the Creative Commons Attribution 4.0 License.
the Creative Commons Attribution 4.0 License.
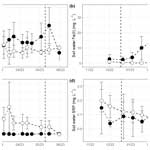
The role of nitrogen and iron biogeochemical cycles in the production and export of dissolved organic matter in agricultural headwater catchments
Rémi Dupas
Patrick Durand
To better understand the seasonal variations in environmental conditions regulating dissolved organic matter (DOM) export in agricultural headwater catchments, we combined the monitoring of nitrate, iron, soluble phosphorus, and DOM concentration (as dissolved organic carbon; DOC) and composition (3D fluorescence) in soil and stream waters at regular intervals during 1 hydrological year. We installed 17 zero-tension lysimeters in organic-rich top soil horizons (15 cm below the surface) in the riparian area of a well-monitored agricultural catchment in French Brittany and collected them at a fortnightly frequency from October 2022 to June 2023. We observed a large increase in DOC concentrations in soil waters during the high-flow period linked to the establishment of Fe-reducing conditions and the subsequent release of DOM. We also noted that the timing and the spatial variability in Fe(II) biodissolution in soils was regulated by nitrate from agricultural origin and the heterogeneity of water flow paths at the hillslope scale. Contrary to our current understanding of DOM export in headwater catchments, these results lead us to consider the winter high-flow period as an active phase of both DOM production and export.
- Article
(1722 KB) - Full-text XML
-
Supplement
(1054 KB) - BibTeX
- EndNote
Dissolved organic matter (DOM) is a key component of the ecological and biogeochemical functioning of aquatic ecosystems (Hanson et al., 2015), affecting for instance light penetration (Kelly et al., 2001), pollutant transport (Aiken et al., 2011), aquatic microbial metabolism (Wetzel, 1992), and the treatment of drinking water (Chow et al., 2005). Aquatic DOM, which is mainly of terrestrial origin, represents a fundamental link between the terrestrial, oceanic, and atmospheric compartments of the global carbon cycle (Dean et al., 2020; Battin et al., 2008). Unravelling the sources and drivers of DOM export has become an urgent environmental issue in a context of long-term increasing concentrations of dissolved organic carbon (DOC; a proxy for DOM content) reported in numerous streams in the Northern Hemisphere (Monteith et al., 2007; De Wit et al., 2021).
Numerous studies carried out in temperate and boreal regions have shown that headwater catchments are the main entry point of DOM into fluvial networks (Ågren et al., 2007; Creed et al., 2015) and identified riparian areas as the dominant sources of DOM at the catchment scale owing to their location at the terrestrial–aquatic interface (Sanderman et al., 2009; Lambert et al., 2014; Laudon et al., 2012; Winterdahl et al., 2014). Subsurface flow through shallow organic-rich soil layers during storm events (at the daily scale) is responsible for the majority of annual DOC loads (Inamdar et al., 2006), and the DOC–discharge relationships show that DOC export is transport-limited at the event scale (Buffam et al., 2001; Zarnetske et al., 2018). Although geomorphological and climatic conditions regulate DOC loads in aquatic ecosystems (Winterdahl et al., 2014; Laudon et al., 2012), DOM export at the annual scale is commonly conceptualized as a two-step process in which DOM is produced and stored in the catchment during the hot and dry period and then exported toward surface waters during the wet and cold period (Boyer et al., 1996). This two-step conceptual model, often described in temperate catchments (Deirmendjian et al., 2018; Strohmenger et al., 2020; Wen et al., 2020; Ruckhaus et al., 2023), is also supported by numerous studies carried out in tropical (Bouillon et al., 2014), boreal (Tiwari et al., 2022), Mediterranean (Butturini and Sabater, 2000), or Arctic fluvial networks (Neff et al., 2006). However the processes regulating the size of the riparian DOM pool remain unclear (Tank et al., 2018, and references below).
Antecedent soil conditions of wetness and temperature have been identified as a dominant control on stream DOC, with concentrations typically increasing after dry events (Turgeon and Courchesne, 2008; Vázquez et al., 2007; Mehring et al., 2013). Periods of drought promote the production and accumulation of DOM in shallow soil horizons through enhanced soil organic matter decomposition (Harrison et al., 2008; Fenner and Freeman, 2011; Xu and Saiers, 2010), resulting in high stream DOC concentrations during the subsequent rewetting phase of the catchment (Werner et al., 2019; Raymond and Saiers, 2010). In good agreement with this conceptual model is the observation based on long-term data that the mean annual DOC concentrations in streams can be related to the intensity and duration of preceding dry periods (Humbert et al., 2015; Tiwari et al., 2022).
However, the establishment of reducing conditions in riparian soils during the winter may have potential implications on our conceptualization of stream DOM export owing to the influence of redox conditions on the iron (Fe) cycle in soils. While particulate Fe hydroxides absorb organic substances with a high affinity when oxidizing conditions prevail, the microbially driven dissolution of Fe oxyhydroxides during reducing conditions leads to the release of organic molecules previously bonded to surface minerals (Hagedorn et al., 2000; Blodau et al., 2008). The release of large amounts of DOC in riparian soils during the winter period – considered non-productive in our current conceptualization of stream DOM export – has been previously reported (Lambert et al., 2013; Lotfi-Kalahroodi et al., 2021), and several studies have suggested that iron redox cycles may play a major role in catchment-scale DOM export (Knorr, 2013; Selle et al., 2019; Musolff et al., 2017). However, the onset of Fe-reducing conditions and the subsequent DOM release could be limited in agricultural catchments owing to large inputs of nitrate (an oxidizing species) from an upslope area via groundwater that may prevent Fe reductive biodissolution (McMahon and Chapelle, 2008; Christensen et al., 2000).
Because most of the studies investigating DOM export in headwater catchments rely on stream water monitoring, the processes regulating the size of the mobile DOM pool in riparian soils and the interaction with other biogeochemical cycles remain largely unknown. We still lack studies investigating how processes occurring in soil waters reflect our conceptualization of solute dynamics based on observations made in surface waters (Knorr, 2013; Dupas et al., 2015; Ledesma et al., 2015; Seibert et al., 2009; Sanderman et al., 2009; Lambert et al., 2013). In this study, we hypothesized that Fe biodissolution may significantly affect DOM release in riparian soils during the winter period with consequences for stream DOM export. We also investigated the potential influence of nitrate from agricultural origin, which may regulate Fe reduction. To this end, we installed zero-tension lysimeters in the riparian area of the Kervidy-Naizin catchment, whose stream waters are continuously monitored for water quality, including DOC at high frequency (Fovet et al., 2018). This catchment is located in Brittany (France), a region where stream DOC concentrations exhibited contrasting trends (increasing, decreasing, or no trend) over the 2007–2020 period despite similar geomorphological and climatic conditions (Fig. S1 in the Supplement). The Kervidy-Naizin catchment for instance exhibits a weak but significant increase in stream DOC concentrations over the last 2 decades (Strohmenger et al., 2020). In this context, another goal of this study was to explore the hypothesis that long-term regional decrease in nitrate inputs (Abbott et al., 2018) have impacted long-term trends in DOC through iron dynamics in riparian soils. We monitored soil water chemistry during the 2022–2023 hydrological year through measurements of not only DOC, Fe(II), and NO3 concentrations but also DOM composition (absorbance and fluorescence properties coupled with parallel factor analysis) and soluble reactive phosphorus (SRP) as an additional tracer of Fe reductive dissolution (Gu et al., 2017; Smith et al., 2021). The results allowed us to decipher complex interactions among C, N, and Fe cycles in agricultural catchments and to highlight the occurrence of several processes sustaining DOM export during the winter period.
2.1 Study site
The Kervidy-Naizin research observatory is a 4.9 km2 agricultural headwater catchment located in Brittany (western France; Fig. 1). It belongs to the French OZCAR (Critical Zone Observatories: Research and Application) network and has been instrumented since the 1970s for the long-term monitoring of the soil–atmosphere–hydrosphere continuum in a context of intensive agriculture (see Fovet et al., 2018, for a complete presentation of the study site).
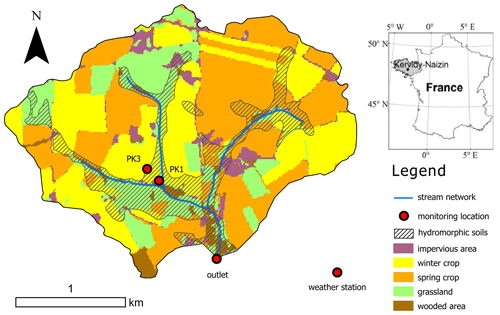
Figure 1Location map of the Kervidy-Naizin experimental catchment showing land uses. Hatched areas located along the stream channel network indicate the extent of hydromorphic soils commonly waterlogged during the winter period. Lysimeters were located downslope of piezometer PK1.
The site is characterized by gentles slopes (<5 %) and low elevation that ranges from 98–140 m above sea level. The bedrock is composed of impermeable Brioverian schists above which a locally fractured layer of schists is underlain by 1–30 m of weathered material and silty loam soils. Soils are well drained except in riparian zones, where water excess leads to hydromorphic, poorly drained soil. Soil organic carbon content presents lateral (riparian versus upland soils) and vertical (surface versus deep soils) gradients, with the highest values of about 5.3 %–5.6 % in the uppermost soil horizons (0–20 cm depth) of the riparian area, while soil organic content drops under 1 % below 20 cm depth (Lambert et al., 2011).
The land use is intensive mixed farming, with 91 % of the catchment area being used for agriculture that grows crops to feed a high density of dairy cattle, pigs, and poultry. Maize (38 %), straw cereals (30 %), and grasslands (15 %) dominate, and wooded areas are mainly confined to valley bottoms along the stream channel or to some hedgerows (Fig. 1).
The climate is temperate oceanic, with a mean annual temperature of 11.2 ± 0.6 °C and mean annual precipitation of 810 ± 180 mm. Precipitation varies seasonally throughout the year, with higher precipitation from October to February (mean monthly precipitation of 92 ± 31 mm) and lower precipitation from March to July (mean monthly precipitation of 50 ± 14 mm). The dynamics of the intermittent stream reflects the seasonal pattern of rainfall and evapotranspiration with high-discharge periods from November to April and completely dry periods lasting 1 to 3 months between July and October depending on the hydrological year.
Groundwater level fluctuations are recorded every 15 min along the Kerolland (K) transect, rainfall is monitored at hourly intervals using a weather station located ∼1400 m from the catchment outlet, and stream discharge is recorded every minute with an automatic gauge station at the outlet of the catchment. A s::scan probe is installed at the outlet of the catchment for the measurement of DOC and other variables at high frequency (Fovet et al., 2018).
2.2 Monitoring and manual sampling
We investigated the seasonal variability in riparian DOM concentration and composition using zero-tension lysimeters designed to collect free soil waters (Fig. S2) and installed in September 2022 in topsoil horizons (15 cm depth) in the Kerolland riparian zone, an area known to be a major contributor to stream DOM export in this catchment (Lambert et al., 2014). We placed the lysimeters along three lines parallel to the stream channel, about 10–20 m apart from each other and from the stream, with the aim to capture the heterogeneity of water flow paths and nitrate concentration coming from the upslope cultivated fields. Lysimeters were all located in the hydromorphic soil unit according to the soil map (Fig. 1). We installed 29 zero-tension lysimeters, but some were lost during the study period because of damage by rodents. We kept lysimeters for which at least seven consecutive dates were available, resulting in 17 lysimeters used for the study. We collected soil waters from November 2022 to June 2023 at a weekly to fortnightly frequency depending on the hydro-climatic conditions (Fig. 2). The end of sampling was imposed by the lack of water in lysimeters owing to the gradual drawdown of the water table in the riparian zone during the spring period. We sampled soil waters with a vacuum pump and filtered them at 0.2 µm with acetate cellulose syringe encapsulated filters directly on site for all analyses including DOC, NO3, SRP, Fe(II), and DOM composition (absorbance and fluorescence). We used unfiltered water samples to measure physico-chemistry variables including temperature and pH with an ODEON probe. In addition, we collected surface waters right next to the riparian area where lysimeters were located and at the outlet of the catchment. The laboratory analyses were identical for soil and surface waters.
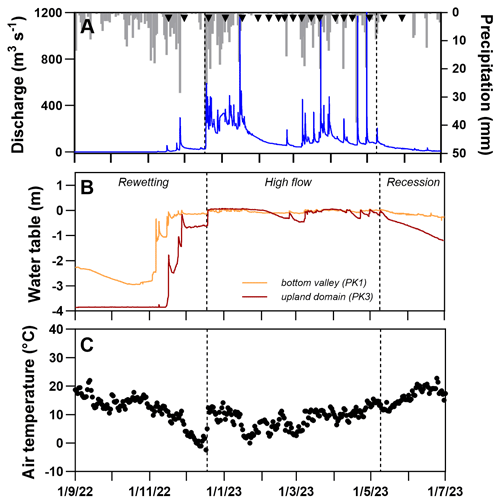
Figure 2(a) Record of hourly discharge and daily rainfall, (b) record of hourly piezometric levels in wetland (PK1) and upland (PK3) domains, and (c) record of daily air temperature. Black triangles in panel (a) indicate fieldwork for the manual sampling of soil and stream waters. Vertical dashed black lines delimit the different hydrologic periods, namely the rewetting, high-flow, and recession phases. See the text for details.
2.3 Analytical procedures
With the exception of Fe(II) measurements that were performed the same day as sampling, all analyses were done within 2 weeks after sampling. Samples were stored in a 4 °C room in the dark. Fe(II) analyses were determined using the 1.10-phenanthroline colorimetric method (Lambert et al., 2013): dissolved iron was trapped on site, and the optical density of the complex formed with phenanthroline was measured the same day once back at the laboratory at 510 nm with a UV–vis spectrophotometer. DOC concentrations were measured using a total carbon analyser (Shimadzu TOC-V) with a precision estimated at ±5 % using a standard potassium hydrogen phthalate solution (SIGMA ALDRICH). Nitrate as N– and SRP were determined by spectrometry with an automatic sequential analyser (SmartChem 200, AMS Alliance, France).
The absorbance of coloured DOM (CDOM) was measured with a LAMBDA 365 UV–vis spectrophotometer (PerkinElmer) from 200 to 700 nm (1 nm increment) using a 1 cm quartz cuvette. Samples were diluted in most cases due to the DOM-rich nature of soil waters. The only purpose of CDOM spectra was to correct excitation–emission matrices (EEMs) for inner filter effects (Ohno, 2002). The dilution factor used for fluorescence measurements was applied to CDOM spectra. Fluorescence DOM (FDOM) was collected as EEMs with a LAMBDA LS 45 (PerkinElmer) using a 1 cm quartz cuvette across excitation wavelengths of 270–450 nm (5 nm increment) and emission wavelengths of 290–600 nm (0.5 nm increment). Samples were diluted so absorbance at 254 nm was below 0.3 to reduce inner filter effects (Ohno, 2002).
In our study, the Fe(II) : DOC ratio was 0.30 ± 0.24, implying that significant interferences on DOM fluorescence from iron can be expected (Poulin et al., 2014). The degree of iron quenching, however, varies greatly between samples depending not only on the iron : DOC ratio (Pullin et al., 2007) but also on DOM composition (Jia et al., 2021; Poulin et al., 2014) and Fe(III) concentrations (Ohno et al., 2008), making it difficult to predict the influence of Fe on EEMs. That being said, quenching was clearly apparent in some samples (n<10) that showed the fluorescence intensity increasing with the dilution factor, reflecting the influence of a high level of Fe that reduces DOM fluorescence (Pullin et al., 2007). The quenching impacted EEMs at low (<270 nm) and moderate to high (420–490 nm) levels of excitation and emission wavelengths, respectively, which is consistent with previous studies concluding that Fe mainly impacts fluorescence intensity in EEM locations associated with humic-like fluorophores, namely peaks A and C (Jia et al., 2021; Poulin et al., 2014). Thus, although we cannot rule out an effect of iron on EEMs, this would have impacted the relative contribution of humic-like fluorophores associated with components C1 and C2 of our model (see below) which behaved similarly between clusters and across seasons.
2.4 PARAFAC modelling
EEM preprocessing (Raman scattering removal and standardization to Raman units) was performed prior to the PARAFAC (parallel factor) modelling. Normalization was done using a Milli-Q water sample run the same day as the sample. A five-component PARAFAC model was obtained using the drEEM 0.3.0 toolbox (Murphy et al., 2013) for MATLAB (MathWorks, Natick, MA, USA). Split-half analysis, random initialization, and the visualization of residual EEMs were used to test and validate the model. The positions of the maximum peaks of the PARAFAC components were compared to previous studies carried out in a similar context of human-impacted catchments with the open fluorescence database OpenFluor using the OpenFluor add-on for the open-source chromatography software OpenChrom (Murphy et al., 2014). The maximum fluorescence FMax values of each component for a particular sample provided by the model were summed to calculate the total fluorescence signal FTot of the sample in Raman units. The relative abundance of any particular PARAFAC component X was then calculated as .
2.5 Statistical analyses
A principal component analysis (PCA) coupled to a clustering analysis was used to discriminate and group lysimeters based on the presence or absence of iron biodissolution in soil waters. The aim was to help visualize a temporal pattern for each of the two clusters rather than 17 time series if data were plotted for each lysimeter. For this reason, data (DOC, NO3, SRP, and Fe(II) concentrations and the relative contribution of PARAFAC components) were averaged for each lysimeter and then normalized in order to spatially group the lysimeters before investigating temporal patterns. The PCA was performed using the prcomp function in the R software, and the factoextra package was used to identify the variables that contribute the most to the first two dimensions of the PCA. The cluster analysis, based on the results from the PCA and called hierarchical clustering on principal components (Josse, 2010), was performed with the FactoMineR package for R (Lê et al., 2008). Relationships between variables were investigated either through Pearson or Spearman correlations depending of the nature (linear or not) of the correlations.
3.1 Hydro-climatic context
The hydrological regime of the study site is characterized by a succession of three distinct periods determined by water table fluctuations along the hillslope, corresponding to different hydrological regimes for the riparian soils (Fig. 2; Lambert et al., 2013): (i) a period of progressive rewetting of riparian soils after the dry season and of low groundwater flow and low stream discharge (1 September–18 December 2022; mean and cumulated precipitation of 5.1 ± 5.3 mm d−1 and 338.5 mm, respectively); (ii) a period of prolonged waterlogging of riparian soils induced by the rise in the water table in the upland domain, corresponding to high values of hillslope groundwater flow and stream discharge (18 December 2022–9 May 2023; mean and cumulated precipitation of 6.8 ± 7.9 mm d−1 and 573 mm, respectively); and (iii) a period of drainage and progressive drying of the riparian soils induced by the drawdown first in the upland domain then in the downland domain and corresponding to the decrease in both the hillslope groundwater flow and stream discharge (9 May–1 July 2023; mean and cumulated precipitation of 4.3 ± 4.4 mm d−1 and 42.5 mm, respectively). Air temperature (Fig. 2c) showed a smoothed seasonal variability with decreasing values from September to December (from ∼20 to −2 °C) followed by a rise in temperature from 0 to 20 °C from February to July. This pattern was only interrupted by a relatively short episode of higher temperature (close to 10 °C) during the winter, coinciding with the first intense rainfall period of the year.
3.2 Fluorescence properties of DOM
Five PARAFAC components were identified in soil waters (Fig. S3), all of which have already been described in previous studies. All five components had humic-like fluorescence properties (Fellman et al., 2010). Components C1 (excitation and emission peaks of 350 and 444 nm), C2 (<270 and 450 nm), and C5 (410 and 488 nm) predominantly cover the regions of EEMs associated with peaks A and C and are common tracers of terrestrially derived DOM in surface waters (Kothawala et al., 2015; Stedmon and Markager, 2005; Logozzo et al., 2023; Lambert et al., 2017), while C3 (330 and 406 nm) and C4 (295 and 410 nm) are both located near classical peak M, indicating a microbial transformation of terrestrial DOM (Williams et al., 2010; Lambert et al., 2022; Yamashita et al., 2010). The maximum fluorescence intensity of all components were strongly related to DOC concentrations (not shown), and the relative contribution of each component is ordered in a decreasing manner as .
3.3 Seasonal variations in soil and stream waters
Temperature in soil waters (Fig. 3a) followed the same pattern as air temperature: values oscillated between 5 and 15 °C during November–January, reached minimums between 4 and 7 °C in January–March, and then increased gradually during the end of the study period up to 18–20 °C in June. pH varied between 6.2 and 7.4 (mean of 6.9 ± 0.3) across lysimeters and did not exhibit significant trends over the study period (Fig. 3b). Solutes, however, exhibited complex patterns with a high variability across lysimeters and time, especially during the high-flow period (Fig. 3c–f). Despite the fact that lysimeters were installed along three lines ranging 10–30 m from the stream, no spatial pattern was identified. Overall, these elements were strongly linked to each other (Fig. 4). DOC concentrations ranged from 2.3 to 87.4 mg L−1 (mean of 30.2 ± 12.8 mg L−1) over the study period and were linearly and positively (Pearson r of 0.73, p value of <0.0001) associated with Fe(II) that ranged from 0 to 45.8 mg L−1 (mean of 9.8 ± 7.6 mg L−1). Fe(II) was negatively (Spearman r of −0.56, p value of <0.0001) correlated with NO3 (from 0 to 16.4 mg L−1, mean of 0.9 ± 1.1 mg L−1), and SRP (from 0 to 0.5 mg L−1, mean of 0.1 ± 0.1 mg L−1) was also positively (Pearson r of 0.21, p value of 0.0005) related to Fe(II) but not as strongly as for DOC.
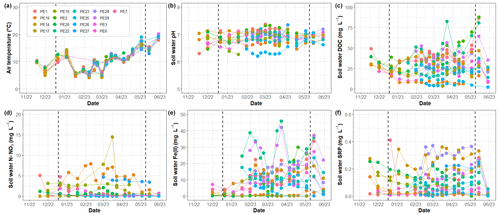
Figure 3Evolution of (a) air temperature and (b) pH, (c) DOC, (d) NO3, (e) Fe(II), and (f) SRP in soil waters during the study period. Vertical dashed black lines delimit the different hydrologic periods, namely the rewetting, high-flow, and recession phases. See the text for details. PE1 to PE29 refer to the lysimeters.
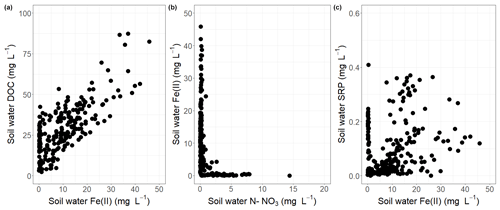
Figure 4Relationships between (a) DOC and Fe(II), (b) Fe(II) and NO3, and (c) SRP and Fe(II) in soil waters during the study period.
DOC concentrations in stream waters varied from 2.9 to 36.8 mg L−1 during the study period (Fig. 5). Maximum concentrations were reached during storm events due to a rapid response to rainfall and the mobilization of riparian wetland waters (Durand and Juan Torres, 1996). There was a tendency for minimum (at base flow) and maximum (at peak discharge) concentrations to decrease from November to March. From March to July, however, minimal concentrations remained stable, while maximum values showed a slight increasing trend.
3.4 Clustering of soil waters
The first two components of the PCA explained 69.4 % of the total variance of the data and discriminated lysimeters depending on the presence or absence of Fe(II) biodissolution in soil waters of the riparian area (Fig. 6). The first principal component (PC1; 54 % of the total variance) was mainly related to NO3 concentrations and terrestrial humic-like components (C1, C2, and C5) regarding positive scores and to DOC and Fe(II) concentrations and the microbial humic-like component C4 regarding negative scores. The second component (PC2; 15.4 % of the total variance) was related to SRP (positive score) and the component C3 (negative score). PARAFAC components had similar or even higher scores than DOC, Fe(II), and NO3 concentrations regarding the two first dimensions of the PCA (Fig. S4), illustrating the importance of DOM composition as an important contributing factor in explaining the spatial variability across lysimeters. The distribution of PARAFAC components along the first dimension reflects the relationships between their relative contribution and Fe(II) concentrations (not shown). More specifically, C4 was strongly and positively correlated with Fe(II) (R2 of 0.38, Pearson r of 0.62) compared to other components that exhibited the weakest and negative relationships with Fe(II) (R2 from 0.09 to 0.19, Pearson r from −0.30 to −0.43). In other words, lysimeters capturing Fe biodissolution in the riparian area were associated with high DOC and a greater proportion of the microbial component C4 compared to lysimeters enriched in nitrate where no Fe(II) was measured.
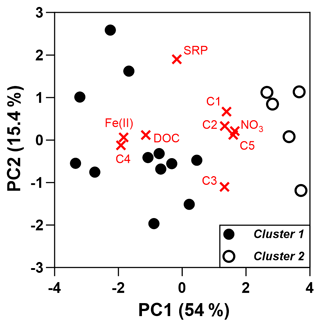
Figure 6PCA biplot, including the loading plot for the input variables and score plot for lysimeters. One point represents one lysimeter, with PCA being based on average values calculated over the study period. Markers are coloured according to the cluster identified by the hierarchical clustering on principal components (see “Material and methods”).
The hierarchical clustering based on the PCA results grouped the lysimeters into two distinct clusters based on the presence (cluster 1) or absence (cluster 2) of Fe(II) (Fig. 6). This approach allowed us to gain insight into the temporal evolution of solutes in soil waters since clear patterns appeared once the data were grouped by cluster (Fig. 7). In cluster 1, DOC, N–NO3, and SRP decreased from 39.8 ± 13.3 to 23.4 ± 8.4 mg L−1, from 2.6 ± 3.6 to 1.2 ± 1.8 mg L−1, and from 0.18 ± 0.18 to 0.08 ± 0.15 mg L−1, respectively, during the rewetting phase of the catchment, while Fe(II) was not measured at significant levels. During the high-flow period, however, Fe(II) increased gradually from 3.7 ± 3.2 to 26.5 ± 7.8 mg L−1, and both DOC and SRP followed a similar trend, with concentrations raising from 27.3 ± 9.5 to 54.9 ± 25.0 mg L−1 and from 0.07 ± 0.13 to 0.18 ± 0.11 mg L−1, respectively. During this period and until the end of the hydrological cycle, N–NO3 values were very low, decreasing from 0.54 ± 0.66 mg L−1 at the beginning of the high-flow period to values below 0.15 mg L−1 during the rest of the survey. The start of the third hydrological period, corresponding to the drawdown of the water table and the consecutive aeration of riparian soils, was marked by the rapid drop of values, with Fe(II) at 8.1 ± 7.4 mg L−1, DOC at 17.5 ± 10.9 mg L−1, and SRP at 0.02 ± 0.02 mg L−1.
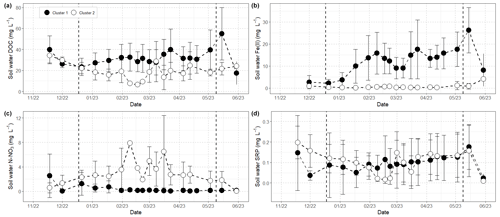
Figure 7Evolution of (a) DOC, (b) Fe(II), (c) NO3, and (d) SRP in soil waters for each cluster. Lysimeters are grouped according the hierarchical clustering on principal components (see the text and Fig. 6 for details). Vertical dashed black lines delimit the different hydrologic periods, namely the rewetting, high-flow, and recession phases. See the text for details.
Similarly to cluster 1, soil waters from the cluster 2 exhibited a decline in DOC and SRP concentrations during the rewetting phase of the catchment, but these trends continued during the high-flow period, with minimal values reached in the middle of February. Thus, DOC dropped from 34.5 ± 7.1 to 9.4 ± 3.1 mg L−1 and SRP dropped from 0.19 ± 0.08 to 0.02 ± 0.01 mg L−1 during this period, before showing an increasing trend and reaching concentrations of about 21.0 ± 6.1 mg L−1 for DOC and 0.16 ± 0.13 mg L−1 for SRP at the end of the high-flow period. DOC remained elevated (24.1 ± 3.1 mg L−1) at the start of the dry period, but SRP dropped close to depletion. In contrast, N–NO3 first increased from 0.57 ± 0.81 mg L−1 in November to maximum values of 6.5 ± 5.9 mg L−1 in the middle of March and then exhibited decreasing concentrations until a complete depletion at the beginning of the third hydrological period. Contrary to cluster 1, Fe(II) was not measured at significant concentrations in cluster 2 (i.e. below 0.5 mg L−1), except in March, during which Fe(II) increased from 1.2 ± 1.9 to 4.1 ± 0.2 mg L−1.
4.1 The buffering effect of nitrate on iron reductive dissolution
The reductive biodissolution of iron during the high-water winter period is a recurrent process in riparian soils of headwater catchments (Smolders et al., 2017; Knorr, 2013; Selle et al., 2019). The magnitude of variations in Fe(II) and associated DOC and SRP dynamics reported in this study are in line with previous works conducted in the same research catchment (Lambert et al., 2013; Lotfi-Kalahroodi et al., 2021; Gu et al., 2017). In addition, our results evidenced a marked variability in the intensity of iron dissolution across lysimeters that we attributed to the spatial distribution of NO3-rich water flow paths that can inhibit and delay the release of Fe(II) and DOC in soil waters.
A fundamental condition for the establishment of reductive conditions is the prolonged waterlogging of riparian soils. As shown earlier for this and other lowland catchments on impervious bedrock, the increase in the hydraulic gradient induced by the rise in groundwater in the upland domain during the high-flow period maintains a strong hydrologic connection between upland and riparian domains (Pacific et al., 2010; Molenat et al., 2008). Under these conditions, riparian soils remain waterlogged, owing to a high and continuous hillslope groundwater flow, leading to the gradual establishment of reductive conditions and the subsequent triggering of Fe biodissolution as long as inputs of oxidizing species remained limited and/or counterbalanced by a higher rate of consumption through microbial activity (Lotfi-Kalahroodi et al., 2021; Lambert et al., 2013). This pattern is well illustrated by data from lysimeters of the first cluster (Fig. 7). After a quick depletion of an initial stock of nitrate accumulated during the previous summer, reductive conditions were rapidly established at the beginning of the high-flow period and increasing Fe(II) concentrations in soil waters led to the onset of the reductive Fe biodissolution in riparian soils. The gradual increase in Fe(II) during the high-flow period despite variations in temperature or rainfall patterns (with some intense precipitation events of >20 mm d−1) suggests a limited impact for these climatic episodes, except during a period of low precipitation during which both Fe(II) and DOC exhibited a slight decrease in February–March. We attributed this small drop to the drawdown of the water table in upland groundwater flow following a prolonged absence of precipitation (see PK3 fluctuations, Fig. 2) that may have re-oxygenated soil waters (as no changes in N–NO3 occurred).
Therefore, a large release of DOC occurred in soils of the first cluster. Iron biodissolution also affected SRP, but the relationship was weaker, suggesting that the reductive dissolution of soil Fe was not the primary driver of SRP concentrations in soils. For instance, soil properties and, more specifically, soil phosphorus content and speciation have been shown to strongly regulate SRP in soil waters of the Kervidy-Naizin catchment (Gu et al., 2017). Regarding DOC, the mean DOC : Fe(II) molar ratio was 142.4 ± 285.5. This was higher than the DOC : Fe(II) ratio measured in experimental conditions (74.5 ± 74.6) but similar to value measured in the field (134.4 ± 25.6) by Lotfi-Kalahroodi et al. (2021), who aimed to investigate Fe reduction in the riparian area of our study catchment. Fe(III) concentrations in soil waters were not measured, but, based on the work of Lotfi-Kalahroodi et al. (2021), we can estimate a ratio between total Fe and Fe(II) of 4.8. Keeping in mind that this is a rough estimation, our mean DOC : Fe ratio would be about 29.3 ± 58.8, which is consistent with previous studies (e.g. Selle et al., 2019; Musolff et al., 2017; Grybos et al., 2009; Cabezas et al., 2013). The nature of processes releasing DOC upon the reduction in soil Fe oxyhydroxides in the riparian soils of our study site has been studied in laboratory conditions (Grybos et al., 2009). Results have shown that up to 60 % of the release is due to DOC desorption caused by the pH increase that accompanies the reduction in Fe oxyhydroxides in these soils, with the remaining 40 % being due to the dissolution of Fe oxyhydroxides that strongly adsorb organic compounds previously bonded to surface minerals (e.g. Hagedorn et al., 2000). In good agreement with these results, soil DOC was positively related to pH (Fig. S5). The abrupt decrease in DOC in June illustrates the restoration of aerobic conditions owing to the drawdown of the water table in the downland domain that led to the formation of Fe minerals and the subsequent retention of DOC and SRP (Gu et al., 2017).
Lysimeters from the second cluster showed a very different pattern. Although some of them were located close (3–4 m) to lysimeters in which reducing conditions prevailed, there was no evidence of Fe(II) release, arguably because of the presence of nitrate. Indeed, in agreement with studies carried out in wetland (Lucassen et al., 2004) and lacustrine (Andersen, 1982) sediments, we argue that the Fe biodissolution was inhibited as long as long as a sufficient quantity of NO3 remained in soil waters. In the absence of such a production or regeneration process, both DOC and SRP showed a net depletion pattern from November to March. The influence of nitrate as a buffer of Fe biodissolution was furthermore supported by the observation of a slight release of Fe(II) in May, at a moment when nitrate became depleted from soil waters, probably because of plant uptake. Interestingly, we found that the threshold value of nitrate above which the process is activated (based on the NO3–Fe(II) relationship (Fig. 4) as well as the timing of Fe biodissolution identified in cluster 1 and cluster 2) ranged between 1.2 and 1.8 N–NO3 (4.1–6.2 mg L−1), which is close to the threshold value of 6 mg L−1 established at the catchment scale by Musolff et al. (2017) in German streams.
The PARAFAC components identified in the model suggest a dominance of highly aromatic and conjugated molecules across all lysimeters and dates, which is typical of DOM derived from soil organic matter and found in poorly drained soils in riparian or wetland areas (Sanderman et al., 2009; Lambert et al., 2013; Yamashita et al., 2010). The larger proportion of C4 in the first cluster however indicates that the Fe oxyhydroxide reduction leads to a greater proportion of microbially derived compounds within the DOM pool. In agreement with previous studies showing that the Fe(III) reduction could enhance the decomposition of organic matter in soils (Chen et al., 2020; Kappler et al., 2021), the close link between Fe(II) and C4 likely reflects an indirect effect of Fe biodissolution promoting the degradation of soil OM and the subsequent incorporation of microbially derived compounds into the DOM pool (Dong et al., 2023). This hypothesis is very consistent with previous experimental studies performed with soils from the Kervidy-Naizin riparian area which showed that bacterial reduction in Fe(III) oxides to Fe(II) was concomitant with the release of large biological organic byproducts upon the growth of bacterial communities (Lotfi-Kalahroodi et al., 2021).
Our study evidences a strong spatial heterogeneity of the establishment of reducing conditions in the riparian area of the Kervidy-Naizin catchment associated with differences in the composition of DOM released in soil waters. However, the reason for such variability in biogeochemical processes in riparian soils remains to be determined. A first explanation can be related to the heterogeneity in water flow paths in soils. In intensive agricultural catchments such as our study site, the inflow of NO3-rich water may arise from the rise in contaminated groundwater in valley bottoms and/or from subsurface flow paths that connect upland soils to riparian soils (Molenat et al., 2008). It is likely that lysimeters from the second cluster captured preferential flow paths of NO3-rich waters, while lysimeters from the first cluster were disconnected from that preferential water circulation. Alternatively, the absence of nitrate in soil waters may arise from a higher rate of denitrification that counterbalanced NO3 inputs. Research based on field observation remained limited to deciphering the respective role of hydrology versus biogeochemistry in controlling Fe(II) biodissolution in riparian soils, and experimental studies would be required to provide more quantitative values for these potential drivers and their interactions.
4.2 Implication for stream DOM export at the catchment scale
The current understanding of DOM export in headwater catchments is based on a two-step conceptual model, in which a pool of mobile DOM is built in soils during the dry season and then flushed towards surface waters during the following wet season (e.g. Tiwari et al., 2022; Ruckhaus et al., 2023; Strohmenger et al., 2020; Raymond and Saiers, 2010). However, the high-frequency measurements of DOC in the stream do not fully support this statement. The establishment of a hydrological connection between riparian soils and the stream during the winter period showed the stream DOC to gradually decrease both at peak discharge during successive storm events and at base flow during inter-storm periods (Fig. 5). This pattern, which repeats every year in this catchment (Strohmenger et al., 2020), is very consistent with the hypothesis of the mobilization and exhaustion of a DOM pool limited in size built during the summer period (Humbert et al., 2015). However, stream DOC values were found to increase slightly in March–April after the low-flow period that showed the hydrological connection between soils and the stream decreasing. It is unlikely that the mobilization of an additional pool of DOM from upland soils may explain these small raises in stream DOC because this pool is (1) relatively small in terms of size and (2) quickly exhausted at the beginning of the winter period (Lambert et al., 2014). Therefore, the seasonal pattern of stream DOC likely reflects the regeneration of the riparian DOM pool during the winter period as shown by our data collected in soil waters of riparian wetlands.
Stable carbon isotopes have indeed demonstrated that riparian soils of the Kervidy-Naizin catchment – and more particularly the DOM-rich uppermost soil horizons – are the dominant source of stream DOC at the catchment scale (Lambert et al., 2014), a feature commonly shared by headwater catchments (e.g. Sanderman et al., 2009). Thus, the decline in DOC and SRP observed in soil waters, particularly in the second cluster, whereby these elements became almost depleted (Fig. 7), was consistent with the general flushing behaviour of the catchment shown by stream DOC from November to February. Similarly, the large 2- to 3-fold increase in DOC concentrations in riparian soils (in clusters 1 and 2, respectively) denotes a large mobilization of DOM between March and May despite wet and low-temperature conditions, which could explain in turn the pattern observed in stream DOC at the same time. While part of this regeneration can be attributed to iron biodissolution, the release of a large amount of DOC in cluster 2, where the reductive biodissolution of Fe(III) was limited, implies that another production mechanism contributed to releasing DOM in riparian soils. It is unlikely that agricultural inputs (crop residues, manure application, etc.) mainly explain the increases in the riparian area, as these sources are episodic and/or size-limited (Lambert et al., 2014; Humbert et al., 2015; Pacific et al., 2010). This observation echoes previous works from the Kervidy-Naizin catchment showing effective inter-annual regeneration mechanisms of the pool of soluble phosphorus in soils unrelated to iron dynamics (Gu et al., 2017), a statement supported here by the fact that SRP concentrations followed a pattern similar to DOC in soils grouped in the second cluster (Fig. 7).
The PARAFAC results suggest that DOM mobilized from soil to streams is only composed of aromatic molecules of high molecular weight. Although complex organic molecules indeed dominate stream DOM export (Fellman et al., 2009), it should be noted, however, that protein-like components are commonly found in stream waters (Inamdar et al., 2012), including in our study site (Humbert et al., 2020). The lack of such components in our model results from our sampling approach and not from their absence in catchment soils. Indeed, the production of protein-like components in catchment soils is restricted to the hot and dry summer period during which a pool made of low-aromatic and microbially derived compounds built up in riparian soils (Lambert et al., 2013). However, this DOM pool is quickly flushed and exhausted during the rewetting phase in October–November, and soil DOM during the winter period is mainly composed of highly aromatic molecules originating from soil organic material (Lambert et al., 2014). Agricultural practices such as fertilizer applications can represent another source of protein-like DOM in the catchment (Humbert et al., 2020), but these inputs remain episodic with a low impact on DOM at the catchment scale (Humbert et al., 2015; Lambert et al., 2014). For instance, a recent year of monitoring soil waters at different locations in the catchment has shown that protein-like components represent only 3.44 % ± 2.8 % of the total fluorescence signal in catchment soils, with this contribution being particularly low in riparian areas (Humbert et al., 2020). Therefore, the absence of protein-like components in our PARAFAC model is the consequence of our sampling design that focused on DOM production mechanisms in riparian soils (distant from agricultural inputs) during the winter period (period of production of highly aromatic compounds in soils).
Taken together, our results have two important implications regarding our conceptualization of DOM export in headwater catchments. First, it challenges the idea that the wet period acts solely as a passive export period for DOM, with no or little DOM production (Strohmenger et al., 2020; Ruckhaus et al., 2023; Wen et al., 2020). Second, it emphasis that stream DOC dynamics at the outlet is an integrative signal, potentially masking the high spatial heterogeneity of the system owing to complex interactions between biogeochemical cycles in soils, nutrient transfer at the soil–stream interface, and the hydrological functioning of catchments. While the patterns of stream DOC were consistent with those observed in soils, our study remains however limited in its capacity to quantify the relative contribution of the cluster identified to stream DOC export. Additionally, we do not have the necessary data such as isotopes or molecular markers to elucidate the precise origin and DOM (and SRP) release in soils unrelated to iron biodissolution, and this should be the focus of future work combining experimental and field studies.
The combined monitoring of soil and stream waters in a temperate headwater catchment allowed us to evidence the dual role of the high-flow period as both an active phase of DOM production and export. In agreement with previous studies (e.g. Selle et al., 2019; Knorr, 2013), the establishment of Fe-reducing conditions in riparian areas was identified as a major mechanism for the release of a large amount of DOM in soil waters. In agricultural catchments, however, we found that this process can be buffered by nitrate, leading to a strong spatial heterogeneity in the magnitude of iron biodissolution and its consequences on soil DOM dynamics. Our study also evidenced that another production mechanism unrelated to Fe dynamics contributed to the release of DOM in riparian soils during the winter period, pointing to the need to further investigate stream DOC export at the soil–stream interface.
The interactions between the N and Fe biogeochemical cycles may have potential implications regarding long-term increases in DOC in the streams of Brittany. Indeed, stream DOC in the Kervidy-Naizin catchment has been slowly but significantly increasing in the last 2 decades, and this trend is mirrored by a decline in NO3 concentrations (Strohmenger et al., 2020). While part of the DOC trend can be related to changes in climatic conditions as winters have tended to wetter over the years (Strohmenger et al., 2020), the long-term decline in N inputs from agriculture may have favoured the increase in stream DOC by enhancing Fe(II) biodissolution in riparian soils. This hypothesis could partly explain why catchments having similar geomorphological and climatic properties present contrasting long-term trends at the scale of the Brittany region (Fig. S1). Indeed, nitrate concentrations have largely decreased during the last decades, but the rate of recovery is not uniform across the region (Abbott et al., 2018). Studies carried out at the regional scale aiming to decipher the interactions between the local (agricultural practices) and global (climatic conditions) scale and the consequences to stream DOC export would be critical considering the influence of DOM on water quality and on the ecological and biogeochemical functioning of surface waters.
Data on soil waters is published on https://doi.org/10.5281/zenodo.13950018 (Lambert, 2024). Hydrological and climatic data from the Kervidy-Naizin site are available at https://geosas.fr/web/?page_id=103 (last access: 18 October 2024).
The supplement related to this article is available online at: https://doi.org/10.5194/bg-21-4533-2024-supplement.
TL conceived the study. TL defined protocols with contributions from RD and PD. TL collected field samples with help from RD. TL performed laboratory analysis. TL analysed the data and drafted the manuscript with input from RD and PD. All authors contributed to and approved the manuscript.
The contact author has declared that none of the authors has any competing interests.
Publisher's note: Copernicus Publications remains neutral with regard to jurisdictional claims made in the text, published maps, institutional affiliations, or any other geographical representation in this paper. While Copernicus Publications makes every effort to include appropriate place names, the final responsibility lies with the authors.
We thank Militza Gallardo, Harald Edmond Fiala de Saint Denis, Patrice Petitjean, and Céline Bouillis for their assistance with fieldwork and lab work. We thank the associate editor, Ji-Hyung Park, and Clayton Williams, Benny Selle, and one anonymous reviewer for constructive comments on the previous version of the manuscript.
This research has been supported by the European Research Council H2020 project through the Marie Skłodowska-Curie Actions (COSTREAM; grant agreement no. 101064945).
This paper was edited by Ji-Hyung Park and reviewed by Clayton Williams and Benny Selle and one anonymous referee.
Abbott, B. W., Moatar, F., Gauthier, O., Fovet, O., Antoine, V., and Ragueneau, O.: Trends and seasonality of river nutrients in agricultural catchments: 18 years of weekly citizen science in France, Sci. Total Environ., 624, 845–858, https://doi.org/10.1016/j.scitotenv.2017.12.176, 2018.
Ågren, A., Buffam, I., Jansson, M., and Laudon, H.: Importance of seasonality and small streams for the landscape regulation of dissolved organic carbon export, J. Geophys. Res.-Biogeo., 112, G03003, https://doi.org/10.1029/2006JG000381, 2007.
Aiken, G. R., Hsu-Kim, H., and Ryan, J. N.: Influence of Dissolved Organic Matter on the Environmental Fate of Metals, Nanoparticles, and Colloids, Environ. Sci. Technol., 45, 3196–3201, https://doi.org/10.1021/es103992s, 2011.
Andersen, J. M.: Effect of nitrate concentration in lake water on phosphate release from the sediment, Water Res., 16, 1119–1126, https://doi.org/10.1016/0043-1354(82)90128-2, 1982.
Battin, T. J., Kaplan, L. A., Findlay, S., Hopkinson, C. S., Marti, E., Packman, A. I., Newbold, J. D., and Sabater, F.: Biophysical controls on organic carbon fluxes in fluvial networks, Nat. Geosci., 1, 95–100, https://doi.org/10.1038/ngeo101, 2008.
Blodau, C., Fulda, B., Bauer, M., and Knorr, K.-H.: Arsenic speciation and turnover in intact organic soil mesocosms during experimental drought and rewetting, Geochim. Cosmochim. Ac., 72, 3991–4007, https://doi.org/10.1016/j.gca.2008.04.040, 2008.
Bouillon, S., Yambele, A., Gillikin, D. P., Teodoru, C., Darchambeau, F., Lambert, T., and Borges, A. V.: Contrasting biogeochemical characteristics of the Oubangui River and tributaries (Congo River basin), Sci. Rep.-UK, 4, 5402, https://doi.org/10.1038/srep05402, 2014.
Boyer, E. W., Hornberger, G. M., Bencala, K. E., and McKnight, D.: Overview of a simple model describing variation of dissolved organic carbon in an upland catchment, Ecol. Model., 86, 183–188, https://doi.org/10.1016/0304-3800(95)00049-6, 1996.
Buffam, I., Galloway, J. N., Blum, L. K., and McGlathery, K. J.: A stormflow/baseflow comparison of dissolved organic matter concentrations and bioavailability in an Appalachian stream, Biogeochemistry, 53, 269–306, https://doi.org/10.1023/A:1010643432253, 2001.
Butturini, A. and Sabater, F.: Seasonal variability of dissolved organic carbon ina Mediterranean stream, Biogeochemistry, 51, 303–321, https://doi.org/10.1023/A:1006420229411, 2000.
Cabezas, A., Gelbrecht, J., and Zak, D.: The effect of rewetting drained fens with nitrate-polluted water on dissolved organic carbon and phosphorus release, Ecol. Eng., 53, 79–88, https://doi.org/10.1016/j.ecoleng.2012.12.016, 2013.
Chen, C., Hall, S. J., Coward, E., and Thompson, A.: Iron-mediated organic matter decomposition in humid soils can counteract protection, Nat. Commun., 11, 2255, https://doi.org/10.1038/s41467-020-16071-5, 2020.
Chow, A. T., Gao, S., and Dahlgren, R. A.: Physical and chemical fractionation of dissolved organic matter and trihalomethane precursors: A review, J. Water Supply Res. T., 54, 475–507, https://doi.org/10.2166/aqua.2005.0044, 2005.
Christensen, T. H., Bjerg, P. L., Banwart, S. A., Jakobsen, R., Heron, G., and Albrechtsen, H.-J.: Characterization of redox conditions in groundwater contaminant plumes, J. Contam. Hydrol., 45, 165–241, https://doi.org/10.1016/S0169-7722(00)00109-1, 2000.
Creed, I. F., McKnight, D. M., Pellerin, B. A., Green, M. B., Bergamaschi, B. A., Aiken, G. R., Burns, D. A., Findlay, S. E. G., Shanley, J. B., Striegl, R. G., Aulenbach, B. T., Clow, D. W., Laudon, H., McGlynn, B. L., McGuire, K. J., Smith, R. A., and Stackpoole, S. M.: The river as a chemostat: fresh perspectives on dissolved organic matter flowing down the river continuum, Can. J. Fish. Aquat. Sci., 72, 1272–1285, https://doi.org/10.1139/cjfas-2014-0400, 2015.
de Wit, H. A., Stoddard, J. L., Monteith, D. T., Sample, J. E., Austnes, K., Couture, S., Fölster, J., Higgins, S. N., Houle, D., Hruška, J., Krám, P., Kopáček, J., Paterson, A. M., Valinia, S., Van Dam, H., Vuorenmaa, J., and Evans, C. D.: Cleaner air reveals growing influence of climate on dissolved organic carbon trends in northern headwaters, Environ. Res. Lett., 16, 104009, https://doi.org/10.1088/1748-9326/ac2526, 2021.
Dean, J. F., Meisel, O. H., Martyn Rosco, M., Marchesini, L. B., Garnett, M. H., Lenderink, H., van Logtestijn, R., Borges, A. V., Bouillon, S., Lambert, T., Röckmann, T., Maximov, T., Petrov, R., Karsanaev, S., Aerts, R., van Huissteden, J., Vonk, J. E., and Dolman, A. J.: East Siberian Arctic inland waters emit mostly contemporary carbon, Nat. Commun., 11, 1627, https://doi.org/10.1038/s41467-020-15511-6, 2020.
Deirmendjian, L., Loustau, D., Augusto, L., Lafont, S., Chipeaux, C., Poirier, D., and Abril, G.: Hydro-ecological controls on dissolved carbon dynamics in groundwater and export to streams in a temperate pine forest, Biogeosciences, 15, 669–691, https://doi.org/10.5194/bg-15-669-2018, 2018.
Dong, H., Zeng, Q., Sheng, Y., Chen, C., Yu, G., and Kappler, A.: Coupled iron cycling and organic matter transformation across redox interfaces, Nature Reviews Earth & Environment, 4, 659–673, https://doi.org/10.1038/s43017-023-00470-5, 2023.
Dupas, R., Gruau, G., Gu, S., Humbert, G., Jaffrézic, A., and Gascuel-Odoux, C.: Groundwater control of biogeochemical processes causing phosphorus release from riparian wetlands, Water Res., 84, 307–314, https://doi.org/10.1016/j.watres.2015.07.048, 2015.
Durand, P. and Juan Torres, J. L.: Solute transfer in agricultural catchments: the interest and limits of mixing models, J. Hydrol., 181, 1–22, https://doi.org/10.1016/0022-1694(95)02922-2, 1996.
Fellman, J. B., Hood, E., D'Amore, D. V., Edwards, R. T., and White, D.: Seasonal changes in the chemical quality and biodegradability of dissolved organic matter exported from soils to streams in coastal temperate rainforest watersheds, Biogeochemistry, 95, 277–293, https://doi.org/10.1007/s10533-009-9336-6, 2009.
Fellman, J. B., Hood, E., and Spencer, R. G. M.: Fluorescence spectroscopy opens new windows into dissolved organic matter dynamics in freshwater ecosystems: A review, Limnol. Oceanogr., 55, 2452–2462, https://doi.org/10.4319/lo.2010.55.6.2452, 2010.
Fenner, N. and Freeman, C.: Drought-induced carbon loss in peatlands, Nat. Geosci., 4, 895–900, https://doi.org/10.1038/ngeo1323, 2011.
Fovet, O., Ruiz, L., Gruau, G., Akkal, N., Aquilina, L., Busnot, S., Dupas, R., Durand, P., Faucheux, M., Fauvel, Y., Fléchard, C., Gilliet, N., Grimaldi, C., Hamon, Y., Jaffrezic, A., Jeanneau, L., Labasque, T., Le Henaff, G., Mérot, P., Molénat, J., Petitjean, P., Pierson-Wickmann, A.-C., Squividant, H., Viaud, V., Walter, C., and Gascuel-Odoux, C.: AgrHyS: An Observatory of Response Times in Agro-Hydro Systems, Vadose Zone J., 17, 180066, https://doi.org/10.2136/vzj2018.04.0066, 2018.
Grybos, M., Davranche, M., Gruau, G., Petitjean, P., and Pédrot, M.: Increasing pH drives organic matter solubilization from wetland soils under reducing conditions, Geoderma, 154, 13–19, https://doi.org/10.1016/j.geoderma.2009.09.001, 2009.
Gu, S., Gruau, G., Dupas, R., Rumpel, C., Crème, A., Fovet, O., Gascuel-Odoux, C., Jeanneau, L., Humbert, G., and Petitjean, P.: Release of dissolved phosphorus from riparian wetlands: Evidence for complex interactions among hydroclimate variability, topography and soil properties, Sci. Total Environ., 598, 421–431, https://doi.org/10.1016/j.scitotenv.2017.04.028, 2017.
Hagedorn, F., Kaiser, K., Feyen, H., and Schleppi, P.: Effects of Redox Conditions and Flow Processes on the Mobility of Dissolved Organic Carbon and Nitrogen in a Forest Soil, J. Environ. Qual., 29, 288–297, https://doi.org/10.2134/jeq2000.00472425002900010036x, 2000.
Hanson, P. C., Pace, M. L., Carpenter, S. R., Cole, J. J., and Stanley, E. H.: Integrating Landscape Carbon Cycling: Research Needs for Resolving Organic Carbon Budgets of Lakes, Ecosystems, 18, 363–375, https://doi.org/10.1007/s10021-014-9826-9, 2015.
Harrison, A. F., Taylor, K., Scott, A., Poskitt, J., Benham, D., Grace, J., Chaplow, J., and Rowland, P.: Potential effects of climate change on DOC release from three different soil types on the Northern Pennines UK: examination using field manipulation experiments, Glob. Change Biol., 14, 687–702, https://doi.org/10.1111/j.1365-2486.2007.01504.x, 2008.
Humbert, G., Jaffrezic, A., Fovet, O., Gruau, G., and Durand, P.: Dry-season length and runoff control annual variability in stream DOC dynamics in a small, shallow groundwater-dominated agricultural watershed, Water Resour. Res., 51, 7860–7877, https://doi.org/10.1002/2015WR017336, 2015.
Humbert, G., Parr, T. B., Jeanneau, L., Dupas, R., Petitjean, P., Akkal-Corfini, N., Viaud, V., Pierson-Wickmann, A.-C., Denis, M., Inamdar, S., Gruau, G., Durand, P., and Jaffrézic, A.: Agricultural Practices and Hydrologic Conditions Shape the Temporal Pattern of Soil and Stream Water Dissolved Organic Matter, Ecosystems, 23, 1325–1343, https://doi.org/10.1007/s10021-019-00471-w, 2020.
Inamdar, S., Finger, N., Singh, S., Mitchell, M., Levia, D., Bais, H., Scott, D., and McHale, P.: Dissolved organic matter (DOM) concentration and quality in a forested mid-Atlantic watershed, USA, Biogeochemistry, 108, 55–76, https://doi.org/10.1007/s10533-011-9572-4, 2012.
Inamdar, S. P., O'Leary, N., Mitchell, M. J., and Riley, J. T.: The impact of storm events on solute exports from a glaciated forested watershed in western New York, USA, Hydrol. Process., 20, 3423–3439, https://doi.org/10.1002/hyp.6141, 2006.
Jia, K., Manning, C. C. M., Jollymore, A., and Beckie, R. D.: Technical note: Effects of iron(II) on fluorescence properties of dissolved organic matter at circumneutral pH, Hydrol. Earth Syst. Sci., 25, 4983–4993, https://doi.org/10.5194/hess-25-4983-2021, 2021.
Josse, J.: Principal component methods – hierarchical clustering – partitional clustering: why would we need to choose for visualizing data?, Technical Report – Applied Mathematics Department, https://www.semanticscholar.org/paper/Principal-component-methods-hierarchical-clustering-Josse/04335d99d840ac3370f5aeb262828cf127d3ff1c (last access: March 2023), 2010.
Kappler, A., Bryce, C., Mansor, M., Lueder, U., Byrne, J. M., and Swanner, E. D.: An evolving view on biogeochemical cycling of iron, Nat. Rev. Microbiol., 19, 360–374, https://doi.org/10.1038/s41579-020-00502-7, 2021.
Kelly, D. J., Clare, J. J., and Bothwell, M. L.: Attenuation of solar ultraviolet radiation by dissolved organic matter alters benthic colonization patterns in streams, J. N. Am. Benthol. Soc., 20, 96–108, https://doi.org/10.2307/1468191, 2001.
Knorr, K.-H.: DOC-dynamics in a small headwater catchment as driven by redox fluctuations and hydrological flow paths – are DOC exports mediated by iron reduction/oxidation cycles?, Biogeosciences, 10, 891–904, https://doi.org/10.5194/bg-10-891-2013, 2013.
Kothawala, D. N., Ji, X., Laudon, H., Ågren, A. M., Futter, M. N., Köhler, S. J., and Tranvik, L. J.: The relative influence of land cover, hydrology, and in-stream processing on the composition of dissolved organic matter in boreal streams, J. Geophys. Res.-Biogeo., 120, 1491–1505, https://doi.org/10.1002/2015JG002946, 2015.
Lambert, T.: The role of nitrogen and iron biogeochemical cycles in the production and export of dissolved organic matter in agricultural headwater catchments, Zenodo [data set], https://doi.org/10.5281/zenodo.13950018, 2024.
Lambert, T., Pierson-Wickmann, A.-C., Gruau, G., Thibault, J.-N., and Jaffrezic, A.: Carbon isotopes as tracers of dissolved organic carbon sources and water pathways in headwater catchments, J. Hydrol., 402, 228–238, https://doi.org/10.1016/j.jhydrol.2011.03.014, 2011.
Lambert, T., Pierson-Wickmann, A.-C., Gruau, G., Jaffrezic, A., Petitjean, P., Thibault, J.-N., and Jeanneau, L.: Hydrologically driven seasonal changes in the sources and production mechanisms of dissolved organic carbon in a small lowland catchment, Water Resour. Res., 49, 5792–5803, https://doi.org/10.1002/wrcr.20466, 2013.
Lambert, T., Pierson-Wickmann, A.-C., Gruau, G., Jaffrezic, A., Petitjean, P., Thibault, J. N., and Jeanneau, L.: DOC sources and DOC transport pathways in a small headwater catchment as revealed by carbon isotope fluctuation during storm events, Biogeosciences, 11, 3043–3056, https://doi.org/10.5194/bg-11-3043-2014, 2014.
Lambert, T., Bouillon, S., Darchambeau, F., Morana, C., Roland, F. A. E., Descy, J.-P., and Borges, A. V.: Effects of human land use on the terrestrial and aquatic sources of fluvial organic matter in a temperate river basin (The Meuse River, Belgium), Biogeochemistry, 136, 191–211, https://doi.org/10.1007/s10533-017-0387-9, 2017.
Lambert, T., Perolo, P., Escoffier, N., and Perga, M.-E.: Enhanced bioavailability of dissolved organic matter (DOM) in human-disturbed streams in Alpine fluvial networks, Biogeosciences, 19, 187–200, https://doi.org/10.5194/bg-19-187-2022, 2022.
Laudon, H., Buttle, J., Carey, S. K., McDonnell, J., McGuire, K., Seibert, J., Shanley, J., Soulsby, C., and Tetzlaff, D.: Cross-regional prediction of long-term trajectory of stream water DOC response to climate change, Geophys. Res. Lett., 39, L18404, https://doi.org/10.1029/2012GL053033, 2012.
Lê, S., Josse, J., and Husson, F.: FactoMineR: An R Package for Multivariate Analysis, J. Stat. Softw., 25, 1–18, https://doi.org/10.18637/jss.v025.i01, 2008.
Ledesma, J. L. J., Grabs, T., Bishop, K. H., Schiff, S. L., and Köhler, S. J.: Potential for long-term transfer of dissolved organic carbon from riparian zones to streams in boreal catchments, Glob. Change Biol., 21, 2963–2979, https://doi.org/10.1111/gcb.12872, 2015.
Logozzo, L. A., Hosen, J. D., McArthur, J., and Raymond, P. A.: Distinct drivers of two size fractions of operationally dissolved iron in a temperate river, Limnol. Oceanogr., 68, 1185–1200, https://doi.org/10.1002/lno.12338, 2023.
Lotfi-Kalahroodi, E., Pierson-Wickmann, A.-C., Rouxel, O., Marsac, R., Bouhnik-Le Coz, M., Hanna, K., and Davranche, M.: More than redox, biological organic ligands control iron isotope fractionation in the riparian wetland, Sci. Rep.-UK, 11, 1933, https://doi.org/10.1038/s41598-021-81494-z, 2021.
Lucassen, E. C. H. E. T., Smolders, A. J. P., van der Salm, A. L., and Roelofs, J. G. M.: High groundwater nitrate concentrations inhibit eutrophication of sulphate-rich freshwater wetlands, Biogeochemistry, 67, 249–267, https://doi.org/10.1023/B:BIOG.0000015342.40992.cb, 2004.
McMahon, P. B. and Chapelle, F. H.: Redox Processes and Water Quality of Selected Principal Aquifer Systems, Groundwater, 46, 259–271, https://doi.org/10.1111/j.1745-6584.2007.00385.x, 2008.
Mehring, A. S., Lowrance, R. R., Helton, A. M., Pringle, C. M., Thompson, A., Bosch, D. D., and Vellidis, G.: Interannual drought length governs dissolved organic carbon dynamics in blackwater rivers of the western upper Suwannee River basin, J. Geophys. Res.-Biogeo., 118, 1636–1645, https://doi.org/10.1002/2013JG002415, 2013.
Molenat, J., Gascuel-Odoux, C., Ruiz, L., and Gruau, G.: Role of water table dynamics on stream nitrate export and concentration in agricultural headwater catchment (France), J. Hydrol., 348, 363–378, https://doi.org/10.1016/j.jhydrol.2007.10.005, 2008.
Monteith, D. T., Stoddard, J. L., Evans, C. D., de Wit, H. A., Forsius, M., Høgåsen, T., Wilander, A., Skjelkvåle, B. L., Jeffries, D. S., Vuorenmaa, J., Keller, B., Kopácek, J., and Vesely, J.: Dissolved organic carbon trends resulting from changes in atmospheric deposition chemistry, Nature, 450, 537–540, https://doi.org/10.1038/nature06316, 2007.
Murphy, K. R., Stedmon, C. A., Graeber, D., and Bro, R.: Fluorescence spectroscopy and multi-way techniques. PARAFAC, Anal. Methods-UK, 5, 6557–6566, https://doi.org/10.1039/C3AY41160E, 2013.
Murphy, K. R., Stedmon, C. A., Wenig, P., and Bro, R.: OpenFluor– an online spectral library of auto-fluorescence by organic compounds in the environment, Anal. Methods-UK, 6, 658–661, https://doi.org/10.1039/C3AY41935E, 2014.
Musolff, A., Selle, B., Büttner, O., Opitz, M., and Tittel, J.: Unexpected release of phosphate and organic carbon to streams linked to declining nitrogen depositions, Glob. Change Biol., 23, 1891–1901, https://doi.org/10.1111/gcb.13498, 2017.
Neff, J. C., Finlay, J. C., Zimov, S. A., Davydov, S. P., Carrasco, J. J., Schuur, E. A. G., and Davydova, A. I.: Seasonal changes in the age and structure of dissolved organic carbon in Siberian rivers and streams, Geophys. Res. Lett., 33, L23401, https://doi.org/10.1029/2006GL028222, 2006.
Ohno, T.: Fluorescence Inner-Filtering Correction for Determining the Humification Index of Dissolved Organic Matter, Environ. Sci. Technol., 36, 742–746, https://doi.org/10.1021/es0155276, 2002.
Ohno, T., Amirbahman, A., and Bro, R.: Parallel Factor Analysis of Excitation–Emission Matrix Fluorescence Spectra of Water Soluble Soil Organic Matter as Basis for the Determination of Conditional Metal Binding Parameters, Environ. Sci. Technol., 42, 186–192, https://doi.org/10.1021/es071855f, 2008.
Pacific, V. J., Jencso, K. G., and McGlynn, B. L.: Variable flushing mechanisms and landscape structure control stream DOC export during snowmelt in a set of nested catchments, Biogeochemistry, 99, 193–211, https://doi.org/10.1007/s10533-009-9401-1, 2010.
Poulin, B. A., Ryan, J. N., and Aiken, G. R.: Effects of Iron on Optical Properties of Dissolved Organic Matter, Environ. Sci. Technol., 48, 10098–10106, https://doi.org/10.1021/es502670r, 2014.
Pullin, M. J., Anthony, C., and Maurice, P. A.: Effects of Iron on the Molecular Weight Distribution, Light Absorption, and Fluorescence Properties of Natural Organic Matter, Environ. Eng. Sci., 24, 987–997, https://doi.org/10.1089/ees.2006.0040, 2007.
Raymond, P. A. and Saiers, J. E.: Event controlled DOC export from forested watersheds, Biogeochemistry, 100, 197–209, https://doi.org/10.1007/s10533-010-9416-7, 2010.
Ruckhaus, M., Seybold, E. C., Underwood, K. L., Stewart, B., Kincaid, D. W., Shanley, J. B., Li, L., and Perdrial, J. N.: Disentangling the responses of dissolved organic carbon and nitrogen concentrations to overlapping drivers in a northeastern United States forested watershed, Frontiers in Water, 5, 1–4, https://doi.org/10.3389/frwa.2023.1065300, 2023.
Sanderman, J., Lohse, K. A., Baldock, J. A., and Amundson, R.: Linking soils and streams: Sources and chemistry of dissolved organic matter in a small coastal watershed, Water Resour. Res., 45, W03418, https://doi.org/10.1029/2008WR006977, 2009.
Seibert, J., Grabs, T., Köhler, S., Laudon, H., Winterdahl, M., and Bishop, K.: Linking soil- and stream-water chemistry based on a Riparian Flow-Concentration Integration Model, Hydrol. Earth Syst. Sci., 13, 2287–2297, https://doi.org/10.5194/hess-13-2287-2009, 2009.
Selle, B., Knorr, K.-H., and Lischeid, G.: Mobilisation and transport of dissolved organic carbon and iron in peat catchments – Insights from the Lehstenbach stream in Germany using generalised additive models, Hydrol. Process., 33, 3213–3225, https://doi.org/10.1002/hyp.13552, 2019.
Smith, G. J., McDowell, R. W., Condron, L. M., Daly, K., Ó hUallacháin, D., and Fenton, O.: Reductive dissolution of phosphorus associated with iron-oxides during saturation in agricultural soil profiles, J. Environ. Qual., 50, 1207–1219, https://doi.org/10.1002/jeq2.20256, 2021.
Smolders, E., Baetens, E., Verbeeck, M., Nawara, S., Diels, J., Verdievel, M., Peeters, B., De Cooman, W., and Baken, S.: Internal Loading and Redox Cycling of Sediment Iron Explain Reactive Phosphorus Concentrations in Lowland Rivers, Environ. Sci. Technol., 51, 2584–2592, https://doi.org/10.1021/acs.est.6b04337, 2017.
Stedmon, C. A. and Markager, S.: Resolving the variability in dissolved organic matter fluorescence in a temperate estuary and its catchment using PARAFAC analysis, Limnol. Oceanogr., 50, 686–697, https://doi.org/10.4319/lo.2005.50.2.0686, 2005.
Strohmenger, L., Fovet, O., Akkal-Corfini, N., Dupas, R., Durand, P., Faucheux, M., Gruau, G., Hamon, Y., Jaffrezic, A., Minaudo, C., Petitjean, P., and Gascuel-Odoux, C.: Multitemporal Relationships Between the Hydroclimate and Exports of Carbon, Nitrogen, and Phosphorus in a Small Agricultural Watershed, Water Resour. Res., 56, e2019WR026323, https://doi.org/10.1029/2019WR026323, 2020.
Tank, S. E., Fellman, J. B., Hood, E., and Kritzberg, E. S.: Beyond respiration: Controls on lateral carbon fluxes across the terrestrial-aquatic interface, Limnology and Oceanography Letters, 3, 76–88, https://doi.org/10.1002/lol2.10065, 2018.
Tiwari, T., Sponseller, R. A., and Laudon, H.: The emerging role of drought as a regulator of dissolved organic carbon in boreal landscapes, Nat. Commun., 13, 5125, https://doi.org/10.1038/s41467-022-32839-3, 2022.
Turgeon, J. M. L. and Courchesne, F.: Hydrochemical behaviour of dissolved nitrogen and carbon in a headwater stream of the Canadian Shield: relevance of antecedent soil moisture conditions, Hydrol. Process., 22, 327–339, https://doi.org/10.1002/hyp.6613, 2008.
Vázquez, E., Romaní, A. M., Sabater, F., and Butturini, A.: Effects of the Dry–Wet Hydrological Shift on Dissolved Organic Carbon Dynamics and Fate Across Stream–Riparian Interface in a Mediterranean Catchment, Ecosystems, 10, 239–251, https://doi.org/10.1007/s10021-007-9016-0, 2007.
Wen, H., Perdrial, J., Abbott, B. W., Bernal, S., Dupas, R., Godsey, S. E., Harpold, A., Rizzo, D., Underwood, K., Adler, T., Sterle, G., and Li, L.: Temperature controls production but hydrology regulates export of dissolved organic carbon at the catchment scale, Hydrol. Earth Syst. Sci., 24, 945–966, https://doi.org/10.5194/hess-24-945-2020, 2020.
Werner, B. J., Musolff, A., Lechtenfeld, O. J., de Rooij, G. H., Oosterwoud, M. R., and Fleckenstein, J. H.: High-frequency measurements explain quantity and quality of dissolved organic carbon mobilization in a headwater catchment, Biogeosciences, 16, 4497–4516, https://doi.org/10.5194/bg-16-4497-2019, 2019.
Wetzel, R. G.: Gradient-dominated ecosystems: sources and regulatory functions of dissolved organic matter in freshwater ecosystems, Hydrobiologia, 229, 181–198, https://doi.org/10.1007/BF00007000, 1992.
Williams, C. J., Yamashita, Y., Wilson, H. F., Jaffé, R., and Xenopoulos, M. A.: Unraveling the role of land use and microbial activity in shaping dissolved organic matter characteristics in stream ecosystems, Limnol. Oceanogr., 55, 1159–1171, https://doi.org/10.4319/lo.2010.55.3.1159, 2010.
Winterdahl, M., Erlandsson, M., Futter, M. N., Weyhenmeyer, G. A., and Bishop, K.: Intra-annual variability of organic carbon concentrations in running waters: Drivers along a climatic gradient, Global Biogeochem. Cy., 28, 451–464, https://doi.org/10.1002/2013GB004770, 2014.
Xu, N. and Saiers, J. E.: Temperature and Hydrologic Controls on Dissolved Organic Matter Mobilization and Transport within a Forest Topsoil, Environ. Sci. Technol., 44, 5423–5429, https://doi.org/10.1021/es1002296, 2010.
Yamashita, Y., Scinto, L. J., Maie, N., and Jaffé, R.: Dissolved Organic Matter Characteristics Across a Subtropical Wetland's Landscape: Application of Optical Properties in the Assessment of Environmental Dynamics, Ecosystems, 13, 1006–1019, https://doi.org/10.1007/s10021-010-9370-1, 2010.
Zarnetske, J. P., Bouda, M., Abbott, B. W., Saiers, J., and Raymond, P. A.: Generality of Hydrologic Transport Limitation of Watershed Organic Carbon Flux Across Ecoregions of the United States, Geophys. Res. Lett., 45, 11702–11711, https://doi.org/10.1029/2018GL080005, 2018.