biol., 28, 3651–3664, https://doi.org/10.1111/gcb.16147, 2022.
Tibbett, M., Gil-Martínez, M., Fraser, T., Green, I. D., Duddigan, S., De Oliveira, V. H., Raulund-Rasmussen, K., Sizmur, T. and Diaz, A.: Long-term acidification of pH neutral grasslands affects soil biodiversity, fertility and function in a heathland restoration, Catena, 180, 401–415, https://doi.org/10.1016/j.catena.2019.03.013, 2019.
van Dobben, H. and de Vries, W.: Relation between forest vegetation, atmospheric deposition and site conditions at regional and European scales, Environ. Pollut., 158, 921–933, https://doi.org/10.1016/j.envpol.2009.09.015, 2010.
Violle, C., Navas, M. L., Vile, D., Kazakou, E., Fortunel, C., Hummel, I., and Garnier, E.: Let the concept of trait be functional!, Oikos, 116, 882–892, https://doi.org/10.1111/j.0030-1299.2007.15559.x, 2007.
Vitousek, P. M. and Howarth, R. W.: Nitrogen limitation on land and in the sea-how can it occur?, Biogeochemistry, 13, 87–115, https://doi.org/10.1007/BF00002772, 1991.
Wang, P., Guo, J., Xu, X., Yan, X., Zhang, K., Qiu, Y., Zhao, Q., Huang, K., Luo, X., Yang, F., Guo, H., and Hu, S.: Soil acidification alters root morphology, increases root biomass but reduces root decomposition in an alpine grassland, Environ. Pollut., 265, 115016, https://doi.org/10.1016/j.envpol.2020.115016, 2020.
Yang, F., Zhang, Z., Barberán, A., Yang, Y., Hu, S., and Guo, H.: Nitrogen-induced acidification plays a vital role driving ecosystem functions: Insights from a 6-year nitrogen enrichment experiment in a Tibetan alpine meadow, Soil Biol. Biochem., 153, 108107, https://doi.org/10.1016/j.soilbio.2020.108107, 2021.
Yang, G., Lü, X., Stevens, C. J., Zhang, G., Wang, H., Wang, Z., Zhang, Z., Liu, Z., and Han, X.: Mowing mitigates the negative impacts of N addition on plant species diversity, Oecologia, 189, 769–779, https://doi.org/10.1007/s00442-019-04353-9, 2019.
Yang, Y., Ji, C., Ma, W., Wang, S., Wang, S., Han, W., Mohammat, A., Robinson, D., and Smith, P.: Significant soil acidification across northern China's grasslands during 1980s–2000s, Glob. Change Biol., 18, 2292–2300, https://doi.org/10.1111/j.1365-2486.2012.02694.x, 2012.
Yu, H., He, N., Wang, Q., Zhu, J., Gao, Y., Zhang, Y., Jia, Y., and Yu, G.: Development of atmospheric acid deposition in China from the 1990s to the 2010s, Environ. Pollut., 231, 182–190, https://doi.org/10.1016/j.envpol.2017.08.014, 2017.
Yu, Q., Chen, Q., Elser, J. J., He, N., Wu, H., Zhang, G., Wu, J., Bai, Y., and Han, X.: Linking stoichiometric homoeostasis with ecosystem structure, functioning and stability, Ecol. Lett., 13, 1390–1399, https://doi.org/10.1111/j.1461-0248.2010.01532.x, 2010.
Zhang, B., Cadotte, M. W., Chen, S., Tan, X., You, C., Ren, T., Chen, M., Wang, S., Li, W., Chu, C., Jiang, L., Bai, Y., Huang, J., and Han, X.: Plants alter their vertical root distribution rather than biomass allocation in response to changing precipitation, Ecology, 100, e02828, https://doi.org/10.1002/ecy.2828, 2019.
Zhang, D., Peng, Y., Li, F., Yang, G., Wang, J., Yu, J., Zhou, G., and Yang, Y.: Above- and belowground resource acquisition strategies determine plant species responses to nitrogen enrichment, Ann. Bot., 128, 31–44, https://doi.org/10.1093/aob/mcab032, 2021.
Zhang, Q., Zhu, J., Wang, Q., Xu, L., Li, M., Dai, G., Mulder J., Xi Y., and He, N.: Soil acidification in China's forests due to atmospheric acid deposition from 1980 to 2050, Sci. Bull., 67, 914–917, https://doi.org/10.1016/j.scib.2022.01.004, 2022.
Zuchi, S., Cesco S., and Astolfi S.: High S supply improves Fe accumulation in durum wheat plants grown under Fe limitation, Environ. Exp. Bot., 77, 25–32, https://doi.org/10.1016/j.envexpbot.2011.11.001, 2012.