the Creative Commons Attribution 4.0 License.
the Creative Commons Attribution 4.0 License.
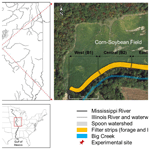
Biomass yield potential, feedstock quality, and nutrient removal of perennial buffer strips under continuous zero fertilizer application
Cheng-Hsien Lin
Colleen Zumpf
Chunhwa Jang
Thomas Voigt
Guanglong Tian
Olawale Oladeji
Albert Cox
Rehnuma Mehzabin
DoKyoung Lee
Perennial-based buffer strips have been promoted as having the potential for improving ecosystem services from riparian areas while producing biomass as livestock feed or as a bioenergy feedstock. Both biomass production and nutrient removal of buffer strips are substantially influenced by the vegetation types for the multipurpose perennial buffers. In this 2016–2019 study in western Illinois, two perennial cropping systems, including forage crops composed of cool-season grass mixtures (forage system) and bioenergy crops made up of warm-season grass mixtures (bioenergy system), were used to establish buffer strips for assessing biomass production, feedstock quality, nutrient removal, and buffer longevity. Treatments for this study reflecting agronomic practices included (1) two harvests occurring in summer (at anthesis) and fall (after complete senescence) and (2) one harvest in fall for the forage system (two-cut vs. one-cut forage) and (3) one fall harvest for the bioenergy system (one-cut bioenergy). Successively harvesting without any fertilizer input resulted in a yield decline in forage biomass over 3 years by approximately 30 % (6.3 to 4.4 Mg DM ha−1 (dry matter) with a rate of 1.0 Mg ha−1 yr−1) in the two-cut forage and by 35 % (4.9 to 3.2 Mg DM ha−1 with a rate of 0.9 Mg ha−1 yr−1) in the one-cut forage systems. The feed quality also decreased over the years by showing declined rates of 12.9 (crucial protein), 0.9 (calcium), 0.7 (copper), and 1.3 g kg−1 DM yr−1 (zinc). Empirical models predicted enteric CH4 emissions from cattle ranged from 225.7 to 242.6 g per cow per day based on the feed nutritive values. In contrast, bioenergy biomass yield increased by 27 % from 4.9 to 6.7 Mg DM ha−1 with a consistent quality (cellulose of ∼ 397.9 g kg−1; hemicellulose of ∼ 299.4 g kg−1), corresponding to the increased total theoretical ethanol yield from 1.8 × 103 to 2.4 × 103 L ha−1 (∼ 33 % increase). Annual nutrient removals of N, P, K, Ca, and Mg were significantly higher in the forage systems (e.g., two-cut: 52.6–106.9 kg N ha−1; one-cut: 44.5–84.1 kg N ha−1) than those in the bioenergy system (e.g., 25.9–34.4 kg N ha−1); however, the removal rate declined rapidly over 3 years (e.g., ∼ 49 % reduction) as the annual biomass yield declined in the forage systems. This on-farm field study demonstrated the potential of the perennial crop used as buffer strip options for biomass production and buffer sustainability at the edge of the field.
- Article
(2473 KB) - Full-text XML
-
Supplement
(377 KB) - BibTeX
- EndNote
The edges of croplands are usually less productive or profitable for conventional row crop cultivation due to rapid nutrient transportation via surface runoff and leaching (Dodds and Oakes, 2008; David et al., 2010). Field borders are hotspots for severe environmental degradation where substantial erosion and nutrient loss to adjacent waterbodies can result in poor soil and water quality and contribute to downstream impacts such as the Gulf of Mexico hypoxic zone (Turner and Rabalais, 1994; Rabalais et al., 2002; Vitousek et al., 2009; Márquez et al., 2017). Planting buffer strips along field edges can effectively combat these problems by preventing sediment transportation and intercepting/removing excess nutrients (Lovell and Sullivan, 2006; Sweeney and Newbold, 2014; Schmitt et al., 1999; Gopalakrishnan et al., 2012). Perennial grasses such as tall fescue (cool-season C3 forage grasses), switchgrass, and Miscanthus (warm-season C4 bioenergy grasses) species are suggested as good candidates for buffer strips due to their high stress tolerances and efficient uptake abilities (Dosskey, 2001; Clausen et al., 2000; Vogel et al., 2002; Mulkey et al., 2006; Varvel et al., 2008; van der Weijde et al., 2013). These perennials have shown great potential for providing numerous ecosystems services (e.g., the mitigation of soil erosion and greenhouse gas emissions, the improvement of soil health and biodiversity, soil carbon sequestration) for marginal areas (Lee et al., 2007a, b; Monti et al., 2012; Yang et al., 2019). Also, a productive perennial system may provide landowners with alternative income sources through the sale of harvested biomass as either forage or bioenergy feedstocks (Lee et al., 2018; Mehmood et al., 2017; Eranki et al., 2013; Anderson et al., 2016; Golkowska et al., 2016).
A minimum requirement of a successful perennial buffer is to offer stable yield production with high-quality feedstock. The key is to establish a persistent buffer system to serve the agroecosystem of marginal croplands continuously. For perennial monocultures, a successively productive system can be achieved under appropriate management. For instance, well-managed alfalfa and switchgrass can last 5–10 years or longer under proper nutrient and harvest management (Bélanger et al., 2006; Parrish and Fike, 2005; Fike et al., 2006a; Lee et al., 2018). Harvest management, mainly the harvest frequency (e.g., single cut vs. multiple cut) and timing (e.g., at anthesis/peak standing vs. at the end of growing season/after killing frost), can be identified as the most critical factors influencing the perennial's health and production (Guretzky et al., 2011). Frequent harvesting could improve the annual biomass yield, especially for cool-season grasses that typically have two growth cycles (spring and late summer/fall) every year (MacAdam and Nelson, 2003); however, intensive harvest (e.g., more than three harvests every year) likely reduces perennials regrowth vigor compared to less intensive harvest management (e.g., two harvests every year) (Bélanger et al., 2006). For warm-season grasses, a single annual harvest has been suggested to optimize biomass yield, minimize energy inputs, and sustain long-term biomass production (Sanderson et al., 1999; Mitchell and Schmer, 2012). Harvest timing is another key factor ensuring a sustainable perennial system. Many studies show delayed harvest after plant senescence substantially improves stand regrowth potential and longevity by increasing internal nutrient cycling and lengthening the time for root development and productive tiller growth (Mitchell and Schmer, 2012; Vogel et al., 2002; Lee et al., 2014; Zumpf et al., 2019).
Compared to monoculture systems, the perennial polyculture buffer strips showed more advantages in terms of ecosystem services, resistance to weed and pest pressure, and biomass productivity (Carlsson et al., 2017; De Deyn et al., 2011; Dhakal and Islam, 2018; Jungers et al., 2015; Nyfeler et al., 2011; Quijas et al., 2010; Sanderson et al., 2012; Suter et al., 2015; Yang et al., 2019; Lin et al., 2022). Nevertheless, it is more complicated and challenging to optimize management practices in polycultures (e.g., grass mixtures) than in monoculture systems for simultaneously achieving stable productivity, feedstock quality, and system longevity because each species responds differently to different treatments. For instance, studies have observed increasing nitrogen (N) inputs usually benefited the biomass yield of perennial grasses but less so for legume production and persistence (Harmoney et al., 2016; Lee et al., 2013; Lin et al., 2022). From a quality standpoint, additional N input improved the quality of forage feedstocks (e.g., cool-season grasses) by increasing protein content in plant tissue but lowered the quality of lignocellulosic feedstocks (e.g., warm-season grasses) by reducing the concentration of cell wall components and increasing the biomass N and ash contents for bioenergy production (Hodgson et al., 2010; Ibrahim et al., 2017; Lin et al., 2022). For harvest management, our previous studies showed that a delayed harvest after complete senescence and less frequent harvest practices can improve the sustainability of the legume–grass polycultures established on Conservation Reserve Program (CRP) lands, in terms of the long-term productivity and vegetative vigor (Lin et al., 2022).
Perennial-plant buffers designed for multifunctionality offer opportunities to bridge biomass production and ecosystem services in agricultural watersheds; however, limited regional information has identified optimal plant types coupled with specific practices for producers (Lovell and Sullivan, 2006; Golkowska et al., 2016). Research on site-specific and continuous cropping practices can provide local farmers with valuable information for developing strategic plans for improving financial performance and the sustainability of buffer systems, simultaneously. The overall goals of this study were to evaluate the effect of minimum management efforts (only harvest without nutrient applications) on biomass yield, feedstock quality, nutrient removal, and the buffer sustainability of cool- and warm-season perennial mixtures cultivated on low-fertility croplands. Since no additional fertilizer was applied to the buffer strips, the nutrients from parent soil materials and transported from the main crop fields via leaching and surface runoff were the only nutrient sources for the perennials. Thus, it was hypothesized that the warm-season perennial buffer (bioenergy system) could be more sustainable than the cool-season perennials (forage system) due to its high nutrient use efficiency, great ability of nutrient scavenging, and stress tolerance for the marginal area (van der Weijde et al., 2013; Pedroso et al., 2014; Lee et al., 2018). Besides the evaluation of the continuous biomass supply of both forage and bioenergy-type buffers under local common harvest practices, the specific objectives of this study were to assess their (1) feedstock quality, including nutritive values of crude protein (CP), crude fibers of neutral detergent fiber (NDF), acid detergent fiber (ADF), the digestibility-related indices, and other essential elements for ruminant feeds, and (2) dynamics of cell wall compositions (e.g., cellulose, hemicellulose, and lignin) for lignocellulosic biofuel production. Furthermore, enteric methane (CH4) from ruminants constitutes a significant portion (approximately 32 %) of global anthropogenic methane emissions, with cattle alone accounting for about 75 % of these emissions from livestock (UNEP and CCAC report, 2021). Since the production of methane is notably influenced by the intake and quality of feed, the last objective of this study was to employ empirical models to predict enteric CH4 production based on forage nutritive qualities.
2.1 Site description
This study was conducted during the 2016–2019 growing seasons in a riparian area of a 10 ha field located in Fulton County, Illinois (IL; 40°28′23′′ N; 90°6′44′′ W), with an annual corn–soybean (C–S) rotation cropping history (Fig. 1). The site is in a temperate climate region with a 30-year average annual temperature of 11 °C and annual precipitation of 970 mm. Weather information, including monthly temperature and precipitation, and cumulative precipitation, from 2016–2019, along with 30-year averages (1990–2019) were obtained from the National Oceanic and Atmospheric Administration for Fulton County, IL (Peoria International Airport station, USW00014842), and shown in Fig. 2. The field is mostly a Sawmill silty clay loam soil (fine–silty, mixed, superactive, mesic Cumulic Endoaquolls), while Wakeland silt loam (coarse–silty, mixed, superactive, nonacid, mesic Aeric Fluvaquents) is found at its southern edge along Big Creek (soil survey, USDA, 2016). Nine soil cores (0–100 cm) were collected across the riparian area in 2016, and each core was segmented into 0–5, 5–10, 10–20, 20–30, 30–60, and 60–100 cm depths and analyzed for more soil details (Table 1).
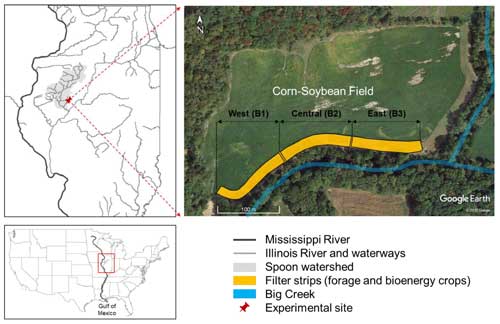
Figure 1The research site was in the Spoon watershed in Fulton County, Illinois (IL; 40°28′23′′ N, 90°6′44′′ W). The perennial-based buffer strips were established at the edge of the farm field with continuous corn–soybean rotation from 2016–2019 (the letter B means block for the experimental design) (Image source: © Google Earth 2020).
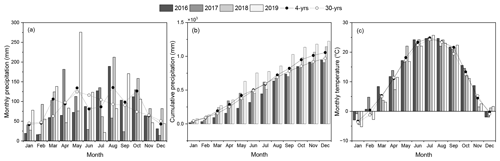
Figure 2Local weather conditions at the experimental site located in Fulton County, IL, across the 4 years of study (2016–2019), including (a) monthly and (b) cumulative precipitation and (c) monthly average temperature and the 30-year monthly average (1990–2019) (data: NOAA).
Table 1Basic physical and chemical properties of soil profiles (0–100 cm) of the buffer strips prior to the study in 2016. Three blocks were compared from different depths using a two-way ANOVA with a significance level of 0.05. The block (B), the soil depth, and their interactions were considered fixed factors, while the replicates were considered random. The lowercase letters indicate mean separation organized from the highest to the lowest value for each column using the Tukey test (no mean separations were applied if the variable effect was not significant).
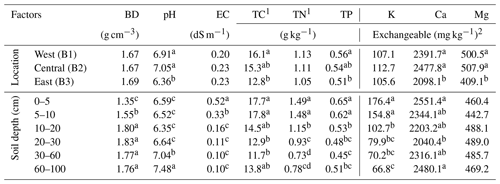
BD: bulk density; EC: electrical conductivity; TC: total carbon; TN: total nitrogen; TP: total phosphorus (direct colorimetric method). 1 TC and TN were determined by dry-combustion method. 2 Exchangeable nutrients (K, Ca, Mg) determined by the Mehlich-3 method.
2.2 Experimental design
Two crop systems (forage and bioenergy) and two harvest frequencies (two-cut or one-cut annually) were used in the experiment. The forage-crop system (forage system) was composed of alfalfa (Medicago sativa L., AL) at a seeding rate of 5.6 kg ha−1 and cool-season grasses at a total seeding rate of 28 kg ha−1, which included smooth bromegrass (Bromus inermis Leyss., 20 %, SB), tall fescue (Lolium arundinaceum (Schreb.) Darbysh., 20 %, TF), orchardgrass (Dactylis glomerata L., 20 %, OR), perennial ryegrass (Lolium perenne L. ssp. perenne, 15 %, PR), timothy grass (Phleum pratense L., 15 %, TG), and meadow fescue (Schedonorus pratensis (Huds.) P. Beauv., 10 %, MF). The bioenergy-crop system (bioenergy system) was composed of warm-season grasses at a seeding rate of 12 kg ha−1, including switchgrass (Panicum virgatum L., 40 %, SW), big bluestem (Andropogon gerardi Vitman, 20 %, BB), Indiangrass (Sorghastrum nutans (L.) Nash, 20 %, IN), and prairie cordgrass (Spartina pectinata Link, 20 %, PC). The seeding rate of both cool- and warm-season grass mixtures was 323 pure live seeds (PLSs) for every square meter. Based on the grass growing behavior, the forage system was either harvested twice, at anthesis in early summer (June) and at the end of the growing season (after complete senescence) in the fall (two-cut), or once in the fall (one-cut) annually (October–November). The bioenergy system was only harvested in the fall (Table 2). Thus, three treatments, including two forage (two-cut vs. one-cut) and one bioenergy (one-cut) systems, were used in this experiment within a randomized complete block with three replicates at each location. For each treatment, the plot size was approximately 385 m2.
Table 2Cropping systems, plant species composition, harvest frequency (once and twice), and harvest dates from 2017 to 2019 for perennial buffer strips established at Fulton County, IL.
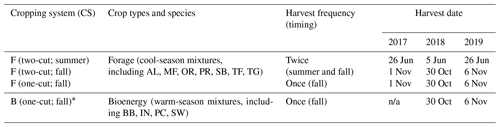
F (two-cut; summer): forages with two-harvest management (first harvest in summer ranging from 1 June to 31 August). F (two-cut; fall): forages with two-harvest management (second harvest in fall ranging from 1 September to 31 November). F (one-cut; fall): forages with one-harvest management in fall. B (one-cut; fall): bioenergy crop with one-harvest management in fall. * Bioenergy crops were not harvested in 2017 for biomass nutrient analysis due to insufficient biomass production, and this system was reestablished in 2017. AL: alfalfa; BB: big bluestem; IN: Indiangrass; MF: meadow fescue; OR: orchardgrass; PC: prairie cordgrass; PR: perennial ryegrass; SB: smooth bromegrass; SW: switchgrass; TF: tall fescue; TG: timothy grass. n/a: not applicable.
2.3 Field management
The buffer strips were established in May 2016. Disking was conducted for seedbed preparation, and herbicide was used to control weeds prior to planting. Glyphosate was applied in the forage systems, and atrazine was applied in the bioenergy system. The seeds were directly drilled into a firm, non-tilled seedbed at approximately 10 mm deep with a row spacing of 15 cm using a Great Plains no-till drill (Salina, KS, USA). Replanting was done for the bioenergy system in April 2017 due to the substantial loss of grasses resulting from the herbicide drift from the C–S field. Before the replanting, the plots were burned to remove dead biomass residues, and atrazine was applied 2 weeks later to control weeds. For the fertilizer management in the C–S field, aqueous urea ammonium nitrate [UAN: N(32 %)–P2O5(0 %)–K2O(0 %)] was used as the N source, and 202 kg N ha−1 was applied before corn planting. Diammonium phosphate [DAP: N(18 %)–P2O5(46 %)–K2O(0 %)] was applied to soybean at the rate of 28 kg P2O5 ha−1 before planting. No fertilizers were applied to the buffer strips of either perennial system. The first harvest year was different between the two crop systems. For the forage system, the first harvest occurred in 2017 because of the insufficient biomass in the establishment year of 2016. For the bioenergy system, the first harvest year was delayed to 2018 due to the reestablishment in 2017.
2.4 Data collection, analysis, and calculations
Aboveground biomass from a 14 m × 2.8 m area in each forage and bioenergy system was harvested at a height of 10 cm using a biomass plot harvester (Cibus S, Wintersteiger, Salt Lake City, UT, USA). The fresh weight of the harvested biomass from each plot was measured, and the dry-matter (DM) weight was determined by placing wet-matter subsamples (∼ 1 kg) in a forced-air oven at 60 °C for 5 d. The oven-dried biomass was then weighed and ground to pass through a 1 mm screen using a Retsch cutting mill (Retsch Inc., Haan, Germany) for feedstock quality analysis and biomass nutrients. Fiber analyses were analyzed by measuring the concentrations of neutral detergent fiber (NDF), acid detergent fiber (ADF), and acid detergent lignin (ADL), which were analyzed using a sequential extraction and filtration process and an ANKOM 200 fiber analyzer (ANKOM Technology, Fairport, NY, USA). Plant-tissue ash content was determined from the mass lost by placing the dry sample in a muffle furnace at 600 °C for 8 h. For the feedstock nutrient analysis, the dried biomass was analyzed by a dry-combustion method using a LECO FP-528 N determinator (LECO Inc., St. Joseph, MI, USA). Other macro- and micro-nutrients were measured using inductively coupled plasma (ICP) spectrometry with optical emission spectrometry (Thermo Scientific iCAP 6500 Duo ICP, Thermo Fisher Scientific Inc., Waltham, MA, USA) following a concentrated nitrate acid and hydrochloric acid microwave digestion procedure in a MARSXpress vessel (CEM, Matthews, NC, USA).
Feedstock quantity and quality indices were calculated on a dry-matter basis using the measured fiber compositions (i.e., NDF, ADF, and ADL) and equations shown in Table 3 (Ball et al., 2017; Ameen et al., 2019). Dry-matter intake (DMI) served as a quantitative index of animal feed to estimate the daily feed consumption per animal on a dry-matter basis using the biomass NDF concentration. Quality indices included crude protein (CP), dry-matter digestibility (DMD), total digestible nutrient (TDN), net energy for lactation (NEL), and relative feed value (RFV). For bioenergy productions based on the biochemical process, the concentrations of cellulose and hemicellulose (Hemi-C), theoretical ethanol yield (TEY), and total theoretical ethanol yield (TTEY) are commonly used indices. TEY was estimated based on the concentrations of cellulose and Hemi-C, and TTEY was predicted by multiplying TEY with biomass yield.
Table 3Calculations of feedstock quality indices.
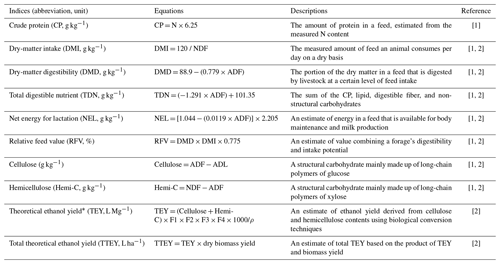
* F1 = 0.51 (the coefficient of conversion of sugar to ethanol); F2 = 0.85 (the conversion efficiency of sugar to ethanol; F3 = 1.11 (the coefficient of conversion of cellulose and hemicellulose to sugar); F4 = 0.85 (the conversion efficiency of cellulose and hemicellulose to sugar); ρ=0.79 g mL−1 (the specific gravity of ethanol). [1] Ball et al. (2017). [2] Ameen et al. (2019).
Enteric CH4 production from dairy and beef cattle was estimated using nine published models in this study (Table 4). The predictions were based on the relationship between CH4 production (a response variable) and the forage nutritive quality (predictor variables), including the chemical compositions (i.e., CP, NDF, ADF, and ADL) and derived indices (mainly DMI). These models were developed using the dataset from (1) actual measurements of CH4 productions (e.g., respiratory chamber or sulfur hexafluoride (SF6) tracers) and (2) the predictor variables with the lowest root mean square prediction error (RMSPE) shown in the original articles (Ellis et al., 2007; Nielsen et al., 2013). Most of these models required the daily dry-matter intake (dDMI, kg d−1). Although the DMI was commonly reported on a per unit animal basis, in this study, the dDMI was calculated by multiplying the DMI (expressed in per unit of body weight for comparisons across different animals and regions, g kg−1) with the average body weight of a cow across North America, Europe, Australia and New Zealand, which is 606 kg as reported by Appuhamy (2016). The calculated dDMI was used for further calculations of daily intake (kg d−1) of ADF (ADFi) and ADL (ADLi). Some models required the nutritive values that were not analyzed in this study (e.g., the daily metabolizable energy intake (MEi) in model no. 3 and dietary fatty acid (FA) in model no. 4 and no. 5). In this case, the MEi and FA were considered constants of 162 MJ d−1 and 28 g kg−1, respectively (Dalley et al., 1999; Ominski et al., 2006; Hegarty et al., 2007).
Table 4A summary of the published equations for predicting methane (CH4) production based on feedstock nutritive quality.
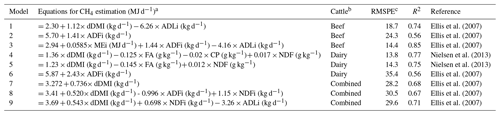
a dDMI (kg d−1): daily dry-matter intake, calculated by multiplying DMI (g kg−1) and the average body weight of a cow (606 kg, from Appuhamy, 2016); ADFi (kg d−1): daily acid detergent fiber intake; ADLi (kg d−1): daily acid detergent lignin intake; MEi (MJ d−1): daily metabolizable energy intake (∼ 162); FA (g kg−1): dietary fatty acid (∼ 28); CP (g kg−1): crude protein concentration of DM; NDF: neutral detergent fiber concentration of DM; NDFi (kg d−1): daily neutral detergent fiber intake. b Combined: beef + dairy. c RMSPE: root mean square prediction error reported by the original papers.
2.5 Statistical analysis
Treatment effects on biomass yield, feedstock chemical compositions, quality indices, and enteric CH4 productions were analyzed using the two-way repeated-measures analysis of variance (ANOVA) using the PROC MIXED procedure in SAS (SAS Institute, 2007). The harvest year (2017, 2018, and 2019), the cropping system (forage two-cut, forage one-cut, and bioenergy one-cut), and their interactions were considered fixed factors, while the replicates were considered random. The measurement year was used as the repeated factor, and each plot was considered a subject in the repeated measurement. The data normality and homogeneity were assessed by the model-predicted residuals using a Shapiro–Wilk test and equal-variance test to meet the ANOVA assumption. Since the feedstock chemical compositions are substantially influenced by harvest management (frequency coupled with timing), the summer- and fall-harvested biomass from the forage two-cut system were analyzed separately for comparing feedstock fiber compositions, quality indices, tissue nutrients, and CH4 production among other crop systems (i.e., forage two-cut (summer), forage two-cut (fall), forage one-cut, and bioenergy one-cut). Also, the first harvest year differed between the two crop systems, so the quality comparisons of the harvested biomass among crop systems only included forage systems in 2017–2019 but contained both forage and bioenergy systems in 2018–2019. Statistical mean differences among treatments were tested using the Tukey method at α=0.05.
3.1 General soil and weather information
The ANOVA test showed that the sampling location and depth significantly affected soil properties even though no location × depth interaction was shown in this study (data not shown). Compared to the west (B1) and central sides (B2) of the buffer strip, the soil in the east (B3) (Table 1) was more acidic and its fertility was lower by showing lower contents of total soil carbon (∼ 18.5 % decrease) and other essential nutrients. For instance, the contents of total phosphorus (TP), exchangeable Ca, and Mg were lower by approximately 7.0 %, 13.8 %, and 18.9 %, respectively. Soil electrical conductivity (EC) is used to measure the ability of soil water to conduct electricity, which is highly correlated with the soil nutrient concentrations (e.g., NH, K+, Na+, NO, SO, and Cl−) and often used as an alternative indicator for fertility. Although the average soil EC across the 0–100 cm profile was similar at three sampling locations, the averages across locations showed that the EC in topsoil (0–10 cm) was around 3–4 times higher than in deeper soil (< 10 cm). Monthly and cumulative precipitation during the study period (2016–2019) and their 30-year average (1990–2019) for this study site are shown in Fig. 2. The 30-year average indicated that the monthly precipitation in Illinois is well distributed throughout the year, with substantial amounts occurring in mid-spring (April) and early fall (September). Compared to the 30-year average, the 4-year average precipitation displayed a more variable pattern, except for the generally low precipitation in winter (December to February). The cumulative data indicated that the precipitation in 2019 was substantially higher than in other years and the 30-year average precipitation. During the growing season of perennial grasses (April to November), the cumulative precipitation in 2019 (909 mm) increased by approximately 11 % and 18.9 %, respectively, compared to the 4-year (820 mm) and 30-year (765 mm) averages. The pattern of monthly temperature during the experimental year followed the 30-year average data (Fig. 2c).
3.2 Biomass yield and nutrient removal
Two-way interaction between the year (2017–2019) and the cropping system (two-cut and one-cut forage and bioenergy crops) for biomass yield showed a significant yield reduction in forage systems but an increase in bioenergy crops (Fig. 3). From 2017 to 2019, biomass yield declined by approximately 30 % in the two-cut forage system (6.3 to 4.4 Mg DM ha−1 with a reduction rate of 1.0 Mg ha−1 yr−1) and 35 % in the one-cut forage system (4.9 to 3.2 Mg DM ha−1 with a rate of 0.9 Mg ha−1 yr−1). By contrast, the biomass yield of bioenergy feedstock increased by 27 % from 4.9 (2018) to 6.7 Mg DM ha−1 (2019). Based on the harvest frequency, averages across years showed that the two-cut forage system produced 33 % more biomass than the one-cut forage system (Fig. 3a). The year × cropping system interaction also influenced nutrient removals significantly (Fig. 3b). For both two-cut and one-cut forage, the maximum removal of N, P, K, Ca, and Mg occurred in the first harvest year (2017) and declined over time (Fig. 3b). Nutrient removal is a function of biomass productivity and biomass nutrient concentrations, and the decline in nutrient removal corresponded to the decreased biomass yield and nutrient concentrations in the forage system over the 3 years (Figs. 3 and 4). For instance, the total biomass N removal from the two-cut forage system reached 106.9 kg ha−1 in 2017 and reduced to 52.6 kg ha−1 in 2019 (∼ 50.8 % reduction); the one-cut forage system resulted in a total N removal of 84 kg ha−1 in 2017, which reduced to 44.5 kg ha−1 in 2019 (∼ 47 % reduction). For other nutrients, the P, K, Ca, and Mg removals from the two-cut forage system were 19.8, 132.7, 37.9, and 15.0 kg ha−1, respectively, in 2017 and reduced to 14.8, 93.7, 15.9, and 8.6 kg ha−1 in 2019; the one-cut forage system removed around 18.6 (P), 148.5 (K), 25.9 (Ca), and 12.4 kg ha−1 (Mg) in 2017, which reduced to 8.8, 55.7, 15.4, and 6.9 kg ha−1 in 2019. Although the overall results of forage systems showed the declining annual trend for yield production and nutrient removals (besides P), comparisons between 2018 and 2019 were insignificant. For the bioenergy system, the biomass nutrient removals were also similar in 2018 and 2019 for both primary and secondary nutrients (Fig. 3b).
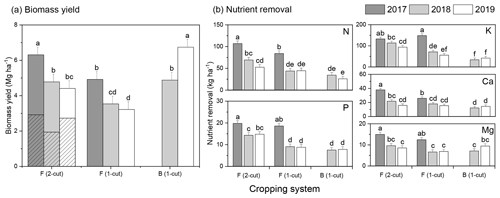
Figure 3Annual (a) biomass yields and (b) nutrient removal of forage and bioenergy feedstocks of the buffer strips, influenced by the harvest year × cropping system interaction (Y × CS) from 2017–2019. In the two-cut forage system, the summer- and fall-harvested biomass were combined to evaluate the annual biomass yield (the summer-harvested yield was indicated by the sparse pattern).
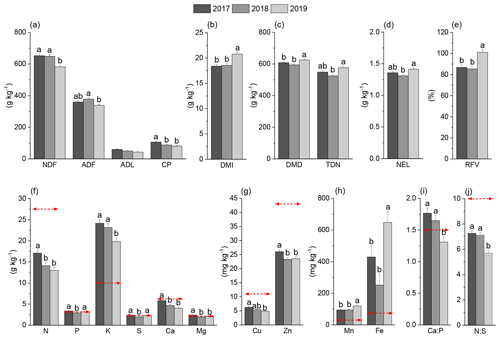
Figure 4The effect of cultivation year on the feedstock quality of the harvested forage crops (averages of the one-cut and two-cut systems) from 2017–2019. The lowercase letters indicate mean separation (α=0.05), and no mean separations were applied if the variable effect was not significant. The dashed red line indicated the critical level of minerals required by dairy cattle (NRC, 2001).
3.3 Feedstock chemical compositions and qualities
The ANOVA analysis showed that the effects of the harvest year; the cropping system; and their interactions on feedstock chemical compositions, nutrient concentrations, and quality indices (Table 5). Comparisons of these compositional analyses and indices among the forage two-cut summer harvest, two-cut fall harvest, and one-cut harvest were evaluated in 2017–2019 (forage biomass only), and these comparisons included the bioenergy one-cut harvest in 2018 and 2019 (forage and bioenergy biomass). For the forage biomass, both the harvest year (2017–2019) and year × cropping system (i.e., harvest management) interaction significantly influenced the concentrations of NDF, ADF, macronutrients, and feed-quality-related indices. Comparisons between forage and bioenergy systems in 2018–2019 showed substantial cropping system effects on all parameters except for Zn. For the forage biomass in 2017–2019, the effects of summer and fall harvest on the NDF and ADF concentrations were similar in the first 2 years. The NDF and ADF averages across 2017–2018 and three forage harvests were 650.6 ± 9.5 and 369.1 ± 10.7 g kg−1, respectively (Table 6). In 2019, the NDF and ADF of the two-cut forage system remained relatively constant under the summer harvest management but significantly decreased by approximately 15 % under the fall harvest management, similar to the one-cut forage system. The reduced NDF and ADF concentrations likely enhanced digestibility-related indices (Table 6), and the averages across three forage harvests showed that the 2019 fall-harvested biomass resulted in 12.5 %, 4.0 %, 7.3 %, 6.0 %, and 26.8 % higher DMI, DMD, TDN, NEL, and RFV, respectively, than the 2017–2018 averages (Fig. 4b–e). The forage CP concentrations also decreased over the years with a reduction rate of 12.9 g kg−1 yr−1 (Fig. 4a). The bioenergy biomass tended to have higher fiber contents than the forage biomass (Table 6). The averages of the bioenergy cellulose, hemicellulose, and lignin concentrations and the predicted TEY in 2018–2019 were 397.9, 299.4, and 66.5 g kg−1 DM and 361.0 L Mg−1, respectively, approximately 27.5 %, 16.7 %, 43.0 %, and 22.6 % higher than forage feedstock (Supplement Fig. S1). TTEY, the product of biomass yield and TEY, from the bioenergy system increased from 1.8 × 103 L ha−1 in 2018 to 2.4 × 103 L ha−1 in 2019 (∼ 33 % increase shown in Fig. 5). The TTEY from the forage systems, however, did not show significant differences between 2018 and 2019, even though the overall trend of the forage TTEY declined. From 2017–2019, the TTEY from the forage system declined by 34.2 % and 41.9 % in the two-cut and one-cut forage systems, respectively (Fig. 5).
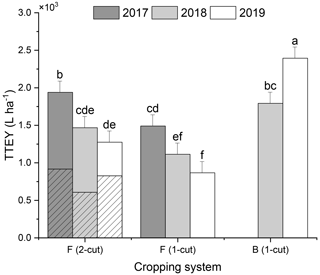
Figure 5The total theoretical ethanol yield (TTEY) of forage and bioenergy feedstocks, influenced by the harvest year × cropping system interaction (Y × CS) from 2017–2019. In the two-cut forage system, the summer- and fall-harvested biomass were combined to evaluate the annual biomass yield (the summer-harvested yield was indicated by the sparse pattern).
Table 5Analysis of variance (ANOVA) showed the effects of the main factors, including the year (Y); cropping system (CS); and interactions on biomass fiber analysis, quality indices, and the nutrient analysis of the forage and bioenergy feedstocks of the buffer strips with a significance level of 0.05.
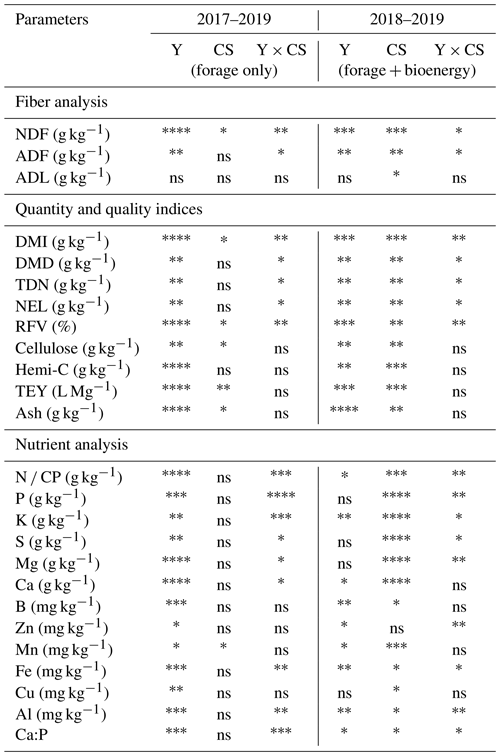
Level 1 (*): 0.05<p<0.01; level 2 (): 0.01<p<0.001; level 3 (): 0.001<p<0.0001; level 4 (): p<0.0001; ns: not significant.
Table 6Fiber analysis and the derived forage quality indices of the harvested biomass, influenced by the year × cropping system interaction (Y × CS) from 2017 to 2019. The superscript lowercase letters indicate the mean separation (α=0.05) of the forage crops collected from 2017–2019. The superscript uppercase letters indicate the mean separation of both forage and bioenergy crops collected from 2018 and 2019 (no mean separations were applied if the variable effect was not significant).
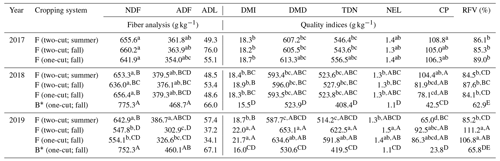
F (two-cut; summer): forage crop with two-harvest management (first harvest in summer). F (two-cut; fall): forage crop with two-harvest management (second harvest in fall). F (one-cut; fall): forage crop with one-harvest management in fall. B (one-cut; fall): bioenergy crop with one-harvest management in fall. * Bioenergy crops were not harvested in 2017 for biomass nutrient analysis due to insufficient biomass production.
3.4 Nutrient analysis
For nutrient analysis, even though the ANOVA test (Table 5) showed a significant interaction effect between the year and cropping systems on macronutrients, no consistent pattern was observed (Supplement Table S1). Averages across three forage harvests showed that most of the macronutrients likely showed the highest concentration in the first harvest year of 2017 (Fig. 4). From 2017 to 2019, the N, K, Ca, and Mg concentrations decreased by approximately 24.1 %, 17.9 %, 30.9 %, and 16.3 %, respectively (Fig. 4f). Both forage N and Ca concentrations were below the threshold of the recommended levels for dairy cows, approximately 27.5 g N kg−1 DM and 6.2 g Ca kg−1 DM shown with the dashed red line (NRC, 2001). For micronutrients, a similar declining trend of the concentrations was observed for Cu and Zn, reduced by around 22.1 % and 10.0 %, respectively; by contrast, the concentrations of Mn and Fe increased by 25.9 % and 51.0 %, respectively, from 2017 to 2019 (Fig. 4g and h). Both Cu and Zn were lower than the recommended nutrient levels of 11 and 43 mg kg−1 DM. For nutrient ratios, Ca : P and N : S decreased substantially from 1.76 to 1.31 and 7.27 to 5.72, respectively, in the third harvest year, and both ratios were lower than the recommendation levels (the dashed red lines shown in Fig. 4i and j). In 2018 and 2019, the nutrient concentrations of the bioenergy feedstock were not significantly different besides Zn. The averages across 2 years indicated that the nutrient concentrations of the bioenergy feedstock were generally lower than the forage feedstock by approximately 29 %–69 % and 32 %–80 % for macro- and micro-nutrients, respectively (Supplement Table S1).
3.5 Methane production
The enteric CH4 production was only predicted from forage crops using nine models, and the box plots were shown in Fig. 6. The averages of the modeled CH4 ranged from 207.8 to 380.5 g per cow per day with the standard deviation (SD) from 4.9 to 31.9 g per cow per day (Fig. 6). The M1–M3 models, trained by the dataset of beef cows, resulted in the CH4 average of 234.8 ± 42.1 g per cow per day with a coefficient of variation (CV) of 18 %. The M4–M6 models, trained by the dataset of dairy cattle, led to a higher CH4 average of 329.1 ± 40.3 g per cow per day but a lower variation (CV = 12 %) than the M1–M3 predictions. Increases in the M4–M6 average were driven by the highest estimation (380.5 ± 11.2 g per cow per day) using the M4 model, based on the dDMI, CP, and NDF predictors, among other models. The increased variation in the M1–M3 predictions, on the other hand, was due to the highest variation (CV = 17 %) from the M1 model, based on the dDMI and ADLi predictors. The CH4 predictions using the M7–M9 models, built based on the integrated dataset of beef and dairy cows, resulted in an average of 231.9 ± 19.1 g per cow per day with the lowest variation (CV = 8 %). For the individual model, the two-way ANOVA showed that most of the CH4 predictions were sensitive to variation by year, but only the M5-, M7-, and M8-predicted CH4 significantly responded to the harvest management and their interaction (Supplement Table S2). The averages from each category (e.g., M1–M3 average from the beef category) showed that the modeled CH4 increased over the years at rates of 11.0 (beef), 8.1 (dairy), and 8.4 g per cow per day (combined), respectively (Table 7). No significant year × forage system interaction was observed for the CH4 predicted from beef and dairy categories. The interaction effect was only shown in the “combined” category (p value = 0.0074). In the combined category, the modeled CH4 remained stable (i.e., 221.1–228.7 g per cow per year) in the first 2 harvest years of 2017 and 2018 but increased by approximately 10.8 % under the fall harvest management in 2019 (i.e., 250.9 g per cow per day) compared to the 2017–2018 average (i.e., 226.4 g per cow per day).
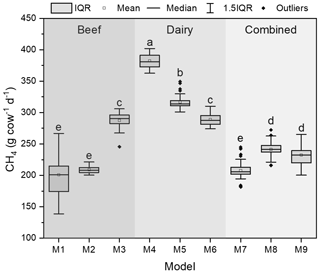
Figure 6Box plots of the predicted methane (CH4) production based on forage nutritive quality using different prediction models (M1–M9). The predicted CH4 of forage feedstock was compared using the one-way ANOVA with a significance level of 0.05. The lowercase letters indicate mean separation organized from the highest to the lowest value for each column using the Tukey test. IQR: interquartile range.
4.1 Biomass yield and nutrient removal
This study evaluated the feasibility of utilizing forage (perennial cool-season grass mixtures) and bioenergy (warm-season grass mixtures) systems for establishing a sustainable buffer strip (Table 2). For the forage buffer, Kelly et al. (2007) reported that the monoculture SB and AL produced annual biomass yields ranging from 5–6 and 5–7 Mg ha−1, respectively. The polyculture forage systems (PR–clover or SB–TG–Kentucky bluegrass mixtures) established in riparian areas produced annual yields from 2 to 10 Mg ha−1, and the yields of grass mixtures cultivated on other marginal lands (e.g., on the CRP-registered lands) were 2.8 Mg ha−1 (the AL–pubescent wheatgrass mixture in Montana), 3.4 (the TF–OR mixture in Georgia), and 4.2 (the red-clover–TF mixture in Missouri), respectively (Anderson er al., 2016; Christen et al., 2013; Lee et al., 2013; Tufekcioglu et al., 2003). For the bioenergy buffer, the monoculture SW showed a range of annual yield from 4 to 13 Mg ha−1, and the grass mixtures (mainly SW, PC, BB, and IN) ranged from 2.8 to 10.7 Mg ha−1 (Cooney et al., 2023; Ferrarini et al., 2017; Gamble et al., 2016; Gopalakrishnan et al., 2012; Kelly et al., 2007; Tufekcioglu et al., 2003; Zamora et al., 2013). This yield variation was likely due to the confounding effects of species, growth environment, and management practices (Cooney et al., 2023). Thus, the perennial buffers in this study showed their economic potential by producing substantial biomass yield (forage: 3.2–6.3 Mg ha−1; bioenergy yield: 4.9–6.7 Mg ha−1), especially under no fertilizer application.
Compared to the bioenergy system, greater nutrient concentrations in forage biomass (Supplement Table S1) indicated that forage-type grasses have higher nutrient demand (Kering et al., 2012; Pedroso et al., 2014). The increased nutrient requirement also implied that cool-season forages require more available soil nutrients (e.g., high-N fertilization) to produce amounts of dry biomass similar to warm-season bioenergy grasses, also suggesting that more nutrients are likely removed from soils by cool-season forage grasses (Minson, 1981; Mullahey et al., 1992; Follett and Wilkinson, 1995; Kering et al., 2012; Pedroso et al., 2014). In this study, the higher nutrient removal of the cool-season grasses resulted in the possible depletion of soil nutrients over time and the declined soil nutrients presumably accounted for a gradual decline in biomass yield in the forage system (approximate reduction of 0.9 Mg ha−1 yr−1), even though the yearly changes in soil fertility were not assessed. From 2017 to 2019, decreases in biomass N, K, Ca, and Mg concentrations implied that soil nutrients, including the nutrient transported from the main C–S field via runoff, might be inadequate to fully support the growth of the cool-season grasses in the forage system. Harvest management (frequency and timing) also influences biomass yield and crop nutrient removal. Our results support previous findings in which total annual biomass yield and nutrient removal are usually increased by multiple cuttings in the forage system (Schultz et al., 1995; Fike et al., 2006b; Mitchell and Schmer, 2012). Harvest timing also plays a critical role in yield sustainment and the “regrowth vigor” potential, which influences long-term buffer strip production and sustainability (Mulkey et al., 2006; Mitchell et al., 2014). In this study, the harvest was delayed to the end of the growing season for both forage systems and until after a killing frost for the bioenergy system. This approach aimed to improve feedstock stand longevity by providing extended time for vegetative development and reproductive tiller growth and to translocate nutrients to underground crop tissues that can be recycled for use in the following year (MacAdam and Nelson, 2003; Lee et al., 2014; Zumpf et al., 2019).
Table 7The model-predicted CH4 productions of the forage feedstock, influenced by the year × cropping system interaction (Y × CS) from 2017–2019. The superscript lowercase letters indicate the mean separation (α=0.05) of the forage crops collected from 2017–2019 (no mean separations were applied if the variable effect was not significant).
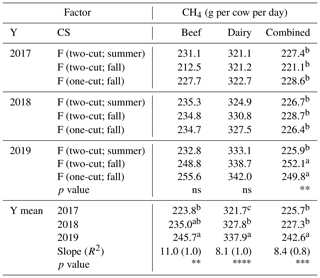
Level 1 (*): 0.05<p<0.01; level 2 (): 0.01<p<0.001; level 3 (): 0.001<p<0.0001; level 4 (): p<0.0001; ns: not significant.
Compared to the forage systems in which biomass yield and nutrient concentrations declined over time, the bioenergy system showed an increased yield potential under continuously unfertilized conditions (Fig. 3). There was no biomass harvest in the bioenergy system in 2017 due to the reestablishment that year. Therefore, the first harvest year for the bioenergy biomass was in 2018. Based on the harvest year, the side-by-side comparisons showed that the two-cut forage system produced a higher amount of biomass than the bioenergy system in the first harvest year, but its biomass yield declined in the following years; conversely, the bioenergy system showed the highest yield potential in the second harvest year for any system (Fig. 3a). Although the bioenergy system was only harvested for 2 successive years in our case, many studies report that warm-season grasses have high nutrient use efficiency and consistent biomass production across years (Brown, 1978; Sage et al., 1987; Ghannoum et al., 2011; Sage and Zhu, 2011; van der Weijde et al., 2013; Pedroso et al., 2014; Lee et al., 2018). For example, Lee et al. (2018) showed that maximum bioenergy feedstock biomass yield occurred in the third year after the establishment (∼ 2 times more than in the establishment year) and the stable yield production can be continuous for up to 7 years. For scavenging nutrients leaching/runoff from the C–S field, however, a cool-season grass-based forage system can be considered an ideal short-term candidate for riparian-zone filter strips by showing more effective erosion control, sediment trapping, and nutrient removal than a warm-season grass-based bioenergy system (Lynn, 2004). Based on the first two harvest events (2017 and 2018 in the forage system and 2018 and 2019 in the bioenergy system), the two-cut and one-cut forage systems resulted in total N removal of 176.2 and 128.0 kg ha−1, respectively, from the harvested biomass, while only 60.3 kg ha−1 was removed from the bioenergy system (Fig. 3b). These substantial N removals from the forage system mainly resulted from significantly high yield and biomass nutrient concentrations in the first harvest year (2017). On the other hand, the bioenergy system can be considered for long-term nutrient loss reduction plans as it produced consistently high biomass.
4.2 Feedstock quality
Biomass quality characteristics are defined by its use as livestock feed or biofuel feedstock. As forage feedstocks, biomass CP and crude fibers (NDF and ADF) are critical factors for animal performance and quality, such as livestock weight or milk production (Assefa and Ledin, 2001; Collins and Fritz, 2003). The biomass NDF and ADF can be used to evaluate the digestibility, palatability, and energy level of animal feeds based on their predictions of DMD, DMI, TDN, NEL, and RFV indices (Guretzky et al., 2011). For example, a higher NDF generally lowers the ingestion of dry matter (DMI), and a higher ADF likely reduces the overall digestibility (DMD), digestible nutrients (TDN), and energy level (NEL) of forage feeds for animals and lowers forage qualities (Collins and Fritz, 2003). Delayed harvest usually reduced biomass CP and increased fiber concentrations resulting from the N translocation to belowground rhizomes; however, no significant harvest effects on CP or crude fibers (NDF and ADF) were shown in this study (Table 6), presumably due to a substantial environmental impact. In polyculture systems, the response of species compositions to management practices, along with the biomass yield and chemical compositions, was significantly modulated by environmental variations such as weather and soil conditions (Cooney et al., 2023; Hong et al., 2014; Lin et al., 2023). In this study, the interaction between highly erodible zones and fluctuating precipitation levels likely intensified the complexities of the growth environment (e.g., inconsistent nutrient input via leaching and runoff), thereby diminishing the discernibility of experimental treatment effects. Without direct N fertilizer replenishment, the 3 years of continuous crop harvests resulted in decreases in both biomass yield and CP content in the forage systems (Figs. 3 and 4a). The forage NDF also gradually reduced with increasing N depletion over the years (Fig. 4), even though several studies showed that the cell wall compositions were not influenced by different N input and soil N contents (Liu et al., 2015; Ameen et al., 2019). Several abiotic (e.g., severe drought, salinity, heat) or biotic (diseases or pests) stresses might damage cell structure, inhibit crop growth, stunt tissue development, and reduce structural cell wall compositions (Hoover et al., 2018; Fan et al., 2020). Thus, the abrupt NDF reduction (∼ 10.8 %) in 2019 presumably resulted from the harsh environmental stresses (i.e., the severe leaching and nutrient depletion resulting from intense precipitation in 2019; Fig. 2).
Mineral levels in forage are also essential for both livestock health and performance and the effectiveness of ruminal microorganisms for fiber digestion. The mineral concentrations in biomass usually declined with advancing maturity (Fleming, 1973); however, this study did not observe the reduced mineral content in forage feedstock for two-harvest timing (at the anthesis in summer (June) vs. after complete senescence in fall (October/November)), possibly resulting from a substantial environmental effect. Besides the N content, Ca and P deficiency are often considered critical among other macronutrients because of their roles in the development of skeletal structure, metabolism, and milk production. Under the continuously unfertilized management, the forage P concentrations (average across forage harvests) remained fairly adequate for cattle requirements (∼ 3.2 g kg−1 shown in NRC, 2001). The continuously declining Ca concentrations, however, showed the feed Ca content was insufficient for a dairy cow's daily diet (minimum requirement of ∼ 6.2 g kg−1 DM; NRC, 2001). A long-term Ca deficiency in animal diet could result in abnormalities of bond development and the depression of milk yield (McDowell, 2003; Suttle, 2022). The potassium, as the primary intracellular cation, recommendation for dairy cows ranges from 6 to 12 g kg−1 DM, and its deficiency is rarely observed in forage biomass given the high K content in grasses and legumes (Marijanušić et al., 2017). Although excess dietary K uptake (e.g., 20–24 g kg−1 DM in this study) could interfere with Ca homeostasis, K toxicity is rare in cattle because dairy cattle showed a great ability to easily excrete excessive K intake (NRC, 2005). Most of the micronutrients act as critical components of metalloenzymes or metalloproteins, significantly related to the metabolic function, immune system, and antioxidant status of the livestock (Suttle, 2022). The Cu, Zn, and Fe deficiency in ruminant grazing forages is a widespread issue in many areas of the world and often necessitates supplementation (McDowell, 2003; Spears, 1994; Marijanušić et al., 2017). This study also showed the deficiency of Cu and Zn in forages but not that of Fe. Both Cu and Zn concentrations were below the critical levels by approximately 50 % and 43 %, respectively. Furthermore, appropriate nutrient ratios are as critical as individual elements for animal growth and performance. For instance, the Ca : P ratio is important for supporting appropriate bone development (especially for young, growing animals) and milk production of lactating cows. The recommended ideal Ca : P ratio ranges from 1.5:1 to 2.0:1 for dairy cows, but the ratio of the third-year forage (average across forage systems) was below this threshold due to substantial decreases in the tissue Ca concentration (Fig. 4i). The N : S ratio is another important factor for ruminant microbial protein synthesis and S-containing amino acid production (NRC, 2005). The N : S ratio in this study (5.7–7.3) was substantially below the recommended ratio ranging from 10:1 to 12:1.
The dietary composition for cattle typically includes forage (e.g., hay, silage, and pasture) and concentrates (e.g., grains, protein sources, and other energy-dense feeds), supplemented with minerals and vitamins. The ratio of forage to concentrate ranged from 45:55 to 70:30 based on the animal type, sex, target weight, and developmental stage (Aguerre et al., 2011; Briggs and Felix, 2021). For instance, forage constitutes approximately 50 %–70 % of DMI to support proper rumen development during the growth phase, while in the finishing stage, forage usually reduced to about 30 %–40 % to facilitate weight gain (Chen et al., 2015; Mialon et al., 2008). Although these results did not have conclusive impacts on the overall diet quality of cattle, the gradual reductions in fiber (i.e., NDF) and nutrient (i.e., CP, Ca, Cu, Zn) concentrations and the nutrient ratio (i.e., Ca : P and N : S) provided valuable data for tailoring the ratio of forage to concentrate to meet specific nutritional requirements and deficiencies in cattle. Additionally, an increasing number of cattle nutritionists and producers are adopting least-cost feed formulation strategies, which consider the total costs associated with diet mixing and daily feeding (Briggs and Felix, 2021). The minimal management effort applied in this study likely met with low-cost strategies for animal feed.
As bioenergy feedstocks, the increased cell wall contents often observed in warm-season grasses are generally considered indications of desirable biofuel quality, especially the structural carbohydrates of cellulose and hemicellulose for producing bioethanol via the biochemical (i.e., fermentation) process (Li et al., 2016). Previous studies (Hong et al., 2013; Guo et al., 2017; Ameen et al., 2019) showed that the cellulose concentrations of the monocultures of big bluestem (BB), Indiangrass (IND), switchgrass (SW), and prairie cordgrass (PC) were in ranges of 378–420, 380–451, 360–401, and 400–421 g kg−1, respectively. The polyculture system of BB, IND, and SW mixtures showed a similar cellulose range from 360 to 425 g kg−1. For hemicellulose, the concentration ranges were 308–310 (BB), 278–315 (IND), 310–325 (SW), and 293–312 g kg−1 (PC), respectively, and grass mixtures were also within a similar range (300–315 g kg−1). This study showed that the grass-mixture bioenergy buffer can also offer feedstock with reasonable qualities (i.e., a 2-year average of cellulose of ∼ 397.9 g kg−1 and hemicellulose of ∼ 299.4 g kg−1) and a great potential for increasing ethanol yield productions based on the increased TTEY of 1.8 × 103 (2018) to 2.4 × 103 L ha−1 (2019).
4.3 Environmental impact
The forage nutritive values not only influence cattle performance but also highly correlate to the CH4 production in the rumen. For instance, the increased feed quality likely increases feed consumption, usually expressed by DMI, and the increased DMI further accelerates cattle CH4 emissions. Instead of direct CH4 measurements, several empirical models have been used for the CH4 predictions based on DMI, which is the most often used predictor, and other attributes, such as CP, NDF, ADF, and ADL (Ellis et al., 2007; Storlien et al., 2014; Appuhamy et al., 2016). Although the DMI of individual cows was not routinely measured due to the limited budget in commercial farms, the estimated DMI can also predict CH4 emissions with a performance as good as the measured DMI using suitable models (Appuhamy et al., 2016). Enteric CH4 emissions are highly variable among ruminants. Generally speaking, the species with heavier body weight tend to consume more feed and produce more CH4 via feed fermentation (e.g., cattle > goat or sheep). For example, the average body weight of beef cattle was approximately 450 kg per cow with a daily DMI (dDMI) of 8.0 kg in North America, and the CH4 emissions ranged from 50 to 250 g per cow per day. The average weight of dairy cattle was around 644 kg, with a dDMI of 21 kg, producing CH4 of 200 to 600 g per cow per day (Ellis et al., 2007; Appuhamy et al., 2016; Hales et al., 2022). This study also showed a similar range of the CH4 estimations, and the predicted CH4 emissions increased with advancing DMI in the third harvest year. Furthermore, it is possibly misguiding for farmers to optimize livestock management practices based on a simple judgment of the overall CH4 emissions. For instance, although the overall CH4 emissions of the dairy cows were higher (128 kg per cow per year) in North America than in the European Union (117 kg per cow per year) and Oceania (99 kg per cow per year), the higher milk yield led to lower emission intensity in North America (FAO, 2014). Since livestock production is a consequence of the overall feed quality, which needs to consider both digestibility (e.g., DMD) and nutrient levels (e.g., CP and minerals), the overall high-quality forage likely increases animal production as well as mitigating CH4 emission intensity (Lee et al., 2017).
Perennial grass mixtures are ideal polyculture systems for building productive and sustainable buffer stripes. The cool-season forage and warm-season bioenergy grasses showed different strengths as buffers by serving specific purposes. The forage-type buffer can be an ideal short-term candidate for riparian areas with high leaching potential due to its great efficiency of nutrient scavenging, which can be further improved under multiple-harvest management. The high nutrient demand of forage crops, however, likely compromised buffer sustainability under the successive nutrient starvation condition. From a quality perspective, the successively harvested forage buffer without nutrient input through fertilizer application was incapable of providing livestock with adequate nutritive values, especially yearly reductions in crucial protein and major mineral contents (i.e., Ca, Cu, Zn), even though the forage digestibility seemed to increase in the third harvest year. Without any forage/concentrate adjustments, the overall low-quality feed likely lower ruminant performance and possibly aggravates the impact of enteric CH4 emissions on the global greenhouse gas burden. On the other hand, although this is a short-term study, the bioenergy-type buffer showed better sustainability than the forage buffer and a potential for continuous and stable yield supply based on our previous and other long-term studies, which could provide local stakeholders with a long-term opportunity for offering extra economic benefits and ecosystem services simultaneously.
Data can be directly obtained by contacting the lead author.
The supplement related to this article is available online at: https://doi.org/10.5194/bg-21-4765-2024-supplement.
Conceptualization: CHL, CZ, CJ, TV, GT, OO, AC, and DKL. Data curation: CHL and DKL. Formal analysis: CHL, CZ, and RM. Funding acquisition: GT and DKL. Investigation: GT and DKL. Methodology: CHL, CZ, TV, GT, and DKL. Project administration: GT and DKL. Resources: GT and DKL. Software: CHL. Supervision: GT and DKL. Validation: CHL, CZ, CJ, RM, and DKL. Visualization: CHL. Writing (original draft): CHL. Writing (review and editing): CZ, CJ, TV, RM, and DKL. All authors have read and agreed to the published version of the paper.
The contact author has declared that none of the authors has any competing interests.
This research was funded by the Metropolitan Water Reclamation District (MWRD) of Greater Chicago. Any opinions, findings, and recommendations expressed in this paper are those of the author(s) and do not necessarily reflect the views of the MWRD of Greater Chicago. The authors do not have any other relevant affiliations or financial involvement with any organization with a financial interest in or financial conflict with the subject matter or materials discussed in the paper apart from those disclosed.
Publisher’s note: Copernicus Publications remains neutral with regard to jurisdictional claims made in the text, published maps, institutional affiliations, or any other geographical representation in this paper. While Copernicus Publications makes every effort to include appropriate place names, the final responsibility lies with the authors.
The authors appreciate the crop and field management from Jacob Baylor, Hyemi Kim, Danielle Cooney, Jia Guo, Moonsub Lee, and Soohyun Lim, as well as technical assistance from the Soils laboratory of MWRD.
This research has been supported the MWRD of Greater Chicago.
This paper was edited by Anja Rammig and reviewed by two anonymous referees.
Aguerre, M. J., Wattiaux, M. A., Powell, J. M., Broderick, G. A., and Arndt, C.: Effect of forage-to-concentrate ratio in dairy cow diets on emission of methane, carbon dioxide, and ammonia, lactation performance, and manure excretion, J. Dairy Sci., 94, 3081–3093, https://doi.org/10.3168/jds.2010-4011, 2011.
Ameen, A., Tang, C., Liu, J., Han, L., and Xie, G. H.: Switchgrass as forage and biofuel feedstock: Effect of nitrogen fertilization rate on the quality of biomass harvested in late summer and early fall, Field Crop. Res., 235, 154–162, https://doi.org/10.1016/j.fcr.2019.03.009, 2019.
Anderson, E. K., Aberle, E., Chen, C., Egenolf, J., Harmoney, K., Kakani, V. G., Kallenbach, R., Khanna, M., Wang, W., and Lee, D.: Impacts of management practices on bioenergy feedstock yield and economic feasibility on Conservation Reserve Program grasslands, GCB Bioenergy, 8, 1178–1190, https://doi.org/10.1111/gcbb.12328, 2016.
Appuhamy, J., France, J., and Kebreab, E.: Models for predicting enteric methane emissions from dairy cows in North America, Europe, and Australia and New Zealand, Glob. Change Biol., 22, 3039–3056, https://doi.org/10.1111/gcb.13339, 2016.
Assefa, G. and Ledin, I.: Effect of variety, soil type and fertiliser on the establishment, growth, forage yield, quality and voluntary intake by cattle of oats and vetches cultivated in pure stands and mixtures, Anim. Feed Sci. Technol., 92, 95–111, https://doi.org/10.1016/S0377-8401(01)00242-5, 2001.
Bélanger, G., Castonguay, Y., Bertrand, A., Dhont, C., Rochette, P., Couture, L., Drapeau, R., Mongrain, D., Chalifour, F.-P., and Michaud, R.: Winter damage to perennial forage crops in eastern Canada: Causes, mitigation, and prediction, Can. J. Plant Sci., 86, 33–47, https://doi.org/10.4141/p04-171, 2006.
Ball, D. M., Collins, M., Lacefield, G. D., Martin, N. P., Mertens, D. A., Olson K. E., Putnam, D. H., Undersander, D. J., and Wolf, M. W.: Understanding forage quality, American Farm Bureau Federation Publication 1-01, Park Ridge, IL, 21 pp., https://fyi.extension.wisc.edu/forage/files/2017/04/FQ.pdf (last access: 14 May 2024), 2017.
Briggs, N. and Felix, T. L.: Ration formulation for growing cattle, Code EE0603, Penn State Extension, https://extension.psu.edu/ration-formulation-for-growing-cattle (last access: 16 May 2024), 2021.
Brown, R. H.: A Difference in N Use Efficiency in C3 and C4 Plants and its Implications in Adaptation and Evolution, Crop Sci., 18, 93–98, https://doi.org/10.2135/cropsci1978.0011183X001800010025x, 1978.
Carlsson, G., Martensson, L. M., Prade, T., Svensson, S. E., and Jensen, E. S.: Perennial species mixtures for multifunctional production of biomass on marginal land, GCB Bioenergy, 9, 191–201, https://doi.org/10.1111/gcbb.12373, 2017.
Chen, G. J., Song, S. D., Wang, B. X., Zhang, Z. F., Peng, Z. L., Guo, C. H., Zhong, J. C., and Wang, Y.: Effects of forage:concentrate ratio on growth performance, ruminal fermentation and blood metabolites in housing-feeding yaks, Asian-Austr. J. Anim. Sci., 28, 1736–1741, https://doi.org/10.5713/ajas.15.0419, 2015.
Christen, B. and Dalgaard, T.: Buffers for biomass production in temperate European agriculture: A review and synthesis on function, ecosystem services and implementation, Biomass Bioenerg., 55, 53–67, https://doi.org/10.1016/j.biombioe.2012.09.053, 2013.
Clausen, J. C., Guillard, K., Sigmund, C. M., and Dors, K. M.: Ecosystem restoration – water quality changes from riparian buffer restoration in Connecticut, J. Environ. Qual., 29, 1751–1761, https://doi.org/10.2134/jeq2000.00472425002900060004x, 2000.
Collins, M. and Fritz, J. O.: Forage quality, in: Forages: An introduction to grassland agriculture, edited by: Barnes, R. F., Nelson, C. J., Collins, M., and Moore, K. J., 6th Edn., Iowa State Press, Ames, IA, Wiley-Blackwell, 363–390, ISBN: 978-0813804217, 2003.
Cooney, D. R., Namoi, N., Zumpf, C., Lim, S.-H., Villamil, M., Mitchell, R., and Lee, D. K.: Biomass production and nutrient removal by perennial energy grasses produced on a wet marginal land, BioEnergy Res., 16, 886–897, https://doi.org/10.1007/s12155-022-10488-0, 2023.
Dalley, D. E., Roche, J. R., Grainger, C., and Moate, P. J.: Dry matter intake, nutrient selection and milk production of dairy cows grazing rainfed perennial pastures at different herbage allowances in spring, Aust. J. Exp. Agric., 39, 923–931, https://doi.org/10.1071/ea99022, 1999.
David, M. B., Drinkwater, L. E., and McIsaac, G. F.: Sources of nitrate yields in the mississippi river basin, J. Environ. Qual., 39, 1657–1667, https://doi.org/10.2134/jeq2010.0115, 2010.
De Deyn, G. B., Shiel, R. S., Ostle, N. J., McNamara, N. P., Oakley, S., Young, I., Freeman, C., Fenner, N., Quirk, H., and Bardgett, R. D.: Additional carbon sequestration benefits of grassland diversity restoration, J. Appl. Ecol., 48, 600–608, https://doi.org/10.1111/j.1365-2664.2010.01925.x, 2011.
Dhakal, D. and Islam, M. A.: Grass-legume mixtures for improved soil health in cultivated agroecosystem, Sustainability, 10, 2718, https://doi.org/10.3390/su10082718, 2018.
Dodds, W. K. and Oakes, R. M.: Headwater Influences on Downstream Water Quality, Environ. Manag., 41, 367–377, https://doi.org/10.1007/s00267-007-9033-y, 2008.
Dosskey, M. G.: Toward Quantifying Water Pollution Abatement in Response to Installing Buffers on Crop Land, Environ. Manag., 28, 577–598, https://doi.org/10.1007/s002670010245, 2001.
Ellis, J. L., Kebreab, E., Odongo, N. E., McBride, B. W., Okine, E. K., and France, J.: Prediction of methane production from dairy and beef cattle, J. Dairy Sci., 90, 3456–3466, https://doi.org/10.3168/jds.2006-675, 2007.
Eranki, P. L., Manowitz, D. H., Bals, B. D., Izaurralde, R. C., Kim, S., and Dale, B. E.: The watershed-scale optimized and rearranged landscape design (WORLD) model and local biomass processing depots for sustainable biofuel production: Integrated life cycle assessments, Biofuels, Bioprod. Bioref., 7, 537–550, https://doi.org/10.1002/bbb.1426, 2013.
FAO: FAO Statistical Yearbook, Food and Agriculture Organization of the United Nations, Rome, Italy, FAO, ISBN: 978-92-5-138262-2, 2014.
Fan, J. B., Zhang, W. H., Amombo, E., Hu, L. X., Kjorven, J. O., and Chen, L.: Mechanisms of environmental stress tolerance in turfgrass, Agronomy-Basel, 10, 522, https://doi.org/10.3390/agronomy10040522, 2020.
Ferrarini, A., Serra, P., Almagro, M., Trevisan, M., and Amaducci, S.: Multiple ecosystem services provision and biomass logistics management in bioenergy buffers: A state-of-the-art review, Renew. Sust. Energ. Rev., 73, 277–290, https://doi.org/10.1016/j.rser.2017.01.052, 2017.
Fike, J. H., Parrish, D. J., Wolf, D. D., Balasko, J. A., Green, J. T., Rasnake, M., and Reynolds, J. H.: Long-term yield potential of switchgrass-for-biofuel systems, Biomass Bioenerg., 30, 198–206, https://doi.org/10.1016/j.biombioe.2005.10.006, 2006a.
Fike, J. H., Parrish, D. J., Wolf, D. D., Balasko, J. A., Green, J. T., Rasnake, M., and Reynolds, J. H.: Switchgrass production for the upper southeastern USA: Influence of cultivar and cutting frequency on biomass yields, Biomass Bioenerg., 30, 207–213, https://doi.org/10.1016/j.biombioe.2005.10.008, 2006b.
Fleming, G. A.: Mineral composition of herbage, in: Chemistry and biochemistry of herbage, edited by: Butler, G. W. and Bailey, R. W., Academic Press, New York, 529–566, ISBN: 0121481018, 9780121481018, 1973.
Follett, R. F. and Wilkinson, S. R.: Nutrient management of forages, in: Forages, edited by: Barnes, R. F., Miller, D. A., and Nelson, C. J., Vol. II, The Science of Grassland Agriculture, , Ames, Iowa State Univ. Press, 55–82, ISBN: 9780813806839, 1995.
Gamble, J. D., Johnson, G., Current, D. A., Wyse, D. L., and Sheaffer, C. C.: Species pairing and edge effects on biomass yield and nutrient uptake in perennial alley cropping systems, Agron. J., 108, 1020–1029, https://doi.org/10.2134/agronj2015.0456, 2016.
Ghannoum, O., Evans, J. R., and von Caemmerer, S.: Chapter 8 Nitrogen and Water Use Efficiency of C4 Plants, in: C4 Photosynthesis and Related CO2 Concentrating Mechanisms, edited by: Raghavendra, A. S. and Sage, R. F., 129–146, Dordrecht, Springer Netherlands, https://doi.org/10.1007/978-90-481-9407-0_8, 2011.
Golkowska, K., Rugani, B., Koster, D., and Van Oers, C.: Environmental and economic assessment of biomass sourcing from extensively cultivated buffer strips along water bodies, Environ. Sci. Policy, 57, 31–39, https://doi.org/10.1016/j.envsci.2015.11.014, 2016.
Gopalakrishnan, G., Negri, M. C., and Salas, W.: Modeling biogeochemical impacts of bioenergy buffers with perennial grasses for a row-crop field in Illinois, GCB Bioenergy, 4, 739–750, https://doi.org/10.1111/j.1757-1707.2011.01145.x, 2012.
Guo, J., Thapa, S., Voigt, T., Owens, V., Boe, A., and Lee, D. K.: Biomass yield and feedstock quality of prairie cordgrass in response to seeding rate, row spacing, and nitrogen fertilization, Agron. J., 109, 2474–2485, https://doi.org/10.2134/agronj2017.03.0179, 2017.
Guretzky, J. A., Biermacher, J. T., Cook, B. J., Kering, M. K., and Mosali, J.: Switchgrass for forage and bioenergy: harvest and nitrogen rate effects on biomass yields and nutrient composition, Plant Soil, 339, 69–81, https://doi.org/10.1007/s11104-010-0376-4, 2011.
Hales, K. E., Coppin, C. A., Smith, Z. K., McDaniel, Z. S., Tedeschi, L. O., Cole, N. A., and Galyean, M. L.: Predicting metabolizable energy from digestible energy for growing and finishing beef cattle and relationships to the prediction of methane, J. Anim. Sci., 100, skac013, https://doi.org/10.1093/jas/skac013, 2022.
Harmoney, K. R., Lee, D. K., Kallenbach, R. L., and Aberle, E. Z.: Species composition changes in conservation reserve program (crp) grassland when managed for biomass feedstock production, BioEnergy Res., 9, 1180–1188, https://doi.org/10.1007/s12155-016-9764-9, 2016.
Hegarty, R. S., Goopy, J. P., Herd, R. M., and McCorkell, B.: Cattle selected for lower residual feed intake have reduced daily methane production, J. Anim. Sci., 85, 1479–1486, https://doi.org/10.2527/jas.2006-236, 2007.
Hodgson, E. M., Fahmi, R., Yates, N., Barraclough, T., Shield, I., Allison, G., Bridgwater, A. V., and Donnison, I. S.: Miscanthus as a feedstock for fast-pyrolysis: Does agronomic treatment affect quality?, Bioresour. Technol., 101, 6185–6191, https://doi.org/10.1016/j.biortech.2010.03.024, 2010.
Hong, C. O., Owens, V. N., Lee, D. K., and Boe, A.: Switchgrass, big bluestem, and indiangrass monocultures and their two- and three-way mixtures for bioenergy in the northern great plains, BioEnergy Res., 6, 229–239, https://doi.org/10.1007/s12155-012-9252-9, 2013.
Hong, C. O., Owens, V. N., Bransby, D., Farris, R., Fike, J., Heaton, E., Kim, S., Mayton, H., Mitchell, R., and Viands, D.: Switchgrass response to nitrogen fertilizer across diverse environments in the USA: A regional feedstock partnership report, BioEnergy Res., 7, 777–788, https://doi.org/10.1007/s12155-014-9484-y, 2014.
Hoover, A., Emerson, R., Ray, A., Stevens, D., Morgan, S., Cortez, M., Kallenbach, R., Sousek, M., Farris, R., and Daubaras, D.: Impact of drought on chemical composition and sugar yields from dilute-acid pretreatment and enzymatic hydrolysis of miscanthus, a tall fescue mixture, and switchgrass, Front. Energy Res., 6, 54, https://doi.org/10.3389/fenrg.2018.00054, 2018.
Ibrahim, M., Hong, C. O., Singh, S., Kumar, S., Osborne, S., and Owens, V.: Switchgrass biomass quality as affected by nitrogen rate, harvest time, and storage, Agron. J., 109, 86–96, https://doi.org/10.2134/agronj2016.07.0380, 2017.
Jungers, J. M., Clark, A. T., Betts, K., Mangan, M. E., Sheaffer, C. C., and Wyse, D. L.: Long-term biomass yield and species composition in native perennial bioenergy cropping systems, Agron. J., 107, 1627–1640, https://doi.org/10.2134/agronj15.0014, 2015.
Kelly, J. M., Kovar, J. L., Sokolowsky, R., and Moorman, T. B.: Phosphorus uptake during four years by different vegetative cover types in a riparian buffer, Nutr. Cycl. Agroecosyst., 78, 239–251, https://doi.org/10.1007/s10705-007-9088-4, 2007.
Kering, M. K., Butler, T. J., Biermacher, J. T., and Guretzky, J. A.: Biomass yield and nutrient removal rates of perennial grasses under nitrogen fertilization, BioEnergy Res., 5, 61–70, https://doi.org/10.1007/s12155-011-9167-x, 2012.
Kering, M. K., Butler, T. J., Biermacher, J. T., Mosali, J., and Guretzky, J. A.: Effect of Potassium and Nitrogen Fertilizer on Switchgrass Productivity and Nutrient Removal Rates under Two Harvest Systems on a Low Potassium Soil, BioEnergy Res., 6, 329–335, https://doi.org/10.1007/s12155-012-9261-8, 2013.
Lee, D. K., Aberle, E., Chen, C., Egenolf, J., Harmoney, K., Kakani, G., Kallenbach, R. L., and Castro, J. C.: Nitrogen and harvest management of Conservation Reserve Program (CRP) grassland for sustainable biomass feedstock production, GCB Bioenergy, 5, 6–15, https://doi.org/10.1111/j.1757-1707.2012.01177.x, 2013.
Lee, D. K., Doolittle, J. J., and Owens, V. N.: Soil carbon dioxide fluxes in established switchgrass land managed for biomass production, Soil Biol. Biochem., 39, 178–186, https://doi.org/10.1016/j.soilbio.2006.07.004, 2007a.
Lee, D. K., Owens, V. N., and Doolittle, J. J.: Switchgrass and soil carbon sequestration response to ammonium nitrate, manure, and harvest frequency on conservation reserve program land, Agron. J., 99, 462–468, https://doi.org/10.2134/agronj2006.0152, 2007b.
Lee, D. K., Parrish A. S., and Voigt, T.: Chap. 3, Switchgrass and giant miscanthus agronomy, in: Engineering and science of biomass feedstock production and provision, edited by: Shastri, Y., Hansen, A., Rodriguez, L., and Ting, K. C., 37–59, Springer New York, NY, https://doi.org/10.1007/978-1-4899-8014-4, 2014.
Lee, D. K., Aberle, E., Anderson, E. K., Anderson, W., Baldwin, B. S., Baltensperger, D., Barrett, M., Blumenthal, J., Bonos, S., Bouton, J., Bransby, D. I., Brummer, C., Burks, P. S., Chen, C., Daly, C., Egenolf, J., Farris, R. L., Fike, J. H., Gaussoin, R., Gill, J. R., Gravois, K., Halbleib, M. D., Hale, A., Hanna, W., Harmoney, K., Heaton, E. A., Heiniger, R. W., Hoffman, L., Hong, C. O., Kakani, G., Kallenbach, R., Macoon, B., Medley, J. C., Missaoui, A., Mitchell, R., Moore, K. J., Morrison, J. I., Odvody, G. N., Richwine, J. D., Ogoshi, R., Parrish, J. R., Quinn, L., Richard, E., Rooney, W. L., Rushing, J. B., Schnell, R., Sousek, M., Staggenborg, S. A., Tew, T., Uehara, G., Viands, D. R., Voigt, T., Williams, D., Williams, L., Wilson, L. T., Wycislo, A., Yang, Y., and Owens, V.: Biomass production of herbaceous energy crops in the United States: field trial results and yield potential maps from the multiyear regional feedstock partnership, GCB Bioenergy, 10, 698–716, https://doi.org/10.1111/gcbb.12493, 2018.
Lee, M. A., Davis, A. P., Chagunda, M. G. G., and Manning, P.: Forage quality declines with rising temperatures, with implications for livestock production and methane emissions, Biogeosciences, 14, 1403–1417, https://doi.org/10.5194/bg-14-1403-2017, 2017.
Li, Q., Yu, P. J., Li, G. D., and Zhou, D. W.: Grass-legume ratio can change soil carbon and nitrogen storage in a temperate steppe grassland, Soil Till. Res., 157, 23–31, https://doi.org/10.1016/j.still.2015.08.021, 2016.
Lin, C.-H., Namoi, N., Hoover, A., Emerson, R., Cortez, M., Wolfrum, E., Payne, C., Egenolf, J., Harmoney, K., Kallenbach, R., and Lee, D. K.: Harvest and nitrogen effects on bioenergy feedstock quality of grass-legume mixtures on Conservation Reserve Program grasslands, GCB Bioenergy, 15, 283–302, https://doi.org/10.1111/gcbb.12980, 2022.
Liu, X.-J. A., Fike, J. H., Galbraith, J. M., Fike, W. B., Parrish, D. J., Evanylo, G. K., and Strahm, B. D.: Effects of harvest frequency and biosolids application on switchgrass yield, feedstock quality, and theoretical ethanol yield, GCB Bioenergy, 7, 112–121, https://doi.org/10.1111/gcbb.12124, 2015.
Lovell, S. T. and Sullivan, W. C.: Environmental benefits of conservation buffers in the United States: Evidence, promise, and open questions, Agr. Ecosyst. Environ., 112, 249–260, https://doi.org/10.1016/j.agee.2005.08.002, 2006.
Lynn, J.: Comparing warm-season and cool-season grasses for erosion control, water quality, and wildlife habitat. USDA-NRCS publication, https://efotg.sc.egov.usda.gov/references/public/va/NWSG_CSG_comparison.pdf (last access: 16 October 2023), 2004.
MacAdam, J. W. and Nelson, C. J.: Physiology of forage plants, edited by: Barnes, R. F., Nelson, C. J., Collins, M., and Moore, K. J., in: Forages: An introduction to grassland agriculture, 6th Edn., Iowa State Press, Ames, IA, 73–97, Wiley-Blackwell, ISBN: 978-0813804217, 2003.
Marijanušić, K., Manojlović, M., Bogdanović, D., Čabilovski, R., and Lombnaes, P.: Mineral composition of forage crops in respect to dairy cow nutrition, Bulg. J. Agric. Sci., 23, 204–212, 2017.
Márquez, C. O., Garcia, V. J., Schultz, R. C., and Isenhart, T. M.: Assessment of soil degradation through soil aggregation and particulate organic matter following conversion of riparian buffer to continuous cultivation, Eur. J. Soil Sci., 68, 295–304, https://doi.org/10.1111/ejss.12422, 2017.
McDowell, L. R.: Minerals in Animal and Human Nutrition, 2nd Edn., Elsevier, ISBN: 978-0-444-51367-0, 2003.
Mehmood, M. A., Ibrahim, M., Rashid, U., Nawaz, M., Ali, S., Hussain, A., and Gull, M.: Biomass production for bioenergy using marginal lands, Sustain. Prod. Consump., 9, 3–21, https://doi.org/10.1016/j.spc.2016.08.003, 2017.
Mialon, M. M., Martin, C., Garcia, F., Menassol, J. B., Dubroeucq, H., Veissier, I., and Micol, D.: Effects of the forage-to-concentrate ratio of the diet on feeding behaviour in young Blond d'Aquitaine bulls, Animal, 2, 1682–1691, https://doi.org/10.1017/S1751731108002905, 2008.
Minson, D. J.: Forage quality: Assessing the plant-animal complex, in: Proc. XIV Int. Grassl. Congr., edited by: Smith, J. A. and Hays, V. W., p. 23, Westview Press, Inc., Boulder, CO, CRC Press, ISBN: 9780429303142, 1981.
Mitchell, R. and Schmer, M.: Switchgrass Harvest and Storage, in: Switchgrass, editedby: Monti, A., Green Energy and Technology, Springer, London, ISBN: 978-1447129028, 2012.
Mitchell, R., Lee, D. K., and Casler, M.: Switchgrass, in: Cellulosic Energy Cropping Systems, edited by: Karlen, D. L., 1st Edn., Wiley, New York, 75–89, ISBN: 9781118676325, 2014.
Monti, A., Barbanti, L., Zatta, A., and Zegada-Lizarazu, W.: The contribution of switchgrass in reducing GHG emissions, GCB Bioenergy, 4, 420–434, https://doi.org/10.1111/j.1757-1707.2011.01142.x, 2012.
Mulkey, V. R., Owens, V. N., and Lee, D. K.: Management of switchgrass-dominated conservation reserve program lands for biomass production in South Dakota, Crop Sci., 46, 712–720, https://doi.org/10.2135/cropsci2005.04-0007, 2006.
Mullahey, J. J., Waller, S. S., Moore, K. J., Moser, L. E., and Klopfenstein, T. J.: Insitu ruminal protein-degradation of switchgrass and smooth bromegrass, Agron. J., 84, 183–188, https://doi.org/10.2134/agronj1992.00021962008400020012x, 1992.
Nielsen, N. I., Volden, H., Akerlind, M., Brask, M., Hellwing, A. L. F., Storlien, T., and Bertilsson, J.: A prediction equation for enteric methane emission from dairy cows for use in NorFor, Acta Agric. Scand. A. Anim. Sci., 63, 126–130, https://doi.org/10.1080/09064702.2013.851275, 2013.
NRC: Nutrient Requirements of Dairy Cows, 7th Edn., National Academy of Sciences, Washington, DC, National Academies Press, ISBN: 9780309069977, 2001.
NRC: Mineral Tolerances of Animals, 2nd Edn., National Academy of Sciences, Washington, DC, National Academies Press, ISBN: 9780309096546, 2005.
Nyfeler, D., Huguenin-Elie, O., Matthias, S., Frossard, E., and Luscher, A.: Grass-legume mixtures can yield more nitrogen than legume pure stands due to mutual stimulation of nitrogen uptake from symbiotic and non-symbiotic sources, Agric. Ecosyst. Environ., 140, 155–163, https://doi.org/10.1016/j.agee.2010.11.022, 2011.
Ominski, K. H., Boadi, D. A., and Wittenberg, K. M.: Enteric methane emissions from backgrounded cattle consuming all-forage diets, Can. J. Anim. Sci., 86, 393–400, https://doi.org/10.4141/a05-051, 2006.
Parrish, D. J. and Fike, J. H.: The biology and agronomy of switchgrass for biofuels, Crit. Rev. Plant Sci., 24, 423–459, https://doi.org/10.1080/07352680500316433, 2005.
Pedroso, G. M., Hutmacher, R. B., Putnam, D., Six, J., van Kessel, C., and Linquist, B. A.: Biomass yield and nitrogen use of potential C4 and C3 dedicated energy crops in a Mediterranean climate, Field Crop. Res., 161, 149–157, https://doi.org/10.1016/j.fcr.2014.02.003, 2014.
Quijas, S., Schmid, B., and Balvanera, P.: Plant diversity enhances provision of ecosystem services: A new synthesis, Basic Appl. Ecol., 11, 582–593, https://doi.org/10.1016/j.baae.2010.06.009, 2010.
Rabalais, N. N., Turner, R. E., and Wiseman Jr., W. J.: Gulf of mexico hypoxia, a.k.a. “the dead zone”, Annu. Rev. Ecol. Syst., 33, 235–263, https://doi.org/10.1146/annurev.ecolsys.33.010802.150513, 2002.
Sage, R. F. and Zhu, X.-G.: Exploiting the engine of C4 photosynthesis, J. Exp. Bot., 62, 2989–3000, https://doi.org/10.1093/jxb/err179, 2011.
Sage, R. F., Pearcy, R. W., and Seemann, J. R.: The nitrogen use efficiency of C3 and C4 plants 1: III. leaf nitrogen effects on the activity of carboxylating enzymes in chenopodium album (L.) and amaranthus retroflexus (L.), Plant Physiol., 85, 355–359, https://doi.org/10.1104/pp.85.2.355, 1987.
Sanderson, M. A., Read, J. C., and Reed, R. L.: Harvest management of switchgrass for biomass feedstock and forage production, Agron. J., 91, 5–10, https://doi.org/10.2134/agronj1999.00021962009100010002x, 1999.
Sanderson, M. A., Brink, G., Ruth, L., and Stout, R.: Grass-legume mixtures suppress weeds during establishment better than monocultures, Agron. J., 104, 36–42, https://doi.org/10.2134/agronj2011.0130, 2012.
SAS Institute, SAS/STAT 9.2 Users's guide. SAS Inst, Cary, NC, SAS Publishing, ISBN: 978-1607644392, 2007.
Schmitt, T. J., Dosskey, M. G., and Hoagland, K. D.: Filter strip performance and processes for different vegetation, widths, and contaminants, J. Environ. Qual., 28, 1479–1489, https://doi.org/10.2134/jeq1999.00472425002800050013x, 1999.
Schultz, R. C., Colletti, J. P., Isenhart, T. M., Simpkins, W. W., Mize, C. W., and Thompson, M. L.: Design and placement of a multispecies riparian buffer strip system, Agrofor. Syst., 29, 201–226, https://doi.org/10.1007/bf00704869, 1995.
Spears, J. W.: Minerals in Forages. In Forage Quality, Evaluation, and Utilization, American Society of Agronomy, 281–317, ISBN: 9780891181194, 1994.
Storlien, T. M., Volden, H., Almoy, T., Beauchemin, K. A., McAllister, T. A., and Harstad, O. M.: Prediction of enteric methane production from dairy cows, Acta Agr. Scand. A. Anim. Sci., 64, 98–109, https://doi.org/10.1080/09064702.2014.959553, 2014.
Suter, M., Connolly, J., Finn, J. A., Loges, R., Kirwan, L., Sebastia, M. T., and Luscher, A.: Nitrogen yield advantage from grass-legume mixtures is robust over a wide range of legume proportions and environmental conditions, Glob. Change Biol., 21, 2424–2438, https://doi.org/10.1111/gcb.12880, 2015.
Suttle, N. F.: Mineral Nutrition of Livestock. India, CABI, ISBN: 9781789240924, 2022.
Sweeney, B. W. and Newbold, J. D.: Streamside forest buffer width needed to protect stream water quality, habitat, and organisms: A literature review, J. Am. Water Resour. Assoc., 50, 560–584, https://doi.org/10.1111/jawr.12203, 2014.
Tufekcioglu, A., Raich, J. W., Isenhart, T. M., and Schultz, R. C.: Biomass, carbon and nitrogen dynamics of multi-species riparian buffers within an agricultural watershed in Iowa, USA, Agrofor. Syst., 57, 187–198, https://doi.org/10.1023/A:1024898615284, 2003.
Turner, R. E. and Rabalais, N. N.: Coastal eutrophication near the Mississippi river delta, Nature, 368, 619–621, https://doi.org/10.1038/368619a0, 1994.
UNEP and CCAC report: Global methane assessment: benefits and costs of mitigating methane emissions, https://www.ccacoalition.org/resources/global-methane-assessment-full-report (last access: 16 May 2024), 2021.
van der Weijde, T., Alvim Kamei, C., Torres, A., Vermerris, W., Dolstra, O., Visser, R., and Trindade, L.: The potential of C4 grasses for cellulosic biofuel production, Front. Plant Sci., 4, 107, https://doi.org/10.3389/fpls.2013.00107, 2013.
Varvel, G. E., Vogel, K. P., Mitchell, R. B., Follett, R. F., and Kimble, J. M.: Comparison of corn and switchgrass on marginal soils for bioenergy, Biomass Bioenerg., 32, 18–21, https://doi.org/10.1016/j.biombioe.2007.07.003, 2008.
Vitousek, P. M., Naylor, R., Crews, T., David, M. B., Drinkwater, L. E., Holland, E., Johnes, P. J., Katzenberger, J., Martinelli, L. A., Matson, P. A., Nziguheba, G., Ojima, D., Palm, C. A., Robertson, G. P., Sanchez, P. A., Townsend, A. R., and Zhang, F. S.: Nutrient imbalances in agricultural development, Science, 324, 1519–1520, https://doi.org/10.1126/science.1170261, 2009.
Vogel, K. P., Brejda, J. J., Walters, D. T., and Buxton, D. R.: Switchgrass biomass production in the Midwest USA: Harvest and nitrogen management, Agron. J., 94, 413–420, https://doi.org/10.2134/agronj2002.0413, 2002.
Yang, Y., Reilly, E. C., Jungers, J. M., Chen, J., and Smith, T. M.: Climate benefits of increasing plant diversity in perennial bioenergy crops, One Earth, 1, 434–445, https://doi.org/10.1016/j.oneear.2019.11.011, 2019.
Zamora, D. S., Wyatt, G. J., Apostol, K. G., and Tschirner, U.: Biomass yield, energy values, and chemical composition of hybrid poplars in short rotation woody crop production and native perennial grasses in Minnesota, USA, Biomass Bioenerg., 49, 222–230, https://doi.org/10.1016/j.biombioe.2012.12.031, 2013.
Zumpf, C., Lee, M.-S., Thapa, S., Guo, J., Mitchell, R., Volenec, J. J., and Lee, D.: Impact of warm-season grass management on feedstock production on marginal farmland in Central Illinois, GCB Bioenergy, 11, 1202–1214, https://doi.org/10.1111/gcbb.12627, 2019.