the Creative Commons Attribution 4.0 License.
the Creative Commons Attribution 4.0 License.
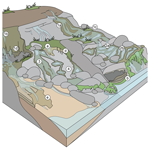
Reviews and syntheses: Tufa microbialites on rocky coasts – towards an integrated terminology
Thomas W. Garner
J. Andrew G. Cooper
Alan M. Smith
Gavin M. Rishworth
Matt Forbes
Microbialites are known from a range of terrestrial, freshwater, marine, and marginal settings. The descriptive terminology used in each instance depends largely on the historical legacy derived from previous studies in similar environments. This has led to a diversity of nomenclature and a lack of conformity in the terms used to describe and categorise microbialites. As the role of microbial mats and biofilms is increasingly recognised in the formation of tufa and terrestrial carbonates, deposits such as tufa microbialites bridge the spectrum of microbialites and terrestrial carbonate deposits. Groundwater spring-fed tufa microbialites in supratidal rock coast environments that occur at the interface of terrestrial and marine domains demonstrate the need for an integrative and systematic nomenclature approach. To date, their global distribution and complex relationships with pre-defined deposits have resulted in the application of a variety of descriptive terminologies, most frequently at the macro- and mesoscale. Here we review and consolidate the multi-scale library of terminologies for microbialites and present a new geomorphological scheme for their description and classification. This scheme has greater alignment with terrestrial carbonate nomenclature at the macroscale and with marine and lacustrine microbialites at the mesoscale. The proposed terminology can primarily be applied to tufa microbialites in spring-fed supratidal environments but may also be applicable in other relevant depositional environments including terrestrial carbonates, microbial mats, and other microbialites.
- Article
(14388 KB) - Full-text XML
- BibTeX
- EndNote
Burne and Moore (1987) define microbialites as “organosedimentary deposits that have accreted as a result of a benthic microbial community trapping and binding detrital sediment and/or forming the locus of mineral precipitation”. They have been described from a diverse range of depositional environments in both fossil and modern settings. These include marine environments with hypersaline conditions (e.g. Hamelin Pool, Western Australia; Jahnert and Collins, 2011, 2012; Logan, 1961; Logan et al., 1964; Suosaari et al., 2016, 2019b, 2022) and normal salinity conditions (e.g. The Bahamas; Dill et al., 1986; Reid et al., 1995, 2024) and a range of non-marine environments including, but not limited to, hyposaline (e.g. Moore and Burne, 1994), freshwater fluvial (e.g. Arenas et al., 2019; Caudwell et al., 2001), open lacustrine (e.g. Doddy et al., 2019), ice-covered lacustrine (e.g. Mackey et al., 2015), hot springs (e.g. Walter et al., 1976), and caves (e.g. Brunet and Revuelta, 2014; Lundberg and McFarlane, 2011). Marine and non-marine forms have traditionally been studied independently, and distinct suites of definitions and nomenclature have been applied in each setting. As noted by Shapiro (2005, p. 73), “[because] universal terminology have not been ratified by the general community, clear descriptions will facilitate future research”. Recently, the Handbook for the study and description of microbialites (Grey and Awramik, 2020) attempted to consolidate and refine the terminology applied to microbialites. This, however, explicitly excluded tufa microbialites and microbial mats, including rock coast microbialites, from consideration due to these lacking detailed morphological description.
Tufa microbialites occurring in groundwater springs in the supratidal zone of high-energy rock coastlines have affinities with both terrestrial and marine forms (Rishworth et al., 2020), and their study brings into sharp focus the need for unifying terminology. To date, the terminology applied to rock coast microbialites has been varied and often complex. This is due to the application of terminology from various research areas including microbialites (both fossil and contemporary) and terrestrial carbonates, predominantly tufa. Their apparently scattered distribution in widely separated locations has led to variability in the descriptive terminology applied by independent research groups. Now that they are known to have a global distribution, there is a need for clear and unambiguous terminology that can be refined and further developed by future research.
At the nexus of terrestrial and marine domains, rock coast microbialites afford the opportunity to assess the utility of existing terminology from both fields of research, to identify inter alia duplication and redundancy, and in so doing to present a unifying terminology for rock coast microbialite. This study specifically aims to
-
review the classification and description of rock coast microbialites using different disciplinary approaches;
-
review and integrate terrestrial and marine microbialite terminology applied to rock coast environments; and, on that basis,
-
produce a geomorphological terminology for rock coast microbialites at the macro- and mesoscale.
To that end, this paper begins by reviewing the various contexts in which rock coast microbialites have been studied (microbial structures, geomorphological features, and habitats). It then reviews the terminology that has been applied to rock coast microbialites and distils this to present an integrated geomorphological classification and terminology for rock coast microbialites.
Rock coast microbialites are a microbialite carbonate facies formed through microbial and microalgal biofilm fixation in groundwater-fed springs in the supratidal rock coast environment (Forbes et al., 2024; Rishworth et al., 2020). The term “rock coast” is applied here to describe a specific depositional environment of “rock coast settings in high intertidal to supratidal locations that are affected by occasional marine storms and/or high swells and the effects of sea spray” (Cooper et al., 2022). Generally, rock coasts are characterised by resistant bedrock and/or hardground superficial deposits. Rock coasts are distinct depositional environments that are exposed to a suite of distinct biological, physical, and chemical extrinsic forces and are typically considered erosional environments that are hostile to sedimentary deposition (Kennedy et al., 2014; Trenhaile, 2016). The rock coast environment is considered a key control on their occurrence; it is dependent on the partial exclusion of competing organisms and alternations between terrestrial and marine states, resulting in extreme conditions that facilitate microbialite development (Forbes et al., 2024). After a historic description at Bonza Bay, South Africa, by Mountain (1937), the first modern rock coast microbialite was documented by Smith and Uken (2003) from supratidal pools at Cape Morgan, south of Kei Mouth on the south-eastern coastline of South Africa. Subsequent occurrences were reported along the southern African coastline from Gqeberha (Port Elizabeth) to Tofo, Inhambane, Mozambique (Perissinotto et al., 2014; Smith et al., 2011); in South Australia and Western Australia (Forbes et al., 2010; Lipar and Webb, 2015); and in Northern Ireland, Scotland, and Ireland (Cooper et al., 2013, 2022; Smith et al., 2018).
Rock coast microbialites are considered a tufa microbialite, “a tufa forming as a result of microbial activity” (Grey and Awramik, 2020), with tufa being defined as the “products of calcium carbonate precipitation under cool, ambient temperature freshwater” (Pedley, 1990). Tufa is increasingly considered to have significant biological influence in its formation (Capezzuoli et al., 2014; Perri et al., 2012), and hence, tufa microbialites may be considered a biogenic facies of tufa formed by a benthic microbial community. As such, these deposits may also be considered part of contemporary tufa-forming environments and habitats such as “petrifying springs”. Petrifying spring and tufa formations have been recognised in a variety of coastal settings in Europe (EC, 2016; Farr and Graham, 2017; Faulkner and Crae, 2018; Howie and Ealey, 2009, 2010; Lyons and Kelly, 2016; Rodríguez Guitián et al., 2020). Research on rock coast microbialites has been approached in numerous ways, including as microbialites and as terrestrial carbonate deposits, predominantly tufa, and as part of a wider ecological system or habitat. Each of these approaches is described and discussed below.
2.1 Microbialites and microbial mats
Microbial mats are stratified, highly organised, and diverse microbial communities defined as “discrete benthic structures constructed by microorganisms (eukaryotic and prokaryotic; photosynthetic and nonphotosynthetic)” (Bauld, 1981, in Grey and Awramik, 2020). They are the principal constructional layer of microbialites, including stromatolites, thrombolites, dendrolites, leiolites, and microbially induced sedimentary structures (MISSs) (Noffke et al., 2001; Noffke and Awramik, 2013). Microbial mats are found in a variety of environments including hypersaline, coastal and intertidal, oligotrophic, extreme low-temperature, hot-spring, and acid environments (Prieto-Barajas et al., 2018). In coastal settings, they are predominantly described from the soft-substrate intertidal zone (Gerdes and Krumbein, 1994; Prieto-Barajas et al., 2018) and the rock coast environment and in the supratidal zone, where microbial mats have been described from Northern Ireland and South Africa as thin mats that may develop on sub-horizontal surfaces in shallow water, often in a discharge apron (Cooper et al., 2013; Edwards et al., 2017).
MISSs are defined as “sedimentary structures in siliciclastic sediments and rocks induced by microbial activity” (Noffke et al., 1996). They occur in siliciclastic, evaporitic, and carbonate settings (Bose and Chafetz, 2012; Noffke and Awramik, 2013) and have been described globally, notably in the North Sea (Gerdes et al., 2000), the Mediterranean (Aref, 1998; Gerdes et al., 2000; Lakhdar et al., 2020; Sanchez-Cabeza et al., 1999), the north-west Atlantic (Cameron et al., 1985), and the Gulf of Mexico (Bose and Chafetz, 2009). MISSs form due to the trapping, baffling, binding, and biostabilisation of detrital sediment by a biofilm or microbial mat and its interaction with the extrinsic environment (Noffke, 2008, 2010; Noffke and Awramik, 2013). The formation of MISSs contrasts with that of other microbialites, including stromatolites, which require repeated primary mineral precipitation in the microbial mat's extracellular polymeric substances (EPSs) to accrete into a layered structure (Noffke, 2010; Noffke and Awramik, 2013).
MISSs may occur on rock coasts where precipitation is limited and microbial mats form on loose sediment; however in the rock coast setting microbial mats are commonly carbonate precipitating and fixed on resistant bedrock and/or hardground superficial deposits. The interaction of these microbial mats with the rock coast environment does, however, result in features that are superficially similar to those of MISSs or “microbial mat features”, such as “blister mats” described by Edwards et al. (2017). Thin mats with limited repeat layering or carbonate precipitation share many similarities with MISSs due to their comparable interactions with the environment. Despite their ubiquity, there has been a general lack of description of MISSs or microbial mat features in the rock coast environment to date.
The repeated trapping and binding of detrital sediment and/or the mineral precipitation by the benthic microbial community may form a stromatolite through repeated stacking of microbial mats (Logan et al., 1964; Noffke and Awramik, 2013; Reid et al., 2024). Stromatolites are “a laminated organosedimentary structure produced by precipitation, or by sediment trapping and binding, as a result of the growth, behaviour, and metabolic activity of micro-organisms, principally cyanobacteria” (Grey and Awramik, 2020) based upon definitions provided by Awramik and Margulis in Walter (1976). They are considered a subset of “microbialites”, a term first applied by Burne and Moore (1987) as an umbrella term for stromatolites, thrombolites, dendrolites, leiolites, and MISSs. These subset forms of microbialites arise through different primary depositional or secondary post-depositional and diagenetic processes, and sometimes the nature of the structural drivers is obscure. For example, thrombolites might develop because of distinct microbial communities that give rise to clotted structure or through bioturbation that destroys primary lamination (Burne and Moore, 1987; Harwood Theisen and Sumner, 2016; Moore and Burne, 1994; Shapiro, 2000).
2.2 Terrestrial and marginal marine carbonates
Terrestrial (non-marine/continental) carbonate deposits accumulate in a variety of depositional environments including lacustrine, palustrine, cave, fluvial, subaerial, spring, and hypogean environments (Della Porta, 2015), forming a spectrum of carbonate deposits with no universally accepted classification (Della Porta, 2015). In addition, carbonates also accumulate in transitional marginal marine environments including beach (foreshore), barrier and coastal lagoon, and peritidal environments (Flügel, 2010). Despite numerous coastal tufa deposits being described (Faulkner and Crae, 2018; e.g. Forbes et al., 2010; Howie and Ealey, 2009, 2010; Rodríguez Guitián et al., 2020), rock coast microbialites and associated carbonate deposits are not considered typical transitional marginal marine carbonates.
Tufa, a terrestrial carbonate deposit, has an often controversial and complex nomenclature, with core definitions frequently contested, most notably the distinction between tufa and travertine (Capezzuoli et al., 2014; Della Porta, 2015; Ford and Pedley, 1996; Pentecost, 2005). While definitions based upon a carbon dioxide source and the chemical mechanism of precipitation have been proposed for tufa (see Capezzuoli et al., 2014), it is often defined as “the products of calcium carbonate precipitation under cool, ambient temperature freshwater: they [the tufa deposits] typically contain remains of micro- and macrophytes, invertebrates and bacteria” (Ford and Pedley, 1996; Pedley, 1990), as described by Della Porta (2015). This definition distinguishes between tufa (meteogene travertine) and travertine sensu stricto (s.s.) (thermogene travertine), both constituents of travertine sensu lato (s.l.). If rock coast microbialites are viewed as a biogenic facies of travertine s.l., the term tufa is best applied due to the ambient temperature of the source springs of microbialite occurrences (Cooper et al., 2013; Dodd et al., 2018; Forbes et al., 2010; Perissinotto et al., 2014; Rishworth et al., 2016, 2017). Furthermore, the cool-water-temperature definition for tufa often refers to cyanobacteria, heterotrophic bacteria, and algae being present, or requires they are present, and is dependent on ambient temperatures <30 °C (Capezzuoli et al., 2014), supporting microbialite facies.
The presence of biofilms is ubiquitous in terrestrial carbonate and tufa deposits, as demonstrated by Della Porta (2015), and increasingly tufa is considered to be a microbially induced/influenced sedimentary deposit (Capezzuoli et al., 2014; Perri et al., 2012). This position does not exclude an abiotic control on calcium carbonate precipitation (Ford and Pedley, 1996; Merz-Preiß and Riding, 1999) but suggests that biofilms may have a substantial or dominant role in the formation of tufa, as evidenced by in-field investigation (e.g. Manzo et al., 2012; Shiraishi et al., 2008), field experiments (e.g. Gradziński, 2010), and in vitro mesocosm experiments (e.g. Pedley et al., 2009; Rogerson et al., 2010). While it may be tempting to classify any microbially influenced tufa as microbialites, it has been suggested that while terrestrial carbonates such as lake crusts and laminated travertines s.l. are frequently cited as stromatolites, the use of the term has been applied too loosely (Pentecost and Viles, 1994).
2.3 Tufa microbialite facies and evidence of biogenicity
Rock coast microbialites are considered a distinct biologically mediated tufa facies which includes carbonate-precipitating microbial mats (i.e. not MISSs) and thicker repeated units forming stromatolites and other microbialites in the rock coast environment. Lithologically, in situ tufa microbialites (excluding mobile oncoids and detrital tufa microbialites) have been described as autochthonous tufa deposits as they are “phytohermal constructions where there is an in situ organic framework” (Ford and Pedley, 1996). As a tufa facies, stromatolitic tufa has been described and classified in multiple studies, for example as phytoherm boundstones defined by Pedley (1990) as “in situ stromatolitic build-ups with fringe-cements … often associated with oncoids” (Pedley, 1990, in Pentecost, 2005). While the tufa microbialite facies appears dominant at recognised localities, other associated carbonate facies are frequently present within the same supratidal groundwater spring system, and care should be taken to identify and delineate these facies. This includes non-microbialite tufas (e.g. bryophyte phytoherm framestone), both surface and hypogean; speleothem, pedogenic carbonates (e.g. palaeosols); and beachrock/carbonate cements.
While the biogenicity of this facies has been demonstrated in South African occurrences (e.g. Perissinotto et al., 2014; Smith et al., 2005; Smith and Uken, 2003), the role of microbiota in the formation of other rock coast microbialite localities, such as those in Co. Sligo, Ireland (e.g. Cooper et al., 2022), has not yet been thoroughly assessed. A greater understanding of the role of microbiota and microbialite builders in global occurrences of rock coast microbialites is required to establish biogenicity and accurate delimitation of this distinct facies, allowing for other associated terrestrial and marginal marine carbonate facies to be distinguished. Associated non-microbialite terrestrial carbonate facies have been described in association with rock coast microbialites, including rhizoliths described by Edwards et al. (2017) and surface-cemented rudites, also called beachrock (Edwards et al., 2017); shell conglomerate (Perissinotto et al., 2014); and sand beach deposits (Cooper et al., 2022). More detailed study of biogenicity and physico-chemical vs. biological controls over carbonate precipitation will allow for the refinement of the relationships between transitional carbonates, such as where cave-resurgence-associated speleothem grades into tufa microbialite (Pedley and Rogerson, 2010).
The role of biofilms and microbial mats in the formation of tufa is increasingly considered to be crucial. This is also evident in the numerous descriptions of stromatolitic tufa facies within the terrestrial-carbonate-focused and tufa-focused literature such as those of fluvio-lacustrine, fluvial, and lacustrine tufa deposits (e.g. Arenas et al., 2000, 2010, 2019; Guo and Chafetz, 2012; Manzo et al., 2012; Pedley et al., 1996; Ritter et al., 2017; Rodríguez-Berriguete, 2020; Valero Garcés et al., 2008). This is also extended to thermal travertine s.s. spring deposits (e.g. Gandin and Capezzuoli, 2014). These facies may be considered “tufa stromatolites”, a subset of tufa microbialites, bridging the gap between tufa and microbialites and bringing stromatolitic tufa facies under the umbrella of stromatolites and microbialites.
Tufa microbialites, including tufa stromatolites (Riding, 1991) and tufa thrombolites (Riding, 2000), are “a tufa forming as a result of microbial activity [that may be] … laminated, clotted or shrubby” (Grey and Awramik, 2020), dominated by mineral precipitation on (as opposed to within) organic substances, predominantly by cyanobacteria in freshwater lakes and streams (Riding, 1991). The term “tufa microbialite” supersedes alternative terms such as microbial tufa (Burne and Moore, 1987), algal tufa (Warren, 1982), cryptalgal tufa (Monty, 1976), and cryptomicrobial tufa (Grey and Awramik, 2020).
Tufa and tufa microbialites have been excluded from much microbialite work, including guides to their description and classification due to a lack of detailed morphological description (Grey and Awramik, 2020). However, the integration of tufa, along with travertine, speleothem, sinter, and microbial crust, within microbialite classification has been advocated (Grey and Awramik, 2020).
2.4 Habitats and ecology
The occurrence of active tufa deposits forms a distinct and ecologically unique habitat. Within Europe, the habitat type “H7220 Petrifying springs with tufa formation (Cratoneurion)” is recognised by the Habitats Directive (Council Directive 92/43/EEC on the conservation of natural habitats and of wild fauna and flora) as “hard water springs with active formation of travertine or tufa. These formations are found in such diverse environments as forests or open countryside. They are generally small (point or linear formations) and dominated by bryophytes (Cratoneurion commutati)” (EC, 2013). This corresponds to the UK National Vegetation Classification of “M37 Cratoneuron commutatum – Festuca rubra spring community” and the “M38 Cratoneuron commutatum – Carex nigra spring community” (EC, 2013; Rodwell, 1992). In Ireland, Lyons and Kelly (2016) define eight petrifying spring plant communities including the group 1 plant community “Eucladium verticillatum-Pellia endiviifolia Tufa Cascades”. This plant community describes often near-vertical, steep tufa cascades that occur on coastal rocky cliffs within the supralittoral spray zone (Lyons and Kelly, 2016). The recognition of rock coast microbialites as part of these habitats may lead to their future identification at other localities.
Other attempts to map global occurrences of rock coast microbialites with greater recognition have been undertaken with the aim to acknowledge and protect their biodiversity. South African occurrences have been recognised in the National Biodiversity Assessment of South Africa (Rishworth et al., 2019), and in south-western Western Australia they have been recognised as “Augusta Microbial Threatened Ecological Communities” (Forbes et al., 2010; Onton et al., 2009).
The following section reviews the numerous classification schemes that may be applied to rock coast microbialites from a number of different research areas including microbialites and terrestrial carbonates.
3.1 Previous description and classification schemes
3.1.1 Microbialites
Microbialite researchers have typically avoided the application of established carbonate classifications of Folk (1959) and Dunham (1962) (Bosence et al., 2015), and instead morphogenetic classifications are frequently applied, through the description of internal structure/organisation or of fabric, and are intrinsically linked to depositional processes and products (Burne and Moore, 1987; Riding, 1991). The description of microbialites is most commonly aided through the use of the traditional hierarchical scale of observation from mega-, macro-, meso-, and microstructure, as defined by Shapiro (2005) and discussed by Grey and Awramik (2020) (Fig. 1a). These levels are not mutually exclusive and are partially open-ended (Grey and Awramik, 2020). Other spatio-temporal scales have been suggested such as the scale of Ibarra and Corsetti (2016).
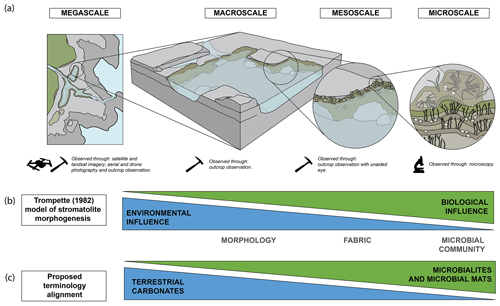
Figure 1(a) Diagram of hierarchical structural scale from megascale to microscale (based on Grey and Awramik, 2020; Shapiro, 2005); (b) Trompette (1982) model of stromatolite morphogenesis, adapted from Suosaari et al. (2019a); (c) the proposed terminology alignment between terrestrial carbonates and microbialites and microbial mats.
Microbialite classification has been much debated and divided, with application of a great variety of terminology and schemes across the globe (Grey and Awramik, 2020). Early attempts at microbialite classifications involved the application of binomial Linnaean nomenclature, with the first description of the stromatolite Cryptozoön of upper Cambrian age (Hall, 1883). Since then, a wide variety of additional classification schemes (e.g. Cao and Bian, 1985; Donaldson, 1963; Szulczewski, 1968) have been proposed, including descriptive polynomial schemes (Hofmann, 1969; Maslov, 1953, 1960) and descriptive geometric formulae (Logan et al., 1964). A vast array of naming classifications and systems have been applied, with many abandoned due to inadequacies, such as lack of consistency and unwieldy terminologies, resulting in the current state of classification being undoubtedly messy and confusing (Grey and Awramik, 2020).
3.1.2 Terrestrial carbonates
In the description of terrestrial carbonates, hierarchical mega- to microscale descriptions, comparable to those applied to microbialites and leading to similar classifications, have been proposed. For example, Ordonez et al. (1986) considered a hierarchical macro- to mesostructural scale for Holocene and modern tufa systems and Guo and Riding (1998) applied a three-part classification scheme to a Late Pleistocene travertine system, considering lithotypes, depositional systems, and facies.
Due to the morphological and geochemical similarities at the macroscale, rock coast microbialite classifications have also been closely aligned to other terrestrial carbonate deposits. This is despite a current lack of a universal nomenclature for terrestrial carbonates (Della Porta, 2015). For example, calcite and aragonite speleothems may be considered hypogean travertines (Pentecost, 2005), although the classification of speleothems is distinct from other terrestrial carbonates, with a vast array of types (see Hill and Forti, 1997). Tufa can be classified through a variety of criteria that are not mutually exclusive, commonly through geochemical, fabric, and morphological criteria (see Pentecost, 2005; Pentecost and Viles, 1994), and these classification schemes are briefly summarised here.
Recognised by Pentecost (1993), tufa can be classified based upon geochemistry, and the origin of the carrier carbon dioxide has been used to distinguish between travertine s.s. and tufa. The origin of the carrier affects the isotopic composition of the deposit and influences the morphology and fabric (Pentecost, 2005). Pentecost (1993) considers meteogene travertines (classified here as tufa) deposited from a meteoric water source and thermogene travertine (travertine s.s.) deposited from a thermal water source (Pentecost, 1993; Pentecost and Viles, 1994). Tufa fabric, “the architecture of the deposit (i.e. the arrangement, density and size of the building units)” (Pentecost and Viles, 1994), present at the micro- and mesoscale and referred to as the micro- and mesofabrics, can also be classified, often with a focus on the biological influences or “biofabric” (Pentecost, 2005).
One of the most commonly applied classifications is that of tufa morphology or geomorphology. A seminal classification corresponding to environmental setting by Pedley (1990) was adapted by Pentecost (1993) and Pentecost and Viles (1994) and was further expanded upon by Ford and Pedley (1996). This thread of geomorphological classifications has been frequently applied to British and Irish tufa deposits (e.g. Farr and Graham, 2017; Lyons and Kelly, 2016), partially due to its British and north-west European scope (Pentecost, 1993); occasionally, classifications have also been applied globally (Forbes et al., 2010). Tufa systems are commonly described and classified through facies approaches that can integrate the above classifications. One of the most frequently applied is that of Ford and Pedley (1996), who divide tufas into allochthonous and autochthonous tufa deposits, the latter of which is subclassified into facies (Jones and Renaut, 2010), based upon established carbonate classification schemes.
3.2 Rock coast microbialite classifications and description
Rock coast microbialites have been described and classified based upon a wide range of sources and schemes. The global distribution of rock coast microbialites, despite the majority of research output being based upon localities in South Africa (Rishworth et al., 2020), has led to inconsistencies in the terminology applied and the need for consolidation, unification, and definition.
Early descriptions consider deposits with different approaches to this dependent on a microbialite (e.g. Perissinotto et al., 2014; Smith and Uken, 2003) or terrestrial carbonate (e.g. Forbes et al., 2010) outlook. As with terrestrial carbonates and other tufa facies, rock coast microbialites may be subclassified based upon their geochemistry and fabric. Rock coast microbialites are partially defined by their characteristic carrier carbon dioxide source of supratidal freshwater springs emerging from a carbonate-saturated groundwater source (Rishworth et al., 2020). This results in the bulk mineralogy for rock coast microbialites dominated by calcite and aragonite (Dodd, 2019; Forbes et al., 2010; Rishworth et al., 2020). Rock coast microbialites also have distinct fabrics (e.g. clotted thrombolites, laminated stromatolites, and aphanitic leiolites). While the classification of geochemistry and fabric may be suitable to apply to rock coast microbialite occurrences, the macroscale geomorphology, equated to microbialite macrostructure, is the most frequently applied classifier requiring a unified classification. This macrostructure geomorphology is typically taken from tufa geomorphological classifications (Ford and Pedley, 1996; Pedley, 1990; Pentecost, 1993; Pentecost and Viles, 1994) and adjusted for the rock coast environment. Recently, a facies approach to rock coast microbialites, which considers a general classification scheme of microbialite facies on siliciclastic rock coasts, has been applied by Cooper et al. (2022). A similar approach was adopted by Forbes et al. (2010).
In order to address the presence of synonyms, duplications, and omissions and the wide variety of terms applied to the same morphological features, predominantly at the macro- and mesoscale, the following proposed classification system will consider microbialite, terrestrial carbonate, and past rock coast microbialite terminology and classifications. This is approached using the hierarchical microbialite scale as defined by Shapiro (2005) and discussed by Grey and Awramik (2020).
Trompette (1982) proposed a morphogenetic model, suggesting that the environment (abiotic influence) was the dominant influence on stromatolite formation at the macroscale and that the biological influences predominated at the microscale (Suosaari et al., 2019a; Trompette, 1982) (Fig. 1b). This model was modified by Suosaari et al. (2019a) on examination of microbialite systems in Hamelin Pool and The Bahamas. These hierarchical structural models for both tufas and microbialites demonstrate comparable controls on morphology and subsequently may be applied to tufa microbialites; however, such models would need to be tested. Therefore, the approach taken here is that of a hierarchical scale that equates to microbialite mega-, macro-, meso-, and microstructure with the application of terminology from terrestrial carbonates. While this appears most suitable at the macroscale where environmental influence is greatest, microbialite terminology may also be applied at the meso- and microscale (Fig. 1b and c).
While a rock coast microbialite classification system is considered to be important for future research, care must be taken to avoid excessive categorisation, especially based upon morphogenesis, which remains poorly understood in rock coast microbialites. Current understanding of terrestrial carbonate morphogenesis is already much more advanced and may be comparable at the macroscale, yet the relationships between these deposits are not understood well enough for direct correlation. While terrestrial carbonates can already be classified based upon fabric and geochemistry, the current lack of description and of sufficiently large datasets concerning rock coast microbialites currently excludes such classification schemes.
4.1 Megastructure
Megastructure addresses the largest-scale (metre to decimetre) aspects of microbialite occurrence and their respective beds, examining the bed-scale or stratum-scale structures, including buildups such as the largest bioherms or biostromes (Grey and Awramik, 2020; Shapiro, 2005). This may refer to the location (e.g. aerial extent and stratigraphic setting), surrounding strata relationships (substrate, initiation, interface, and growth direction), mode of occurrence (e.g. buildup, bioherm, and biostrome), and shape (Grey and Awramik, 2020). For the study of rock coast microbialites, the megastructure has been commonly described within the study site setting.
4.2 Macrostructure
Following Grey and Awramik (2020), macrostructure refers to aspects of the majority of bioherms and biostromes, including features of microbialite gross morphology, and describes features such as shape (e.g. stratiform, oncoidal, and columnar), plan view, and linkage.
Rock coast microbialite macrostructure has followed the definition for microbialite macrostructure relatively closely (Edwards et al., 2017); however, there is generally a focus on the gross morphology of microbialite occurrences as opposed to shape (e.g. columnar), which is generally considered a feature of mesostructure. Terminology has also frequently been adapted from terrestrial carbonate nomenclature (Fig. 2) due to the evident similarity in geomorphology. For example, Forbes et al. (2010) applied tufa nomenclature, producing two depositional models featuring the perched springline model, fluvial barrage model, and cascade of Ford and Pedley (1996). This was referred to and built upon by Perissinotto et al. (2014), who consider “stromatolite formations” consisting of barrage pools with downstream rimstone dams, waterfall deposits (sensu Forbes et al., 2010), and associated “shelly conglomerates”. These seminal works strongly influenced subsequent studies such as the work of Edwards et al. (2017), who along with the previous detailed macrostructures also included discharge aprons. Despite frequent reference to the geomorphology of these deposits, there are scant published data on their thickness and geometry, with the majority of information supplied by Forbes et al. (2010), Perissinotto et al. (2014), and Edwards et al. (2017).
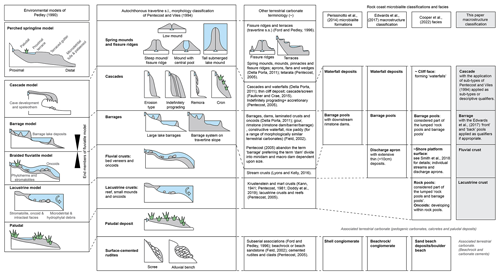
Figure 2Comparison of terrestrial carbonate terminology (applying key classification schemes of Pedley, 1990, and Pentecost and Viles, 1994m, and other terminology; Della Porta, 2011; Doddy et al., 2019; Field, 2002; Faulkner and Crae, 2018; Field, 2002; Ford and Pedley, 1996; Lyons and Kelly, 2016; Pentecost, 1981, 2005) and rock coast microbialite terminology (Cooper et al., 2022; Edwards et al., 2017; Perissinotto et al., 2014; Smith et al., 2018).
The shore-platform environment (Smith et al., 2018) contains microbialites composed of thin crusts, barrage pools, and shallow rock pools, considered in a microbialite “modes of occurrence” facies approach by Cooper et al. (2022) alongside cliff face, boulder beach, rock pool and barrage pool, oncoid, and sand beach deposits. The consistent inclusion of surface-cemented rudites (shell conglomerate, beachrock, sand beach deposits, etc.) without direct evidence of biogenicity means that they are not included within the current classification; however, that is not to say they should not be included within wider studies of rock coast terrestrial and marginal marine carbonates (Fig. 3).
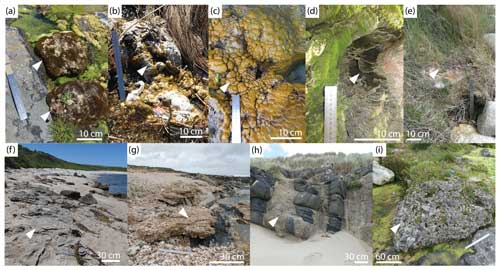
Figure 3Contemporary and Holocene terrestrial carbonates commonly associated with rock coast microbialites: (a) bryophyte phytoherm framestone tufa (Sligo Bay, Ireland); (b) surface (non-microbialite) tufa (Schoenmakerskop, South Africa); (c) hypogean tufa (Quarry Bay, Western Australia); (d) speleothem (Mullaghmore, Ireland); (e) paludal tufa (Canal Rocks, Western Australia); and (f–i) incipient beachrocks and carbonate cements – (f) Quarry Bay, Western Australia; (g) Lauries Bay, South Africa; (h) Saligo Bay, Isle of Islay, Scotland; and (i) Sligo Bay, Ireland.
The following geomorphological classification distinguishes between the geomorphology of the microbialite deposit and the environment or wider setting (e.g. fluvial and lacustrine crust macrostructures present in a discharge apron in the shore-platform environment). Due to this complex nomenclature, the following terminology is suggested (Fig. 4).
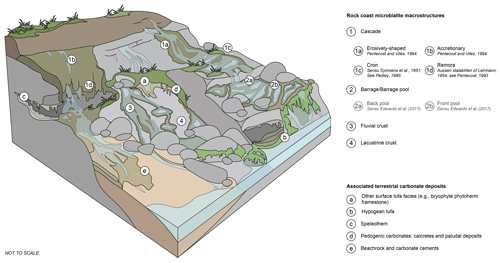
Figure 4Block diagram of rock coast microbialite macrostructure and associated terrestrial carbonate deposits.
4.2.1 Cascade
Cascades are tufa microbialite deposits deposited on steep shore topographies producing a waterfall-like morphology, ranging from a few millimetres to 30 cm in thickness (Cooper et al., 2022).
In rock coast microbialite nomenclature, a variety of terms have been used to describe tufa microbialite deposits on steep gradients; however, cascade deposits (Fig. 5a–c) have been rarely termed as such. Researchers have frequently cited the “waterfall deposit” of Forbes et al. (2010) (Edwards et al., 2017; Perissinotto et al., 2014) despite considering that such deposits bear a striking resemblance to the morphology of tufa deposits (Edwards et al., 2017). The cliff face microbialite facies described by Cooper et al. (2022) is considered to be dominated by the cascade macrostructure, as the cliff face facies is partially defined by its steep shore topography that produces cascade macrostructures.
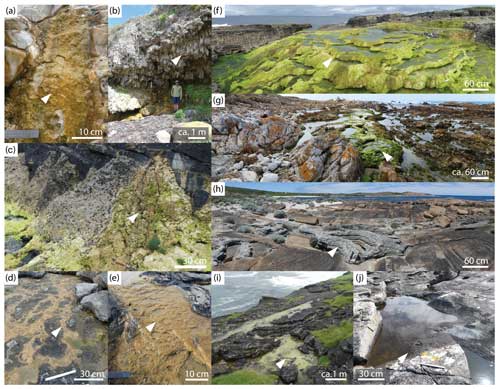
Figure 5Rock coast microbialite macrostructures: (a) erosively shaped cascade (Cape St Francis, South Africa); (b) remora (Quarry Bay, Western Australia); (c) accretionary cascades (Aughris Head, Ireland); (d) fluvial crust (Sligo Bay, Ireland); (e) fluvial crust (Sligo Bay, Ireland); (f) barrages (Sligo Bay, Ireland); (g) barrages (Lauries Bay, South Africa); (h) inactive barrages (Quarry Bay, Western Australia); (i) lacustrine crust (Bundoran, Ireland); (j) lacustrine crust (Mullaghmore, Ireland).
Pentecost and Viles (1994) considered tufa “cascades” and identified two distinct forms controlled by variable erosion–deposition rates. The first form is “erosively shaped” deposits, paraboloid in section, with the surface morphology controlled by the spate water trajectory (Fig. 5a), and the second form is “accretionary” deposits, where the rate of deposition is greater than the rate of erosion, resulting in progradation (Fig. 5c). This second form consists of subtypes. These include keeled cascades which consist of a narrow slot constricting water flow before falling over a travertine s.l. nose and “tubes” forming a travertine spout at the top of cascades (Pentecost, 1993, 2005). “Cron” is a poorly observed cascade subtype of small staggered dams with boggy pools and is an intermediate between cascades and barrage pools (Pedley, 1990; Pentecost, 1993, 2005; Symoens et al., 1951). Considered a form of speleothem by Pentecost (2005), “Aussen stalaktiten” (Lehmann, 1954), renamed remora (Pentecost, 1993), is a stalactite-like mass of terrestrial carbonate that develops on steep slopes in slow or periodic water flow (Pentecost, 2005) (Fig. 5b). Preferential precipitation of carbonate may result in remora extending from a vertical surface, potentially due to algal growth (Dobat, 1966; Rong et al., 1996), resulting in a “phototrophic stalactite” (Pentecost, 2005). Ford and Pedley (1996) also consider waterfall or cascade tufas but suggest that these forms may be constituents of larger systems and that elements of cascade and paludal models described by Pedley (1990) may be encompassed by the proximal element of the perched springline (slope) model. In addition, comparable descriptive terms have been applied to cascade or cascade-like tufa deposits including waterfalls and thin cliff deposits and screens (e.g. Faulkner and Crae, 2018).
In the future, the resumption of the term “cascade” describing this specific macrostructure is suggested for further work in order to agree with terrestrial carbonate and tufa nomenclature, with macrostructures such as cron and remora applied as qualifiers or subtypes.
4.2.2 Barrage
Barrages are the most distinctive and best morphologically described of rock coast microbialite deposits. They range in size from small barrage pools <1 m diameter (or inter-dam distance, Pentecost, 2005) with walls of ca. 15 cm height up to large pools 1–5 m in diameter with walls of ca. 20 cm height at Canal Rocks and Quarry Bay, Western Australia, respectively (Forbes et al., 2010). At South African localities, barrages are of comparable sizes, with small barrage pools (<1 m diameter) at Schoenmakerskop (Perissinotto et al., 2014) and larger barrage pools of up to 5–7 m diameter and up to 1 m depth and with extensive lateral growth of the barrage up to 1 m thick at Seaview and surrounding localities (Edwards et al., 2017)
In rock coast microbialite nomenclature, barrage deposits (Fig. 5f–h) have been relatively consistently described as such. Perissinotto et al. (2014) and Edwards et al. (2017) describe barrage pools with rimstone dams (Edwards et al., 2017, consider rimstone itself to be a distinct mesostructure). Edwards et al. (2017) also distinguish between “back pools” and “front pools” based upon their position in relation to sea level and morphology. In relation to morphology, back pools form due to microbialite growth on both the pool bottom and margins, forming rims that ultimately close the pool, and front pools form with limited growth at the rims. Edwards et al. (2017) ascribe the morphology of these pools to their level of maturity; however, this morphogenetic classification is yet to be applied outside of South Africa. Cooper et al. (2022) also describe the barrage pool morphology as part of a lumped rock pool and barrage pool facies, combining barrage pool and lacustrine crust macrostructures. The barrage macrostructure is not exclusive to this depositional facies and may also be present on shore-platform surfaces and merge with cascade macrostructures in cliff face environments.
Pentecost and Viles (1994) differentiate barrages from cascades based upon vertical accretion of “barrages” resulting in water impoundment and forming “barrage pools”, of which two forms are recognised. The first form comprises those forming large lake barrages on obstructions or breaks in gradient on the accretion surface, and the second comprises barrage systems that form on pre-existing travertine s.l. slopes. Ford and Pedley (1996) describe the barrage model following the fluvial barrage model of Pedley (1990). Pentecost (2005) re-describes barrages, stating that the term barrage suggests a specific obstruction, and applies the term “dam” instead. Dams are divided into two groups based upon the distance between consecutive dams (or inter-dam distance), with dam systems with an inter-dam distance of 1 cm–1 m described as minidams and those with inter-dam distances of 1–100 m termed macrodams. However, this division is purely artificial with no biomodality or discontinuity between forms (Pentecost, 2005). In addition to tufa barrages, similar barrage-like morphologies occur in other terrestrial carbonates such as speleothem deposits, where the approximate terms “rimstone” and “gours” are applied, with “microgours” used to refer to morphologies at the centimetre scale, and travertine s.s. and silica sinter, where they are termed terraces (Hammer et al., 2010; Pentecost, 2005). A scaled approach to barrage terminology is also applied by Fouke et al. (2000) and Bargar (1978), who use terraces, terracettes, and microterraces to describe travertine morphologies of Yellowstone National Park, USA.
For further work, the term “barrage” or the term “barrage pool” is suggested in line with both terrestrial carbonate and rock coast microbialite nomenclature, with constituents of a barrage, the outer wall of the structure, backed by a barrage pool. The morphogenetic classification of “front” and “back” barrage pools of Edwards et al. (2017) may be applied as qualifiers if appropriate; however, these models should be further tested globally.
4.2.3 Fluvial crust and lacustrine crust
Fluvial and lacustrine crust macrostructures form some of the largest areas of rock coast microbialite of up to 10 m at sites between Seaview and Schoenmakerskop, South Africa (Edwards et al., 2017). However, these deposits are also characterised by being thin, with subaerial exposed fluvial crusts that are 3–5 cm thick at the Kei Mouth locality (Smith and Uken, 2003) and <10 cm at Eastern Cape localities, South Africa (Edwards et al., 2017); <4 cm thick inactive crusts at Western Australian localities (Forbes et al., 2010); and veneers of lacustrine crust as thin as 2–4 mm at the Giant's Causeway locality, Northern Ireland (Cooper et al., 2013).
However, fluvial and lacustrine crusts (Fig. 5d, e, i, and j) are not readily distinguished in rock coast microbialite nomenclature, with both forms being “lumped” within a singular setting or facies. Initially, Edwards et al. (2017) described “discharge aprons” as distinct macrostructures, describing microbialite growth typically <10 cm on inclined bedrock with flowing fresh water. Inactive and active discharge apron fans in Western Australia are also described by Forbes et al. (2010). This macrostructure is encompassed by the shore-platform environment described by Smith et al. (2018), with stromatolites accreting on the sub-horizontal element of rocky coasts, typically within rock pools of shore platforms. At the macrostructure level, they are simply described as being thin crusts (1–30 cm thick) in addition to low mounds, barrages, and oncoids, as described by Edwards et al. (2017). The shore-platform surface facies is also documented by Cooper et al. (2022) to describe rock coast microbialites that develop on the horizontal element of rock coasts, which are thin mats covered by a freshwater film.
Fluvial crusts, also called stream/spring crusts, are characterised by thin crust deposits under flowing water that intergrade with other macrostructures including cascades and barrages (Pentecost, 1993, 2005; Pentecost and Viles, 1994) as part of the fluviatile model of Pedley (1990). There is recognition that these deposits are frequently associated with cyanobacteria and algae (Pentecost, 1993, 2005). Ford and Pedley (1996) consider low-angle sheetlike deposits to be part of the distal component of the perched springline (slope) model and streambed deposits of cyanobacteria-dominated lenses and small bioherms of stromatolite boundstone to be part of the braided fluvial model. A comparable speleothem morphology is flowstone, which is considered analogous by Pentecost (2005); however the surface morphology/texture is smoother due to the lack of macrophyte growth and incorporation of detritus.
Microbial carbonates are considered a distinct lacustrine carbonate facies, identified by MISSs and “carbonate biostructures” that include coated grains (e.g. ooids and oncoids), other microbialites (e.g. stromatolites, thrombolites, and other microbial mats), and tufa mounds (Gierlowski-Kordesch, 2010). These are separated from fluvial crusts by Pentecost (2005), who divides lake deposits into lacustrine crusts and lacustrine reefs. The former, lacustrine crusts, have been defined similarly to fluvial crusts, consisting of superficial crusts and oncoids in static bodies of water (Ford and Pedley, 1996; Pentecost and Viles, 1994). The latter reef-like deposits are also classified in this lacustrine environment, with acknowledgement that such reefs are commonly laminated and microbially precipitated, i.e. stromatolitic (Ford and Pedley, 1996; Pentecost and Viles, 1994). This recognition of microbial influence has led to the term “krustenstein” (Kann, 1941), which has been applied to tufa/marl microbialite crusts (Doddy et al., 2019; Pentecost, 1981).
There is a clear discrepancy between the terminology applied to rock coast tufa microbialite macrostructure and the equivalent tufa morphologies, based upon the unusual setting in which rock coast microbialites form, with the environment (e.g. shore platform or discharge apron) frequently adopted as a macrostructure reserved here for fluvial and lacustrine crusts. Fluvial crusts should be applied to thin crusts with no water impoundment from barrages with flowing fresh water, while lacustrine crusts should be applied to crusts beneath predominantly no flow in a topographically controlled basin (e.g. a rock pool) (Fig. 5i and j). In reality these two macrostructures may form a gradient covering a range of hydrodynamic conditions on a sub-horizontal surface (e.g. on a shore-platform surface) and may grade into other macrostructures such as barrages.
4.3 Mesostructure
Mesostructure, according to Grey and Awramik (2020), refers to visible internal organisation and is the hierarchical level that allows for the differentiation of microbialites into stromatolites, thrombolites, dendrolites, and leiolites. In living microbial mats, the mesostructure has also been termed “mat topography” and refers to the surface features as opposed to the visible internal organisation (Bauld, 1992, in Grey and Awramik, 2020) with “architecture” proposed as a fossil equivalent (Grey and Awramik, 2020). The internal organisation of microbialites has been referred to as macrofabric (e.g. Riding, 2011) and mesofabric (e.g. Edwards et al., 2017); however, Grey and Awramik (2020) suggest that fabric is best used in its sedimentological meaning as a constituent of microstructure.
The first mat topography mesostructure of rock coast microbialites was described by Smith and Uken (2003) as coalescing domal structures, now termed colloform, and following this initial description, Smith et al. (2005) examined the laminated internal organisation further. The first substantial observation of mesostructure was undertaken by Smith et al. (2011), who described three growth forms: pustular, laminar/columnar, and colloform. Described as mesofabrics, Perissinotto et al. (2014) also observed these three forms on the South African coast, and Cooper et al. (2013) compared these to occurrences in Northern Ireland, describing colloform and laminar forms. This was greatly expanded upon by Edwards et al. (2017), who also classified rimstone, root casts, wrinkles, and blister mat mesostructures. Comparably with surface-cemented rudites, or beachrock, the root cast mesostructure, forming at vegetated cascades and resulting in the precipitation of carbonate on roots, is not explicitly biogenic and cannot be included within a classification of rock coast microbialite mesostructure. In the description of the mesostructure of rock coast microbialites, both the growth pattern (visible internal organisation) and the surface morphology (mat topography) have been considered, in some cases mutually exclusively.
The surface morphology of microbial mats and single-taxon microbial colonies can be classified. Whitton et al. (2000) classify the shape of cyanobacterial colonies as spherical/subspherical, hemispherical, cube, plate, clathrate, floc/amorphous, tuft/bushy, film/mat, and crust/fleck. These colonies may form part of the greater surface morphology of microbialites and demonstrate the biological control on mesostructure; this, in combination with the equal environmental control, is the genesis of mesostructure (Suosaari et al., 2019a; Trompette, 1982). Mesostructure is frequently described and classified on a case-study basis; for example, microbialites at Shark Bay, Western Australia, are classified based upon the surface morphology and degree of lamination. Pustular, smooth, colloform, and cerebroid morphologies have been identified (Jahnert and Collins, 2011, 2012; Logan et al., 1974; Suosaari et al., 2019b). However, as noted by Jahnert and Collins (2012), classification based on surface morphology may not be useful regarding the fabric and internal morphologies due to complex or compound microbialites with possible growth hiatuses. The same terms, pustular, smooth, and colloform, have also been applied to Exuma Cays and Highborne Cay, The Bahamas (Reid et al., 1995; Stolz et al., 2009), and to the external biofilm surface of tufa deposits (e.g. Perri et al., 2012).
The term mat topography has been applied to the surface mesostructure of living microbial mats (Bauld, 1992; Grey and Awramik, 2020). Rock coast microbialite terminology has used a wide variety of terms to describe both the mat topography and the internal organisation/laminar profile, with terms often being combined (e.g. the laminar flat mesostructure of Edwards et al., 2017); however, care should be taken so as not to consider these two aspects of mesostructure mutually exclusive unless there is sufficient evidence. This may be addressed through the application of the hierarchical mega- to microstructure approach. The following classification distinguishes between mat topography (visible from the exterior) and internal organisation (which requires examination in section) (Fig. 6).
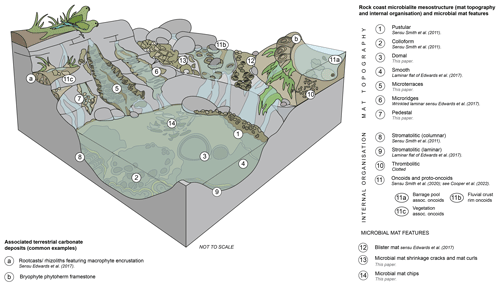
Figure 6Block diagram of rock coast microbialite mesostructures, including mat topography (surface morphology), internal organisation, and microbial mat features, and common associated features of terrestrial carbonate deposits.
4.3.1 Mat topographies: smooth, pustular, colloform, and domes
The terms smooth, pustular, and colloform have each been applied to describe mat topographies of rock coast microbialites.
A laminar mesostructure was initially identified as LLH-C (see Logan et al., 1964) in the wind-shadow margins of pools by Smith et al. (2011). This “laminar flat” mesostructure was also identified by Edwards et al. (2017) and Smith et al. (2018) as continuous stromatolite growth forming in the wind-shadow margins of shallow pools, discharge aprons, and ephemerally wet environments (Fig. 7e). It is similar to the “flat sheet” form of microbial laminates described by Forbes et al. (2010). Smooth mesostructure is associated with a flat laminar internal fabric (Edwards et al., 2017; Smith et al., 2011); however, as with other mat topographies, the relationship with internal structure is not well resolved. Comparable smooth surface morphologies from Shark Bay have been described as “flat, smooth surfaces with a beige colour either as mats or sub-spherical heads” (Jahnert and Collins, 2011), with a laminated fabric and smooth mats in peritidal zones (Gerdes and Krumbein, 1994), and from Highborne Cay, The Bahamas, as smooth mats (Stolz et al., 2009). The term “smooth” is frequently applied to microbialites and microbial mats and is recommended for further use.
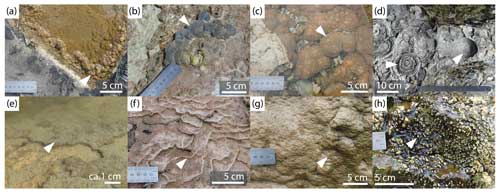
Figure 7Rock coast microbialite mat topographies (mesostructure): (a) pustular (Streedagh, Ireland); (b) colloform (Quarry Bay, Western Australia); (c) colloform (Lauries Bay, South Africa); (d) domal (Conto Spring, Western Australia); (e) smooth (Mullaghmore, Ireland); (f) microterraces (Sligo Bay, Ireland); (g) microridges (Bundoran, Ireland); (h) pedestal (Sligo Bay, Ireland).
A pustular mesostructure was initially described in the rock coast environment by Smith et al. (2011) as partially emergent, surrounding pool rims and shallow water, and has subsequently been identified frequently (Edwards et al., 2017; Smith et al., 2018) (Fig. 7a). Edwards et al. (2017) further define the pustular mesostructure as being formed of “small (0.5–2 cm wide) irregular shaped nodules which often grow on discharge aprons or at shallow margins of barrage pools”. A comparable pustular mesostructure from Shark Bay, Western Australia, was first describe by Logan et al. (1974) as “brown surfaces of gelatinous pustules composed of mucilage (1–2 cm thick)” (Jahnert and Collins, 2011) from the shallow intertidal environment. The pustular morphology is described as having a clotted fabric and is therefore thrombolitic (Jahnert and Collins, 2012). It has also been described from occurrences in normal-salinity marine environments in Exuma Cays, associated with Schizothrix mats (Reid et al., 1995), and Highborne Cay, The Bahamas, associated with Schizothrix and Solentia mats and biofilms (Stolz et al., 2009); from hypersaline Rivularia-rich microbial mats in Laguna Negra, Argentina (Gomez et al., 2018; Mlewski et al., 2018); and from occurrences in peritidal environments (Gerdes and Krumbein, 1994). The term “pustular” as a mat topography mesostructure is recommended with respect to rock coast microbialites to describe the upper surface of microbial mats.
The colloform mesostructure was also initially described by Smith et al. (2011) from deeper water than the pustular mesostructure to depths of 20–30 cm (Fig. 7b and c). The colloform mesostructure has been frequently described (Cooper et al., 2013; Edwards et al., 2017; Smith et al., 2018). Edwards et al. (2017) also consider colloform mesostructure to be depth-controlled, being found in barrage pools and on pool walls. It is defined as having “an interconnected bulbous appearance similar to that of malachite” (Edwards et al., 2017). This colloform mesostructure is similar to the phytohermal “bubble form” from Western Australian microbial laminates described by Forbes et al. (2010). Based upon the pustular and colloform mesostructures west of Seaview, South Africa, Edwards et al. (2017) conclude that a depth-controlled continuum exists between the two growth morphologies. The colloform mesostructure is described as “beige to brown elongate prismatic, spherical and club shaped structures” with each hemispherical head being 1–5 cm in size (Jahnert and Collins, 2011) based on subtidal material from Shark Bay, Western Australia. The colloform mesostructure is well understood and used within other microbialite literature and so is suitable for further use.
Larger interconnected hemispherical structures with heads of up to 15 cm in diameter have been observed between Cape Freycinet and Conto Spring, Western Australia, at an inactive microbialite barrage pool (Fig. 7d). While their morphology is comparable to colloform, the structures are much larger and could be considered a distinct mesostructure/morphology. Indeed, as with pustular and colloform, it is likely that a continuum exists between these mesostructures. The term “domal” is suggested although this does not refer to MISSs such as gas domes and mat expansion structures forming from gas accumulation (e.g. microbial mat decay; Bouougri et al., 2007).
4.3.2 Mat topographies: microterrace, microridge, and pedestal
Edwards et al. (2017) described “wrinkles” as “small (1–5 cm wide) drooping layers” forming on a sub-vertical faces that merge with a laminar flat mesostructure, potentially controlled by gradient and/or water flow velocity (Fig. 7g). In addition to these formally described wrinkle structures, similar structures have been observed to merge with microterrace structures at localities in County Sligo, Ireland, and Eastern Cape, South Africa (Fig. 7f). The terminology for terrace or barrage structures is complex, as discussed when examining the barrage macrostructure, but might be resolved by separate consideration of the macro- and mesostructure. At the mesoscale and centimetre scale, speleothem deposits have been termed “microgours” (Hammer et al., 2010), corresponding to “microterrace” for travertine s.s. (Bargar, 1978; Fouke et al., 2000) and “minidam” for travertine s.l. (Pentecost, 2005). Microterraces and microridges are terms applied predominantly to travertine s.l. and commonly travertine s.s. and speleothems (Pentecost, 2005). Hammer et al. (2010) recognise that on travertine s.l. deposits, where the gradient is so steep that backing pools do not form, a gradation into “microridges” normal to the flow may form. These microridges are considered here to be analogous with the wrinkles described by Edwards et al. (2017). It is recommended that the terms microterraces and microridges be applied to rock coast microbialite nomenclature, in line with other terrestrial carbonate literature, and the term wrinkles be abandoned.
Similarly, on sub-vertical surfaces, a distinct “pedestal”-like topography is apparent, where small groundwater channels flow over the tufa surface, with growth of island-like pedestals in between channels (Fig. 7h). Although this is an uncommon structure, it has been observed across a very wide geographic range with locations at the Eastern Cape, South Africa; Western Australia; and Ireland.
4.3.3 Internal organisation: stromatolites, thrombolites, and leiolites
The dominant internal organisation of rock coast microbialites is laminated, and, as such, they are stromatolites (Figs. 8a–c and 9a–c). The lamination of South African stromatolites has been examined by Smith et al. (2005, 2011), describing laminated (LLH-C; Logan et al., 1964) and columnar (SH-C) mesostructure, and later by Edwards et al. (2017) and Smith et al. (2018), recognising columnar mesostructure.
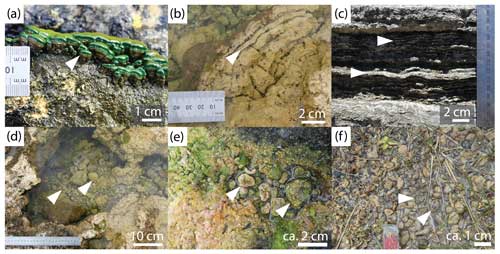
Figure 8Rock coast microbialite internal organisation (mesostructure): (a) stromatolitic columns coalescing to form colloform mat topography (Bundoran, Ireland); (b) stromatolitic (laminar) internal organisation of a colloform stromatolite (Lauries Bay, South Africa); (c) stromatolite (laminar) barrage (Quarry Bay, Western Australia); (d) barrage-pool-associated oncoids; (e) fluvial crust with small oncoids and proto-oncoids (Sligo Bay, Ireland); (f) vegetation-associated oncoids (Islay, Scotland).
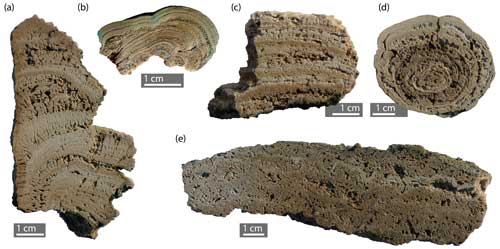
Figure 9Example sections of internal organisation (scale bar: 1 cm): (a) composite microbialite (Lauries Bay, South Africa); (b) top of columnar stromatolite (from field photograph: Fig. 8a) (Bundoran, Ireland); (c) barrage-pool-associated oncoid (from field photograph: Fig. 8d) (Lauries Bay, South Africa); (d) stromatolite (laminar) (Aughris Head, Ireland); (e) thrombolite with macro-laminae partially preserved (Sligo Bay, Ireland).
Smith et al. (2011) described the rimstone mesostructure and the rimstone morphology, the latter of which was subsequently recognised by Edwards et al. (2017) as a bioturbated irregular morphology with a matted fabric that forms at barrage rims. Edwards et al. (2017) explicitly state that lamination is not present. Rimstone tufa was also described from the barrage rims of Western Australian localities (Forbes et al., 2010). The rimstone mesostructure is seen as intrinsically linked to the barrage macrostructure; however, these two structures are not mutually exclusive. The well-laminated stromatolitic internal organisation within the barrage macrostructure at Quarry Bay, Western Australia, for example, indicates that rimstone mesostructures are not exclusively thrombolitic.
This thrombolitic internal organisation has multiple geneses related to the trapping and binding of peloids (Castro-Contreras et al., 2014); the morphology of the microbialite builders (Kennard and James, 1986); and the disruption, destruction, and alteration of stromatolitic internal organisation by biota, i.e. bioturbation (Walter and Heys, 1985). The latter has been suggested as a mechanism for the formation of thrombolites in South African rock coast microbialites, predominantly by metazoans and potentially by foraminifera and coccoids (Dodd et al., 2021; Weston et al., 2018). It may also be involved in the genesis of leiolitic (sensu Braga et al., 1995) or structureless mesofabrics (Dodd et al., 2021).
While stromatolitic, thrombolitic, and leiolitic internal organisation has been observed in the rock coast environment, future work is required to describe these structures and further subsets (e.g. dendrolites) before they can be confidently attributed to processes.
4.3.4 Internal organisation: oncoids and proto-oncoids
Oncoids from rock coast environments have not been comprehensively described; however, Smith et al. (2020) describe clasts entirely encrusted (oncoid) and partially encrusted (proto-oncoid) from rock pools on shore platforms at Cape Morgan, South Africa. These are described by Cooper et al. (2022) as a rock coast microbialite facies. These brief accounts describe barrage-pool-associated oncoids: oncoids that form in the barrage pool basin of variable degrees of encapsulating lamination around a cortex (spherical to irregular debris) (Figs. 8d and 9d). In addition, oncoids form in other supratidal groundwater environment, with variable morphologies and characteristic environments. Fluvial crust “rim” oncoids are oncoids and proto-oncoids with tonsure-like rims that form around debris on fluvial crusts on bare bedrock (Fig. 8e). Proximally to the groundwater spring efflux, vegetation-associated oncoids form in paludal conditions, commonly associated with vegetation (e.g. Phragmites australis in the Eastern Cape, South Africa) (Fig. 8f).
Oncoids are defined by Grey and Awramik (2020) as “unattached, generally spherical to ovoidal, stromatolite with a cortex of encapsulating or nearly encapsulating laminae”. They are considered a form of tufa or skeletal microbialite and, as such, have distinct biofabrics (Pentecost, 2005), frequently formed by cyanobacteria, in which case they may be termed cyanoid (Riding, 1991). The term proto-oncoid has been applied to clasts with partially encapsulating laminae although this term is not widely applied (e.g. Andrews and Trewin, 2014; Rodríguez and Cózar, 1999). They form within fluvial and lacustrine environments alongside other microbialite constituents such as microbial crusts and are commonly considered to be indicators of agitated water, with the turbulence rotating and overturning cortexes forming encapsulating lamination. However, in situ growth of oncoids has also been described (Lencina et al., 2023). As such, oncoids are frequently described as a constituent of terrestrial carbonate models or classification schemes such as the braided fluviatile model (Pedley, 1990) and fluvial and lacustrine crust (Pentecost and Viles, 1994). While these are considered in this morphological classification of rock coast microbialites to equate to macrostructures, oncoids are frequently described as having macro- and mesostructure (e.g. Casanova, 1994; Lencina et al., 2023; Villafañe et al., 2021); hence, a flexible approach to the structural level should be considered.
4.3.5 Microbial mat features
Microbial mats are ubiquitous in the rock coast environment, and observations to date show that a spectrum of MISSs, microbial mat features, and stromatolite mesostructures are present on rock coasts and they exhibit varying degrees of stacking/accretion and mineral precipitation. Environmental interactions with microbial mats and the exposed surface mat topographies of microbialites in the rock coast environment result in a variety of features that are poorly described and understood.
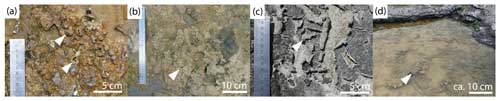
Figure 10Rock coast microbialite mat features: (a) blister mat (Quarry Bay; Western Australia); (b, c) microbial shrinkage cracks and mat curls (Sligo Bay, Ireland); (d) floating microbial mat chips of lacustrine crust following detachment from substrate (Sligo Bay, Ireland).
Few microbial mat features have been described explicitly from rock coast microbialites; however, the “blister mat” mesostructure described by Edwards et al. (2017) as “1–3 cm wide dome-like structures [that] appear to have burst open at the top resulting in a surface morphology that resembles blistering” may be considered to resemble burst gas domes (Fig. 10). Blister mats accrete on low-gradient substrates subject to frequent subaerial exposure (Dodd et al., 2021; Edwards et al., 2017), and Edwards et al. (2017) suggest that their formation is a result of subaerial exposure of microbial mats, splitting domal structures and releasing trapped gases produced by the mat decomposition, as described by Gerdes and Krumbein (1994). These structures are similar to the gas domes described by Noffke (2010), which can blister or collapse, releasing the trapped gas and resulting in a crater-like morphology. These structures may be recolonised by microbial mats (Lakhdar et al., 2020), potentially resulting in laminoid and irregular fenestrae in section as suggested by Dodd et al. (2021). Features like the blister mat mesostructure occur as modifications to microbial mats with notable carbonate precipitation and as such are not MISSs; however, similar environmental interactions result in similar superficial similarities.
Smith et al. (2020) describe wave rip-up clasts of stromatolite within rock pools and areas stabilised by vegetation in a shore-platform environment on the South African coast that form due to increased wave energy and/or water levels during storm conditions. These deposits may accumulate and form an allochthonous tufa microbialite breccia (Cooper et al., 2022; Smith et al., 2020). These wave rip-up clasts are common on shore-platform surfaces where deposits of laminar stromatolites or microbial mats are most common (Cooper et al., 2022; Smith et al., 2020) (Fig. 10d). The descriptions of these features are similar to MISSs due to comparable environmental interactions; however, due to mineral precipitation, they cannot be classified as such. These should instead be regarded as microbial mat features. Wave rip-up clasts are comparable to MISS microbial mat chips, referring to chip and flake-shaped centimetre-sized fragments detached by mechanical erosion of a parent microbial mat through water agitation, predominantly by waves or bottom currents during tides or storm events as well as potentially wind action (Eriksson et al., 2007; Noffke et al., 2001).
While microbial mat chips from rock coast microbialite systems have been described, microbial shrinkage cracks, also called synaeresis cracks or desiccation cracks, despite their ubiquity, have not. Microbial mat shrinkage cracks are a “surface of thin microbial mats marked with isolated lenticular, sinuously curved and even subcircular cracks, spindle shaped and tri-radiate shrinkage cracks” (Eriksson et al., 2007) formed due to subaerial desiccation in the upper intertidal to lower supratidal zones of tidal flats (Eriksson et al., 2007). These cracks may have curled margins that may mature to flipped-over edges and eventually may become a rolled-up mat fragment (Fig. 10b and c). Shrinkage cracks and mat curls of carbonate-precipitating microbial mats have not yet been formally described in detail from rock coast environments; however, their presence warrants mentioning in this scheme.
Environmental interactions with potential MISSs, microbial mats, and microbialites in the rock coast environment are currently poorly described and poorly understood. Future research into these features, the relationship between these and the more common mat topographies, and their genesis is required.
Rock coast microbialite research has expanded greatly in the last 20 years, with the terminology for characterisation borrowed from both microbialite and terrestrial carbonate nomenclature. Therefore, an integrated rock coast microbialite classification is essential in future research on rock coast microbialite systems. We have considered the existing approaches and propose a new integrated geomorphological terminology and classification scheme to promote future consistency. This classification addresses the presence of synonyms, duplications, and omissions and the wide variety of terms applied to the same morphological features, predominantly at the macro- and mesoscale, and it is approached through a hierarchical scale. We propose the following guidelines.
The terminology and overarching classification proposed here are focused on the geomorphology of macrostructures and mesostructures. The review of the literature, however, reveals that drivers and relationships between them are less well understood (e.g. testing and resolving the relationships between mat topography and internal organisation). In addition to this, better qualitative and quantitative geomorphological description is required at the macro- and mesoscale.
Establishing biogenicity of rock coast microbialites and associated carbonate deposits (e.g. beachrock and rhizoliths) is also crucial, considering the genetic definition of microbialites. It is acknowledged that the degassing of carbon dioxide inorganically can contribute to tufa microbialite mineralisation, alongside biomineralisation; the respective contribution of both carbonate-forming mechanisms is not well understood with respect to different macro- and mesostructures. Greater understanding of this may allow for better integration with both terrestrial carbonate and microbialites.
Understanding the global distribution and variability in rock coast microbialites also requires further research, with consideration of drivers such as groundwater seep chemistry, geology/topography, and microbialite builders in order to better understand the microbialites' morphogenesis. Since some of the more intricate drivers of microbialite genesis, especially regarding rock coast microbialites, are currently only partly understood, this classification scheme is out of necessity based on morphology rather than morphogenesis. While this is appropriate for and relevant to the interpretation of rock coast microbialites in the fossil record where the specific drivers cannot be easily observed, better understanding of the current drivers in their formation would make comparisons to deep-time microbialites more meaningful.
The strong focus on British and north-west European studies on tufa and travertine, resulting in a study bias predominantly in cool temperate climates, does not sufficiently consider other (e.g. tropical) climates. This greatly impacts the establishment of classification schemes and diminishes their global value. A global view of rock coast microbialite classification relies upon the detailed description, study, and comparison of localities around the globe. As new rock coast microbialite localities are inevitably discovered and described, consistency in their description and the terminology applied is vital for advances in our understanding of these deposits.
No data sets were used in this article.
TWG proposed, developed, and wrote the manuscript draft, with contributions from JAGC, AMS, GMR, and MF.
The contact author has declared that none of the authors has any competing interests.
Publisher's note: Copernicus Publications remains neutral with regard to jurisdictional claims made in the text, published maps, institutional affiliations, or any other geographical representation in this paper. While Copernicus Publications makes every effort to include appropriate place names, the final responsibility lies with the authors.
The authors would like to thank the Extant Peritidal Stromatolite Network (EPStromNet) team and the Supratidal Spring-Fed Living Microbialite Ecosystems (SSLiME) team for in-field discussion and support, notably, Graham J. C. Underwood, Carla Dodd, and Tristin O'Connell as well as Joerg Arnscheidt.
This review was made possible through Department for the Economy (DfE) sponsorship for a postgraduate studentship, with the support of an EPStromNet – Extant Peritidal Stromatolite Network – NERC grant award (NERC reference NE/V00834X/1) and a Quaternary Research Association (QRA) New Research Workers Grant.
This paper was edited by Tina Treude and reviewed by two anonymous referees.
Andrews, S. D. and Trewin, N. H.: Palaeoenvironmental significance of lacustrine stromatolite forms from the Middle Old Red Sandstone of the Orcadian Basin, Geol. Mag., 151, 414–429, https://doi.org/10.1017/S0016756813000290, 2014.
Aref, M. A. M.: Holocene stromatolites and microbial laminites associated with lenticular gypsum in a marine-dominated environment, Ras El Shetan area, Gulf of Aqaba, Egypt, Sedimentology, 45, 245–262, https://doi.org/10.1046/j.1365-3091.1998.00136.x, 1998.
Arenas, C., Gutiérrez, F., Osácar, C., and Sancho, C.: Sedimentology and geochemistry of fluvio-lacustrine tufa deposits controlled by evaporite solution subsidence in the central Ebro Depression, NE Spain, Sedimentology, 47, 883–909, https://doi.org/10.1046/j.1365-3091.2000.00329.x, 2000.
Arenas, C., Osácar, M. C., Sancho, C., Vázquez-Urbez, M., Auqué, L., and Pardo, G.: Seasonal record from recent fluvial tufa deposits (Monasterio de Piedra, NE Spain): Sedimentological and stable isotope data, Geol. Soc. Lond. Spec. Publ., 336, 119–142, https://doi.org/10.1144/SP336.7, 2010.
Arenas, C., Osácar, M. C., Auqué, L. F., and Sancho, C.: Coupling textural and stable-isotope variations in fluvial stromatolites: Comparison of Pleistocene and recent records in NE Spain, J. Palaeogeogr., 8, 13, https://doi.org/10.1186/s42501-019-0021-y, 2019.
Bargar, K. E.: Geology and Thermal History of Mammoth Hot Springs, Yellowstone National Park, Wyoming, Geology and Thermal History of Mammoth Hot Springs, Yellowstone National Park, Wyoming, U. S. Geological Survey, https://doi.org/10.3133/b1444, 1978.
Bauld, J.: 8. Occurrence of benthic microbial mats in saline lakes, Hydrobiologia, 81, 87–111, https://doi.org/10.1007/BF00048708, 1981.
Bauld, J.: Modern microbial mats, in: Evolution of the Proterozoic biosphere: a multidisciplinary study, vol. 259, Cambridge University Press, New York, 250–252, https://doi.org/10.1017/CBO9780511601064, 1992.
Bose, S. and Chafetz, H.: Topographic control on distribution of modern microbially induced sedimentary structures (MISS): A case study from Texas coast, Sediment. Geol., 213, 136–149, https://doi.org/10.1016/j.sedgeo.2008.11.009, 2009.
Bose, S. and Chafetz, H. S.: Morphology and Distribution of Miss: A Comparison Between Modern Siliciclastic and Carbonate Settings, in: Microbial Mats in Siliciclastic Depositional Systems Through Time, vol. 101, edited by: Noffke, N. and Chafetz, H., SEPM Society for Sedimentary Geology, 101, 3–14, https://doi.org/10.2110/sepmsp.101.003, 2012.
Bosence, D., Gibbons, K., Le Heron, D. P., Morgan, W. A., Pritchard, T., and Vining, B. A.: Microbial carbonates in space and time: introduction, Geol. Soc. Lond. Spec. Publ., 418, 1–15, https://doi.org/10.1144/SP418.14, 2015.
Bouougri, E., Gerdes, G., and Porada, H.: 6(c). Inherent problems of terminology: definition of terms frequently used in connection with microbial mats, in: Atlas of Microbial Mat Features Preserved within the Siliciclastic Rock Record, Elsevier, Amsterdam, 8, eBook ISBN 9780080549309, 2007.
Braga, J. C., Martin, J. M., and Riding, R.: Controls on Microbial Dome Fabric Development along a Carbonate-Siliciclastic Shelf-Basin Transect, Miocene, SE Spain, Palaios, 10, 347–361, https://doi.org/10.2307/3515160, 1995.
Brunet, R. and Revuelta, M. Á.: Exceptional silica speleothems in a volcanic cave: A unique example of silicification and sub-aquatic opaline stromatolite formation (Terceira, Azores), Sedimentology, 61, 2113–2135, https://doi.org/10.1111/sed.12130, 2014.
Burne, R. and Moore, L.: Microbialites: Organosedimentary Deposits of Benthic Microbial Communities, Palaios, 2, 241–254, https://doi.org/10.2307/3514674, 1987.
Cameron, B., Cameron, D., and Jones, J. R.: Modern Algal Mats in Intertidal and Supratidal Quartz Sands, Northeastern Massachusetts, U.S.A., in: Biogenic Structures: Their Use in Interpreting Depositional Environments, vol. 35, edited by: Curran, H. A., SEPM Society for Sedimentary Geology, 35, 211–223, https://doi.org/10.2110/pec.85.35.0211, 1985.
Cao, R. and Bian, L.: An attempt to codify the morphological characteristic numbers of stromatolites, Acta Palaeontol. Sin., 25, 629–634, 1985.
Capezzuoli, E., Gandin, A., and Pedley, M.: Decoding tufa and travertine (fresh water carbonates) in the sedimentary record: The state of the art, Sedimentology, 61, 1–21, https://doi.org/10.1111/sed.12075, 2014.
Casanova, J.: Stromatolites from the East African Rift: A Synopsis, in: Phanerozoic Stromatolites II, edited by: Bertrand-Sarfati, J. and Monty, C., Springer Netherlands, Dordrecht, 193–226, https://doi.org/10.1007/978-94-011-1124-9_8, 1994.
Castro-Contreras, S. I., Gingras, M. K., Pecoits, E., Aubet, N. R., Petrash, D., Castro-Contreras, S. M., Dick, G., Planavsky, N., and Konhauser, K. O.: Textural and Geochemical Features of Freshwater Microbialites from Laguna Bacalar, Quintana Roo, Mexico, Palaios, 29, 192–209, 2014.
Caudwell, C., Lang, J., and Pascal, A.: Lamination of swampy-rivulets Rivularia haematites stromatolites in a temperate climate, Sediment. Geol., 143, 125–147, https://doi.org/10.1016/S0037-0738(00)00191-3, 2001.
Cooper, A., Smith, A., and Arnscheidt, J.: Contemporary stromatolite formation in high intertidal rock pools, Giant's Causeway, Northern Ireland: Preliminary observations, J. Coastal Res., 65, 1675–1680, https://doi.org/10.2112/SI65-283.1, 2013.
Cooper, A., Smith, A., Rishworth, G., Dodd, C., Forbes, M., Cawthra, H., and Anderson, C.: Microbialites of modern siliciclastic rock coasts, J. Sediment. Res., 92, 619–634, https://doi.org/10.2110/jsr.2021.071, 2022.
Della Porta, G.: Non-Marine Carbonates – Facies, Diagenesis and Porosity Development, #30173, https://www.searchanddiscovery.com/pdfz/documents/2011/30173dellaporta/ndx_dellaporta.pdf.html (last access: 29 October 2024), 2011.
Della Porta, G.: Carbonate build-ups in lacustrine, hydrothermal and fluvial settings: comparing depositional geometry, fabric types and geochemical signature, in: Microbial Carbonates in Space and Time: Implications for Global Exploration and Production, vol. 418, edited by: Bosence, D. W. J., Gibbons, K. A., Heron, D. P. L., Morgan, W. A., Pritchard, T., and Vining, B. A., Geological Society of London, 418, 17–68, https://doi.org/10.1144/SP418.4, 2015.
Dill, R. F., Shinn, E. A., Jones, A. T., Kelly, K., and Steinen, R. P.: Giant subtidal stromatolites forming in normal salinity waters, Nature, 324, 55–58, https://doi.org/10.1038/324055a0, 1986.
Dobat, K.: Die Kryptogamenvegetation der Höhlen und Halbbhöhlen im Bereich der Schwäbischen Alb, Abt. für Karst-Höhlenkd., 1–153, 1966.
Dodd, C.: A Geochemical Comparison of Southern African Stromatolites and Stromatolite Pools, Unpublished M.Sc. thesis, Nelson Mandela University, 2019.
Dodd, C., Anderson, C. R., Perissinotto, R., du Plooy, S. J., and Rishworth, G. M.: Hydrochemistry of peritidal stromatolite pools and associated freshwater inlets along the Eastern Cape coast, South Africa, Sediment. Geol., 373, 163–179, https://doi.org/10.1016/j.sedgeo.2018.06.002, 2018.
Dodd, C., Anderson, C. R., Rishworth, G. M., Perissinotto, R., and van Niekerk, X.: Metazoan activity facilitates passive sediment trapping in modern supratidal microbialites: Revealed using μ-CT-scanning and microscopy, Geobiology, 19, 585–600, https://doi.org/10.1111/gbi.12456, 2021.
Doddy, P., Roden, C. M., and Gammell, M. P.: Microbialite crusts in Irish limestone lakes reflect lake nutrient status, Biol. Environ., 119B, 1–11, https://doi.org/10.3318/bioe.2019.01, 2019.
Donaldson, J. A.: Stromatolites in the Denault Formation, Marion Lake, Coast of Labrador, Newfoundland, Bulletin Geological Survey of Canada Place, Ottawa, 102, 1–33, https://doi.org/10.4095/123903, 1963.
Dunham, R. J.: Classification of Carbonate Rocks According to Depositional Textures, in: Classification of Carbonate Rocks – A Symposium, edited by: Ham, W. H., American Association of Petroleum Geologists, 38, 108–121, https://doi.org/10.1306/M1357, 1962.
EC (European Commission): Interpretation Manual of European Union Habitats, version EUR 28, 2013.
EC (European Commission): C2.1b Calcareous spring and spring brook, Publications Office of the European Union, Luxembourg, 2016.
Edwards, M. J. K., Anderson, C. R., Perissinotto, R., and Rishworth, G. M.: Macro- and meso-fabric structures of peritidal tufa stromatolites along the Eastern Cape coast of South Africa, Sediment. Geol., 359, 62–75, https://doi.org/10.1016/j.sedgeo.2017.08.006, 2017.
Eriksson, P. G., Porada, H., Banerjee, S., Bouougri, E., Sarkar, S., and Bumby, A. J.: 4(c). Mat-destruction features, in: Atlas of Microbial Mat Features Preserved within the Siliciclastic Rock Record, Elsevier, Amsterdam, 47, eBook ISBN 9780080549309, 2007.
Farr, G. and Graham, J.: Survey, characterisation and condition assessment of Palustriella dominated springs “H7220 Petrifying springs with tufa formation (Cratoneurion)” in Gloucestershire, British Geological Survey, Report No.: OR/17/020, England, https://nora.nerc.ac.uk/id/eprint/518747 (last access: 31 October 2024), 2017.
Faulkner, T. and Crae, J.: Distribution of tufa and speleothem deposits on the island of Lismore, Argyll, Scotland, Cave Karst Sci., 45, 101–110, 2018.
Field, M.: A Lexicon of Cave and Karst Terminology with Special Reference to Environmental Karst Hydrology, Office of Research and Development, U.S. Environmental Protection Agency, Washington, DC, 221, https://lccn.loc.gov/2002435615 (last access: 31 October 2024), 2002.
Flügel, E.: Microfacies of Carbonate Rocks: Analysis, Interpretation and Application, Springer, Berlin, Heidelberg, https://doi.org/10.1007/978-3-642-03796-2, 2010.
Folk, R. L.: Practical Petrographic Classification of Limestones, AAPG Bull., 43, 1–38, https://doi.org/10.1306/0BDA5C36-16BD-11D7-8645000102C1865D, 1959.
Forbes, M., Vogwill, R., and Onton, K.: A characterisation of the coastal tufa deposits of south–west Western Australia, Sediment. Geol., 232, 52–65, https://doi.org/10.1016/j.sedgeo.2010.09.009, 2010.
Forbes, M., Rishworth, G., Underwood, G., Cooper, A., Garner, T., Hicks, N., Dodd, C., Mcgenity, T., Smith, A., Cawthra, H., Adams, J., and Clark, D.: Rocky coastline bio-mediated carbonate deposits: Quaternary archive, modern environmental stress indicator or both?, Australian Quaternary Association 2024, North Stradbroke Island, https://www.researchgate.net/publication/381850933_Rocky_coastline_bio-mediated_carbonate_deposits_Quaternary_archive_modern_environmental_stress_indicator_or_both (last access: 31 October 2024), 2024.
Ford, T. D. and Pedley, H. M.: A review of tufa and travertine deposits of the world, Earth-Sci. Rev., 41, 117–175, https://doi.org/10.1016/S0012-8252(96)00030-X, 1996.
Fouke, B. W., Farmer, J. D., Des Marais, D. J., Pratt, L., Sturchio, N. C., Burns, P. C., and Discipulo, M. K.: Depositional facies and aqueous-solid geochemistry of travertine-depositing hot springs (Angel Terrace, Mammoth Hot Springs, Yellowstone National Park, U.S.A.), J. Sediment. Res., 70, 565–585, https://doi.org/10.1306/2dc40929-0e47-11d7-8643000102c1865d, 2000.
Gandin, A. and Capezzuoli, E.: Travertine: Distinctive depositional fabrics of carbonates from thermal spring systems, Sedimentology, 61, 264–290, https://doi.org/10.1111/sed.12087, 2014.
Gerdes, G. and Krumbein, W. E.: Peritidal Potential Stromatolites – A Synopsis, in: Phanerozoic Stromatolites II, edited by: Bertrand-Sarfati, J. and Monty, C., Springer Netherlands, Dordrecht, 101–129, https://doi.org/10.1007/978-94-011-1124-9_5, 1994.
Gerdes, G., Klenke, T., and Noffke, N.: Microbial signatures in peritidal siliciclastic sediments: a catalogue, Sedimentology, 47, 279–308, https://doi.org/10.1046/j.1365-3091.2000.00284.x, 2000.
Gierlowski-Kordesch, E. H.: Chap. 1 Lacustrine Carbonates, in: Developments in Sedimentology, vol. 61, edited by: Alonso-Zarza, A. M. and Tanner, L. H., Elsevier, 1–101, https://doi.org/10.1016/S0070-4571(09)06101-9, 2010.
Gomez, F., Mlewski, C., Boidi, F., Farías, M., and Gérard, E.: Calcium Carbonate Precipitation in Diatom-rich Microbial Mats: The Laguna Negra Hypersaline Lake, Catamarca, Argentina, J. Sediment. Res., 88, 727–742, https://doi.org/10.2110/jsr.2018.37, 2018.
Gradziński, M.: Factors controlling growth of modern tufa: results of a field experiment, Geol. Soc. Lond. Spec. Publ., 336, 143–191, https://doi.org/10.1144/SP336.8, 2010.
Grey, K. and Awramik, S.: Handbook for the study and description of microbialites, Geological Survey of Western Australia: Bulletin 147, Perth, ISBN online 978-1-74168-861-0, print 978-1-74168-862-7, 2020.
Guo, L. and Riding, R.: Hot-spring travertine facies and sequences, Late Pleistocene, Rapolano Terme, Italy, Sedimentology, 45, 163–180, https://doi.org/10.1046/j.1365-3091.1998.00141.x, 1998.
Guo, X. and Chafetz, H.: Large tufa mounds, Searles Lake, California, Sedimentology, 59, 1509–1535, https://doi.org/10.1111/j.1365-3091.2011.01315.x, 2012.
Hall, J.: Cryptozoön, n.g.; Cryptozoön proliferum, nsp., New York State Museum of Natural History, 36th Annual Report of the Trustees, 1883.
Hammer, O., Dysthe, D., and Jamtveit, B.: Travertine terracing: patterns and mechanisms, in: Tufas and Speleothems: Unravelling the Microbial and Physical Controls, Geol. Soc. Lond. Spec. Publ., 336, 345–355, https://doi.org/10.1144/SP336.18, 2010.
Harwood Theisen, C. and Sumner, D. Y.: Thrombolite fabrics and origins: Influences of diverse microbial and metazoan processes on Cambrian thrombolite variability in the Great Basin, California and Nevada, Sedimentology, 63, 2217–2252, https://doi.org/10.1111/sed.12304, 2016.
Hill, C. and Forti, P.: Cave Minerals of the World, 2nd edn., National Speleological Society, Huntsville, ISBN-10 1879961075, 1997.
Hofmann, H. J.: Attributes of stromatolites, Dept. of Energy, Mines and Resources, Ottawa, 58 pp., 1969.
Howie, F. and Ealey, P.: Tufa and speleothem occurrence in north and west cornwall, Geosci. South-West Engl., 12, 109–114, 2009.
Howie, F. and Ealey, P.: An appraisal of Quaternary calcium carbonate deposits in Cornwall, Geosci. South-West Engl., 12, 233–240, 2010.
Ibarra, Y. and Corsetti, F.: Lateral Comparative Investigation of Stromatolites: Astrobiological Implications and Assessment of Scales of Control, Astrobiology, 16, 271–281, https://doi.org/10.1089/ast.2015.1388, 2016.
Jahnert, R. J. and Collins, L. B.: Significance of subtidal microbial deposits in Shark Bay, Australia, Mar. Geol., 286, 106–111, https://doi.org/10.1016/j.margeo.2011.05.006, 2011.
Jahnert, R. J. and Collins, L. B.: Characteristics, distribution and morphogenesis of subtidal microbial systems in Shark Bay, Australia, Mar. Geol., 303–306, 115–136, https://doi.org/10.1016/j.margeo.2012.02.009, 2012.
Jones, B. and Renaut, R. W.: Calcareous Spring Deposits in Continental Settings, in: Developments in Sedimentology, vol. 61, edited by: Alonso-Zarza, A. M. and Tanner, L. H., Elsevier, 177–224, https://doi.org/10.1016/S0070-4571(09)06104-4, 2010.
Kann, E.: Krustenstein in Seen, Arch. Hydrobiol., 37, 432–504, 1941.
Kennard, J. M. and James, N. P.: Thrombolites and Stromatolites: Two Distinct Types of Microbial Structures, Palaios, 1, 492–503, https://doi.org/10.2307/3514631, 1986.
Kennedy, D. M., Stephenson, W. J., and Naylor, L. A.: Introduction to the rock coasts of the world, in: Rock Coast Geomorphology: A Global Synthesis, The Geological Society, London, https://doi.org/10.1144/M40.1, 2014.
Lakhdar, R., Soussi, M., and Talbi, R.: Modern and Holocene microbial mats and associated microbially induced sedimentary structures (MISS) on the southeastern coast of Tunisia (Mediterranean Sea), Quaternary Res., 100, 1–21, https://doi.org/10.1017/qua.2020.91, 2020.
Lehmann, H.: Der Tropische Kegelkarst Auf Den Grossen Antillen, Erdkunde, 8, 130–139, 1954.
Lencina, A. I., Soria, M. N., Colla, M. F., Cury, L. F., Farías, M. E., and Gomez, F. J.: In situ growth of modern oncoids from Salado river, Salar de la Laguna Verde Complex, Argentina, Sediment. Geol., 451, 106396, https://doi.org/10.1016/j.sedgeo.2023.106396, 2023.
Lipar, M. and Webb, J.: The Middle–Late Pleistocene Bridgewater Formation on Cape Bridgewater, south-western Victoria: chronostratigraphy and palaeoclimatic significance, Proc. R. Soc. Vic., 127, 81, https://doi.org/10.1071/RS15020, 2015.
Logan, B. W.: Cryptozoon and Associate Stromatolites from the Recent, Shark Bay, Western Australia, J. Geol., 69, 517–533, https://doi.org/10.1086/626769, 1961.
Logan, B. W., Rezak, R., and Ginsburg, R. N.: Classification and Environmental Significance of Algal Stromatolites, J. Geol., 72, 68–83, 1964.
Logan, B. W., Read, J. F., Hagan, G. M., Hoffman, P., Brown, R. G., Woods, P. J., and Gebelein, C. D.: Evolution and Diagenesis of Quaternary Carbonate Sequences, Shark Bay, Western Australia, American Association of Petroleum Geologists, https://doi.org/10.1306/M22379, 1974.
Lundberg, J. and McFarlane, D. A.: Subaerial freshwater phosphatic stromatolites in Deer Cave, Sarawak – A unique geobiological cave formation, Geomorphology, 128, 57–72, https://doi.org/10.1016/j.geomorph.2010.12.022, 2011.
Lyons, M. and Kelly, D.: Monitoring Guidelines for the Assessment of Petrifying Springs in Ireland, National Parks and Wildlife Service, Department of Arts, Heritage, Regional, Rural and Gaeltacht Affairs, Ireland, ISSN 1393 – 6670, 2016.
Mackey, T. J., Sumner, D. Y., Hawes, I., Jungblut, A. D., and Andersen, D. T.: Growth of modern branched columnar stromatolites in Lake Joyce, Antarctica, Geobiology, 13, 373–390, https://doi.org/10.1111/gbi.12138, 2015.
Manzo, E., Perri, E., and Tucker, M. E.: Carbonate deposition in a fluvial tufa system: processes and products (Corvino Valley – southern Italy), Sedimentology, 59, 553–577, https://doi.org/10.1111/j.1365-3091.2011.01266.x, 2012.
Maslov, V. P.: Printsipy nomenklatury i sistematiki stromatolitov, Izv. AN SSSR Geol.+, 4, 105–112, 1953.
Maslov, V. P.: Stromatolity (ikh genezis, metod izucheniya svyaz's fatsiyami i geologicheskoe znachenie na primere ordovika sibiroskoy platformy), Tr. Akad. Nauk SSSR Geol. Inst., 41, 188, 1960.
Merz-Preiß, M. and Riding, R.: Cyanobacterial tufa calcification in two freshwater streams: ambient environment, chemical thresholds and biological processes, Sediment. Geol., 126, 103–124, https://doi.org/10.1016/S0037-0738(99)00035-4, 1999.
Mlewski, E. C., Pisapia, C., Gomez, F., Lecourt, L., Soto Rueda, E., Benzerara, K., Ménez, B., Borensztajn, S., Jamme, F., Réfrégiers, M., and Gérard, E.: Characterization of Pustular Mats and Related Rivularia-Rich Laminations in Oncoids From the Laguna Negra Lake (Argentina), Front. Microbiol., 9, https://doi.org/10.3389/fmicb.2018.00996, 2018.
Monty, C. L. V.: Chap. 5.1 The Origin and Development of Cryptalgal Fabrics, in: Developments in Sedimentology, vol. 20, edited by: Walter, M. R., Elsevier, 193–249, https://doi.org/10.1016/S0070-4571(08)71137-3, 1976.
Moore, L. S. and Burne, R. V.: The Modern Thrombolites of Lake Clifton, Western Australia, Dordrecht, in: Phanerozoic Stromatolites II, Springer, Dordrecht, 3–29, https://doi.org/10.1007/978-94-011-1124-9_1, 1994.
Mountain, E. D.: Tufa-lined basins in coastal platforms, S. Afr. J. Sci., 33, 242–247, https://hdl.handle.net/10520/AJA00382353_8109, 1937.
Noffke, N.: Turbulent lifestyle: Microbial mats on Earth's sandy beaches – Today and 3 billion years ago, GSA Today, 18, 4, https://doi.org/10.1130/GSATG7A.1, 2008.
Noffke, N.: Geobiology: Microbial Mats in Sandy Deposits from the Archean Era to Today, Springer Berlin, Heidelberg, 1 pp., https://doi.org/10.1007/978-3-642-12772-4, 2010.
Noffke, N. and Awramik, S. M.: Stromatolites and MISS – Differences between relatives, GSA Today, 23, 4–9, https://doi.org/10.1130/GSATG187A.1, 2013.
Noffke, N., Gerdes, G., Klenke, T., and Krumbein, W. E.: Microbially induced sedimentary structures – examples from modern sediments of siliciclastic tidal flats, Zbl. Geo. Pal., 1, 307–316, 1996.
Noffke, N., Gerdes, G., Klenke, T., and Krumbein, W. E.: Microbially Induced Sedimentary Structures: A New Category within the Classification of Primary Sedimentary Structures, J. Sediment. Res., 71, 649–656, https://doi.org/10.1306/2DC4095D-0E47-11D7-8643000102C1865D, 2001.
Onton, K., Clarke, V., and Wa, K.: Monitoring Protocol: Augusta Microbial Threatened Ecological Community Version 1.0, Department of Environment and Conservation, Western Australia, 2009.
Ordonez, S., Gonzales, J. A., and Garcia del Cura, M. A.: Pétrographie et morphologie des édifices tuffeux quaternaires du centre de l'Espagne, Méditerranée, 57, 52–60, https://doi.org/10.3406/medit.1986.2368, 1986.
Pedley, H. M.: Classification and environmental models of cool freshwater tufas, Sediment. Geol., 68, 143–154, https://doi.org/10.1016/0037-0738(90)90124-C, 1990.
Pedley, H. M. and Rogerson, M.: Introduction to tufas and speleothems, Geol. Soc. Lond. Spec. Publ., 336, 1–5, https://doi.org/10.1144/SP336.1, 2010.
Pedley, M., Andrews, J., Salvador, O., del-Cura, M. Á., Martin, J.-A., and Taylor, D.: Does climate control the morphologic fabric of freshwater carbonates? A comparative study of Holocene barrage tufas from Spain and Britain, Palaeogeogr. Palaeocl., 121, 239–257, https://doi.org/10.1016/0031-0182(95)00080-1, 1996.
Pedley, M., Rogerson, M., and Middleton, R.: Freshwater calcite precipitates from in vitro mesocosm flume experiments: a case for biomediation of tufas, Sedimentology, 56, 511–527, https://doi.org/10.1111/j.1365-3091.2008.00983.x, 2009.
Pentecost, A.: The tufa deposits of the Malham district, north Yorkshire, Field Stud., 5, 365–387, 1981.
Pentecost, A.: British travertines: a review, P. Geologist. Assoc., 104, 23–39, https://doi.org/10.1016/S0016-7878(08)80152-6, 1993.
Pentecost, A.: Travertine, Springer, https://doi.org/10.1007/1-4020-3606-X, 2005.
Pentecost, A. and Viles, H.: A Review and Reassessment of Travertine Classification, Geogr. Phys. Quatern., 48, 305–314, https://doi.org/10.7202/033011ar, 1994.
Perissinotto, R., Bornman, T. G., Steyn, P.-P., Miranda, N. A. F., Dorrington, R. A., Matcher, G. F., Strydom, N., and Peer, N.: Tufa stromatolite ecosystems on the South African south coast, S. Afr. J. Sci., 110, 01–08, https://doi.org/10.1590/sajs.2014/20140011, 2014.
Perri, E., Manzo, E., and Tucker, M.: Multi-scale study of the role of the biofilm in the formation of minerals and fabrics in calcareous tufa, Sediment. Geol., 263–264, 16–29, https://doi.org/10.1016/j.sedgeo.2011.10.003, 2012.
Prieto-Barajas, C. M., Valencia-Cantero, E., and Santoyo, G.: Microbial mat ecosystems: Structure types, functional diversity, and biotechnological application, Electron. J. Biotechn., 31, 48–56, https://doi.org/10.1016/j.ejbt.2017.11.001, 2018.
Reid, R. P., Macintyre, I. G., Browne, K. M., Steneck, R. S., and Miller, T.: Modern marine stromatolites in the Exuma Cays, Bahamas: Uncommonly common, Facies, 33, 1–17, https://doi.org/10.1007/BF02537442, 1995.
Reid, R. P., Suosaari, E. P., Oehlert, A. M., Pollier, C. G. L., and Dupraz, C.: Microbialite Accretion and Growth: Lessons from Shark Bay and the Bahamas, Annu. Rev. Mar. Sci., 16, 487–511, https://doi.org/10.1146/annurev-marine-021423-124637, 2024.
Riding, R.: Calcareous Algae and Stromatolites, Springer Berlin, Heidelberg, 21–51, https://doi.org/10.1007/978-3-642-52335-9_2, 1991.
Riding, R.: Microbial carbonates: the geological record of calcified bacterial–algal mats and biofilms, Sedimentology, 47, 179–214, https://doi.org/10.1046/j.1365-3091.2000.00003.x, 2000.
Riding, R.: The Nature of Stromatolites: 3500 Million Years of History and a Century of Research, in: Advances in Stromatolite Geobiology, edited by: Reitner, J., Quéric, N.-V., and Arp, G., Springer, Berlin, Heidelberg, 29–74, https://doi.org/10.1007/978-3-642-10415-2_3, 2011.
Rishworth, G., Perissinotto, R., and Bornman, T.: Box 3. South African peritidal stromatolites. South African National Biodiversity Assessment 2018: Technical Report Volume 5: Coast, edited by: Harris, L. R., Sink, K. J., Skowno, A. L., and Van Niekerk, L., South African National Biodiversity Institute, Pretoria, 2019.
Rishworth, G. M., van Elden, S., Perissinotto, R., Miranda, N. A. F., Steyn, P.-P., and Bornman, T. G.: Environmental influences on living marine stromatolites: insights from benthic microalgal communities, Environ. Microbiol., 18, 503–513, https://doi.org/10.1111/1462-2920.13116, 2016.
Rishworth, G. M., Perissinotto, R., Bornman, T. G., and Lemley, D. A.: Peritidal stromatolites at the convergence of groundwater seepage and marine incursion: Patterns of salinity, temperature and nutrient variability, J. Marine Syst., 167, 68–77, https://doi.org/10.1016/j.jmarsys.2016.11.010, 2017.
Rishworth, G. M., Dodd, C., Perissinotto, R., Bornman, T. G., Adams, J. B., Anderson, C. R., Cawthra, H. C., Dorrington, R. A., du Toit, H., Edworthy, C., Gibb, R.-L. A., Human, L. R. D., Isemonger, E. W., Lemley, D. A., Miranda, N. A. F., Peer, N., Raw, J. L., Smith, A. M., Steyn, P.-P., Strydom, N. A., Teske, P. R., and Welman, S.: Modern supratidal microbialites fed by groundwater: functional drivers, value and trajectories, Earth-Sci. Rev., 210, 103364, https://doi.org/10.1016/j.earscirev.2020.103364, 2020.
Ritter, S. M., Schröter, M. I., Schröder-Ritzrau, A., Scholz, C., and Frank, N.: Geochemical Insights Into an Active Calcareous Tufa Depositing System in Southern Germany, Proced. Earth Plan. Sc., 17, 328–331, https://doi.org/10.1016/j.proeps.2016.12.083, 2017.
Rodríguez, S. and Cózar, P.: Descripción e interpretación de los afloramientos del Carbonífero Inferior en las proximidades de Peñarroya-Pueblonuevo (Córdoba, España), Coloq. Paleontol., no. 50, 161–200, ISSN 1132-1660, 1999.
Rodríguez-Berriguete, Á.: Early diagenetic features in Holocene travertine and tufa from a volcanic setting (Azuaje, Gran Canaria, Spain), Facies, 66, 17, https://doi.org/10.1007/s10347-020-00602-z, 2020.
Rodríguez Guitián, M. A., Real, C., Ramil-Rego, P., Franco, R. R., and Castro, H. L.: Characteristics, vulnerability and conservation value of active tufa-forming springs on coastal cliffs in the NW Iberian Peninsula, Ocean Coast. Manage., 189, 105122, https://doi.org/10.1016/j.ocecoaman.2020.105122, 2020.
Rodwell, J. S. (Ed.): British Plant Communities: Vol. 2: Mires and Heaths, Cambridge University Press, Cambridge, https://doi.org/10.1017/9780521391658, 1992.
Rogerson, M., Pedley, H. M., and Middleton, R.: Microbial influence on macroenvironment chemical conditions in alkaline (tufa) streams: perspectives from in vitro experiments, Geol. Soc. Lond. Spec. Publ., 336, 65–81, https://doi.org/10.1144/SP336.5, 2010.
Rong, K., Li, J., An, Y., and He, F.: A study of biogenesis of karst speleothem: an example from Zhijin Cave of Guizhou, Guizhou Geol., 13, 177–180, 1996.
Sanchez-Cabeza, J.-A., Masqué, P., Martínez-Alonso, M., Mir, J., and Esteve, I.: 210Pb Atmospheric Flux and Growth Rates of a Microbial Mat from the Northwestern Mediterranean Sea (Ebro River Delta), Environ. Sci. Technol., 33, 3711–3715, https://doi.org/10.1021/es9900205, 1999.
Shapiro, R.: A field guide to microbialites, Pac. Sect. Am. Assoc. Pet. Geol. Guideb., 99, 68–80, 2005.
Shapiro, R. S.: A Comment on the Systematic Confusion of Thrombolites, Palaios, 15, 166–169, https://doi.org/10.2307/3515503, 2000.
Shiraishi, F., Bissett, A., de Beer, D., Reimer, A., and Arp, G.: Photosynthesis, Respiration and Exopolymer Calcium-Binding in Biofilm Calcification (Westerhöfer and Deinschwanger Creek, Germany), Geomicrobiol. J., 25, 83–94, https://doi.org/10.1080/01490450801934888, 2008.
Smith, A. and Uken, R.: Living marine stromatolites at Kei River mouth, S. Afr. J. Sci., 99, 200, 2003.
Smith, A., Uken, R., and Thackeray, Z.: Cape Morgan peritidal stromatolites: The origin of lamination, S. Afr. J. Sci., 101, 107–108, 2005.
Smith, A., Cooper, A., Misra, S., Bharuth, V., Guastella, L., and Botes, R.: The extant shore platform stromatolite (SPS) facies association: a glimpse into the Archean?, Biogeosciences, 15, 2189–2203, https://doi.org/10.5194/bg-15-2189-2018, 2018.
Smith, A., Cooper, A., Guastella, L., and Bharath, V.: Wave influences in contemporary and Mesoarchaean stromatolites, Sediment. Geol., 396, 105558, https://doi.org/10.1016/j.sedgeo.2019.105558, 2020.
Smith, A. M., Andrews, J. E., Uken, R., Thackeray, Z., Perissinotto, R., Leuci, R., and Marca-Bell, A.: Rock pool tufa stromatolites on a modern South African wave-cut platform: partial analogues for Archaean stromatolites?, Terra Nova, 23, 375–381, https://doi.org/10.1111/j.1365-3121.2011.01022.x, 2011.
Stolz, J., Reid, R., Visscher, P., Decho, A., Norman, R., Aspden, R., Bowlin, E., Franks, J., Foster, J., Paterson, D., Przekop, K., Underwood, G., and Prufert-Bebout, L.: The Microbial Communities of the Modern Marine Stromatolites at Highborne Cay, Bahamas, Atoll Res. Bull., 567, 1–29, https://doi.org/10.5479/si.00775630.567.1, 2009.
Suosaari, E. P., Reid, R. P., Playford, P. E., Foster, J. S., Stolz, J. F., Casaburi, G., Hagan, P. D., Chirayath, V., Macintyre, I. G., Planavsky, N. J., and Eberli, G. P.: New multi-scale perspectives on the stromatolites of Shark Bay, Western Australia, Sci. Rep.-UK, 6, 20557, https://doi.org/10.1038/srep20557, 2016.
Suosaari, E. P., Reid, R. P., and Andres, M. S.: Stromatolites, so what? A tribute to Robert N. Ginsburg, Depositional Rec., 5, 486–497, https://doi.org/10.1002/dep2.72, 2019a.
Suosaari, E. P., Reid, R. P., Oehlert, A. M., Playford, P. E., Steffensen, C. K., Andres, M. S., Suosaari, G. V., Milano, G. R., and Eberli, G. P.: Stromatolite Provinces of Hamelin Pool: Physiographic Controls On Stromatolites and Associated Lithofacies, J. Sediment. Res., 89, 207–226, https://doi.org/10.2110/jsr.2019.8, 2019b.
Suosaari, E. P., Reid, R. P., Mercadier, C., Vitek, B. E., Oehlert, A. M., Stolz, J. F., Giusfredi, P. E., and Eberli, G. P.: The microbial carbonate factory of Hamelin Pool, Shark Bay, Western Australia, Sci. Rep.-UK, 12, 12902, https://doi.org/10.1038/s41598-022-16651-z, 2022.
Symoens, J.-J., Duvigneaud, P., Berghen, C. V., Dewit, J., and Kiwak, A.: Aperçu Sur La Végétation Des Tufs Calcaires De La Belgique, B. Soc. Roy. Bot. Belg., 83, 329–352, 1951.
Szulczewski, M.: Stromatolity jurajskie v Polsce, Acta Geol. Pol., 13, 1–99, 1968.
Trenhaile, A.: Rocky coasts – their role as depositional environments, Earth-Sci. Rev., 159, 1–13, https://doi.org/10.1016/j.earscirev.2016.05.001, 2016.
Trompette, R.: Upper Proterozoic (1800–570 Ma) stratigraphy: A survey of lithostratigraphic, paleontological, radiochronological and magnetic correlations, Precambrian Res., 18, 27–52, https://doi.org/10.1016/0301-9268(82)90035-3, 1982.
Valero Garcés, B. L., Moreno, A., Navas, A., Mata, P., Machín, J., Delgado Huertas, A., González Sampériz, P., Schwalb, A., Morellón, M., Cheng, H., and Edwards, R. L.: The Taravilla lake and tufa deposits (Central Iberian Range, Spain) as palaeohydrological and palaeoclimatic indicators, Palaeogeogr. Palaeocl., 259, 136–156, https://doi.org/10.1016/j.palaeo.2007.10.004, 2008.
Villafañe, P. G., Lencina, A., Soria, M., Saona, L. A., Gomez, F., Alonso, G., and Farías, M.: Las Quínoas oncoids: a new deposit of microbialites in the Salar de Antofalla (Catamarca, Argentina), Andean Geol., 48, 281–302, https://doi.org/10.5027/andgeoV48n2-3292, 2021.
Walter, M. R.: Stromatolites, 1st edn., Elsevier, Amsterdam, eBook ISBN 9780080869322, 1976.
Walter, M. R. and Heys, G. R.: Links between the rise of the metazoa and the decline of stromatolites, Precambrian Res., 29, 149–174, https://doi.org/10.1016/0301-9268(85)90066-X, 1985.
Walter, M. R., Bauld, J., and Brock, T. D.: Chap. 6.2 Microbiology and Morphogenesis of Columnar Stromatolites (Conophyton, Vacerrilla) from Hot Springs in Yellowstone National Park, in: Developments in Sedimentology, vol. 20, edited by: Walter, M. R., Elsevier, 273–310, https://doi.org/10.1016/S0070-4571(08)71140-3, 1976.
Warren, J. K.: The hydrological significance of Holocene tepees, stromatolites, and boxwork limestones in coastal salinas in South Australia, J. Sediment. Res., 52, 1171–1201, https://doi.org/10.1306/212F80F8-2B24-11D7-8648000102C1865D, 1982.
Weston, R.-L. A., Perissinotto, R., Rishworth, G. M., and Steyn, P.-P.: Macroinvertebrate variability between microhabitats of peritidal stromatolites along the South African coast, Mar. Ecol. Prog. Ser., 605, 37–47, https://doi.org/10.3354/meps12741, 2018.
Whitton, B. A., Gemmell, J. J., and Robinson, P. J.: Identification of Blue Green Algae of the British Isles: Guide to the Genera and Use of the Interactive CD-ROM for Identification of Species, Environment Agency, University of Durham, ISBN 1857051327, 2000.
- Abstract
- Introduction
- Defining rock coast microbialites through research approaches
- Describing and classifying rock coast microbialites
- An integrated geomorphological classification for rock coast microbialites
- Summary, integration of nomenclature, and recommendations for future work
- Data availability
- Author contributions
- Competing interests
- Disclaimer
- Acknowledgements
- Financial support
- Review statement
- References
- Abstract
- Introduction
- Defining rock coast microbialites through research approaches
- Describing and classifying rock coast microbialites
- An integrated geomorphological classification for rock coast microbialites
- Summary, integration of nomenclature, and recommendations for future work
- Data availability
- Author contributions
- Competing interests
- Disclaimer
- Acknowledgements
- Financial support
- Review statement
- References